Climate-smart approaches for enhancing livestock productivity, human nutrition, and livelihoods in low- and middle-income countries
Adegbola T. Adesogan A B * , Mulubrhan Balehegn Gebremikael C , Padmakumar Varijakshapanicker B D and Diwakar Vyas
A
B
C
D
Abstract
In low- and middle-income countries (LMIC), particularly in South Asia and sub-Saharan Africa, livestock production is dominated by smallholder production systems characterized by low productivity and high greenhouse gas (GHG) emissions intensity coupled with high vulnerability to climate change-related natural disasters. Yet, these countries lead the world in the future demand for livestock products. For instance, the projected growth in protein demand for red meat between 2020 and 2050 is greatest in south Asia (49%) and sub-Saharan Africa (55%) relative to global estimates (14%). Most LMIC aim to meet the increasing demand for meat and milk by increasing livestock numbers, which perpetuates the high GHG emissions intensity in these countries. Rather, emphasis should be on increasing productivity per animal through increased adoption of climate-smart interventions that sustainably increase productivity, efficiency and resilience. Such interventions must go beyond the current focus on reducing enteric methane emissions from intensive livestock production systems to include interventions that also improve adaptation to climate change, and that are appropriate for extensive smallholder livestock systems. Thus, additional factors such as affordability and socio-cultural appropriateness are particularly important determinants of adoption. We recommend the use of a systems lens to examine existing GHG mitigation strategies in terms of their efficacy as well as their support for adaptation to climate change, socio-cultural acceptability, and promotion of livestock’s contribution to food and nutritional security and livelihoods. Policy changes necessary to foster adoption of such climate-smart livestock production interventions in LMIC are discussed.
Keywords: adaptation, climate, food security, greenhouse gas, livestock, low- and middle-income countries, mitigation, smallholders.
Introduction
Livestock production plays a vital role in the economies of low- and middle-income countries (LMIC), serving as a source of income and livelihoods for 1.7 billion people (World Bank 2021). These regions often face challenges such as food and nutrition insecurity, particularly undernutrition. Animal-sourced foods (ASF), rich in essential nutrients such as protein, vitamins, and minerals, can help address these issues and contribute to public health and improved livelihoods.
Globally, close to 600 million poor people keep livestock (FAO 2018) as a direct or indirect source of their livelihoods. The contribution of livestock to the economies of LMIC ranges from 15% to 80% (Salmon 2018) through contributions to household income, farm productivity, women’s empowerment, and agricultural diversity, all of which are essential for improving nutritional outcomes (Jisso et al. 2022). In addition to being a vital source of macronutrients such as protein, polyunsaturated fatty acids, and energy, ASF provide a greater supply of the bioavailable micronutrients needed for healthy growth and development, especially in nutritionally vulnerable groups including infants, children under-five, and pregnant and lactating women (FAO 2023a). For example, the bioavailable iron provided by ASF can contribute to alleviating the global health problem of anemia, which affects an estimated 1.9 billion people (GBD 2021 Anaemia Collaborators 2023).
Livestock also provide 33% and 17% of the protein and calories consumed in LMIC respectively (FAO 2018). Yet, most (86%) of the feed consumed by livestock is sourced from fibrous feeds, which cannot be consumed by humans (Mottet et al. 2017). However, the importance of ASF in these contexts must be balanced with sustainability considerations to ensure that production practices are both environmentally responsible and socially equitable. In particular, the livestock sector in LMICs contributes significantly to greenhouse gas (GHG) emissions, with overgrazing and grazing land degradation posing a complex challenge that demands immediate attention. Approximately 42% of all livestock emissions are from upper-middle-income economies, 29% are from LMICs, 21% from high-income countries (HICs) and 7% from low-income economies (FAO 2023b). Because of lower productivity levels, LMICs have higher GHG emission intensities from livestock production than do HICs, which have significantly reduced these measures in recent decades (Fig. 1; FAO 2023b). Countries with the highest global GHG emissions from ASF contribute 57% of total food production emissions. Among these, LMIC regions such as South America (14%), South and Southeast Asia (9%), and nations such as China and Mongolia (8%), are the leading emitters of GHGs from ASF production (Xu et al. 2021). The importance of GHG mitigation in livestock production in these regions, and from LMIC in general, cannot be overstated, because it intersects with several issues including global climate change and environmental sustainability, food security, and economic development. Adoption of effective mitigation strategies is even more important because of the escalating demand for animal products and human populations in LMIC.
Beef greenhouse gas (GHG) emissions intensity per regions. Note: estimates are based on historical time series from the Food and Agriculture Organisation Statistics (FAOSTAT) Emissions Agriculture databases (see https://www.fao.org/faostat/en/#data/GT), which are extended with the Agricultural Outlook projections. CO2 equivalents are calculated using the global warming potential of each gas as reported in the Sixth Assessment Report (AR6) of Intergovernmental Panel on Climate Change (IPCC) (OECD/FAO 2022).
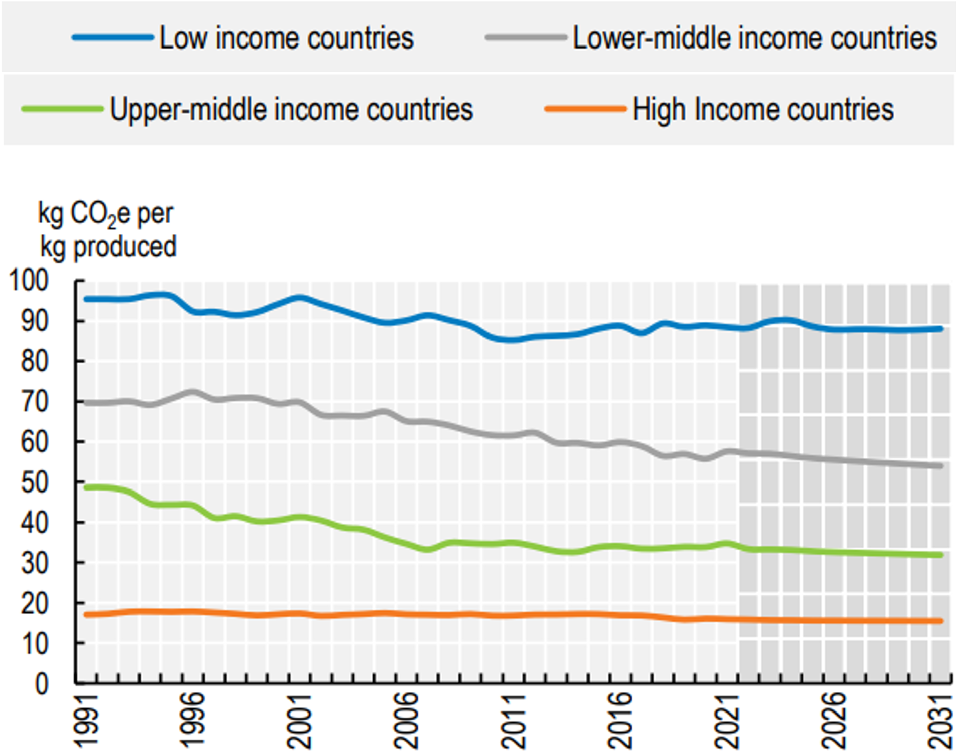
Climate change can affect livestock productivity by altering temperature and water availability, which in turn reduce forage production and cause direct physiological stress to animals, leading to decreased productivity and increased management costs (Henry et al. 2012). Livestock production systems in LMICs are especially vulnerable to these effects, with heat stress, GHG emissions, and drought posing significant threats to animal health, reproductive efficiency, behavior, and overall welfare (Thornton et al. 2022). Furthermore, climate change can negatively affect ASF quantity, safety and marketability through contamination with pathogens or pesticides, and reduced nutritional quality and sensory appeal, which ultimately result in increased prices and greater price volatility (Godde et al. 2021). Hence, LMICs must adopt climate-smart approaches for livestock (CSAL) not only to fulfill their commitments under the Paris Agreement and limit global temperature rise to below 2°C above pre-industrial levels, but also to avoid the negative effects of climate change on livestock production and food security. The objective of this paper is to explore CSAL that are particularly well-suited for LMICs, with a specific focus on smallholders, defined, according to FAO guidelines, as small-scale farmers and pastoralists managing land areas ranging from 1 to 10 ha.
Global appraisals of climate-smart approaches for livestock
Climate-smart approaches are practices that sustainably increase productivity, enhance resilience (adaptation), and reduce GHG emissions (mitigation), while enhancing national food security. These approaches, as defined by FAO (Elbehri et al. 2017), involve a range of strategies, including breeding more productive animals, improving diets to reduce emissions, better manure management, and enhanced feed quality and availability through various interventions. The goal is to achieve a balance between increased productivity, resilience to climate impacts, and reduced environmental footprint, thereby contributing to sustainable development and food security. Various authors have also cataloged several potentially effective CSAL (Hristov et al. 2013; Arndt et al. 2022; Beauchemin et al. 2022). However, selection of CSAL should consider various criteria to ensure technical effectiveness for emissions mitigation as well as adaptation to climate change, and account for the social, environmental, policy and biophysical factors. Yet, previous studies on CSAL have mostly focused on mitigation of GHG emissions from livestock systems, particularly in HIC. Some comprehensive reviews have appraised interventions for their ability to reduce total GHG emissions or GHG emissions intensity, while increasing or maintaining livestock productivity (Hristov et al. 2013; Arndt et al. 2022; Beauchemin et al. 2022), and these reviews are fundamental to understanding the relative efficacy and sustainability of various approaches for mitigating GHG emissions and reducing the global impact of livestock on the environment.
Arndt et al. (2022) conducted a meta-analysis of 98 mitigation strategies across 430 peer-reviewed studies and concluded that three key strategies, namely, increasing feeding levels, decreasing grass maturity, and lowering the dietary forage-to-concentrate ratio, reduced methane (CH4) emissions per unit of meat or milk by an average of 12% and increased animal productivity by a median of 17%. In addition, five strategies focused on reducing absolute emissions, namely CH4 inhibitors, tanniferous forages, electron sinks, oils and fats, and oilseeds decreased daily CH4 by 21%. Arndt et al. (2022) reported that full adoption of these strategies could help achieve the 1.5°C target by 2030, but not by 2050. However, they noted that many of these approaches, including reducing the dietary forage-to-concentrate ratio, using CH4 inhibitors, electron sinks, oils and fats, and oilseeds, are not well-suited for grassland-based livestock systems, which are predominant in many LMICs.
Beauchemin et al. (2022) reviewed the efficacy of several CSAL practices examined by the FAO Livestock Environmental Assessment and Performance (LEAP) Partnership (FAO 2022) and concluded that although several mitigation options exist, most are tailored to intensive production systems, which have been the primary focus of research. However, they noted that adoption rates have been low, emphasizing the need to prioritize affordability. Instead of recommending specific approaches, Beauchemin et al. (2022) outlined the potential benefits and drawbacks of various strategies, with the exception of production intensification strategies (improved feeding and management), which was recommended as the most immediate and broadly applicable method for reducing CH4 emissions intensity in livestock systems.
In a review titled ‘Carbon myopia’, Harrison et al. (2021) criticized the typical approach of focusing on technology applications when targeting CSAL for mitigating emissions, noting that without simultaneously addressing the benefits and trade-offs associated with economic, environment, and social aspects of proposed approaches, their adoption will likely remain very low. Harrison et al. (2021) reviewed 54 CSAL for mitigating emissions from the livestock sector on the basis of their efficacy at reducing CH4 emissions or intensity as well as their environmental, economic, and social impacts and reported that globally, only 16 of the 54 approaches evaluated had medium to high mitigation potential as well as triple-bottom line benefit (economics, environment, and social) for emissions mitigation; only 7 of 54 were considered appropriate for LMIC (concentrate supplementation, improved animal health, improving animal management, pasture management, grazing management, agroforestry, and manure management). The study of Harrison et al. (2021) is one of the few studies that used a more holistic approach for evaluating CSAL, but it focused on mitigating GHG alone, and hence did not cover adaptation to climate change, which is a pressing need in LMIC. On the basis of these findings, the Food and Agricultural Organization (FAO 2023b) reviewed the global emissions reduction or mitigation potential of various ‘low-emission’ CSAL and reported that, in order of magnitude, improving productivity (20%), feed and nutrition improvements (12%), improving animal health (10%), and breeding (8%) were the top four strategies. As with several of the previous reviews, the focus was on mitigation of emissions alone, underscoring the need for future studies to integrate adaptation strategies alongside mitigation efforts to address the complex challenges faced by LMIC.
Most of the technological interventions to improve productivity and reduce GHG emissions that work well with higher-producing animals are impractical, unprofitable, or unaffordable when applied to low-producing local breeds in smallholder production systems in LMICs. These breeds, which are often labeled as having ‘low genetic merit’ on the basis of their performance in intensive production systems, may actually possess valuable traits such as disease resistance, climate tolerance, and adaptability to low-input systems, making them suitable for extensive or small-scale production contexts. The term ‘low genetic merit’ requires careful definition, because it is context-dependent and shaped by the goals of production systems, whether focused on maximizing output or preserving biodiversity and the viability of endangered breeds.
Consequently, the technologies that currently have the greatest potential to reduce GHG emissions from livestock that target rumen manipulation (macroalgae, 3 nitroxypropanol, yeasts, essential oils, ionophores, electron acceptors and direct-fed microbials), or early life mitigation (defaunation, immunization against methanogens, phage therapy, biochar, methane oxidizing devices), are unsuitable for LMIC on the basis of their high cost and inaccessibility to smallholders in such countries (Lovett et al. 2006). Similarly, breeding technologies such as estrus synchronization, induced ovulation and artificial insemination have failed in many countries because of rising costs and low success rates (Omondi et al. 2017).
Reisinger et al. (2021) proposed that key emerging GHG mitigation techniques for livestock, such as CH4 inhibitors, CH4 vaccines, breeding for low CH4 production, and macroalgae, hold significant potential for emissions reductions (20–30%, up to 30%, up to 15%, and 20–50% respectively) and are expected to achieve widespread commercial availability between 2030 and 2050 (Almeida et al. 2021; Honan et al. 2021). However, it is unlikely that these approaches will be readily available and affordable in LMIC in the short to medium term.
Neglected pillars of climate-smart approaches
As noted above, most previous reviews on CSAL have targeted GHG mitigation approaches as they were designed for intensive systems in HIC. Much less attention has been paid to adaptation and food security, which are similarly important pillars of CSAL (Van Wijk et al. 2020). Food security and adaptation to climate change are more urgent needs by smallholders in LMIC than GHG mitigation. Such countries, particularly those located in sub-Saharan Africa, South Asia, and Southeast Asia, have the highest levels of undernutrition globally. For instance, more than one in three children in most parts of sub-Saharan Africa and South Asia is stunted and southern Asia has the highest prevalence of wasting globally (UNICEF/WHO/World Bank Group 2023).
In recent years, smallholders in these regions of the world have also been ravaged by numerous climate-related disasters including floods, droughts, and locust invasions. Longer and harsher droughts and heat waves in Zambia, Malawi, Mozambique, and Zimbabwe have caused enormous crop losses. Earlier last year, because of crop losses of up to 60%, the Zambian government declared a state of national disaster (AGRA 2024). Ethiopia continues to experience the effects of a prolonged drought following five consecutive failed rainy seasons (OCHA 2023). Consequently, 11 million people are food insecure and about 6.85 million livestock have died since 2021 (OCHA 2023). South Asian countries, including India, Pakistan, Sri Lanka, Bangladesh, and Nepal, are highly vulnerable to climate change-induced extreme weather events, including heat waves, floods and droughts (Wester et al. 2019; Rehman et al. 2024). Frequent flooding in these areas results in loss or displacement of livestock, loss of feed and grazing areas, disease, and parasite outbreaks, leading to livelihood disruption among smallholders. Smallholder farmers in such areas need strategies to help livestock cope with such shocks and governments need evidence-based strategies to prioritize for their Nationally Declared Contributions.
This underscores the critical need to incorporate measures of adaptation as well as food security in evaluations of the appropriateness of CSAL in LMIC. Yet, very few of the published reviews of CSAL for livestock have discussed contributions to climate adaptation and food security of potential mitigation approaches. One of the few exceptions that accounted for these three factors was a review in the FAO sourcebook (FAO 2013). These authors emphasized the importance of targeting climate mitigation and adaptation as well as food security and classified proposed CSAL into those appropriate for land-based, mixed, and landless livestock systems. The most promising approaches that addressed these three areas for land-based extensive systems included improving animal health, animal breeding, animal and herd management, agroforestry practices, and supplementary feeding. For mixed systems, the most promising approaches included crop residue management, nutrient management, soil management, feed management, and livestock management. Whereas, for landless systems, the most promising approaches were anaerobic digesters, improved feeding management, and disease surveillance. The FAO sourcebook (FAO 2013) took a global approach to evaluating CSAL options, but did not distinguish between those appropriate for HIC versus LMIC. Given that smallholders in LMIC generally have low-income levels and live in rural areas often with poor road infrastructure, the availability, affordability, and accessibility of potential interventions are determinants of adoption of CSAL. In addition, socio-cultural factors, including religion, culture, and gender norms determine the appropriateness and acceptability of interventions. Therefore, the socio-cultural fit of interventions must also be considered.
Van Wijk et al. (2020) evaluated 15 climate-smart agriculture (CSA) assessment frameworks for suitability to cover biophysical and socio-economic aspects for LMIC smallholders and reported that, in general, GHG mitigation was analyzed well but few tools went beyond GHG to truly analyze environmental sustainability (e.g. effects on water quality, soil health, ecosystem services). Further, livestock productivity was analyzed well with biophysical metrics but social (e.g. food security, gender) and economic (poverty) aspects were largely ignored, and climate change adaptation was the CSAL pillar with the weakest representation. This study and the previous section highlight the urgent need to characterize CSAL for LMIC smallholders in terms of their support for adaptation to climate change, as well as environmental sustainability, economic and socio-cultural appropriateness in addition to their efficacy at mitigation of GHG emissions.
Proposed climate-smart approaches for smallholders in LMIC
To select the most appropriate climate-smart approaches for LMIC, we reviewed the list of interventions discussed in previous studies (Harrison et al. 2021; Beauchemin et al. 2022), and evaluated each one on the basis of their potential to reduce GHG emissions and emissions intensity, to increase animal productivity, their affordability and accessibility and socio-cultural fit, and their ability to increase adaptation to climate change. The non-exhaustive list of climate-smart interventions proposed for smallholder livestock producers are described below. Additional options are presented in Table 1.
Technology/practiceA | Effect | Regions/countries with some successful examples | Adoption constraintsB | Adoption levelC | References | ||||||
---|---|---|---|---|---|---|---|---|---|---|---|
Cost | Technical complexity and/or understanding | Labor intensiveness | Input availability/accessibility | Risk | Returns (awareness) | ||||||
Feed technologies | ** | ** | ** | ** | ** | ** | |||||
Urea–ammonia treatment of crop residues | Increased digestibility and protein content | China, South and Southeast Asia, East Africa | ** | ** | ** | ** | ** | Low | Singh et al. (1993), Owen et al. 2012, Chander (2010), Mudzengi et al. (2014) | ||
Enzyme or white rot fungi treatment of crop residues | Improved cell wall and protein digestibility | South Asia | * | * | ** | * | * | * | Low | Owen et al. (2012), Sarnklong et al. (2010) | |
Reducing crop residue particle size | Reduced sorting, increased feed intake | West Africa, East Africa, South and Southeast Asia | ** | ** | ** | ** | * | * | Moderate | Hou et al. (2019), Kaur et al. (2022) | |
Stall feeding/ad libitum feeding | Selection of most digestible forage fractions | South Asia, East Africa, Latin America | ** | * | * | ** | * | * | Moderate | Owen et al. (2012) | |
Urea supplementation | Meet microbial and animal N needs | South America, South Asia | * | ** | *Storage issues, making the block | * | Low to moderate | Owen et al. (2012) | |||
Urea–molasses multinutrient block supplementation | Meet microbial and animal nutrient needs | South and Southeast Asia, East, North and West Africa | ** | * | ** | * | Moderate to high | Owen et al. (2012), Nimbalkar et al. (2022), Khan et al. (2007), Khanum et al. (2010) | |||
Vitamin–mineral supplementation | Improved health, reproduction, and performance | South and Southeast Asia, East and West Africa South America | ** | ** | * | ** | * | Moderate | Owen et al. (2012), Hundal et al. (2016), Amuge and Osewe (2017), Bekele et al. (2019) | ||
Food–feed crop systems (breeding food–feed forages and integrating feed and forage production) | Improved forage and crop yields, more digestible straw/stover | South Asia, Southeast Asia | * | ** | * | * | * | Moderate | Owen et al. (2012) | ||
Under-exploited feeds | Improved nutrient supply | South and Southeast Asia, East Africa, North Africa, West Africa, South and Central America | ** | ** | ** | ** | * | ** | Low | Owen et al. (2012), Makkar (2018) | |
Pasture improvement and reclamation | Restoration of degraded areas, improved fodder and nutrient supply | South and Southeast Asia, East Africa, North Africa, West Africa, South and Central America | ** | ** | ** | * | * | Low, moderate in South or Central America | Owen et al. (2012) | ||
Silvopasture and agroforestry systems | improved fodder and nutrient supply | South and Central America, East Africa | ** | ** | ** | ** | * | ** | Low to moderate | Frey et al. (2012), Owen et al. (2012), Jha et al. (2021), Zabala et al. (2022) | |
Forage production (including trees, forbes and shrubs) | Increased yields and quality; protein bypass potential of tanniferous forages | South and Southeast Asia, East Africa, North Africa, West Africa, South and Central America | ** | ** | ** | ** | * | ** | Low to moderate | Peters et al. (2001), Owen et al. (2012), Amuge and Osewe (2017), Bekele et al. (2019) | |
Forage conservation as hay or silage | Allows storage of feed for dry or cold seasons | Central America, East Africa. South Asia | * | ** | * | ** | * | Low (silage) Low (hay) | Owen et al. (2012), Amuge and Osewe (2017) | ||
Ration formulation | South and Southeast Asia, East Africa, South Central America | Ensures matching of animal nutrient needs to dietary supply | * | ** | ** | ** | ** | ** | Low to moderate | Amuge and Osewe (2017), Manzana et al. (2014), Ngeno (2024) | |
Breeding and reproduction technologies | |||||||||||
Artificial insemination and embryo transfer | Accelerated genetic gain via the use of superior sires/embryos | South America (Brazil Argentina, Paraguay, Uruguay) | * | ** | * | * | ** | ** | Low to (moderate for dairy cows, low for beef cows) | Ayalew et al. (2003), Kosgey and Okeyo (2007), Rege et al. (2011), Mushonga et al. (2017), Ojango et al. (2017), Mwanga et al. (2019), Baruselli et al. (2018), Baruselli et al. (2019) | |
Estrous synchronization | Early breeding of heifers and cows; facilitates operational programming and management | South America (Brazil Argentina, Paraguay, Uruguay) | ** | ** | ** | ** | ** | ** | Low for beef and dairy cows | Kosgey and Okeyo (2007), Ojango et al. (2017), Mwanga et al. (2019), Baruselli et al. (2018), Baruselli et al. (2019) | |
Crossbreeding | ** | ** | ** | ** | ** | ** | Low in sub-Saharan Africa | Ojango et al. (2017), Mwanga et al. (2019), Camara et al. (2019) | |||
Marker-based/genomic selection | ** | * | ** | ** | * | Low | Marshall et al. (2011) | ||||
Health technologies | |||||||||||
Biosecurity measures | Protect against infectious diseases | China, South and Central America | ** | * | ** | ** | * | Low | Young et al. (2015), Lestari et al. (2022), Li et al. (2023) | ||
Vaccination | Disease prevention | South and Central America | * | * | ** | ** | * | Low | Donadeu et al. (2019), Simiyu et al. (2022), Robi et al. (2024) | ||
Deworming/anthelmintics | Reducing parasite burden | South and Southeast Asia, West, East, and South Africa, South and Central America | * | * | ** | ** | * | Low to moderate | Odoi et al. (2007), Gray et al. (2012), Okello et al. (2021), Rahman et al. (2023), Thirunavukkarasu et al. (2022) | ||
Acaricides | Reducing tick burdens | South and Southeast Asia, West, Each, and South Africa, South and Central America | Low to moderate | Odoi et al. (2007), Thirunavukkarasu et al. (2022), Paucar-Quishpe et al. (2024) |
Sustainable intensification
In LMIC, one of the main reasons for high GHG emissions from livestock is the high number of low-producing animals with much greater emission intensities than in HIC. The urgent imperative for sustainable intensification in LMIC is highlighted by the escalating demand for animal protein in these countries. For instance, the projected growth in protein demand for red meat between 2020 and 2050 is greatest in South Asia (49%) and sub-Saharan Africa (55%) relative to global estimates (14%) (Komarek et al. 2021). Both of these regions also have the greatest projected demand growth for dairy products by 2030 (OECD/FAO 2022). These regions aim to meet the increasing demand for both dairy products and meat by increasing herd numbers and sizes, which will compound GHG emission inventories. Rather, the rising demand should be met with fewer more productive animals, such that emissions from numerous less productive animals will be avoided, while improving food security.
Sustainable intensification can be achieved through an integrated strategy of improved diet and farm management and improved health and breeding (Beauchemin et al. 2020), while reducing the number of herds and less/non-productive animals within herds. Improving feeding is the first priority for several reasons; first, proper feeding is vital to improve livestock productivity and health and feed production. Second, feed interventions have greater global emissions mitigation potential than do other individual approaches (FAO 2023b), partly because enteric fermentation and manure management account for 41% of total GHG emissions from agriculture (Steinfeld et al. 2006; Dillon et al. 2021). Third, along with water, lack of feed is perhaps the primary constraint to livestock production during climate-induced natural disasters. Finally, feed costs account for up to 70% of the total variable costs of livestock production, (Makkar 2016a) and, hence, improved feeding strategies may improve sustainability by reducing production costs. Substantial improvements to feeding ruminant livestock can be achieved through the following strategies, many of which also contribute to improved adaptation to climate change and food security.
Higher dietary roughage to concentrate ratios result in significantly higher enteric CH4 emissions by ruminants (Kumar et al. 2013). For instance, CH4 emissions were higher in continuous culture when a 70% forage diet was fed instead of 50% or 30% forage diets (Eun et al. 2004). Reducing the forage to concentrate ratio reduces structural carbohydrates such as cellulose, hemicellulose and lignin in the diet and increases sugars, starch, and highly fermentable fiber, which favors propionate production and reduces CH4 emissions (Janssen 2010).
Beauchemin and McGinn (2005) reported that increasing the proportion of concentrates in animal diets can reduce enteric CH4 emission by 3–6.5%. Generally, concentrates rich in soluble starch, such as maize, barley and wheat, are more effective than fibrous concentrates at increasing propionate production (Martin et al. 2010). In this context, it is worth mentioning that a diet with corn as concentrate has the advantage of improved milk protein and fat concentration and reduced CH4 emission compared with those with other cereal grains as concentrate (Ramin et al. 2021). Lower proportions of structural carbohydrates and faster rate of passage of grains shift the fermentation pathway towards more propionate production with less CH4 (Johnson and Johnson 1995). However, increasing the level of concentrates above certain levels may also reduce fiber digestibility, causing an overall reduction in productivity and increase in CH4 emissions (Lee et al. 2012). This risk is low on smallholder farms in LMIC, where feeding of high forage or crop residue diets with or without cereal bran or legume supplements is typical.
It is noteworthy that reducing the roughage to concentrate ratio will reduce CH4 emissions only if dry matter intake is not increased (Vyas et al. 2018). In addition, when switching from fiber-rich diet to starch-and protein-rich concentrate diet, care should be taken to ensure that there is no overall increase in fossil CO2 production (FAO 2022). This can be addressed by using food waste, byproducts and grains not fit for human consumption as concentrates to the maximum extent possible (Ominski et al. 2021). Reducing the proportion of forage in livestock diets must be approached cautiously to avoid increasing food–feed competition, which could exacerbate unsustainable resource use. As outlined by van Hal et al. (2019), prioritizing the use of low-opportunity-cost feedstuffs (LCFs) such as crop residues, food processing by-products, and biomass from non-arable land is essential to minimizing competition with human-edible food sources. These LCFs not only recycle nutrients into the food system but also reduce the reliance on arable land for feed production, thereby aligning with the principles of a circular food system (Van Zanten et al. 2019). For instance, the incorporation of by-products such as wheat middlings, sugar beet pulp, or spent grains into livestock diets transforms otherwise wasted biomass into valuable animal-source foods without displacing human food crops. Moreover, innovative approaches such as improving the digestibility of poor-quality forages by using biological or chemical treatments further enhance the viability of LCFs, as they can be efficiently converted by ruminants into high-quality protein and nutrients (van Hal et al. 2019). Expanding on these strategies ensures that reductions in forage do not compromise sustainability but instead promote the efficient use of resources, ultimately contributing to a more resilient and resource-efficient food system. Additional emphasis on developing feed formulations that optimize the use of non-human-edible inputs while supporting livestock productivity is critical to achieving these goals.
Improving digestibility of roughages is a feasible option to improve livestock productivity and reduce CH4 emission for smallholder producers in LMIC. Blaxter and Clapperton (1965) showed that feeds with higher digestibility result in a less CH4 produced per unit of feed consumed.
Further, feeding nutritionally upgraded crop residues to reduce livestock GHG emissions is a more sustainable alternative than leaving them in situ in landfills or burning them (Ortiz-Gonzalo et al. 2017). A 5% increase in organic matter digestibility of straws/stovers, produces 0.2 Mcal of additional metabolisable energy (ME) per kilogram DM (Hamid et al. 2007). ’Thus, improving the digestibility of 1000 kg of straw by 5%, will supply additional 200 Mcal ME for milk production by a lactating cow. This implies the cow could consume 125 kg less straw (200 Mcal/1.6 Mcal ME present in a kg of straw) and still produce the same quantity of milk. When 1 kg of straw undergoes rumen fermentation, it produces about 22.87 L of CH4 (Singh and Mohini 1999). Therefore, improving the digestibility of 1000 kg of straw by 5% reduction could reduce straw intake by 125 kg and could reduce CH4 production by 2857 L. Given that up to 70% of feed consumed in certain developing countries is straw and stover, a 5% improvement in digestibility of straws/stover can avoid production of a considerable quantity of CH4. The quality and digestibility of low-quality roughages can be improved by the following methods.
Crop residues or other fibrous feeds differ in their digestibility and, consequently, the quantity of enteric CH4 produced when they are consumed by ruminant livestock (Giger-Reverdin and Sauvant 2000). Breeding programs have leveraged the inherent variation among cultivars of food crops and their residues. For instance, cultivar-dependent differences for in vitro organic-matter digestibility exceeding 5% have been reported for rice (Subudhi et al. 2020), maize (Anandan et al. 2013) and sorghum (Blümmel and Rao 2006). Crop or forage cultivars with higher non-structural carbohydrate contents have greater digestibility and less CH4 production. Further, among structural carbohydrates, cellulose produces three times more CH4 than does hemicellulose (Moe and Tyrrell 1979). Breeding programs that target higher-digestibility forage cultivars or crop residues, owing to higher non-structural to structural carbohydrate or hemicellulose to cellulose ratios, can increase livestock productivity, while reducing CH4 emissions. Greater straw and stover digestibility can be achieved through selection for brown midrib varieties or those low in lignin and phenolic acids such as ferulic acid (Casler and Jung 2006; Sattler et al. 2014).
Crop breeding can also advance climate adaptation and resilience when traits such as drought, pest, disease, or flooding are targeted (Hart et al. 2022). In addition, recent technologies such as gene editing and CRISPR-Cas9 can be used to advance crop breeding goals. However, such improved varieties must be adapted to the target growing conditions. Even when these conditions are met, adoption of new cultivars is often low among smallholders in LMIC, necessitating free or subsidized provision of seeds or planting materials initially. Such subsidies should be withdrawn overtime to foster development of thriving seed systems in such countries, with the caveat that adoption happens in a self-sustaining mode without any external support.
Given the abundance of crop residues (Fig. 2a, b), about 5 teragrams produced globally (Smil 1999), and the fact that livestock rations in LMICs are composed of up to 70% poor-quality feeds (FAO 2014), which increase GHG emissions when ingested or burned, implementing effective strategies to enhance their nutritional value and make them readily adoptable by smallholders could significantly improve livestock productivity and reduce GHG emissions globally. Different physical, chemical, and biological techniques can break down the lignocellulose biomass (Sarnklong et al. 2010), which contributes to CH4 emissions. Ammoniation and alkali treatment of roughages reduce methanogenesis by enhancing digestibility (Beauchemin et al. 2008). For example, using the estimation methods of the the Intergovernmental Panel on Climate Change (IPCC), CH4 emissions in China were found to be 26–30% lower for cattle fed treated straw residues than for those fed untreated straw residues, due to the improved digestibility (Dong et al. 2004). Additionally, treatment of crop residues with fungi increased the in vitro degradability of biomass in the rumen by up to 100%, depending on the fungus and substrate (Tuyen et al. 2013). Some second-generation biofuel technologies, such as steam explosion ammonia fiber expansion (AFEX), and two-chemical combination treatment (2-CCT), effectively disrupt the lignin–hemicellulose–cellulose matrices in forages. Increases in true in vitro organic-matter digestibility (IVOMD) of straw after 48 h of incubation were highest with 2-CCT treatment (38.2 percentage units), followed by AFEX (19.3 percentage units) and steam treatment (8.9 percentage units) (Blümmel et al. 2019). The 2-CCT treatment increased the average apparent and true IVOMD of straw to over 80% and 90% respectively. However, uptake of most chemical and biological treatment technologies by smallholders is low for economic, accessibility and socio-cultural reasons (Owen and Jayasuriya 1989). Urea treatment is theoretically the most compelling poor-quality forage treatment method for smallholders because of the efficacy, relative availability and affordability and the potential improvement to the ration. Nevertheless, adoption levels are generally low and, among straw treatment methods (Table 1), only particle size reduction via chopping has been moderately adopted for upgrading poor-quality residues (Owens et al. 2012). More research on affordable, accessible, and effective treatments that are a good fit for smallholders is needed.
(a) Cereal residues used for animal feed/bedding in the period 1997–2021 (adapted from Smerald et al. (2023)). (b) Regional analysis of crop residue production and utilization trends over time (adapted from Smerald et al. (2023)).
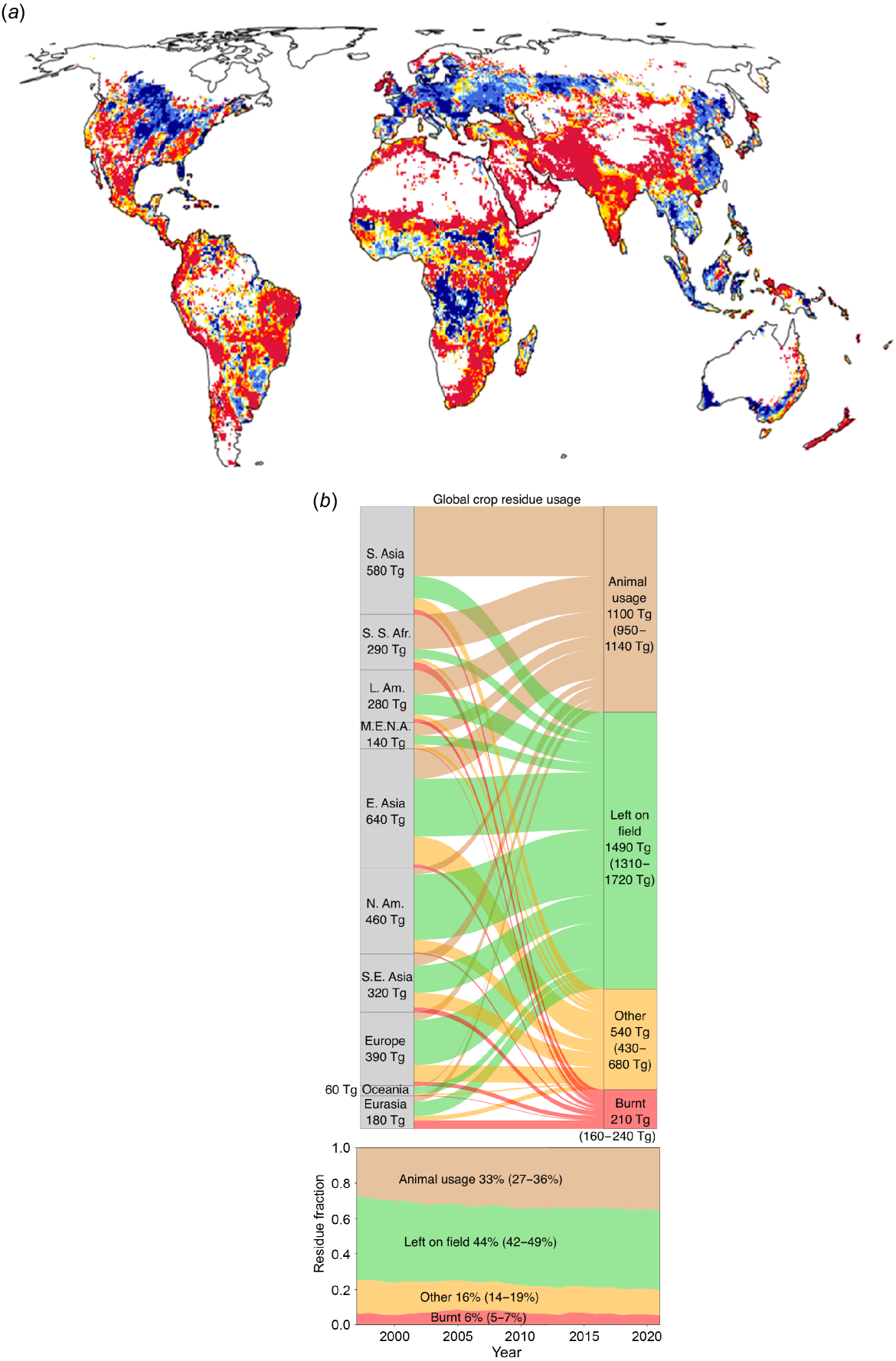
Feeding animals with balanced diets, including protein, energy and minerals and vitamins, results in improved feed consumption efficiency and reduced enteric CH4 production (Kannan et al. 2011). Ration balancing shifts the rumen fermentation more towards propionate and microbial protein production, resulting in reduced enteric CH4 emission (Valli 2020). In most small-scale production systems in LMICs, livestock feeding is largely constrained by resource availability, with animals primarily consuming on-farm feedstuffs that come at little to no cost. In high-yielding animals, these basal rations may be supplemented with homegrown or purchased concentrates. However, despite producers’ efforts to optimize feeding within their means, access to reliable information and tools for precise ration formulation remains a challenge (Balehegn et al. 2020). In many cases, nutrient requirement estimates are based on values established for Western breeds, which may not accurately reflect the needs of indigenous tropical and subtropical breeds (Valergakis et al. 2008). Some tropical countries, such as India, have adapted feeding standards specifically for tropical breeds (ICAR 2013), providing a model that other regions could follow to improve the accuracy of nutritional recommendations while working within existing constraints.
Diets that are inadequate in protein compromise animal productivity as protein is usually the first limiting nutrient in livestock fed poor-quality rations in LMIC. However, rations that contain excess protein can contribute to nitrous oxide (N2O) pollution. Balancing rations for net or metabolizable protein or at least crude protein is an important strategy for reducing excess N excretion, and N2O pollution through manure. Dietary synthetic proteins or amino acids can help reduce the excess protein intake (Ferket et al. 2002), but such strategies are typically too expensive for smallholder farmers. Rather, the target should be to optimize N intake using a balanced ration containing a combination of affordable supplementary N sources. In LMICs, some underexploited supplementary N sources include legumes, urea, insects such as black-fly larvae, and fish waste. Garg et al. (2012) demonstrated that ration balancing for dairy cows and buffaloes for calcium (Ca) and phosphorus (P) increased milk yield by up to 15%, as well as increasing farmers’ income in India. These authors also showed that balancing rations increase milk yield by 2–14%, while reducing enteric CH4 emissions by 15–20%, and reducing emissions intensity of milk by 13.5%, which is associated with reduction of 10.3% of gross energy lost through CH4 (Garg et al. 2013).
Indigenous livestock have thrived in marginal environments of the developing world owing to their adaptive traits, which confer resilience to climate, disease, insect pests, and other stressors. However, few studies have explored the potential of indigenous animals in LMICs for traits such as improved disease resistance, insect resistance, heat tolerance, and digestive efficiency (Burns et al. 1997; Prayaga et al. 2006). Instead, most breeding efforts in LMICs have focused on introducing exotic genetics to maximize productivity. In Kenya, the replacement of indigenous breeds with exotics often results in production losses and death owing to climate stresses and disease (Munywoki 2021). Similarly, in Ethiopia, genetic improvement through the importation of exotic genetics, artificial insemination, and crossbreeding has generally been shown to be unsustainable, leading to a shift in focus towards improving locally adapted genetic resources (Haile et al. 2019).
Indigenous livestock not only play a crucial role in maintaining genetic diversity for future breeding programs but also serve as a valuable genetic reservoir for traits that enhance climate resilience, and disease resistance, and overall animal health (Mwai et al. 2015). The loss of these breeds through poorly planned crossbreeding and replacement with exotics risks eroding unique genetic attributes before they are fully characterized. Therefore, maintaining indigenous breeds is essential for long-term livestock adaptation strategies in the face of climate change and evolving disease threats (Mwai et al. 2015). Beyond their genetic value, many indigenous breeds hold significant cultural and socio-economic importance. In pastoralist and smallholder farming communities, cattle and small ruminants often serve as symbols of wealth, social status, and heritage, playing central roles in ceremonies, marriages, and traditional exchanges (Son et al. 2019). Any selection or breeding efforts must recognize these social roles to ensure community acceptance and sustainability. Neglecting the cultural context of livestock production can lead to resistance from farmers and hinder the adoption of improved breeding strategies.
The failure of centralized nucleus breeding schemes and crossbreeding programs for smallholders in LMICs has led to the piloting of community-based breeding programs in several African, Latin American, and Asian countries (Haile et al. 2023). These programs can be constrained by lack of long-term national funding, weak institutionalization, and reliance on the researcher initiators for keeping the momentum (Wurzinger et al. 2021). Nevertheless, they are promising approaches for integrating community goals while setting breeding objectives, with their focus on organized animal identification and recording of performance and pedigree data. Such programs can leverage technologies such as SNP genotyping, sequencing, and epigenetics to advance breeding goals. They have also led to the retention of ‘best of stock’ breeding lambs and kids that were previously sold and slaughtered, improved animal performance, reduced mortality, and higher market prices (Haile et al. 2019).
Apart from improving productivity, breeding can also be attempted to improve adaptation, which includes thermotolerance and disease resistance. Strategies that target improved animal health are essential for ensuring livestock wellbeing and performance. By increasing the longevity of animals in the herd, these strategies enhance lifetime productivity and thereby reduce GHG emissions intensity (Von Soosten et al. 2020). Similarly, avoiding the need for mortality-induced replacement animals reduces GHG emissions. In fact, high mortality rates along with poor-quality feed have been described as the main causes of high GHG emissions in South Asia (Gerber et al. 2013). Further, the link between livestock disease and GHG emissions is greater in low-income countries; for instance, a disease outbreak affecting 20% of a beef cattle herd was associated with a 60% increase in GHG emissions versus a 42% increase for HIC (Oxford Analytica 2023). In these contexts, improving disease surveillance capacity, vaccination, parasite control and adequate biosecurity measures are important CSAL. However, many of these practices are too expensive for smallholder farmers in LMIC, necessitating initial subsidy programs, which should be replaced by a fee for service model over time to ensure sustainability. The reader is referred to Table 1 and the discussion in the next session on adoption of specific animal health, breeding and assisted technologies for more information.
A circular food system involves practices and technologies that minimize the input of finite resources, encourage the use of regenerative alternatives, prevent the leakage of natural resources from the food system, and stimulate the reuse and recycling of inevitable resource losses in a way that adds the highest possible value to the food system (Jurgilevich et al. 2016). In LMIC, crop production has long been integrated with animal production, particularly ruminant production. Most smallholder ruminant producers use mixed farming systems, in which residues of crops (straws, stovers, haulms) are the basal diet of animals, with other agricultural by-products (oil cakes, bran, rice polish) as supplements. In turn, ruminant manure is used to augment crop production in such countries. Without proper checks and balances, the growing demand for ASF may shift LMIC livestock production systems towards unsustainable intensification with improved breeds of exotic animals and increased use of imported feeds. To address this challenge, it is essential to target underexploited nutrient-dense feeds such as crop and animal byproducts, legumes and grasses, seaweed, single-cell proteins, and insects that are not consumed as food as animal feeds (Makkar 2018). These feeds can help close nutrient cycles, recycle nutrients, and increase the overall efficiency of food systems, reducing feed–food competition and aligning with circular food system objectives.
Another area of a circular bioeconomy is to reduce feed and food waste and loss, which necessitates more feed or food production, and hence greater GHG emissions. In sub-Saharan Africa (SSA), annual post-harvest losses are estimated to exceed US$4 billion, equating to 37% of the total production (World Bank 2011), with significant challenges stemming from inadequate infrastructure, lack of regulatory frameworks, and insufficient public awareness regarding food waste management (FAO 2023b). Strategies to mitigate food loss and waste (FLW) in these regions include improving post-harvest handling and storage technologies, establishing regulatory policies that promote food redistribution and recycling, and enhancing composting and biogas production systems. Additionally, fostering public–private partnerships can support the adoption of sustainable waste management practices, such as using food scraps for animal feed or energy recovery through anaerobic digestion. These strategies, if effectively implemented, could reduce environmental impacts, improve food security, and contribute to meeting the sustainable development goals (Mmereki et al. 2024).
Using food waste as animal feed represents a valuable opportunity to enhance the sustainability of livestock production, while addressing feed–food competition. Annually, approximately 931 million megagrams of food waste is generated globally, with a significant portion suitable for conversion into animal feed (UNEP Food Waste Index Report 2021). Redirecting even a fraction of this waste could substantially reduce the pressure on arable land, as it is estimated that up to 30% of agricultural land is used to produce food that is ultimately wasted (FAO 2013). Moreover, recycling food waste as animal feed can lower CH4 emissions from landfills, where decomposing organic waste contributes approximately 20% of global CH4 emissions (IPCC 2019). Economically, utilizing food waste for feed reduces input costs for farmers, especially in low-resource settings, where feed expenses can account for up to 70% of total livestock production costs (Makkar 2016b). Nutritionally, processed food waste can offer a viable and nutrient-rich alternative, supporting livestock health and productivity, while reducing reliance on conventional feed resources. By implementing strict safety and quality control measures, such as heat treatments and microbial analysis, potential risks such as contamination can be mitigated, making food waste a safe and sustainable feed source for diverse livestock systems. These efforts not only promote a circular bioeconomy but also align with global goals to minimize waste and maximize resource efficiency. This practice reduces the pressure on arable land needed for growing feed crops, thereby mitigating feed–food competition and promoting resource efficiency. Additionally, it minimizes the environmental impact associated with food waste disposal, such as CH4 emissions from landfills. Recycling food waste into animal feed also provides a cost-effective alternative for livestock producers, particularly in low-resource settings, by converting otherwise discarded materials into a valuable input. Furthermore, this approach can improve nutrient recycling within agricultural systems, contributing to a circular bioeconomy that aligns with sustainable development goals. By implementing robust safety protocols to address potential risks, such as contamination, food waste can become a reliable and sustainable feed source for livestock production.
To reduce feed losses, implementing good agricultural practices and proper post-harvest technologies, such as ensuring optimal storage conditions, can prevent the degradation of nutrients, dry matter, and energy in stored grains and forages, reducing spoilage and contamination by mold and mycotoxins (Hodges et al. 2011). Effective rodent control on farms also plays a crucial role in safeguarding feed, particularly grains. Additionally, using byproducts from the food and horticulture industries directly in harvested or ensiled forms, or indirectly as substrates for rearing insects, provides a sustainable feed source while minimizing food waste (Makkar and Ankers 2014). Anaerobic solid-state fermentation can further reduce toxins in by-products and destroy pathogenic microbes, making these resources safer for animal consumption. The use of mycotoxin binders helps mitigate losses caused by fungal contamination, especially in regions with poor storage conditions (Kolosova and Stroka 2011). On-farm measures, such as proper design of feed troughs, adjusting feed levels, and minimizing wastage during feeding and storage, are practical ways to reduce losses. Furthermore, promoting business models for feeding technologies, where private industries or cooperatives prepare and supply nutritionally balanced feed blocks, ammoniated straws, or silage, can ensure consistent availability and reduce the burden on farmers, thereby preventing feed wastage (Makkar 2016b). Together, these strategies enhance the sustainability of livestock production, improve food security, and reduce the environmental footprint of food–feed systems.
Some effective and potentially applicable CSAL for LMICs should be deployed only under certain conditions to account for local norms and constraints. One such strategy is timely culling, which can significantly reduce the lifetime emissions of livestock by ensuring that animals are not maintained beyond their productive years (Nguyen et al. 2023). Timely culling is typically defined as the removal of animals that have reached the end of their reproductive or productive efficiency. However, the implementation of this practice varies on the basis of context and cultural norms. In LMICs, particularly in regions where livestock are kept primarily for social status, ceremonial purposes, or religious reasons, the practice of culling may face resistance. These contexts often prioritize the symbolic or cultural value of animals over their economic or productive utility. Further research and stakeholder engagement are needed to identify culturally sensitive strategies for introducing timely culling, ensuring that it aligns with the values and priorities of local communities. Additional studies, particularly those examining how this strategy could be adapted in different cultural and economic contexts, would provide valuable insights.
Introducing improved forages is another promising strategy to boost livestock productivity and reduce GHG emissions by offering more digestible feed. Improved forages can enhance livestock efficiency, leading to lower emissions per unit of output. However, in African LMICs, adoption of such forages has been limited because of systemic barriers, including underdeveloped supply chains for forage seeds, insufficient infrastructure for harvesting and storage, and poorly functioning distribution and market systems. Most instances of poor adoption have been supply-led, with limited consideration of farmers’ needs and preferences. To overcome these challenges, there is a need to transition toward demand-led, private sector-driven approaches that address constraints to adoption, such as lack of access to reliable forage seeds and inadequate technical support. However, the introduction of improved forages must be balanced with the need to reduce reliance on synthetic fertilizers, which are often used to enhance forage production. Synthetic fertilizers contribute to GHG emissions during production and application and can lead to eutrophication and other environmental issues if overused or mismanaged. A holistic approach that incorporates organic fertilizers, integrated soil fertility management, and sustainable forage production practices can mitigate these risks while promoting environmental sustainability.
Grazing management is a promising mitigation and adaptation strategy, with well managed systems being able to sequester carbon from the environment (Follett and Reed 2010; Chen et al. 2015). However, grazing management is not applicable for most smallholders who have smallholdings (<2 ha), which is typical of many parts of South and Southeast Asia and sub-Saharan Africa (Jayne et al. 2003). Further, the communal grazing widely practiced in many parts of such regions presents significant hurdles to effective grazing management.
Finally, the mitigation of emissions through manure amendments, such as urease inhibitors or best management practices such as aeration or anaerobic digestion, holds potential but is contingent on resource availability. These technologies require financial investments, infrastructure, and technical expertise that may not be readily accessible or affordable for smallholders in LMICs. Future efforts should focus on adapting these technologies to local conditions, making them more cost-effective and scalable for resource-limited settings.
Modelling and measuring adoption of climate-smart practices
As noted above, despite the large number of potentially effective CSAL in existence, their use in practice has been limited by low adoption (Herrero et al. 2016; Beauchemin et al. 2022). In fact, the actual mitigation potential of existing CSAL is often only a small fraction of the true potential because most studies only consider the technical mitigation potential (Fig. 3). Consequently, the economic potential of mitigation approaches is less than 10% of what is technically possible because of adoption constraints, costs and numerous trade-offs (Herrero et al. 2016). These limitations include low climate finance investments for the livestock sector globally (typically less than 1%, World Bank 2021; Buto et al. 2021; Fig. 4). In addition, challenges related to lack of readiness of mitigation technologies or challenges with their field implementation cause low adoption by smallholder farmers. (Elbehri et al. 2017; World Bank 2021). Further limitations include low technical knowledge and capacity of farmers, particularly in LMICs, and limiting trade-offs including factors that are social (barriers to adoption, animal welfare, social license, traditional practices), policy related (carbon price, red tape, emission mitigation legislation), environmental (nutrient leaching, soil erosion, influence on biodiversity, ecosystems services), economic (cost of implementation and relative return on investment) and biophysical (climate, soil, geography, location) (Harrison et al. 2021). Yet, when potential GHG emissions mitigation strategies for livestock are being appraised, many of these issues are ignored or improperly accounted for (Smith 2012; Knapp et al. 2014; Harrison et al. 2021).
Factors limiting attainment of theoretical GHG mitigation potential (adapted from Harrison et al. 2021).
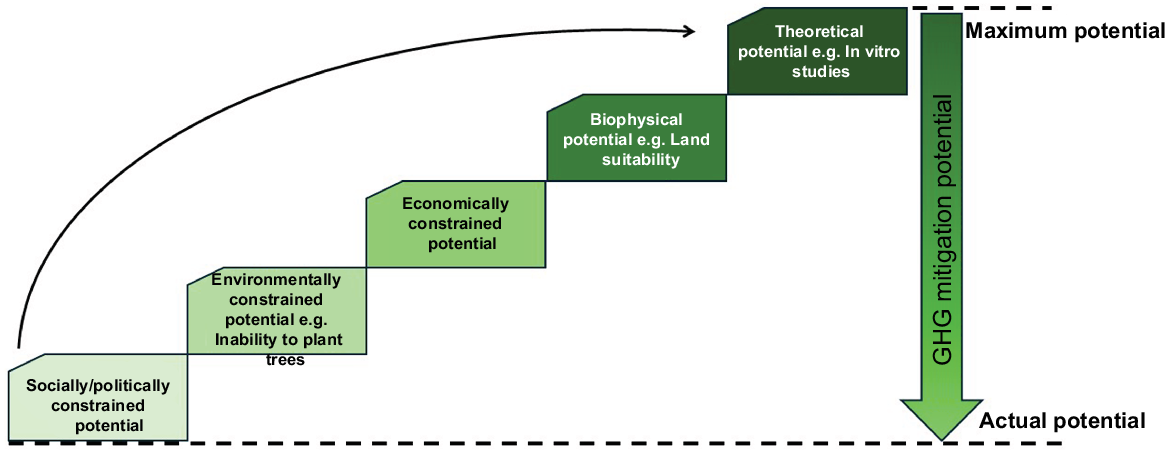
Climate finance for agriculture and land use subsector (Buto et al. 2021; source: OECD DAC Climate-related Development Finance database; compiled and calculated FAO).
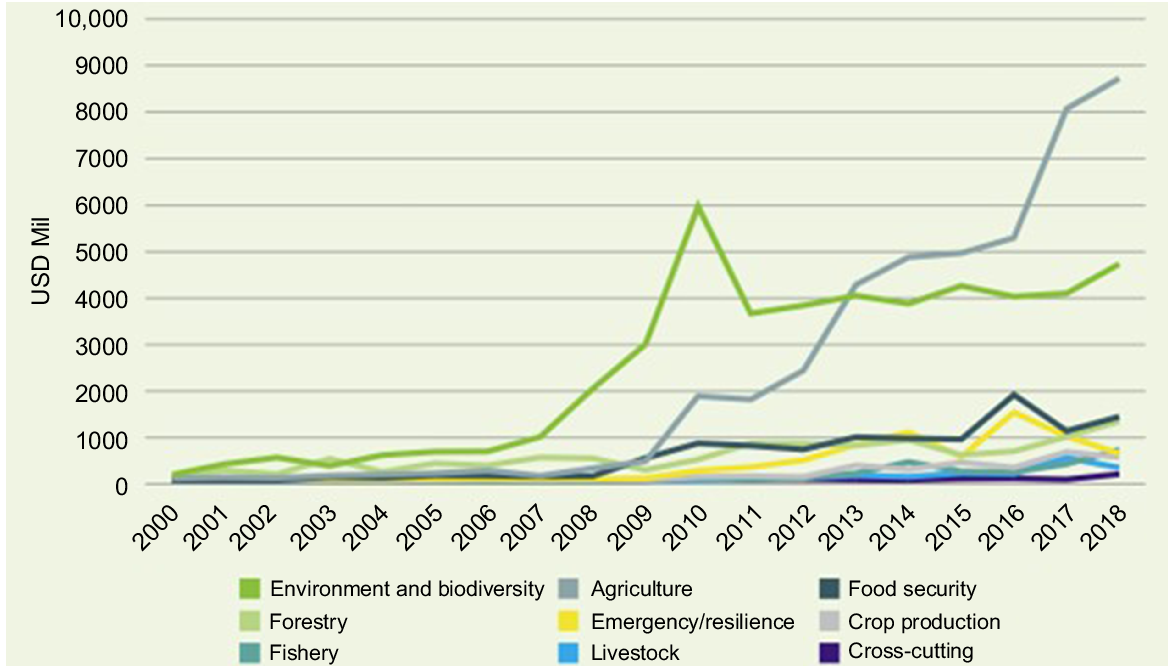
Modelling exercises can be used to assess the potential for adoption of promising CSAL. Several studies have used statistical and econometric approaches to assess the influence of various adoption determinants (Bryan et al. 2009; García de Jalón et al. 2015). García de Jalón et al. (2017) used a logit model to examine the influence of various types of capital on adoption and the overall adoption potential of four CSAL in rural parts of nine countries in sub–Saharan Africa (SSA). The study showed that for the four CSAL examined, the likelihood of adoption was less than 50%, indicating that a large number of households would not adopt any of them. Financial (capital stock that facilitates economic production) and physical (assets such as crop inputs, infrastructure, that are used to provide goods or services) capital had the greatest influence on adoption, followed by social (social networks that foster trust, understanding and cooperation), and human (productive capacities, knowledge and attributes that make people more productive) capital (Costanza and Daly 1992; Goodwin 2003; Pindyck and Rubinfield 2013), whereas natural capital (climate and soil characteristics that predetermine suitability for agriculture; García de Jalón et al. 2017) had no positive influence. Among the four CSAL, improving feed quality and introducing varieties for grasslands had greater probability of adoption across the target countries, whereas the probability of adoption of grassland or herd management was low. Similar robust approaches should be routinely used to examine adoption potential of CSAL before they are introduced to target regions. In addition, other factors such as private sector interest, demand for the farm products and market orientation of the farmers should be considered. In fact, one of the main reasons why CSAL show poor adoption potential is that they are often examined on entire target populations. Although it is well-known that there are five established adopter categories (Rogers 1995), these are rarely considered when examining potential for adoption of CSAL. A more logical approach may be to target innovators and early adopters alone, such as market oriented LMIC smallholders, with novel CSAL, and accept that adoption by other groups will take much longer.
Few global assessments of adoption of CSAL by region have been conducted. Table 1 outlines adoption levels and limiting factors for feed, assisted reproduction, animal health and breeding technologies and practices. In general, the literature suggests that CSAL adoption by smallholders may be greatest in Central and Latin America, Central Asia, South and Southeast Asia and Sub-Saharan Africa.
The report on an FAO electronic conference that discussed successes and failures with adoption of animal nutrition practices and technologies in developing countries (FAO 2011; Owen et al. 2012) is the most comprehensive. In addition, we have also summarized published assessment of global adoption of climate smart feed technologies (Table 1). Despite being a central focus of the electronic FAO conference, and except for limited adoption in China and India, adoption of urea-ammoniation of straw, enzyme or white-rot fungi treatment, were considered a failure (Owens et al. 2012). Whereas urea–molasses–multinutrient blocks, reducing forage particle size (chopping, shredding, grinding), improving forage production, forage breeding, hay making and food–feed approaches, were considered moderately adopted. The main adoption constraints were cost, land availability, marketing, lack of inputs, lack of technical knowledge, poor extension services and lack of awareness of the returns on the investment. Hallmarks of the moderately adopted technologies included being affordable, not laborious, too risky or technical, and with readily available inputs. An important factor highlighted was that smallholders would rather purchase than prepare the improved feeds, which highlights a significant opportunity for the private sector.
Baltenweck et al. (2020) conducted a scoping review of the adoption and impacts on productivity and livelihoods of three feed interventions (crop residue use, agroforestry and improved forages). On the basis of 73 journal papers, the authors noted that half of the papers focused on E. Africa or the Horn of Africa followed by Southeast Asia, perhaps reflecting dominance of smallholder mixed crop livestock systems that are more likely to adopt feed technologies in those areas. Most of the studies focused on improved forages, followed by agroforestry systems. Wide adoption ranges were found for improved forages (0–90%), agroforestry systems (8–87% and crop residue use (20–86%). Consequently, the authors concluded that meaningful deductions were difficult because of the broad range of adoption for each technology, differences in outcome measures, time horizons considered, and site peculiarities including weather and social characteristics. Drivers of adoption included farmer experience or education level, return on investment, extension support, low labor requirement/high labor availability, market access and market orientation of the farmer.
Both of these global feed technology adoption studies highlight the constraints and drivers of adoption and more importantly indicate the need for new research to document actual global adoption levels of such technologies in different regions of the world across specified time spans. Over the past 40–50 years, unlike in developed countries, animal breeding and genetic efforts have yielded little success for smallholder systems when compared with developed countries (Ayalew et al. 2003; Kosgey and Okeyo 2007; Rege et al. 2011). This has been largely attributed to inadequate attention to non-genetic factors when introducing pro-poor genetic improvement programs, such as factors related to the enabling environment (poor extension, private and public sector capacity, infrastructure, performance recording systems, and market access) as well as those characteristic of smallholder systems such as small herd and flock sizes, uncontrolled breeding, difficulty in delivery of artificial insemination (AI), high mortalities and long generation intervals, which are due largely to poor animal nutrition and health (Kosgey and Okeyo 2007; Rege et al. 2011).
A notable exception to the poor adoption of improved genetic technologies for smallholder ruminants is improving the genetic merit of dairy cows by artificial insemination (AI). In a study that examined adoption of breeding and reproductive technologies in Kenya, Tanzania, India and Nicaragua, AI and crossbreeding were the most widely used technologies (Ojango et al. 2017). However, unlike in India, which has the largest AI infrastructure globally, AI adoption in Kenya, Tanzania and Nicaragua were low to very low, and adoption of other assisted reproductive technologies such as in vitro fertilization and embryo sexing were even less common. Further, Makoni et al. (2015) noted that only 18% of the Kenya dairy herd and 0.05% of the beef cattle were bred by AI. Likewise, Mwanga et al. (2019) noted that except for crossbreeding and upgrading, which are commonly used in all countries, genetic improvement technologies were poorly adopted in sub-Saharan Africa. Factors such as farmer dairy farming experience, neighbors’ influence, record keeping ability, management practices including feed and water provision were associated with AI adoption. Therefore, to increase adoption of genetic interventions, much more attention should be paid to providing these enabling factors as well as providing access to markets, service suppliers, training, improved feeding, health and management capacity. In addition, to allow smallholders benefit from the more recent marker-based genomic and gene editing technologies, which are mostly beyond their reach, much more attention should be paid to building capacity for genotyping smallholder livestock and recording their performance.
No published studies on global adoption of animal health technologies and practices by smallholder farmers were found. Hidano et al. (2022) published a scoping review protocol to understand ‘Why animal health practices are or are (not) adopted among smallholders in LMIC’ but published results were not found. Lindahl et al. (2020) conducted a scoping review of efficacy of farm-level livestock interventions in LMIC and identified 70 papers that used randomized controlled trials to demonstrate efficacy of animal health interventions. However, insufficient information was found to estimate adoption rates or extents. Donadeu et al. (2019) reported that adoption of vaccines by smallholder or marginalized livestock farmers is low. Lindahl et al. (2020) cited high rates (>50%) of east coast fever (ECF) vaccine adoption during a project (Lynen et al. 2012), much lower uptake rates (<10%) in Uganda and Tanzania and almost negligible rates when the ECF vaccine production is compared with the number of at risk vaccinated cattle (<1%) (Kasibule 2013; Yiryele 2016). To increase vaccine adoption by smallholders, Donadeu et al. (2019) advocated including addressing technical, policy, private sector challenges and highlighted the need for government and donor support for neglected zoonotic diseases that the animal health industry does not consider to be profitable targets. To improve adoption of biosecurity measures Li et al. (2023) stated that a ‘one-size-fits-all’ should be avoided; rather, they should be educated on biosecurity principles and empowered to make personal decisions rather than adopt prescribed pre-defined interventions. Further, they recommended that interventions should align with farmer motivations and clearly outline the benefits. In a study on operationalizing infectious disease control in the Asian region, the authors noted that science is only half of the solution (Durrance-Bagale et al. 2022). Additional factors to address included lack of political will, regional heterogeneity, geopolitical issues including organizational factors (integration and harmonization; cross-border issues; funding, financing and sustainability; capacity-building; data sharing); governance and diplomacy (building collaborations and partnerships; communication; role of communities; diplomacy; leadership; ownership; sovereignty; political commitment); and stakeholders and multilateral agreements (Durrance-Bagale et al. 2022).
The way forward
Achievement of widespread adoption of climate-smart technologies in LMICs requires adherence to the multifaceted policy approach described by Hristov et al. (2013). This report highlighted the need for policies supporting extension and agricultural services provision, research and development, financial incentives, market friction instruments, advocacy about the many important roles of livestock and livestock products, nationally appropriate mitigation plans and international agreements. Although over a decade old, the persistent low adoption of most CSAL indicates even greater need for implementation of such enabling polices.
Relative to that devoted to developing and deploying CSAL, inadequate attention and funding have been devoted to ensuring their adoption at scale. Researchers and policy makers should shift from a focus on introducing technologies to one that first examines the technology readiness of the smallholder, the suitability of the technology for the context, the availability of the enabling environment, and if it does not exist, if and how it should be provided (Fig. 5). Smallholder technology readiness should be determined on the basis of which of the following groups they belong, because they are not monolithic: (1) the very poor barely surviving group, (2) the vulnerable poor group, (3) the viable market-oriented group and the (4) moderate- to high-income commercial farmers group. Improved technologies and practices should be tailored to smallholders on the basis of which group they belong to and the relative size of the group in a country or region. Grouping should be based on factors such as market orientation and access, income level/credit availability, gender and age, farm management skills, farm size, education and experience, and relationship with influencers such as membership of a cooperative or having progressive neighboring farmer, etc.
Potential technologies should be assessed for appropriateness for climate adaptation and mitigation as well as affordability, gender sensitivity, technical complexity, labor intensiveness, risk. Modelling approaches ranging from tools such as the International Livestock Research Institute (ILRI) TechFit (Padmakumar et al. 2014) for feed interventions to complex systems-based models should be used to determine the fit for purpose and context of the technology.
Enabling environments for technology adoption and scaling should be evaluated such as examining catalysts and hindrances to technology adoption. For instance, efforts to develop the improved forage industry in Ethiopia have failed for over 50 years partly owing to provision of free improved forage seeds by the government and non-governmental organizations (NGOs). Whereas such inputs may need to be subsidized during the demonstration phase, subsidies should be gradually withdrawn over time. Technologies should be introduced in a participatory manner, ensuring that key influencers clearly understand the need, benefits, appropriateness and returns from adopting the intervention, so that they can ‘advocate for’ the adoption process. Where possible, CSAL should be offered as a bundle to smallholder farmers as this is more likely to yield greater returns and foster adoption (Gray et al. 2012; Camara et al. 2019). Sustained extension support beyond the life of projects is integral to adoption whether it is provided in person or in digital format by cooperatives, extension agents, universities private sector, or NGOs. Partnerships among the latter groups should be developed to ensure sustained extension support for smallholders. Care must be taken to confirm that validated and site-specific extension information is provided, particularly when digital approaches are used to convey extension information.
Most CSAL technologies have focused on the supply side, sometimes resulting in low demand despite improved livestock productivity and many are targeted to men. Consequently, gender-sensitive CSAL that improve demand for livestock or livestock products are needed. These could include tools for improved aggregation, market access and strengthening, and price setting. Furthermore, partnerships between smallholders and commercial producers, restaurants, grocery stores and exporters should be fostered to assure strong and steady demand. For instance, smallholder cooperatives should be encouraged to produce inputs required by commercial producers, such as improved forage or milk.
Conclusions
This review has emphasized the importance of adopting a systems approach when assessing climate-smart agriculture for livestock (CSAL), considering not only mitigation, adaptation, and food security, but also environmental, economic, and socio-cultural factors. Sustainable intensification through a suite of CSAs, incorporating these key attributes, is proposed as a way to sustainably increase livestock production in low- and middle-income countries (LMICs). The review further explores promising feed, reproduction, health, and breeding strategies for smallholders, some of which have been adopted, whereas others remain underutilized. Essential prerequisites for the adoption of CSAL include technology simplicity, affordability, credit access, low risk, minimal labor requirements, availability of inputs, and clearly understood returns on investment. It is crucial to tailor technologies to the specific needs of smallholder farmers and create the enabling environment for their adoption. The paper also highlights the need for gender-sensitive and demand-driven solutions, advocating for partnerships between smallholder farmer groups and markets, such as restaurants, exporters, and grocery stores, to strengthen demand for their products. Recognizing that climate-smart technologies that are not adopted cannot be deemed effective, the review calls for greater investment in the factors that support technology adoption at scale by smallholders, ensuring their success and long-term impact.
Data availability
The data that support this study will be shared upon reasonable request to the corresponding author.
Conflicts of interest
Adegbola Adesogan is a Member of the Protein Pact academic advisory board. The authors declare no other conflicts of interest.
References
AGRA (2024) Food security monitor. Ed. 46. Alliance for a Green Revolution in Africa. Available at https://agra.org/wp-content/uploads/2024/05/Food-Security-Monitor_APRIL-ISSUE_Final.pdf
Almeida AK, Hegarty RS, Cowie A (2021) Meta-analysis quantifying the potential of dietary additives and rumen modifiers for methane mitigation in ruminant production systems. Animal Nutrition 7, 1219-1230.
| Crossref | Google Scholar | PubMed |
Amuge ML, Osewe DO (2017) Socio-economic factors influencing adoption of feed based dairy technologies among smallholder farmers in ekerenyo sub-county, Kenya. Asian Journal of Agricultural Extension, Economics & Sociology 16(2), 1-8.
| Crossref | Google Scholar |
Anandan S, Khan AA, Ravi D, Sai Bucha Rao M, Ramana Reddy Y, Blümmel M (2013) Identification of a superior dual purpose maize hybrid among widely grown hybrids in South Asia and value addition to its stover through feed supplementation and feed processing. Field Crops Research 153, 52-57.
| Crossref | Google Scholar |
Arndt C, Hristov AN, Price WJ, McClelland SC, Pelaez AM, Cueva SF, Oh J, Dijkstra J, Bannink A, Bayat AR, Crompton LA, Eugène MA, Enahoro D, Kebreab E, Kreuzer M, McGee M, Martin C, Newbold CJ, Reynolds CK, Schwarm A, Shingfield KJ, Veneman JB, Yáñez-Ruiz DR, Yu Z (2022) Full adoption of the most effective strategies to mitigate methane emissions by ruminants can help meet the 1.5°C target by 2030 but not 2050. Proceedings of the National Academy of Sciences of the United States of America 119(20), e2111294119.
| Crossref | Google Scholar |
Ayalew W, King JM, Bruns E, Rischkowsky B (2003) Economic evaluation of smallholder subsistence livestock production: lessons from an Ethiopian goat development program. Ecological Economics 45, 473-485.
| Crossref | Google Scholar |
Balehegn M, Duncan A, Tolera A, Ayantunde AA, Issa S, Karimou M, Zampaligré N, André K, Gnanda I, Varijakshapanicker P, Kebreab E, Dubeux J, Boote K, Minta M, Feyissa F, Adesogan AT (2020) Improving adoption of technologies and interventions for increasing supply of quality livestock feed in low- and middle-income countries. Global Food Security 26, 100372.
| Crossref | Google Scholar | PubMed |
Baltenweck I, Cherney D, Duncan A, et al. (2020) A scoping review of feed interventions and livelihoods of small-scale livestock keepers. Nature Plants 6, 1242-1249.
| Crossref | Google Scholar |
Baruselli PS, Sales JNS, Sala RV, Vieira LM, Sá Filho MF (2018) History, evolution and perspectives of timed artificial insemination programs in Brazil. Animal Reproduction 9(3), 139-152.
| Google Scholar |
Baruselli PS, Catussi BLC, de Abreu LA, Elliff FM, da Silva LG, Batista EOS (2019) Challenges to increase the AI and ET markets in Brazil. Animal Reproduction 16(3), 364-375.
| Crossref | Google Scholar | PubMed |
Beauchemin KA, McGinn SM (2005) Methane emissions from feedlot cattle fed barley or corn diets. Journal of Animal Science 83, 653-661.
| Crossref | Google Scholar | PubMed |
Beauchemin KA, Kreuzer M, O’Mara F, McAllister TA (2008) Nutritional management for enteric methane abatement: a review. Australian Journal of Experimental Agriculture 48, 21-27.
| Crossref | Google Scholar |
Beauchemin KA, Ungerfeld EM, Eckard RJ, Wang M (2020) Review: Fifty years of research on rumen methanogenesis: lessons learned and future challenges for mitigation. Animal 14, s2-s16.
| Crossref | Google Scholar | PubMed |
Beauchemin KA, Ungerfeld EM, Abdalla AL, Alvarez C, Arndt C, Becquet P, Benchaar C, Berndt A, Maurício RM, McAllister TA, Oyhantçabal W, Salami SA, Shalloo L, Sun Y, Tricarico JM, Uwizeye A, De Camillis C, Bernoux M, Robinson T, Kebreab E (2022) Invited review: current enteric methane mitigation options. Journal of Dairy Science 105, 9297-9326.
| Crossref | Google Scholar | PubMed |
Bekele W, Goshu G, Tamir B, Demissie T, Sahle Z (2019) Characterization of dairy production constraints, existing feeding practices and mineral supplementation in dairy feeds in two districts of East Shoa Zone, Ethiopia. Advances in Dairy Research 7(219), 2.
| Crossref | Google Scholar |
Blaxter KL, Clapperton JL (1965) Prediction of the amount of methane produced by ruminants. British Journal of Nutrition 19, 511-522.
| Crossref | Google Scholar | PubMed |
Blümmel M, Rao PP (2006) Economic value of sorghum stover traded as fodder for urban and peri-urban dairy production in Hyderabad, India. International Sorghum and Millets Newsletter 47, 97-100.
| Google Scholar |
Blümmel M, Sudhakar DS, Teymouri F, Gupta SK, Sharma GVM, Ravindranath K (2019) Spin-off technologies from 2nd generation biofuel: potential to transform fodder quality of crop residues. In ‘27 Annual Conference of the Ethiopian Society of Animal Production (ESAP)’, 29–31 August 2019, Addis Ababa, Ethiopia. (EIAR: Addis Ababa, Ethiopia)
Bryan E, Deressa TT, Gbetibouo GA, Ringler C (2009) Adaptation to climate change in Ethiopia and South Africa: options and constraints. Environmental Science & Policy 12, 413-426.
| Crossref | Google Scholar |
Burns BM, Reid DJ, Taylor JF (1997) An evaluation of growth and adaptive traits of different cattle genotypes in a subtropical environment. Australian Journal of Experimental Agriculture 37, 399-933.
| Crossref | Google Scholar |
Buto O, Galbiati GM, Alekseeva N, Bernoux M (2021) ‘Climate finance in the agriculture and land use sector – global and regional trends between 2000 and 2018.’ (FAO) 10.4060/cb6056en
Camara Y, Moula N, Sow F, Sissokho MM, Antoine-Moussiaux N (2019) Analysing innovations among cattle smallholders to evaluate the adequacy of breeding programs. Animal 13(2), 417-426.
| Crossref | Google Scholar | PubMed |
Casler M, Jung H-J (2006) Relationships of fibre, lignin, and phenolics to in vitro fibre digestibility in three perennial grasses. Animal Feed Science and Technology 125, 151-161.
| Crossref | Google Scholar |
Chander M (2010) Urea treatment of straw: hugely extolled rarely used. In ‘Successes and failures with animal nutrition practices and technologies in developing countries’, FAO Electronic Conference, 1–30 september 2010, Rome, Italy. (Ed. HPS Makkar) pp. 15–20. FAO Animal Production and Health, No. 11. (FAO: Rome, Italy)
Chen W, Huang D, Liu N, et al. (2015) Improved grazing management may increase soil carbon sequestration in temperate steppe. Scientific Reports 5, 10892.
| Crossref | Google Scholar | PubMed |
Costanza R, Daly HE (1992) Natural capital and sustainable development. Conservation Biology 6, 37-46.
| Crossref | Google Scholar |
Dillon JA, Stackhouse-Lawson KR, Thoma GJ, Gunter SA, Rotz CA, Kebreab E, Riley DG, Tedeschi LO, Villalba J, Mitloehner F, Hristov AN, Archibeque SL, Ritten JP, Mueller ND (2021) Current state of enteric methane and the carbon footprint of beef and dairy cattle in the United States. Animal Frontiers 11, 57-68.
| Crossref | Google Scholar | PubMed |
Donadeu M, Nwankpa N, Abela-Ridder B, Dungu B (2019) Strategies to increase adoption of animal vaccines by smallholder farmers with focus on neglected diseases and marginalized populations. PLOS Neglected Tropical Diseases 13(2), e0006989.
| Crossref | Google Scholar | PubMed |
Dong H, Tao X, Xin H, He Q (2004) Comparison of enteric methane emissions in China for different IPCC estimation methods and production schemes. Transactions of the ASAE 47, 2051-2057.
| Crossref | Google Scholar |
Durrance-Bagale A, Marzouk M, Ananthakrishnan A, Nagashima-Hayashi M, Lam ST, Sittimart M, et al. (2022) ‘Science is only half of it’: Expert perspectives on operationalising infectious disease control cooperation in the ASEAN region. PLOS Global Public Health 2(5), e0000424.
| Crossref | Google Scholar | PubMed |
Elbehri A, Challinor A, Verchot L, Angelsen A, Hess T, Ouled Belgacem A, Clark H, Badraoui M, Cowie A, De Silva S, Erickson J, Joar Hegland S, Iglesias A, Inouye D, Jarvis A, Mansur E, Mirzabaev A, Montanarella L, Murdiyarso D, Notenbaert A, Obersteiner M, Paustian K, Pennock D, Reisinger A, Soto D, Soussana J-F, Thomas R, Vargas R, Van Wijk M, Walker R (2017) FAO–IPCC Expert Meeting on Climate Change, Land Use and Food Security: Final Meeting Report; 23–25 January 2017 FAO HQ Rome. FAO and IPCC.
Eun J-S, Fellner V, Gumpertz ML (2004) Methane production by mixed ruminal cultures incubated in dual-flow fermentors. Journal of Dairy Science 87, 112-121.
| Crossref | Google Scholar | PubMed |
FAO (2014) ‘World mapping of animal feeding systems in the dairy sector.’ (Food and Agriculture Organization of the United Nations). Available at http://www.fao.org/3/a-i3913e.pdf
FAO (2018) ‘World livestock: transforming the livestock sector through the sustainable development goals.’ (Food and Agriculture Organization of the United Nations: Rome, Italy) 10.4060/ca1201en
FAO (2023a) ‘The status of women in agrifood systems.’ (Food and Agriculture Organization of the United Nations: Rome, Italy) 10.4060/cc5343en
FAO (2023b) ‘Pathways towards lower emissions – A global assessment of the greenhouse gas emissions and mitigation options from livestock agrifood systems.’ (Food and Agriculture Organization of the United Nations: Rome, Italy) 10.4060/cc9029en
Ferket PR, van Heugten E, van Kempen TATG, Angel R (2002) Nutritional strategies to reduce environmental emissions from nonruminants. Journal of Animal Science 80(E-suppl_2), E168-E182.
| Crossref | Google Scholar |
Follett RF, Reed DA (2010) Soil carbon sequestration in grazing lands: societal benefits and policy implications. Rangeland Ecology & Management 63, 4-15.
| Crossref | Google Scholar |
Frey GE, Fassola HE, Pachas AN, Colcombet L, Lacorte SM, Pérez O, Renkow M, Warren ST, Cubbage FW (2012) Perceptions of silvopasture systems among adopters in northeast Argentina. Agricultural Systems 105(1), 21-32.
| Crossref | Google Scholar |
García de Jalón S, Silvestri S, Granados A, et al. (2015) Behavioural barriers in response to climate change in agricultural communities: an example from Kenya. Regional Environmental Change 15, 851-865.
| Crossref | Google Scholar |
García de Jalón S, Silvestri S, Barnes AP (2017) The potential for adoption of climate smart agricultural practices in Sub-Saharan livestock systems. Regional Environmental Change 17, 399-410.
| Crossref | Google Scholar |
Garg MR, Kannan A, Phondba BT, Shelke SK, Sherasia PL (2012) A study on the effect of ration balancing for improving milk production and reducing methane emission in lactating buffaloes under field conditions. Indian Journal of Dairy Science 65(3), 250-255.
| Google Scholar |
Garg MR, Sherasia PL, Phondba BT, Shelke SK, Patel CT (2013) Effect of feeding balanced ration on milk production, enteric methane emission and metabolic profile in crossbred cows under field conditions. Indian Journal of Dairy Science 66(2), 113-119.
| Google Scholar |
GBD 2021 Anaemia Collaborators (2023) Prevalence, years lived with disability, and trends in anaemia burden by severity and cause, 1990–2021: findings from the Global Burden of Disease Study 2021. The Lancet Haematology 10(9), e713-e734.
| Crossref | Google Scholar |
Godde CM, Mason-D’Croz D, Mayberry DE, Thornton PK, Herrero M (2021) Impacts of climate change on the livestock food supply chain; a review of the evidence. Global Food Security 28, 100488.
| Crossref | Google Scholar |
Gray GD, Connell JG, Phimphachanhvongsod V (2012) Worms in smallholder livestock systems: technologies and practices that make a difference. Veterinary Parasitology 186(1–2), 124-131.
| Crossref | Google Scholar | PubMed |
Haile A, Gizaw S, Getachew T, Mueller JP, Amer P, Rekik M, Mwai O (2019) Community-based breeding programmes are a viable solution for Ethiopian small ruminant genetic improvement but require public and private investments. Frontiers in Genetics 10, 1145.
| Crossref | Google Scholar |
Haile A, Getachew T, Rekik M, Abebe A, Abate Z, Jimma A, Mwacharo JM, Mueller J, Belay B, Solomon D, Hyera E, Nguluma AS, Gondwe T, Rischkowsky B (2023) How to succeed in implementing community-based breeding programs: lessons from the field in eastern and southern Africa. Frontiers in Genetics 14, 1119024.
| Crossref | Google Scholar | PubMed |
Hamid P, Akbar T, Hossein J, Ali MG (2007) Nutrient digestibility and gas production of some tropical feeds used in ruminant diets estimated by the in vivo and in vitro gas production techniques. American Journal of Animal and Veterinary Sciences 2(4), 108-113.
| Crossref | Google Scholar |
Harrison MT, Cullen BR, Mayberry DE, Cowie AL, Bilotto F, Badgery WB, Liu K, Davison T, Christie KM, Muleke A, Eckard RJ (2021) Carbon myopia: the urgent need for integrated social, economic, and environmental action in the livestock sector. Global Change Biology 27(22), 5726-5761.
| Crossref | Google Scholar | PubMed |
Hart EH, Christofides SR, Davies TE, Rees Stevens P, Creevey CJ, Müller CT, Rogers HJ, Kingston-Smith AH (2022) Forage grass growth under future climate change scenarios affects fermentation and ruminant efficiency. Scientific Reports 12, 4454.
| Crossref | Google Scholar | PubMed |
Henry AF, Charmley B, Eckard R, Gaughan JB, Hegarty R (2012) Livestock production in a changing climate: adaptation and mitigation research in Australia. Crop & Pasture Science 63(3), 191-202.
| Crossref | Google Scholar |
Herrero M, Henderson B, Havlík P, Thornton PK, Conant RT, Smith P, Wirsenius S, Hristov AN, Gerber PJ, Gill M, Butterbach-Bahl K, Valin H, Garnett T, Stehfest E (2016) Greenhouse gas mitigation potentials in the livestock sector. Nature Climate Change 6(5), 452-461.
| Crossref | Google Scholar |
Hidano A, Holt H, Durrance-Bagale A, Tak M, Rudge JW (2022) Exploring why animal health practices are (not) adopted among smallholders in low and middle-income countries: a realist framework and scoping review protocol. Frontiers in Veterinary Science 9, 915487.
| Crossref | Google Scholar | PubMed |
Hodges RJ, Buzby JC, Bennett B (2011) Postharvest losses and waste in developed and less developed countries: opportunities to improve resource use. Journal of Agricultural Science 149, 37-45.
| Crossref | Google Scholar |
Honan M, Feng X, Tricarico JM, Kebreab E (2021) Feed additives as a strategic approach to reduce enteric methane production in cattle: modes of action, effectiveness, and safety. Animal Feed Science and Technology 62, 1303-1317.
| Crossref | Google Scholar |
Hou L, Chen X, Kuhn L, Huang J (2019) The effectiveness of regulations and technologies on sustainable use of crop residue in Northeast China. Energy Economics 81, 519-527.
| Crossref | Google Scholar |
Hristov AN, Oh J, Firkins JL, Dijkstra J, Kebreab E, Waghorn G, Makkar HPS, Adesogan AT, Yang W, Lee C, Gerber PJ, Henderson B, Tricarico JM (2013) SPECIAL TOPICS – Mitigation of methane and nitrous oxide emissions from animal operations: I. A review of enteric methane mitigation options. Journal of Animal Science 91(11), 5045-5069.
| Crossref | Google Scholar | PubMed |
Hundal JS, Aparna, Singh U, Bhatti JS (2016) Methodical investigation of cognitive domain of dairy farmers on the practice of mineral mixture supplementation and its interaction with socio-personal characters. Indian Journal of Animal Nutrition 33(2), 164-168.
| Crossref | Google Scholar |
IPCC (2019) 2019 Refinement to the 2006 IPCC Guidelines for National Greenhouse Gas Inventories. (Eds E Calvo Buendia, K Tanabe, A Kranjc, J Baasansuren, M Fukuda, S Ngarize, A Osako, Y Pyrozhenko, P Shermanau, S Federici). Volume 5, Chapter 6. IPCC, Switzerland. Available at https://www.ipcc.ch/report/2019-refinement-to-the-2006-ipcc-guidelines-for-national-greenhouse-gas-inventories/
Janssen PH (2010) Influence of hydrogen on rumen methane formation and fermentation balances through microbial growth kinetics and fermentation thermodynamics. Animal Feed Science and Technology 160, 1-22.
| Crossref | Google Scholar |
Jayne TS, Yamano T, Weber MT, Tschirley D, Benfica R, Chapoto A, Zulu B (2003) Smallholder income and land distribution in Africa: implications for poverty reduction strategies. Food Policy 28(3), 253-275.
| Crossref | Google Scholar |
Jha S, Kaechele H, Sieber S (2021) Factors influencing the adoption of agroforestry by smallholder farmer households in Tanzania: case studies from Morogoro and Dodoma. Land Use Policy 103, 105308.
| Crossref | Google Scholar |
Jisso M, Tesfaye T, Biadgilign S, Tareke AA, Zerfu TA (2022) The role of multi-dimensional women’s empowerment in agriculture to improve the nutritional status of under-five children in rural cash crop producing, resource-limited settings of Ethiopia. Journal of Nutritional Science 11, e92.
| Crossref | Google Scholar | PubMed |
Johnson KA, Johnson DE (1995) Methane emissions from cattle. Journal of Animal Science 73(8), 2483-2492.
| Crossref | Google Scholar | PubMed |
Jurgilevich A, Birge T, Kentala-Lehtonen J, Korhonen-Kurki K, Pietikäinen J, Saikku L, Schösler H (2016) Transition towards circular economy in the food system. Sustainability 8(1), 69.
| Crossref | Google Scholar |
Kannan A, Garg MR, Mahesh Kumar BV (2011) Effect of ration balancing on milk production, microbial protein synthesis and methane emission in crossbred cows under field conditions in Chittoor district of Andhra Pradesh. Indian Journal of Animal Nutrition 28(2), 117-123.
| Google Scholar |
Kasibule D (2013) Impact of the immunisation of cattle against east coast fever in Bugabula County, Kamuli District, Uganda. Makerere University, Uganda. Available at http://dspace.mak.ac.ug/handle/10570/2053
Kaur M, Malik DP, Malhi GS, Sardana V, Bolan NS, Lal R, Siddique KHM (2022) Rice residue management in the Indo-Gangetic Plains for climate and food security: a review. Agronomy for Sustainable Development 42, 92.
| Crossref | Google Scholar |
Khanum SA, Hussain M, Hussain HN, Ishaq M (2010) Impact of urea–molasses–multinutrient block supplementation on livestock production in Pakistan. In ‘Successes and failures with animal nutrition practices and technologies in developing countries, Proceedings of the FAO Electronic Conference’, 1–30 September 2010, Rome, Italy, pp. 25–28. (Food and Agriculture Organization of the United Nations)
Knapp JR, Laur GL, Vadas PA, Weiss WP, Tricarico JM (2014) Invited review: enteric methane in dairy cattle production: quantifying the opportunities and impact of reducing emissions. Journal of Dairy Science 97(6), 3231-3261.
| Crossref | Google Scholar |
Kolosova A, Stroka J (2011) Substances for reduction of the contamination of feed by mycotoxins: a review. World Mycotoxin Journal 4, 225-256.
| Crossref | Google Scholar |
Komarek AM, Dunston S, Enahoro D, Godfray HCJ, Herrero M, Mason-D’Croz D, Rich KM, Scarborough P, Springmann M, Sulser TB, Wiebe K, Willenbockel D (2021) Income, consumer preferences, and the future of livestock-derived food demand. Global Environmental Change 70, 102343.
| Crossref | Google Scholar | PubMed |
Kosgey IS, Okeyo AM (2007) Genetic improvement of small ruminants in low-input, smallholder production systems: technical and infrastructural issues. Small Ruminant Research 70(1), 76-88.
| Crossref | Google Scholar |
Kumar S, Dagar SS, Sirohi SK, Upadhyay RC, Puniya AK (2013) Microbial profiles, in vitro gas production and dry matter digestibility based on various ratios of roughage to concentrate. Annals of Microbiology 63(2), 541-545.
| Crossref | Google Scholar |
Lee C, Hristov AN, Dell CJ, Feyereisen GW, Kaye J, Beegle D (2012) Effect of dietary protein concentration on ammonia and greenhouse gas emitting potential of dairy manure. Journal of Dairy Science 95(4), 1930-1941.
| Crossref | Google Scholar | PubMed |
Lestari VS, Rahardja DP, Sirajuddin SN (2022) Barriers to adopt biosecurity at smallholder farmers. IOP Conference Series: Earth and Environmental Science 1012(1), 012020.
| Crossref | Google Scholar |
Li J, Yuan M, Wang H, Zhou K (2023) Government regulations, biosecurity awareness, and farmers’ adoption of biosecurity measures: evidence from pig farmers in Sichuan Province, China. Frontiers in Sustainable Food Systems 7, 1106766.
| Crossref | Google Scholar |
Lindahl JF, Mutua F, Grace D (2020) Evaluating farm-level livestock interventions in low-income countries: a scoping review of what works, how, and why. Animal Health Research Reviews 21(2), 108-121.
| Crossref | Google Scholar | PubMed |
Lovett DK, Shalloo L, Dillon P, O’Mara FP (2006) A systems approach to quantify greenhouse gas fluxes from pastoral dairy production as affected by management regime. Agricultural Systems 88(2–3), 156-179.
| Crossref | Google Scholar |
Lynen G, Yrjö-Koskinen AE, Bakuname C, Di Giulio G, Mlinga N, Khama I, Hanks J, Taylor NM, James AD, McKeever D, Peters AR, Rushton J (2012) East Coast fever immunisation field trial in crossbred dairy cattle in Hanang and Handeni districts in northern Tanzania. Tropical Animal Health and Production 44, 567-572.
| Crossref | Google Scholar |
Makkar HPS (2016b) Smart livestock feeding strategies for harvesting triple gain – the desired outcomes in planet, people and profit dimensions: a developing country perspective. Animal Production Science 56(3), 519-534.
| Crossref | Google Scholar |
Makkar HPS (2018) Review: feed demand landscape and implications of food-not feed strategy for food security and climate change. Animal 12(8), 1744-1754.
| Crossref | Google Scholar | PubMed |
Makkar HPS, Ankers P (2014) A need for generating sound quantitative data at national levels for feed-efficient animal production. Animal Production Science 54(10), 1569-1574.
| Crossref | Google Scholar |
Manzana NP, McCrindle CME, Sebei PJ, Prozesky L (2014) Optimal feeding systems for small-scale dairy herds in the North West Province, South Africa. Journal of the South African Veterinary Association 85(1), 1-8.
| Crossref | Google Scholar |
Marshall K, Quiros-Campos C, Van der Werf JHJ, Kinghorn B (2011) Marker-based selection within smallholder production systems in developing countries. Livestock Science 136(1), 45-54.
| Crossref | Google Scholar |
Martin C, Morgavi D, Doreau M (2010) Methane mitigation in ruminants: from microbe to the farm scale. Animal 4(3), 351-365.
| Crossref | Google Scholar | PubMed |
Mmereki D, David VE, Jr., Brownell AHW (2024) The management and prevention of food losses and waste in low- and middle-income countries: a mini-review in the Africa region. Waste Management & Research 42(4), 287-307.
| Crossref | Google Scholar | PubMed |
Moe PW, Tyrrell HF (1979) Methane production in dairy cows. Journal of Dairy Science 62(10), 1583-1586.
| Crossref | Google Scholar |
Mottet A, De Haan C, Falcucci A, Tempio G, Opio C, Gerber PJ (2017) Livestock: On our plates or eating at our table? A new analysis of the feed/food debate. Global Food Security [14] 1-8.
| Google Scholar |
Mudzengi CP, Taderera LM, Tigere A, Kapembeza CS, Moyana S, Zimondi M, Derembwe ET, Dahwa E (2014) Adoption of urea treatment of maize stover technology for dry season supplementation of cattle in Wedza, Zimbabwe. Livestock Research for Rural Development 26(9), 1-8.
| Google Scholar |
Munywoki GN (2021) Adapting to the effects of climate change on livestock production through animal-breeding in Kenya: a brief review of the literature. International Journal of Veterinary Science and Medical Diagnosis 2(2), 108.
| Crossref | Google Scholar |
Mushonga B, Dusabe JP, Kandiwa E, Bhebhe E, Habarugira G, Samkange A (2017) Artificial insemination in Nyagatare District: level of adoption and the factors determining its adoption. Alexandria Journal of Veterinary Sciences 55(1), 1-7.
| Google Scholar |
Mwai O, Hanotte O, Young-jun K, Seo-ae J (2015) Invited review – African indigenous cattle: unique genetic resources in a rapidly changing world. Animal Bioscience 28(7), 911-921.
| Crossref | Google Scholar |
Mwanga G, Mujibi FDN, Yonah ZO, et al. (2019) Multi-country investigation of factors influencing breeding decisions by smallholder dairy farmers in sub-Saharan Africa. Tropical Animal Health and Production 51, 395-409.
| Crossref | Google Scholar | PubMed |
Ngeno V (2024) Adoption of dairy feed technology and its impact on smallholder farmers’ income and poverty in Kenya’s south-western region. Scientific African 23, e02123.
| Crossref | Google Scholar |
Nguyen T, Smith J, Johnson L (2023) Timely culling as a strategy to reduce lifetime emissions of livestock. Journal of Agricultural Science 15(2), 45-58.
| Google Scholar |
Nimbalkar V, Verma HK, Singh J (2022) Impact of Urea–Molasses Multinutrient Block (UMMB) Technology Adoption on Dairy Animal Performance and Factors Associated with its Adoption. Journal of Community Mobilization and Sustainable Development 17, 80-86.
| Google Scholar |
OCHA (2023) Situation Report: Ethiopia: Drought Situation Update #1 - As of 10 March 2023. UN Office for the Coordination of Humanitarian Affairs. Available at https://reliefweb.int/report/ethiopia/ethiopia-drought-situation-update-1-10-march-2023
Odoi A, Gathuma JM, Gachuiri CK, Omore A (2007) Risk factors of gastrointestinal nematode parasite infections in small ruminants kept in smallholder mixed farms in Kenya. BMC Veterinary Research 3, 6.
| Crossref | Google Scholar |
OECD/FAO (2022) ‘OECD–FAO Agricultural Outlook 2022–2031.’ (OECD Publishing) 10.1787/f1b0b29c-en
Ojango JMK, Wasike CB, Enahoro DK, Okeyo AM (2017) Dairy production systems and the adoption of genetic and breeding technologies in Tanzania, Kenya, India and Nicaragua. Animal Genetic Resources 59, 81-95.
| Crossref | Google Scholar |
Okello D, Owuor G, Larochelle C, Gathungu E, Mshenga P (2021) Determinants of utilization of agricultural technologies among smallholder dairy farmers in Kenya. Journal of Agriculture and Food Research 6, 100213.
| Crossref | Google Scholar |
Ominski K, McAllister T, Stanford K, Mengistu G, Kebebe EG, Omonijo F, Cordeiro M, Legesse G, Wittenberg K (2021) Utilization of by-products and food waste in livestock production systems: a Canadian perspective. Animal Frontiers 11(2), 55-63.
| Crossref | Google Scholar | PubMed |
Omondi IA, Zander KK, Bauer S, Baltenweck I (2017) Understanding farmers’ preferences for artificial insemination services provided through dairy hubs. Animal 11(4), 677-686.
| Crossref | Google Scholar | PubMed |
Ortiz-Gonzalo D, Vaast P, Oelofse M, de Neergaard A, Albrecht A, Rosenstock TS (2017) Farm-scale greenhouse gas balances, hotspots and uncertainties in smallholder crop-livestock systems in Central Kenya. Agriculture, Ecosystems & Environment 248, 58-70.
| Crossref | Google Scholar |
Owen E, Jayasuriya MCN (1989) Use of crop residues as animal feeds in developing countries. Research and Development in Agriculture 6(3), 129-138.
| Google Scholar |
Owen E, Smith T, Makkar H (2012) Successes and failures with animal nutrition practices and technologies in developing countries: a synthesis of an FAO e-conference. Animal Feed Science and Technology 174, 211-226.
| Crossref | Google Scholar |
Oxford Analytica (2023) Animal health and sustainability: a global data analysis. Oxford Analytica. Available at https://pages.oxan.com/rs/109-ILL-989/images/Animal-health-and-Sustainability-A-Global-Data-Analysis-FINAL.pdf
Paucar-Quishpe V, Pérez-Otáñez X, Rodríguez-Hidalgo R, Pérez-Escalante C, Cepeda-Bastidas D, Grijalva J, Enríquez S, Arciniegas-Ortega S, Vanwambeke SO, Ron-Garrido L, Saegerman C (2024) Farmers’ adoption, knowledge, and perceptions of tick control measures on dairy farms in subtropical areas of continental Ecuador. Transboundary and Emerging Diseases 2024(1), 5023240.
| Crossref | Google Scholar |
Peters M, Horne P, Schmidt A, Holmann F, Kerridge PC, Tarawali SA, Schultze-Kraft R, Lascano CE, Argel P, Stür W, Fujisaka S, Müller-Sämann K, Wortmann C (2001) The role of forages in reducing poverty and degradation of natural resources in tropical production systems. Network Paper No. 117. Agricultural Research and Extension Network.
Prayaga KC, Barendse W, Burrow HM (2006) Genetic approaches to enhance adaptability of livestock to tropical environments. Journal of Animal Science 84(E. Suppl), E205-E210.
| Crossref | Google Scholar |
Rahman MS, Sujan MHK, Sherf-Ul-Alam M, Sultana M, Akter MS (2023) Adoption of improved management practices of livestock: case of small-scale farmers in rural Bangladesh. Heliyon 9(8), e18667.
| Crossref | Google Scholar |
Ramin M, Fant P, Huhtanen P (2021) The effects of gradual replacement of barley with oats on enteric methane emissions, rumen fermentation, milk production, and energy utilization in dairy cows. Journal of Dairy Science 104, 5617-5630.
| Crossref | Google Scholar | PubMed |
Rege JEO, Marshall K, Notenbaert A, Ojango JMK, Okeyo AM (2011) Pro-poor animal improvement and breeding – What can science do? Livestock Science 136(1), 15-28.
| Crossref | Google Scholar |
Rehman A, Batool Z, Ma H, et al. (2024) Climate change and food security in South Asia: the importance of renewable energy and agricultural credit. Humanities and Social Sciences Communications 11, 342.
| Crossref | Google Scholar |
Reisinger A, Clark H, Cowie AL, Emmet-Booth J, Gonzalez Fischer FC, Herrero M, Howden M, Leahy S (2021) How necessary and feasible are reductions of methane emissions from livestock to support stringent temperature goals? Philosophical Transactions of the Royal Society A: Mathematical, Physical and Engineering Sciences 379, 20200452.
| Crossref | Google Scholar |
Robi DT, Bogale A, Temteme S, Aleme M, Urge B (2024) Adoption of veterinary vaccines, determining factors, and barriers in Southwest Ethiopia: implications for livestock health and disease management strategies. Preventive Veterinary Medicine 225, 106143.
| Crossref | Google Scholar | PubMed |
Salmon G (2018) Fact Check 2: Livestock and Economy: Does the livestock sector make up 40% of total agricultural GDP globally? LD4D Livestock Fact Check 2. Supporting Evidence Based Interventions project, University of Edinburgh, Edinburgh, UK. Available at http://hdl.handle.net/1842/30115
Sarnklong C, Cone JW, Pellikaan W, Hendriks WH (2010) Utilization of rice straw and different treatments to improve its feed value for ruminants: a review. Asian-Australasian Journal of Animal Sciences 23(5), 680-692.
| Crossref | Google Scholar |
Sattler SE, Saballos A, Xin Z, Funnell-Harris DL, Vermerris W, Pedersen JF (2014) Characterization of novel Sorghum brown midrib mutants from an EMS-mutagenized population. G3: Genes, Genomes, Genetics 4(11), 2115-2124.
| Crossref | Google Scholar | PubMed |
Simiyu A, Chemuliti J, Nyamongo I, Bukachi S (2022) Informal institutional barriers to access and utilisation of Newcastle disease vaccines among women smallholder chicken farmers in Makueni, Kenya. Anthropology Preprint.
| Crossref | Google Scholar |
Singh GP, Mohini M (1999) Effect of different levels of rumensin in diet on rumen fermentation, nutrient digestibility and methane production in cattle. Asian-Australasian Journal of Animal Sciences 12(8), 1215-1221.
| Crossref | Google Scholar |
Smerald A, Rahimi J, Scheer C (2023) A global dataset for the production and usage of cereal residues in the period 1997–2021. Scientific Data 10, 685.
| Crossref | Google Scholar |
Smil V (1999) Crop residues: Agriculture’s largest harvest: crop residues incorporate more than half of the world’s agricultural phytomass. Bioscience 49(4), 299-308.
| Crossref | Google Scholar |
Smith P (2012) Agricultural greenhouse gas mitigation potential globally, in Europe and in the UK: what have we learnt in the last 20 years? Global Change Biology 18(1), 35-43.
| Crossref | Google Scholar |
Son HN, Chi DTL, Kingsbury A (2019) Indigenous knowledge and climate change adaptation of ethnic minorities in the mountainous regions of Vietnam: a case study of the Yao people in Bac Kan Province. Agricultural Systems 176, 102683.
| Crossref | Google Scholar |
Subudhi HN, Prasad KVSV, Ramakrishna C, Rameswar PS, Pathak H, Ravi D, Khan AA, Padmakumar V, Blümmel M (2020) Genetic variation for grain yield, straw yield and straw quality traits in 132 diverse rice varieties released for different ecologies such as upland, lowland, irrigated and salinity prone areas in India. Field Crops Research 245, 107626.
| Crossref | Google Scholar |
Thirunavukkarasu D, Jothilakshmi M, Silpa MV, Sejian V (2022) Factors driving adoption of climatic risk mitigating technologies with special reference to goat farming in India: evidence from meta-analysis. Small Ruminant Research 216, 106804.
| Crossref | Google Scholar |
Thornton P, Nelson GC, Mayberry D, Herrero M (2022) Impacts of heat stress on global cattle production during the 21st century: a modelling study. The Lancet Planetary Health 6(3), e192-e201.
| Crossref | Google Scholar | PubMed |
Tuyen VD, Cone JW, Baars JJP, Sonnenberg ASM, Hendriks WH (2013) Fungal strain and incubation period affect chemical composition and nutrient availability of wheat straw for rumen fermentation. Bioresource Technology 150, 202-208.
| Crossref | Google Scholar |
UNEP Food Waste Index Report (2021) Available at https://www.unep.org/resources/report/unep-food-waste-index-report-2021 [accessed 10 December 2024]
Valergakis GE, Arsenos G, Basdagianni Z, Banos G (2008) Grouping strategies and lead factors for ration formulation in milking ewes of the Chios breed. Livestock Science 115(2–3), 211-218.
| Crossref | Google Scholar |
Valli C (2020) Mitigating enteric methane emission from livestock through farmer-friendly practices. In ‘Global climate change and environmental policy’. (Eds V Venkatramanan, S Shah, R Prasad) pp. 257–273. (Springer: Singapore) 10.1007/978-3-030-45443-9_13
van Hal O, Weijenberg AAA, de Boer IJM, van Zanten HHE (2019) Accounting for feed-food competition in environmental impact assessment: towards a resource efficient food-system. Journal of Cleaner Production 240, 118241.
| Crossref | Google Scholar |
van Wijk MT, Merbold L, Hammond J, Butterbach-Bahl K (2020) Improving assessments of the three pillars of climate smart agriculture: current achievements and ideas for the future. Frontiers in Sustainable Food Systems 4, 558483.
| Crossref | Google Scholar |
Van Zanten HHE, Van Ittersum MK, De Boer IJM (2019) The role of farm animals in a circular food system. Global Food Security 21, 18-22.
| Crossref | Google Scholar |
Von Soosten D, Meyer U, Hummel J, Sudekum KH, Dänicke S (2020) Reducing greenhouse gas emissions in livestock production by balancing nutrient supply and improving feed efficiency. Journal of Dairy Science 103(6), 5591-5604.
| Crossref | Google Scholar |
Vyas D, Alemu AW, McGinn SM, Duval SM, Kindermann M, Beauchemin KA (2018) The combined effects of supplementing monensin and 3-nitrooxypropanol on methane emissions, growth rate, and feed conversion efficiency in beef cattle fed high-forage and high-grain diets. Journal of Animal Science 96(7), 2923-2938.
| Crossref | Google Scholar | PubMed |
World Bank (2021) Opportunities for climate finance in the livestock sector: removing obstacles and realizing potential. World Bank, Washington, DC, USA. Available at http://hdl.handle.net/10986/35495.
Wurzinger M, Gutiérrez GA, Sölkner J, Probst L (2021) Community-based livestock breeding: coordinated action or relational process? Frontiers in Veterinary Science 8, 671656.
| Crossref | Google Scholar |
Xu X, Sharma P, Shu S, Lin T-S, Ciais P, Tubiello FN, Smith P, Campbell N, Jain AK (2021) Global greenhouse gas emissions from animal-based foods are twice those of plant-based foods. Nature Food 2(9), 724-732.
| Crossref | Google Scholar | PubMed |
Yiryele, M (2016) Prevalence of Theileria parva and Trypanosome Infections in the Dry Season: A Case of Monduli District, Northern Tanzania. Sokoine University Of Agriculture, Tanzania. Available at http://suaire.suanet.ac.tz:8080/xmlui/handle/123456789/1575
Young JR, Evans-Kocinski S, Bush RD, Windsor PA (2015) Improving smallholder farmer biosecurity in the Mekong region through change management. Transboundary and Emerging Diseases 62(5), 491-504.
| Crossref | Google Scholar | PubMed |
Zabala A, Barrios LEG, Pascual U (2022) From participation to commitment in silvopastoral programmes: insights from Chiapas, Mexico. Ecological Economics 200, 107544.
| Crossref | Google Scholar |