Environmental impacts of the Australian poultry industry. 1. Chicken meat production
M. A. Copley
A Integrity Ag & Environment, 10511 New England Highway, Highfields, Qld 4352, Australia.
Animal Production Science 63(5) 489-504 https://doi.org/10.1071/AN22230
Submitted: 15 June 2022 Accepted: 26 August 2022 Published: 29 November 2022
© 2023 The Author(s) (or their employer(s)). Published by CSIRO Publishing. This is an open access article distributed under the Creative Commons Attribution-NonCommercial-NoDerivatives 4.0 International License (CC BY-NC-ND)
Abstract
Context: Steadily increasing consumption of chicken meat (Australia’s most consumed meat protein) has resulted in expanded production. With societal expectations that industries improve sustainability, understanding baseline impacts is vital.
Aims: This study determined carbon footprint (kg CO2-e), fossil energy (MJ), fresh water consumption (L), stress (L H2O-e) and scarcity (m3), and land-occupation (m2) impacts for conventional (C) and free-range (FR) production systems, identified hotspots and the implications of changes in production over the past decade, to establish targets for future improvement.
Methods: In the largest study of its kind, attributional life-cycle assessment with data collected for ~50% of birds processed was used, reporting impacts per kilogram of the typical market mix of chicken products, and boneless chicken. Uncertainty was assessed through Monte Carlo analysis, and results are presented as the means and standard deviation.
Key results: Slightly lower impacts per kilogram of chicken meat product were observed for C production (2.1 ± 0.03 kg CO2-e, 18.0 ± 0.3 MJ, 178.6 ± 22.4 L, and 10.2 ± 0.1 m2) than for FR (2.2 ± 0.03 kg CO2-e, 18.5 ± 0.3 MJ, 189.6 ± 24.6 L, and 10.6 ± 0.1 m2). Feed production was the major hotspot, followed by grow-out and meat processing. Land use (LU) and direct land use-change (dLUC) impacts associated with imported soymeal added 1.7 ± 0.3 and 1.8 ± 0.3 kg CO2-e to C and FR respectively. FR carbon footprint and land occupation were significantly (P < 0.05) higher. Since 2010, fossil energy, arable land, and greenhouse-gas emissions have declined. One countertrend was LU and dLUC emissions, which increased due to changed soy imports, resulting in a slightly higher C carbon footprint.
Conclusions: Multi-indicator analysis is fundamental to understanding, communicating, and improving performance, and distinguishing between short-term fluctuations and long-term trends. Since 2010, feed-production impacts have increased (due to imported soymeal in poultry diets), indicating that alternative feed protein sources are a priority. Efficiency improvements reduced per-kilogram impacts across other indicators, demonstrating a positive trend in producing more food from fewer inputs.
Implications: Australian chicken meat is a low-impact animal protein. Future improvements require alternative feed proteins, technology adoption and practice change to maintain or reduce impacts as production expands alongside consumer demand.
Keywords: carbon footprint, chicken meat, energy, greenhouse gases, land use change, life cycle assessment, sustainability indicators, sustainable agriculture, water stress.
Introduction
The environmental sustainability of industries and food products has become a key focus for consumers and governments worldwide. Sustainability covers a broad range of topics, from the purely environmental to socio-economic issues (WCED 1987). This is reflected in how, although climate action is the most prominent sustainability issue, energy, water and land are also priorities in Australian life-cycle assessment (LCA) of agri-food products (Harris and Narayanaswamy 2009).
LCAs of chicken meat have, in multiple regions of the world, found that it is a low-impact meat product (see Williams et al. 2006; Lesschen et al. 2011; Leinonen et al. 2012; Clune et al. 2017). For Australian production, Wiedemann et al. (2017) reported greenhouse-gas (GHG) impacts that were similar or slightly lower than those for chicken meat overseas, with the majority of impacts arising from feed production, meat processing and the grow-out phase for the birds. The study used data from 2008–2009 and covered two major supply chains but omitted some major production regions and was therefore incomplete as a baseline for the industry. Shifts in production region, productivity and feed sources over the subsequent decade are expected to have changed the environmental footprint of chicken meat, and further insight is needed to understand change over time before considering potential reduction targets into the future.
In 2021, the Australian Government made a commitment to deliver net-zero economy-wide emissions by 2050. The pathway, outlined in Australia’s Long-Term Emissions Reduction Plan (LTERP; Commonwealth of Australia 2021a), is technology-driven and aims to facilitate emissions reduction in all sectors of the economy by reducing the cost of existing and emerging technologies and accelerating their adoption. Understanding the contribution of fossil energy to the environmental footprint of the Australian chicken meat industry, and how it compares with other sources of impact, is a first step in assessing how and to what extent the industry can rely on the LTERP and decarbonisation of electricity grids to reduce fossil energy consumption and GHG emissions.
A key challenge the industry faces is how to accelerate other viable emission reduction strategies and work with supply industries, such as the feed grains sector, to reduce impacts in the mid-term (2035) and long-term (2050). A core principle of the LTERP is that the transition to a net zero economy should not put industries, regions, or jobs in jeopardy. Therefore, the chicken meat industry faces an imperative to balance its economic and environmental priorities while continuing to provide high-quality food. In response to this, the industry is in the process of developing a sustainability framework to assess, report and track change in performance. Critical to the success of the framework is the establishment of industry-wide benchmarks across a range of environmental categories, identification of factors contributing to these impacts, understanding past performance, and ongoing reporting to track performance over time.
While action on climate change is an imperative, assessing a broader suite of indicators is beneficial to bring a more holistic approach to improving performance, and to avoid unintended burden-shifting. Water consumption, stress and availability are major issues in the Australian environment and counter-cyclical consumption and availability (i.e. where consumption is greatest when availability is lowest) can have far-reaching consequences (Mekonnen and Hoekstra 2016). Although the chicken meat industry is responsible for water consumption and stress impacts, it is also exposed to impacts arising outside its operation control in other industries, most notably via feed grains. Similarly, land occupation is an environmental priority where, although the chicken meat industry has a direct contribution to land occupation and management, it is exposed to impacts arising from outside its operational boundary.
This study provides a contemporary baseline for the Australian chicken meat industry and focussed on environmental impacts as one key area of sustainability. This baseline is fundamental to the identification of major impact sources, the investigation of mitigation strategies and to supporting future improvement in industry performance in the face of emerging market and economy-wide priorities.
Materials and methods
Goal and scope
The study was an attributional LCA of Australian chicken meat production from conventional (C) and free-range (FR) housing systems. All major production regions were assessed using primary industry data, representing nearly 50% of birds processed in Australia.
This study aimed to (i) determine carbon footprint, fresh water consumption (water footprint) and water stress, fossil energy and land occupation impacts for Australian C and FR chicken meat production, (ii) assess impact hotspots in the supply chain, and (iii) compare trends over the past decade. This information will be used in subsequent work to determine impact reduction pathways for the Australian industry.
Impact assessment
The study assessed GHG emissions using the IPCC AR5 global warming potentials (GWP100) of 28 kg CO2-e/kg CH4 and 265 kg CO2-e/kg N2O as applied in the National Greenhouse Accounts Factors (Commonwealth of Australia 2021b). GHG emissions associated with land use (LU) and direct land-use change (dLUC) were included and reported separately, as recommended in ISO 14067 (2018).
Demand for fossil fuel energy was assessed by aggregating all fossil fuel energy inputs throughout the system and reporting these per megajoule (MJ) of energy, using lower heating values. Land occupation, reported in square metres (m2), was assessed by aggregating impacts throughout the supply chain.
As described in the following sections, freshwater consumption (L) was assessed using methods consistent with ISO (2014). Stress-weighted water use was assessed using two methods, namely, the water-stress index (WSI) (Pfister et al. 2009) and the available water remaining (AWARE) method (Boulay et al. 2018). Both methods aim to quantify the impact of water consumption, on the basis of the relationship between total freshwater consumption and freshwater availability. Areas with scarce water resources and/or high demand have high levels of water stress.
While several other environmental indicators could be assessed in addition to those selected, this study covered the impacts that were deemed most significant for Australia, and that have high weightings in international systems, such as the Product Environmental Footprint (Directorate-Generale for Environment 2021). Indicators that are less mature in the Australian context, including eutrophication, eco-toxicity and impacts on human health, were not covered at this time but future work in these areas would be warranted to provide a more comprehensive environmental analysis.
All modelling was performed using SimaPro™ 9.3 (Pré-Consultants 2021).
Supply chains, system boundary and functional units
Production from the following two housing systems operating in six Australian states was investigated: C housing (indoor housing with tunnel ventilation); and FR, where birds are given access to an outdoor range area once adequately feathered (on average, after 21 days). The FR supply chain included farms in four of the six states to provide a sufficiently large dataset.
The primary production supply chain included breeding (rearing of parent birds, fertile egg production and hatchery processes), grow-out and meat processing, with all associated inputs. Inventory data were not collected for grandparent and great grandparent breeding systems as Wiedemann et al. (2017) found that these systems contributed <1% of impacts.
As part of the study, data were collected from seven major vertically integrated poultry producers to cover a 12-month production period from 1 July 2019 to 30 June 2020. Data were de-identified and aggregated to ensure that company data remained confidential. Australian average processes were developed for feed milling, meat processing, and breeding and hatchery operations. State averages were devised from the inventory data, including for grow-out farming and ration composition, and were then weighted according to each state’s contribution to total industry production (Commonwealth of Australia 2021c). In the FR supply chain, where inventory data for rations and grow-out farming were not available for all states, an average of the relevant data was used to avoid unduly weighting against states for which full datasets were not available.
The endpoint of the supply chain is the cold storage unit where chicken meat is stored prior to wholesale distribution (Fig. 1). Results are presented relative to the production of 1 kg of chicken meat product ready for distribution to retail. The functional unit reflects the retail product mix, including whole birds, bone-in and boneless chicken meat portions. In addition, results are reported per kilogram of boneless chicken portions, ready for packaging and distribution to retail.
Life-cycle inventory
The study collected data from a representative cross-section of the industry, representing some 50% of supply chains in all major regions, and included meat processing, grow-out, feed milling, breeding and hatching. Inventory data were collected for the separate supply-chain stages, and methods are described in the following sections. Background databases used to model major inputs (excluding feed inputs) are reported in Table S2.
Feed use and milling
Ration data for meat chickens were reported for each company, and existing datasets were used for breeder feed composition. All companies reported commodity inputs for their feed, and those that operated their own feed mill reported energy and water use and transport distances (Table 1).
![]() |
Birds are phase fed, and diets may change during the year due to changes in the availability of commodities. The aggregated meat chicken rations are described in Table 2, where less commonly used inputs (although modelled individually) are grouped under general headings for conciseness.
![]() |
Feed production
Major feed grains were modelled from Australian grain processes from the AusLCI database (ALCAS 2017), where available (see Table S1). These processes included emission factors from the Australian National Inventory Report (NIR; Commonwealth of Australia 2021d). Average irrigation rates in each region and the proportion of cropland irrigated were used to determine the proportion of cereal grains produced in dryland and irrigated systems (ABS 2021a, 2021b). Grain processes were then aggregated to build an average market process for the major cereal grains produced in each state. Each process included the supply losses associated with the provision of irrigation from rivers, bores, and dams. This process was performed for the 2018–2019 and 2019–2020 financial years and then averaged. This average of two financial years was preferred as it recognised that cereals used in meat chicken feed in a given year were not necessarily produced in that year but may have been surplus to requirements in the previous year and stored.
Accounting for LU and dLUC emissions was difficult, as datasets were not available that disaggregated these emissions by crop, as noted by Sevenster et al. (2020). To understand the impact of a change in soil carbon, the minimum recommended analysis period is one full crop rotation (ISO 14067 2018); however, considering that carbon changes occur over longer time periods influenced by management and season, a longer averaging period may be warranted. In the present study, national average datasets from the NIR (Commonwealth of Australia 2021e) were used to determine emissions and sequestration over the 5 years preceding the inventory time period (2015–2019), reflecting the recent change in soil carbon attributable to cropping. Total carbon change over this time period was summed, amortised and reported as an annual value for 2020. This was considered appropriate to avoid unusual seasonal influences on carbon sequestration or loss. Sequestration was observed in the most recent 5-year period of 2015–2019 (−135 kg CO2-e/ha, negative valuing reflecting removal of CO2 from the atmosphere). This change may be reflective of long-term changes in crop management in Australia, from cultivation to zero tillage and stubble retention, which can increase soil carbon concentrations (Luo et al. 2010). The effect of assessing a 2-year (with sequestration rate of −145 kg CO2-e/ha) and 10-year (with rate of carbon loss of 34.2 kg CO2-e/ha) time sequence was considered as part of the sensitivity analysis.
Imported soybean meal was modelled (see Table S1) using data from the ecoinvent database (ecoinvent 2020) where South America was the dominant market (98%), dwarfing the next largest source, the United States (US), which accounted for only 0.2% of imported soybean meal in the Australian market (OEC 2019).
Where model processes were unavailable for some small dietary inputs constituting less than 3% of the diet, substitutions were made with other feed inputs. As described by Wiedemann et al. (2012), inputs requiring substitution were typically low-cost products associated with low levels of manufacturing and high-cost products that were typically associated with high levels of manufacturing and international transportation. The substitution ratio was informed by economic value and known processing requirements; low-cost inputs (e.g. salt) were substituted for other mined products such as lime, and high-cost inputs (e.g. enzymes) were substituted for synthetic amino acids.
Breeding and hatching
Data were collected from a limited number of producers and augmented with previous industry datasets (Wiedemann et al. 2017). Major inputs are shown in Table 3. Water use was collected from farm records and water was predominantly used for drinking and cleaning. After ingestion, drinking water was respired, excreted with manure or integrated into the bird or egg and it was therefore treated as a consumptive use. Likewise, cleaning water was considered freshwater consumption; small volumes were used, and sheds were left to dry out after cleaning, resulting in evaporation. Where water was supplied from a system incorporating open water storages, evaporative losses were assessed and included in the total freshwater consumption. Each of these flows resulted in consumption or removal of water from the original catchment.
![]() |
Meat chicken grow-out phase
Flock performance, including feed intake and the total mass of birds harvested, was determined from records supplied by each company and it represents the actual performance under commercial conditions (major inputs shown in Table 4). In Australia, the grow-out phase is typically contracted out to third-party growers responsible for animal husbandry, housing, and litter management. Records of bird numbers, water use, and energy use were collected from FR and C farms operating in five states. Drinking and cleaning water were handled as described for the breeding flocks. Water used in evaporative cooling systems was treated as a consumptive use.
![]() |
Manure management
A mass balance, based on feed and bird production data, was used to estimate manure excretion. Manure GHG emissions (methane [CH4], nitrous oxide [N2O]) and indirect emission precursors (ammonia [NH3]) were estimated by predicting nitrogen (N) and volatile solids (VS) excretion by using mass-balance principles and by applying emission factors for birds housed on litter from the NIR (Commonwealth of Australia 2021d), including state-specific factors for manure CH4 and emissions from range areas. Subtracting N inputs (in feed) from N outputs in bird mass, mortalities and eggs (in the case of breeders) gave excreted N. Excreted VS was determined by subtracting manure ash from excreted total solids (TS), which represented the residual of non-digested feed (Dong et al. 2006). N retention was determined using methods and factors determined by Wiedemann et al. (2016a). Briefly, retention was determined using liveweight data from farms and poultry composition data (Wiedemann et al. 2016a; see Table S3).
Manure was typically removed from the site and sold as a low-value fertiliser and soil-amendment product and was therefore treated as a residual, meaning no allocation process was applied, and impacts from manure following removal from the sheds were assumed to be attributed to the system using it as a fertiliser (LEAP 2016). As grain production occurred outside the system boundary and tracing manure application and displaced synthetic fertiliser through to grain produced for chicken meat feed was not feasible, no reduction in synthetic fertiliser was modelled, which was a conservative assumption.
At the farm level, indirect N2O was modelled from NH3 volatilisation. All sheds were assumed to be constructed with impervious floors according to environmental regulations, and therefore nitrate leaching from sheds was assumed to be negligible. Leaching and runoff in range areas (Commonwealth of Australia 2021d) were assessed for the fraction of manure (20%) deposited in the FR area (Zeltner and Maurer 2009; Commonwealth of Australia 2021d).
Meat processing
An average process (Table 5) was developed from inventory data collected from meat-processing plants (n = 10) operating across six states. All plants used water from reticulated supplies. Two meat-processing plants had covered anaerobic ponds, one of which utilised the biogas to generate heat energy. Most plants utilised dissolved air-flotation systems instead of uncovered ponds and treated effluent water was typically released to sewer or, in limited cases, used for irrigation.
![]() |
The processing plants reported inputs relative to carcase weight, but all produced a combination of chicken products, including whole birds and both bone-in and boneless portions. We report inventory inputs relative to carcase weight, but results are reported relative to the average product mix for chicken meat leaving the processing plant as an average across the industry, which was 0.51 whole birds, 0.16 bone-in chicken pieces, and 0.33 boneless chicken pieces.
Handling co-production
Total product mass from the system was inclusive of meat chickens, small volumes of meat from end-of-life breeding hens, and edible offal. Co-products included manure, pet food and processing by-products for rendering (co-products from feed inputs arose outside the system boundary). Because manure is a very low-value output from the system, it was treated as a residual, as described previously. Meat processing by-products (renderable products, pet foods) were handled using economic allocation, as recommended in the LEAP Guidelines (LEAP 2016; comparison between allocation methods was performed by Wiedemann et al. (2012)). Wiedemann and Yan (2014) previously described product mass flows and edible yields. In this study, the economic value of primary products represented 98.5% of the output from meat processing, with the renderable and pet food products contributing the remaining revenue. Impacts reported per kilogram of chicken meat product were determined using yield factors provided by each processing plant.
To determine impacts per kilogram of boneless chicken meat, product volumes of whole dressed birds, and bone-in and boneless portions reported by each processing plant were used to derive the average Australian product mix at the primary processing plant gate. Edible yields for the whole dressed birds and bone-in portions were then determined from factors reported previously (Wiedemann and Yan 2014). The weighted average factor by which to upscale results was then calculated on the basis of the proportion of each product type in the market mix. It should be noted that the functional unit is then 1 kg of boneless chicken meat at the primary processing plant gate. The proportion of whole birds that leave primary processing for secondary processing was not included in this study.
Sensitivity analysis
The sensitivity of the model and results to key assumptions and parameters was tested. Methodological details for the sensitivity analyses are outlined in the Sensitivity analysis methodology in the Supplementary materials.
Uncertainty analysis
After Leinonen et al. (2012), two types of uncertainty (alpha and beta) in the input variables were considered. Alpha uncertainty relates to variations among vertically integrated processing networks, reflecting variation in the inventory data collected, whereas beta uncertainties relate to uncertainties in the model and background processes.
Monte Carlo analysis in SimaPro (Pré-Consultants 2021) was used to assess alpha and beta uncertainty, using one thousand iterations to provide a 95% confidence interval for the results. Results for C and FR production are presented using the means and the standard deviation, and both alpha and beta uncertainties were used to calculate the standard deviation.
Alpha uncertainties included all grow-out farm input and production data, and housing-specific manure factors, and source regions for cereal grains. Beta uncertainties included meat processing energy and water inputs, feedmilling energy and water inputs, feed composition, breeding and hatchery operation processes.
Comparison of the mean results between C and FR was based on alpha uncertainties only, as beta uncertainties were shared between both systems. Significant differences were determined using the equation from Wiltshire et al. (2009):
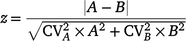
where, A and B are the mean values and CVA and CVB are coefficients of variance between the two systems.
Results
Impacts per kilogram of chicken meat product
GHG emissions (excluding LU and dLUC) were 2.1 ± 0.03 kg CO2-e/kg chicken meat product for C production and 2.2 ± 0.03 kg CO2-e/kg for FR production (Fig. 2). Emissions from LU and dLUC were 1.7 ± 0.3 kg CO2-e/kg chicken meat product for C and 1.8 ± 0.3 kg CO2-e for FR. LU and dLUC emissions were dominated by high levels of soil carbon loss associated with the production of soybeans on recently converted cropland in South America. Carbon sequestration in Australian cropland amounted to −0.06 kg CO2-e/kg chicken meat product, equivalent to ~2.5% of net emissions per kg of product. The carbon footprints (including LU and dLUC) were, therefore, 3.8 ± 0.3 and 4.0 ± 0.3 kg CO2-e/kg chicken meat product for C and FR respectively.
![]() |
Feed production represented the largest contributing stage for GHG emissions, with impacts ranging from 58% to 61% excluding LU and dLUC, and from 77% to 79% of net LU and dLUC. Emissions from the grow-out phase were 17–21% of the total, excluding LU and dLUC, or 9–12% including LU and dLUC, where energy use for housing and manure emissions were the major contributors. For FR production, emissions from manure (deposited on ranges and in sheds) accounted for almost half of GHG emissions during grow-out, compared with a quarter for C (indoor only) production (excluding LU and dLUC). Meat processing contributed 14–15% excluding LU and dLUC (7–8% incl LU and dLUC), where the major emission sources were fossil energy consumption and effluent treatment, with the latter representing a smaller portion of impacts. Breeding and hatchery operations accounted for 7% of emissions (excluding LU and dLUC), or 4% where LU and dLUC emissions were included.
Fossil energy use was 18.0 ± 0.3 and 18.5 ± 0.3 MJ/kg chicken meat product for C and FR production respectively. Feed production accounted for 61% of fossil energy use (averaged across C and FR, which did not differ substantially), primarily from field operations and energy associated with fertiliser manufacture. Meat processing (13%) and housing (20%), averaged across C and FR, were also substantial contributors to fossil energy use, with breeding and hatchery operations accounting for the remaining 6%.
Freshwater consumption ranged from 178.6 ± 22.4 to 189.6 ± 24.6 L/kg chicken meat product for the C and FR production systems. Water used for feed production, predominantly for irrigation, accounted for 88–89% of freshwater consumption per kilogram of chicken meat product, averaged across C and FR, as they did not differ substantially. Drinking and cooling water provided in the grow-out phase constituted 5–6%, and meat processing a similar proportion, whereas breeding and hatchery operations (1%) represented a smaller source of consumption.
Using the WSI method, stress-weighted water use was 128.0 ± 14.5 and 140.8 ± 14.5 L H2O-e/kg chicken meat product for C and FR production respectively. Irrigation water represented 91% of the stress-weighted water use, averaged across C and FR. Using the AWARE method, water scarcity was 23.1 ± 1.7 and 24.5 ± 2.2 m3 for C and FR production. The majority of water scarcity (67–68%; representing slightly more in FR production) was attributable to feed production. Grow-out (15%), meat processing (11–12%), and breeding operations (6%) accounted for significantly smaller portions of the scarcity.
Arable land occupation for C chicken meat production was 9.7 ± 0.1 m2/kg chicken meat product, and 10.1 ± 0.1 m2/kg chicken meat product for FR production. When the small amounts of land occupied by other supply chain stages (housing, processing, breeding) were included, total-land occupation was 5% higher than arable-land occupation.
Impacts per kilogram of boneless chicken portions
Impacts per kilogram of bone-adjusted (boneless) chicken portions are shown in Table 6. Results were 25% higher than for the chicken meat product mix because of the loss of mass associated with bones.
![]() |
Differences between production systems
Across all impact areas, mean impacts were found to be modestly higher in FR production than in C, but the only statistically significantly (P < 0.05) different impact categories were GHG emissions and land occupation.
Statistically significant results for land occupation and GHG emissions were driven by higher feed conversion ratios (FCRs) in the FR system, meaning higher volumes of grain (and more cropland) were needed to produce 1 kg of chicken meat which, in turn, increased emissions associated with feed and led to greater emissions from manure. Emissions from manure deposited on ranges were also unique to the FR system.
Sensitivity analysis
The sensitivity of the results to production region and state grid emission intensity were tested and analysis showed that, for a model vertically integrated production system, the carbon footprint using the most efficient energy network (Tasmania, TAS) was 0.4 kg CO2-e lower than if the production system operated with the least efficient energy network (Victoria, VIC; Table S4), indicating the important role that the decarbonisation of the energy network has in reducing supply chain emissions for chicken meat.
The sensitivity of the results to FCR showed that a 0.1 improvement in FCR (relative to the Australian averages in Table 4) reduced impacts across all indicators in both conventional (C1) and free-range (F1) production (Table S5). GHG (excluding LU and dLUC) was 4% lower, LU and dLUC were 6% lower, fossil energy and water scarcity 4% lower, and land occupation, water stress and water consumption were 5% lower, than were the baseline results. Emissions from manure were directly correlated with FCRs. Further to this, the analysis showed that a 10% reduction in dietary crude protein reduced GHG emissions (excluding LU and dLUC) by 0.3% for FR (F2) when there was no change in FCR.
In this study, 11% of cereal grains in meat chicken feed for processing companies operating on the eastern seaboard were shipped from Western Australia due to drought-driven local supply shortages. For some individual regions, the amount was up to 25%. The sensitivity of this was tested (Table S6) by comparing a scenario for a model vertically integrated processor based in Queensland (QLD) that sourced 25% of wheat from Western Australia (WA), in comparison to a more typical year when all grain was sourced locally (Scenario A). The sensitivity of the results to a for the combined eastern seaboard grain supply (i.e. a combined QLD, New South Wales (NSW) and VIC grain market) was also tested (Scenario B).
The sensitivity of the results to methodological choice of a 5-year analysis period for soil carbon change in Australian cropland was tested through comparison with a 2-year period and a 10-year period. Analysis showed that, for the 2-year period of 2018–2019, carbon sequestration in Australian cropland was slightly greater at −0.07 kg CO2-e/kg chicken meat product, but that the 10-year period of 2010–2019 was associated with soil carbon losses equivalent to 0.02 kg CO2-e/kg chicken meat (Table S7).
Discussion
In Australia, chicken meat represents nearly 50% of meat consumption per capita and has been the most consumed meat protein for over a decade (ACMF 2020). The industry has expanded by 40% over that time, and with that growth comes the challenge of sustaining output while reducing environmental impacts. Achieving this balance will be aided by gains made elsewhere in the economy, but the most significant determinants will be the ability of the industry to reduce emissions, energy, and water consumption, particularly in upstream feed production. Improving performance requires a thorough understanding of impacts, as described in the following sections.
Main impact sources in the supply chain
For both C and FR systems, feed production was the major impact source across all environmental indicators. Of this, cereals were the major contributor to all indicators, except for LU and dLUC, where soymeal was the major commodity driving emissions. This study found that the average emission intensity of feed was 0.45 kg CO2-e/kg (or 1.09 kg CO2-e/kg, including LU and dLUC), compared with 0.36–0.46 kg CO2-e/kg (or 0.38–0.88 kg CO2-e/kg, including LU and dLUC; Wiedemann et al. 2017), adjusted to AR5 values. The emission intensities of feed, excluding LU and dLUC, in a study of UK production (Leinonen et al. 2012) were approximately twice (1.11, 1.03 and 0.91 kg CO2-e/kg) that of this study, and feed production in that study, still the major emission source, represented slightly more of the total GHG impacts (70%).
A key driver behind the difference in emission intensity of feed (excluding LU and dLUC) between this study and the 2010 study was the increased N2O emissions from feed grain production identified over the past decade. Brock et al. (2012), studying grain production in 2010, found that the emission intensity of wheat produced in central New South Wales (NSW) was 0.2 kg CO2-e/kg, whereas Simmons et al. (2019) reported the intensity as 0.315 kg CO2-e/kg (both in AR4 values) for wheat from the same region, an increase of more than 50% reported 8 years later. This finding did not necessarily reflect the actual increases in emissions, rather a better insight into key emission factors and production systems, resulting in higher reportable emissions for cereal grain. More generally, Simmons et al. (2019) reported the emission intensities of cereals produced in 14 regions, all of which were higher than that reported by Brock et al. (2012) in their single-region case study. This suggests that the reported emission intensity of Australian wheat is higher than was previously understood, resulting in an underestimate of impacts in previous studies. At this time, the Australian feed grain sector is yet to release a strategy for emission reduction, meaning that there is no short-term indication on whether a change in the emission intensity of feed grains (and the emission intensity of meat chicken feed) can be expected.
The water and land footprints of chicken meat production were also highly exposed to upstream impacts from feed production. As most land occupation was attributable to arable uses, the key variable influencing arable land use was crop yield, which was influenced by source region and use of irrigation (data not shown). Irrigation of grain resulted in a trade-off between water and land, which trended in different directions when comparing irrigated and non-irrigated grain (high irrigation led to high water consumption but lower arable-land occupation, and vice versa). Accordingly, water consumption, stress-weighted water use in the supply chain, and the emission intensity of feed exhibited considerable variation based on grain source regions (data not shown).
Although differences in freshwater consumption and water stress are inherent, given general climatic differences among source regions, the environmental impacts of grain and, by extension, chicken meat are significantly affected by inter-annual climatic variability. The freshwater consumption and water stress results also demonstrate the potential of inter-annual variability and the need for ongoing assessments to establish a clear trend and benchmark for environmental impacts. A severe drought on Australia’s eastern seaboard, beginning in early 2017, which saw rainfall levels at or below the previous record lows for a significant portion of NSW and southern QLD, was made worse by record-high temperatures that increased the evaporation rate of what water was available (BOM 2019). The years 2017–2019 saw the record-lowest rainfall for NSW and the Murray Darling Basin; record-low rainfall was also recorded in parts of VIC and WA (BOM 2020). This had significant repercussions for the average freshwater consumption and water stress in each state’s grain market, which flowed through to the chicken meat industry.
Drought conditions meant that the proportion of irrigated grain available for feed on the eastern seaboard was substantially and anomalously over-represented in comparison to years where rainfall was closer to the average. Water consumption in the NSW and QLD average wheat markets of FY18/19 and FY19/20 for meat chicken feed was 114.5 and 80.3 L/kg wheat respectively; water stress was 113.4 and 44.0 L H2O-e/kg respectively. This indicates that the regions in NSW from which wheat was sourced for meat chicken rations relied on higher levels of irrigation and water was considerably more scarce, to which the AWARE indicators (13.6 and 8.2 m3 respectively) align. In comparison, water associated with the average WA wheat market for meat chicken feed was 6.7 L/kg wheat, with a WSI of 2.7 L H2O-e/kg as the vast majority of WA wheat was grown in dryland conditions, even with the low rainfall recorded in southern WA.
Although domestically produced feed grains accounted for such a significant proportion of impacts, they were (except for water consumption and stress) of a relatively low impact compared with those of imported soybean meal. Demand for this low-cost, high-protein commodity was high, not only within the Australian chicken meat industry but also in the egg and pork industries (Willis 2003). With soybean meal being a major export commodity for South America, global demand has driven a rapid expansion and the conversion of pasture or forest to cropland (Arrieta et al. 2018), leading to substantial GHG emissions from dLUC.
As most imported soybean meal in the Australian feed market is now derived from South American production, compared with a decade ago where a greater volume came from the USA, imported soybean meal has become a significantly higher impact feed commodity (especially in terms of LU and dLUC), driving the difference between the LU and dLUC results from this study and those of Wiedemann et al. (2017). The difference in emission intensity between soybean meal produced in the USA (GHG emissions of 0.28 kg CO2-e/kg, and LUC of 0.0001 kg CO2-e/kg) and that produced in Brazil and Argentina (0.43 kg CO2-e/kg, and LU and dLUC impacts of 3.27 kg CO2-e/kg) is such that, even in supply chains where inclusion rates have fallen since 2010 and despite improvement in FCRs, impacts per kilogram of feed, and chicken meat (where LU and dLUC were included), are much greater (Ecoinvent 2020).
Utilising accredited soybean meal or alternative protein sources (e.g. canola meal, field pea, animal meals) as a partial or complete substitute for high-impact imported soybean meal would reduce LU and dLUC emissions. However, trade-offs and challenges may emerge as a result of doing so. First, either option is likely to result in higher cost rations, meaning that companies may need to investigate the feasibility of passing costs onto customers to maintain profitability, likely increasing the selling price of chicken meat. Second, some alternative proteins may reduce LU and dLUC impacts but increase impacts across other indicators; cottonseed meal, for example, is a by-product of a system with very high levels of irrigation, meaning that it has a very high water footprint. Third, sourcing difficulties may emerge as the availability of canola and cottonseed meals in Australia is variable. An alternative method, which would reduce net soil carbon losses from LU and dLUC and avoid at least some of the issues described here, is to improve soil carbon in cropland through, for example, changes to land management. However, applying the offset to chicken meat production would require industry-wide adoption or the ability to link feed grains used in meat chicken feed to a particular enterprise that is actively storing soil carbon.
Emissions and sequestration reported in the Australian NIR showed no change in average annual emissions associated with the conversion of forestland to cropland (described here as dLUC). Given that soil carbon concentrations may vary significantly year-to-year, averaging over a long timeframe (e.g. the 10 years in the sensitivity testing) may disguise variability and be less representative of soil carbon change for a specific analysis period (Sevenster et al. 2020). In the most recent 5 years to 2019, soil carbon losses from dLUC in Australia have moderated and sequestration in crop soils has increased, resulting in net sequestration of −135 kg CO2-e/ha or −145 kg CO2-e/ha for the 2-year period of 2018–2019. The net effect per kilogram of chicken meat did not differ substantially under either time frame (see section ‘Sensitivity analysis’). Given uncertainty as to whether the sequestration (and benefit to net emissions per kilogram of chicken meat) will continue over time, and the magnitude of the LU and dLUC impacts from imported soybean meal, the scope for reducing these emissions to reduce the carbon footprint of chicken meat is (on the basis of the current data) largely confined to soybean meal.
As a key efficiency and cost indicator, FCR was also found to be a significant factor influencing environmental performance. Any improvement in FCR reduced impacts across all indicators (see Table S5). Accordingly, a high FCR resulted in higher impacts across all categories. However, for an individual vertically integrated processor, the effects of FCR improvement were not uniform due to the influence of other factors, such as climate (see section ‘Regional differences’).
The most significant contributions to carbon footprint, energy, and water consumption, after feed production, occurred at the grow-out farm and meat-processing plant. A major contributor to GHG, and an environmental indicator itself, was fossil energy. Many growers drew a proportion of their energy requirements from renewable sources (almost exclusively solar), but this accounted for only a small portion of the total industry energy demand. Aside from drinking water, the major consumptive use of water at the farm was for cooling. Innovations or management changes in cooling, such as combined sprinkler and evaporative cooling pads (Dunlop and McAuley 2021) may offer reductions in on-farm water consumption.
At the meat processing plant, average energy, water and GHG impacts declined slightly compared with the 2010 study, indicating improved plant efficiency.
Comparison with previous Australian study
The previous Australian study reported environmental impacts for chicken meat production in 2008–2009, covering two of the five major production states (Wiedemann et al. 2017). While these previous results did not cover the breadth of the industry, it was nonetheless informative to investigate differences between the studies using the standard unit of 1 kg boneless chicken meat.
Impacts were found to have declined for energy, arable land occupation, and there was a slight decline in carbon footprint (excluding LU, dLUC) for C production, per kilogram of boneless meat, but not when LU and dLUC were included. The reduction in fossil energy consumption was attributable to energy efficiency improvements and increased solar adoption, particularly on farm, whereas the reduction in land use was largely driven by the introduction of higher-yielding grain markets, particularly in VIC, compared with only South Australia (SA) and QLD a decade ago. Yields for wheat and barley in VIC in 2019–2020 were 2.48 and 3.05 t/ha, and 1.1 and 1.2 t/ha in NSW, compared with 1.05 and 1.33 t/ha in QLD and 1.32 and 2.24 t/ha in SA (Australian Bureau of Agricultural and Resource Economics and Sciences 2020), meaning that, on average, both wheat and barley in this study were produced on less land than in the previous analysis period.
Freshwater consumption and stress-weighted water use were higher than in 2010 (Wiedemann et al. 2017). The present study is an average of a greater number of state markets for feed grains and a greater number of catchments. However, water stress and consumption in QLD and SA were also found to have increased over the decade, contributing to the higher results in this study. Drought in the analysis period of this study (and in the same regions assessed previously) is likely to have skewed the results substantially, meaning that the two studies do not provide meaningful insight into any change in the water impacts associated with Australian chicken meat. The previous study did not report AWARE water.
Overall, results suggested that despite improving FCRs since 2010 (evident in inventory data from 2010 and 2020 and in industry monitoring; ACMF 2020), evidence of renewable energy adoption, and energy-efficiency gains at meat processing plants, the environmental footprint of chicken meat is critically reliant on the footprint of the feed grain sector and South American soy production, and increased impacts from these sources resulted in marginally higher impacts than in the case studies from the 2010 study. However, there are three major factors that must be considered when evaluating this outcome. First, the present study assessed the whole country, while the 2010 study reflected two regions only and utilised data from a small number of farms supplying a total of five processing plants, operating in QLD and SA. Second, in light of insights from new research in the Australian grain sector, impacts from feed grain may have been under-estimated in the previous assessment. Third, severe drought in the analysis period had wide-reaching effects and, so, although these results are representative of the environmental impacts of Australian chicken meat production in the 2019–2020 financial year, and provide a baseline for the industry, ongoing assessment is required to establish a trend of environmental performance and to determine any anomalies. Ultimately, it is apparent that ongoing productivity improvements are no guarantee of sustained environmental improvement, highlighting the challenge for industries to reduce emissions upstream impacts may result in countertrends.
Regional differences
Differences in fossil energy and water consumption were observed among the regions assessed (data not shown). The contribution of the state electricity grid was heavily influenced by its emission intensity, i.e. the proportion of energy derived from fossil fuels compared with renewable sources (see Senstivity analysis methodology for detail and Table S4 for results of the sensitivity analysis).
At the farm (breeder or grow-out), climatic conditions, including humidity levels, were a major influence on water consumption (data not shown). Farm water consumption was highest in states that experience hotter weather due to the need for cooling to maintain ideal optimal conditions for birds; consumption should be monitored on a regional basis to allow for identification of atypical consumption within similar climates. Similar to water, energy demand varied among regions and climates; higher energy demands due to heating requirements were typically reported by producers operating in states with cooler weather (data not shown).
Some regional differences in feed composition were also observed. However, whether rations were wheat- or sorghum-based did not have a substantial effect on environmental impacts. Whether irrigated or non-irrigated grain was used was the greatest driver in regional differences among the environmental impacts of feed production. In addition to the implications for water consumption and stress, the drought conditions on the eastern seaboard also meant that some supply chains transported at least some of their cereal grains by sea from WA. This was atypical and may have slightly elevated the emission intensity of feed used by those companies while lowering the water intensity; wheat produced in predominantly dryland production in WA had a higher emission intensity (0.37 kg CO2-e/kg) than did wheat from NSW (0.29 kg CO2-e/kg), even before trans-national transport was considered.
Comparison with international LCA results
Wiedemann et al. (2017) noted that the comparison of LCA studies is confounded by differing system boundaries, assessment methods, and assumptions. For GHG, the comparison of the results will not include LU and dLUC impacts, as these were not typically assessed in the comparison studies.
On a liveweight basis, per kilogram, impacts in this study were 1.8 ± 0.02–1.9 ± 0.02 kg CO2-e, similar to those in recent Japanese (Ogino et al. 2021) and Serbian (Skunca et al. 2018) studies, but lower than the value reported in a Brazilian study (da Silva Lima et al. 2019). Per kilogram of meat product, results were also lower than the equivalents (2.5–5.4 kg CO2-e) from a study of Iranian meat chicken production (Kalhor et al. 2016), although liveweight results from this study were higher than those in that study. Results presented here were lower than the global average of 4.12 kg CO2-e/kg bone-free chicken meat (excluding LU and dLUC) reported by Clune et al. (2017) in their meta-analysis. Aside from these more recent publications, liveweight results were lower than in another Australian LCA (2.6 kg CO2-e, reported as 2613 kg CO2-e/t LW; Bengtsson and Seddon 2013), and impacts per kilogram of meat product were lower than those reported for the UK (Leinonen et al. 2012) and Tunisia (Ibidhi et al. 2017).
Differences in the emission intensity of feed are the key driver of the comparatively low emission intensity of Australian chicken meat. The average emission intensity (excluding LU and dLUC) of meat chicken feed in the present study of 0.45 kg CO2-e/kg feed, although, on average, higher than that in the 2010 study, was half of those reported by Leinonen et al. (2012) for their standard and free-range rations (1.03–1.11 kg CO2-e/kg feed), and roughly a third of the intensity in Bengtsson and Seddon’s (2013) study (1.9 kg CO2-e/kg feed). Feed commodities produced in Australia (i.e. commodities excluding imported soybean meal or synthetic additives) are typically lower-impact products due to zero-till farming and low rates of fertiliser application, corresponding with comparatively low emission factors. Although crop yields are also comparatively low as a result, zero-till farming is the norm in Australia, meaning that the fossil energy footprint per 1000 kg of grain is significantly lower than that in other production systems. Despite the increase in the reported emission intensity of Australian wheat (Brock et al. 2012; Simmons et al. 2019) between this study and that of Wiedemann et al. (2017), it remains a lower-emission commodity than is produced in most northern hemisphere systems.
Fossil energy consumption per kilogram of LW in this study was 15.7 ± 0.2 and 16.2 ± 0.2 MJ/kg LW for C and FR production. Similar to the value given for the USA (Pelletier 2008), these values fell slightly outside the upper bound of the range reported for Serbian production (Skunca et al. 2018) and were lower than the reported value for Japanese production (Ogino et al. 2021). Per kilogram of chicken meat product, fossil energy consumption was slightly lower than or consistent with the slaughterhouse and meat processing plant equivalents for Brazil and for the UK (Leinonen et al. 2012).
Where water footprints were reported, these were found to vary significantly. For Australian production, freshwater consumption per kilogram of chicken meat product was higher than the water depletion values for whole roast chickens (52–60 L; Bengtsson and Seddon 2013). The water footprint, dominated by water used in feed production, reported for Tunisian chicken meat production (6030 L/kg chicken meat) was substantially higher than that in this study (Ibidhi et al. 2017). Excluding water associated with feed production, consumption in this study was 20.4 ± 2.6 and 20.8 ± 2.7 L/kg chicken meat product, higher than the equivalents for UK (Leinonen et al. 2012) and Brazilian (Skunca et al. 2018) production.
Other than our previous study, no studies were found that have reported stress-weighed water use using either WSI or AWARE factors. However, as noted by Wiedemann et al. (2017), the values from this study would presumably be greater than the equivalents for production in cooler climates, as there is a greater demand for and scarcity of water than in several other regions.
Due to significantly lower crop yields in Australia, the land occupation footprint of Australian chicken meat was also slightly higher than for Tunisian production (Ibidhi et al. 2017) and, despite a slight reduction over the decade since Wiedemann et al. (2017) did their assessment, the footprint was still higher than for UK production (Leinonen et al. 2012).
A further factor contributing to the comparatively low emissions from Australian chicken meat production is that the Australian National Greenhouse Accounts (Commonwealth of Australia 2021b), due to low moisture levels around poultry sheds and N deficiencies in Australian soils, applies a factor to determine indirect N2O (from NH3 volatilisation) that is an 80% lower factor than the default IPCC value (Gavrilova et al. 2019). This study relied on findings from Wiedemann et al. (2016a), who found that manure N2O emissions from grow-out litter-based housing were slightly higher than the IPCC value, but methane conversion factors were significantly lower.
The finding that FR production was a slightly higher-impact system than was C production was consistent with those of other comparative studies (Williams et al. 2006; Leinonen et al. 2012). Leinonen et al. (2012), in the only international chicken meat LCA found to report statistical differences, also used the approach outlined in Wiltshire et al. (2009). The authors found statistically significantly different primary energy use between organic and non-organic production, and that the higher carbon footprint in the organic system was statistically different from that of the standard indoor production system. No significant differences between the FR and indoor production systems were reported.
Comparison with other animal proteins
Per kilogram of boneless product, GHG emissions for chicken meat were lower than those of Australian pork and substantially lower than for Australian beef and lamb but higher than for shell- and protein-corrected eggs (Wiedemann 2018). Similarly, fossil energy was lower than the reported footprints for boneless beef, lamb and pork, but higher than shell- and protein-corrected eggs (Wiedemann 2018). Although arable-land occupation for boneless chicken meat was substantially higher than that for boneless beef and lamb (Wiedemann et al. 2015a, 2016b; Wiedemann 2018), total land occupation for boneless chicken meat production was far lower, consistent with the trend (although still higher than) identified for shell- and protein-corrected Australian eggs (M. A. Copley and S. G. Wiedemann, unpubl. data). Of the animal proteins compared here, stress-weighted water use (WSI) was lower than all but shell- and protein-corrected eggs; AWARE results were similar to (although still higher than) the equivalent results for shell- and protein-corrected eggs. Freshwater consumption was generally lower than for boneless beef and fell within a similar range for shell- and protein-corrected eggs. Therefore, compared with other Australian animal proteins, boneless chicken meat is one of the lowest environmental-impact products.
Greenhouse-gas mitigation
In light of the lack of improvement in the emission intensity of chicken meat over the past decade, mitigation strategies should be investigated, targeting major sources of emissions associated with chicken meat production. Technologies and management strategies targeting feed-related impacts include reducing inclusion rates of high-impact imported soybean via substitution with alternative protein meals or certified soy, reduced dietary crude protein (see Nahm 2007; Wiedemann et al. 2016a), and FCR improvement (see Mckay et al. 2007). Adoption of renewable energy or energy-efficiency measures (see McGahan et al. 2014) could be investigated to reduce fossil energy consumption and, by extension, emissions. Carbon storage and sequestration opportunities may arise from manure deposited on ranges, vegetation (see Ramachandran Nair et al. 2010; Doran-Browne et al. 2016) and application of spent litter to land. Reducing emissions from manure may be possible with the addition of litter additives (see Cockerill et al. 2020), or through strategies such as covering stockpiles (see Wiedemann et al. 2015b), and fertiliser replacement or nutrient recovery (see Szögi et al. 2008; Craddock and Hollitt 2010; Warn 2013). Waste-to-energy technologies, such as anaerobic digestion, combustion, gasification and pyrolysis, may be prospective options to better manage manure and generate energy (see McGahan et al. 2013).
A comprehensive assessment of all technologies and management strategies is needed to identify the technical mitigation potential, compatibility with other technologies or practices, and the economic implications of adoption. While some technologies are mature and proven in the Australian chicken meat industry (e.g. solar), others, even if adopted overseas, require further investigation to determine their viability.
In line with their emission-reduction targets, state governments have set targets to decarbonise energy grids via changes to renewable sources of electricity generation over fossil energy sources. Reducing the carbon footprint of grid electricity should reduce the carbon footprint of chicken meat. The extent to which the industry can rely on this to reduce emissions in unclear. A quantified and projected analysis is needed to model the emission intensity and total emissions of chicken meat.
Conclusions
Australian chicken meat is a low environmental-footprint food product. Modestly higher GHG emissions and land occupation were found to occur in FR systems (P < 0.05) than in C systems. This study found that the impact of direct land-use change from imported soymeal was a significant source of GHG emissions, which resulted in a small increase in the carbon footprint per kilogram of chicken meat compared with supply chains assessed 10 years prior. Although impacts can be reduced through substitution for alternative proteins or certified soybean meal, this will likely increase cost of production.
Due to the high exposure of chicken meat to impacts from grain production, this study showed that the impact of drought led to higher water consumption than in average years, pointing to higher levels of inter-annual variability in impacts than was previously appreciated. A similar short-term effect (resulting from improved land management) was observed in the moderate levels of carbon sequestration in Australian cropland which, when carried through to grains used in meat chicken feed, may amount to an approximately 2.5% reduction in the carbon footprint of chicken meat. Consequently, future analyses must acknowledge natural variability while tracking longer-term, management-induced trends in water consumption and stress impacts, and soil carbon associated with grain production. This favours the use of rolling averages (for example, over a 3-year period) rather than single analysis years.
Notwithstanding small improvements brought about by the decarbonisation of state energy grids, the lack of significant improvement over the past 10 years indicates that deliberate interventions via technology adoption and practice change are needed to reduce the emission intensity of chicken meat. In the face of expanding production, substantial reductions in emission intensity will be needed to maintain, rather than increase, total emissions. Quantified and projected analysis is needed to understand the level of emission reduction required, building on this starting point for the development of a robust, long-term sustainability framework to track and report the improvement in industry performance into the future.
Supplementary material
Supplementary material is available online.
Data availability
The disaggregated inventory data that support this study cannot be publicly shared because of confidentiality requirements for supply of commercially sensitive data.
Conflicts of interest
The authors declare no conflicts of interest. Mary-Frances Copley is currently a Master of Philosophy candidate at the University of New England, Armidale, NSW 2350, Australia.
Declaration of funding
This research was funded by AgriFutures Chicken Meat Program (Project No. 31RS005IA). Funding for AgriFutures Chicken Meat Program is primarily provided by the Australian chicken meat industry through a levy and by the Australian Government, which provides a matching contribution for R&D.
Acknowledgements
The authors gratefully acknowledge the support of the AgriFutures Chicken Meat Program and the Australian Chicken Meat Federation. The participating companies, growers and facility managers are thanked for supplying data.
References
ABS (2021a) Water use on Australian farms, 2019–2020 – catalogue number 4618.0. (Australian Bureau of Statistics (ABS): Canberra, ACT, Australia) Available at https://www.abs.gov.au/statistics/industry/agriculture/water-use-australian-farms/ABS (2021b) Agricultural Commodities, Australia, 2019–20 – Catalogue number 7121.0. (Australian Bureau of Statistics (ABS)). Available at https://www.abs.gov.au/statistics/industry/agriculture/agricultural-commodities-australia/2019-20
ACMF (2020) ‘Facts and figures.’ (ACMF: Australia) Available at https://www.chicken.org.au/facts-and-figures/
ALCAS (2017) AusLCI. Australian Life Cycle Assessment Society (ALCAS). (ALCAS: Australia) Available at http://auslci.com.au/
Arrieta EM, Cuchietti A, Cabrol D, González AD (2018) Greenhouse gas emissions and energy efficiencies for soybeans and maize cultivated in different agronomic zones: a case study of Argentina. Science of The Total Environment 625, 199–208.
| Greenhouse gas emissions and energy efficiencies for soybeans and maize cultivated in different agronomic zones: a case study of Argentina.Crossref | GoogleScholarGoogle Scholar |
Australian Bureau of Agricultural and Resource Economics and Sciences (2020) Australian crop report. Australian Bureau of Agricultural and Resource Economics and Sciences.
Bengtsson J, Seddon J (2013) Cradle to retailer or quick service restaurant gate life cycle assessment of chicken products in Australia. Journal of Cleaner Production 41, 291–300.
| Cradle to retailer or quick service restaurant gate life cycle assessment of chicken products in Australia.Crossref | GoogleScholarGoogle Scholar |
BOM (2019) Rainfall. Australian Government, Bureau of Meteorology, Australia. Available at http://www.bom.gov.au/climate/current/annual/aus/2019/#tabs=Rainfall
BOM (2020) Special Climate Statement 70 update: drought conditions in Australia and impact on water resources in the Murray–Darling Basin. (Australian Government, Bureau of Meteorology: Australia). Available at http://www.bom.gov.au/climate/current/statements/scs70.pdf
Boulay A-M, Bare J, Benini L, Berger M, Lathuillière MJ, Manzardo A, Margni M, Motoshita M, Núñez M, Pastor AV, Ridoutt B, Oki T, Worbe S, Pfister S (2018) The WULCA consensus characterization model for water scarcity footprints: assessing impacts of water consumption based on available water remaining (AWARE). The International Journal of Life Cycle Assessment 23, 368–378.
| The WULCA consensus characterization model for water scarcity footprints: assessing impacts of water consumption based on available water remaining (AWARE).Crossref | GoogleScholarGoogle Scholar |
Brock P, Madden P, Schwenke G, Herridge D (2012) Greenhouse gas emissions profile for 1 tonne of wheat produced in Central Zone (East) New South Wales: a life cycle assessment approach. Crop & Pasture Science 63, 319–329.
| Greenhouse gas emissions profile for 1 tonne of wheat produced in Central Zone (East) New South Wales: a life cycle assessment approach.Crossref | GoogleScholarGoogle Scholar |
Clune S, Crossin E, Verghese K (2017) Systematic review of greenhouse gas emissions for different fresh food categories. Journal of Cleaner Production 140, 766–783.
| Systematic review of greenhouse gas emissions for different fresh food categories.Crossref | GoogleScholarGoogle Scholar |
Cockerill SA, Gerber PF, Walkden-Brown SW, Dunlop MW (2020) Suitability of litter amendments for the Australian chicken meat industry. Animal Production Science 60, 1491–1481.
| Suitability of litter amendments for the Australian chicken meat industry.Crossref | GoogleScholarGoogle Scholar |
Commonwealth of Australia (2021a) Australia’s long-term emissions reduction plan. (Commonwealth of Australia)
Commonwealth of Australia (2021b) National greenhouse accounts factors: 2021. (Australian Government, Department of the Environment and Energy: Canberra, ACT, Australia)
Commonwealth of Australia (2021c) Livestock Products, Australia. Table 15 – Chicken meat produced: all series (tonnes) – catalogue number 7215.0. (Commonwealth of Australia) Available at https://www.abs.gov.au/statistics/industry/agriculture/livestock-products-australia/latest-release
Commonwealth of Australia (2021d) National inventory report 2019. Vol. 1. Australian Government, Department of Industry, Energy and Resources: Canberra, ACT, Australia.
Commonwealth of Australia (2021e) National inventory report 2019. Vol. 2. (Commonwealth of Australia: Canberra, ACT, Australia) Available at https://www.dcceew.gov.au/climate-change/publications/national-greenhouse-accounts-2019/national-inventory-report-2019
Craddock T, Hollitt J (2010) ‘Piloting chicken litter usage in broadacre cropping-Setting research directions.’ (Rural Industry Research and Development Corporation (RIRDC): Canberra, ACT, Australia)
Directorate-Generale for Environment (2021) ‘Recommendation on the use of environmental footprint methods.’ (European Commission). Available at https://ec.europa.eu/environment/publications/recommendation-use-environmental-footprint-methods_en
Dong H, Mangino J, McAllister T, Hatfield J, Johnson D, Lassey K, Aparecida de Lima M, Romanovskaya A, Bartram D, Gibb D, Martin J (2006) Emissions from Livestock and Manure Management. In ‘IPCC Guidelines for National Greenhouse Gas Inventories. Vol. 4: Agriculture, Forestry and other Land Use’. (Eds S Eggleston, L Buendia, K Miwa, T Ngara, K Tanabe) pp. 10.1–10.87. (Institute for Global Environmental Strategies: Kanagawa, Japan)
Doran-Browne NA, Ive J, Graham P, Eckard RJ (2016) Carbon-neutral wool farming in south-eastern Australia. Animal Production Science 56, 417–422.
| Carbon-neutral wool farming in south-eastern Australia.Crossref | GoogleScholarGoogle Scholar |
da Silva Lima ND, de Alencar Nääs I, Garcia RG, de Moura DJ (2019) Environmental impact of Brazilian broiler production process: evaluation using life cycle assessment. Journal of Cleaner Production 237, 117 752
| Environmental impact of Brazilian broiler production process: evaluation using life cycle assessment.Crossref | GoogleScholarGoogle Scholar |
Dunlop MW, McAuley J (2021) Direct surface wetting sprinkler system to reduce the use of evaporative cooling pads in meat chicken production: indoor thermal environment, water usage, litter moisture content, live market weights, and mortalities. Poultry Science 100, 101 078
| Direct surface wetting sprinkler system to reduce the use of evaporative cooling pads in meat chicken production: indoor thermal environment, water usage, litter moisture content, live market weights, and mortalities.Crossref | GoogleScholarGoogle Scholar |
Ecoinvent (2020) ‘ecoinvent 3.6 database.’ (Ecoinvent Centre). Available at https://www.ecoinvent.org/database/ecoinvent-36/ecoinvent-36.html
Gavrilova O, Leip A, Dong H, MacDonald J, Alfredo C, Bravo G, Amon B, Rosales R, Prado A, Lima M, Oyhantcabal W, Weerden T, Widiawati Y (2019) Emissions From Livestock and Manure Management. In ‘2019 Refinement to the IPCC Guidelines for National Greenhouse Gas Inventories’. (Eds E Calvo Buendia, K Tanabe, A Kranjc, J Baasansuren, M Fukuda, S Ngarize, A Osako, Y Pyroshenko, P Shermanau, S Federici).
Harris S, Narayanaswamy V (2009) A literature review of life cycle assessment in agriculture. Available at http://www.rirdc.gov.au
Ibidhi R, Hoekstra AY, Gerbens-Leenes PW, Chouchane H (2017) Water, land and carbon footprints of sheep and chicken meat produced in Tunisia under different farming systems. Ecological Indicators 77, 304–313.
| Water, land and carbon footprints of sheep and chicken meat produced in Tunisia under different farming systems.Crossref | GoogleScholarGoogle Scholar |
ISO (2014) Environmental management – water footprint – principles, requirements and guidelines. ISO 14046:2014. International Organisation for Standardisation (ISO), Geneva.
ISO 14067 (2018) ‘ISO 14067:2018 – Greenhouse gases – Carbon footprint of products – Requirements and guidelines for quantification.’ (International Organization for Standardization (ISO): Geneva, Switzerland)
Kalhor T, Rajabipour A, Akram A, Sharifi M (2016) Environmental impact assessment of chicken meat production using life cycle assessment. Information Processing in Agriculture 3, 262–271.
| Environmental impact assessment of chicken meat production using life cycle assessment.Crossref | GoogleScholarGoogle Scholar |
LEAP (2016) ‘Greenhouse gas emissions and fossil energy use from poultry supply chains: guidelines for assessment.’ (FAO: Rome, Italy) Available at http://www.fao.org/partnerships/leap/publications/en/
Leinonen I, Williams AG, Wiseman J, Guy J, Kyriazakis I (2012) Predicting the environmental impacts of chicken systems in the United Kingdom through a life cycle assessment: broiler production systems. Poultry Science 91, 8–25.
| Predicting the environmental impacts of chicken systems in the United Kingdom through a life cycle assessment: broiler production systems.Crossref | GoogleScholarGoogle Scholar |
Lesschen JP, van den Berg M, Westhoek HJ, Witzke HP, Oenema O (2011) Greenhouse gas emission profiles of European livestock sectors. Animal Feed Science and Technology 166–167, 16–28.
| Greenhouse gas emission profiles of European livestock sectors.Crossref | GoogleScholarGoogle Scholar |
Luo Z, Wang E, Sun OJ (2010) Soil carbon change and its responses to agricultural practices in Australian agro-ecosystems: a review and synthesis. Geoderma 155, 211–223.
| Soil carbon change and its responses to agricultural practices in Australian agro-ecosystems: a review and synthesis.Crossref | GoogleScholarGoogle Scholar |
McGahan E, Barker S, Poad G, Wiedemann S, Batstone D (2013) ‘Conversion of waste to energy in the chicken meat industry.’ (Rural Industries Research and Development Corporation (RIRDC): Australia)
McGahan E, Davis R, Poad G (2014) ‘Quantifying on-farm energy usage in the australian meat chicken industry.’ RIRDC Publication No. 14/124. (Rural Industries Research and Development Corporation (RIRDC): Australia).
Mckay JC, Barton NF, Koerhuis ANM, Mcadam J (2007) The challenge of genetic change in the broiler chicken. BSAP Occasional Publication 27, 1–7.
| The challenge of genetic change in the broiler chicken.Crossref | GoogleScholarGoogle Scholar |
Mekonnen MM, Hoekstra AY (2016) Four billion people facing severe water scarcity. Science Advances 2, e1500323
| Four billion people facing severe water scarcity.Crossref | GoogleScholarGoogle Scholar |
Nahm KH (2007) Feed formulations to reduce N excretion and ammonia emission from poultry manure. Bioresource Technology 98, 2282–2300.
| Feed formulations to reduce N excretion and ammonia emission from poultry manure.Crossref | GoogleScholarGoogle Scholar |
OEC (2019) Where does Australia import Soybean Meal from? Observatory of Economic Complexity (OEC). Available at https://oec.world/en/resources/about
Ogino A, Oishi K, Setoguchi A, Osada T (2021) Life cycle assessment of sustainable broiler production systems: effects of low-protein diet and litter incineration. Agriculture 11, 921
| Life cycle assessment of sustainable broiler production systems: effects of low-protein diet and litter incineration.Crossref | GoogleScholarGoogle Scholar |
Pelletier N (2008) Environmental performance in the US broiler poultry sector: Life cycle energy use and greenhouse gas, ozone depleting, acidifying and eutrophying emissions. Agricultural Systems 98, 67–73.
| Environmental performance in the US broiler poultry sector: Life cycle energy use and greenhouse gas, ozone depleting, acidifying and eutrophying emissions.Crossref | GoogleScholarGoogle Scholar |
Pfister S, Koehler A, Hellweg S (2009) Assessing the environmental impacts of freshwater consumption in LCA. Environmental Science & Technology 43, 4098–4104.
| Assessing the environmental impacts of freshwater consumption in LCA.Crossref | GoogleScholarGoogle Scholar |
Pré-Consultants (2021) ‘SimaPro 9.3 Software.’ (Pré-Consultants: Amersfoort, Netherlands)
Ramachandran Nair PK, Nair VD, Mohan Kumar B, Showalter JM (2010) Carbon sequestration in agroforestry systems. In ‘Advances in agronomy’. (Ed. DL Sparks) pp. 237–307. (Academic Press) https://doi.org/10.1016/S0065-2113(10)08005-3
Sevenster M, Luo Z, Eady S, Grant T (2020) Including long-term soil organic carbon changes in life cycle assessment of agricultural products. The International Journal of Life Cycle Assessment 25, 1231–1241.
| Including long-term soil organic carbon changes in life cycle assessment of agricultural products.Crossref | GoogleScholarGoogle Scholar |
Simmons AT, Murray A, Brock PM, Grant T, Cowie AL, Eady S, Sharma B (2019) Life cycle inventories for the Australian grains sector. Crop & Pasture Science 70, 575–584.
| Life cycle inventories for the Australian grains sector.Crossref | GoogleScholarGoogle Scholar |
Skunca D, Tomasevic I, Nastasijevic I, Tomovic V, Djekic I (2018) Life cycle assessment of the chicken meat chain. Journal of Cleaner Production 184, 440–450.
| Life cycle assessment of the chicken meat chain.Crossref | GoogleScholarGoogle Scholar |
Szögi AA, Vanotti MB, Hunt PG (2008) Phosphorus recovery from poultry litter. Transactions of the ASABE 51, 1727–1734.
| Phosphorus recovery from poultry litter.Crossref | GoogleScholarGoogle Scholar |
Warn L (2013) ‘Poultry litter: alternative fertiliser for pastures and way to increase soil organic carbon.’ (Rural Industries Research & Development (RIRDC))
WCED (1987) ‘Our common future.’ (World Commission on Environment and Development (WCED): Brussels, Belgium) https://doi.org/10.4324/9781912281220
Wiedemann S (2018) Analysis of resource use and greenhouse gas emissions from four australian meat production systems, with investigation of mitigation opportunities and trade-offs. Doctoral thesis, Charles Sturt University, Australia. Available at https://researchoutput.csu.edu.au/ws/portalfiles/portal/75667383/Stephen_ Wiedemann_Thesis.pdf
Wiedemann S, Yan MJ (2014) Livestock meat processing: inventory data and methods for handling co-production for major livestock species and meat products. In ‘Proceeedings of the 9th International Conference on Life Cycle Assessment in the Agri-Food Sector (LCA Food 2014), San Francisco, California, USA, 8–10 October, 2014’. pp. 1512–1520. American Centre for Life Cycle Assessment.
Wiedemann S, McGahan E, Poad G (2012) Using life cycle assessment to quantify the environmental impact of chicken meat production. RIRDC Publication No. 12/029. (Rurual Industries Research and Development Corporation (RIRDC), Australia). Available at https://www.agrifutures.com.au/wp-content/uploads/publications/12-029.pdf
Wiedemann S, McGahan E, Murphy C, Yan M-J (2015a) Resource use and environmental impacts from beef production in eastern Australia investigated using life cycle assessment. Animal Production Science 56, 882–894.
| Resource use and environmental impacts from beef production in eastern Australia investigated using life cycle assessment.Crossref | GoogleScholarGoogle Scholar |
Wiedemann SG, Bielefeld EN, McGahan EJ, Valentine JG, Murphy CM (2015b) ‘Grower options for spent litter utilisation.’ (Rural Industries Research & Development (RIRDC))
Wiedemann SG, Phillips FA, Naylor TA, McGahan EJ, Keane OB, Warren BR, Murphy CM (2016a) Nitrous oxide, ammonia and methane from Australian meat chicken houses measured under commercial operating conditions and with mitigation strategies applied. Animal Production Science 56, 1404–1417.
| Nitrous oxide, ammonia and methane from Australian meat chicken houses measured under commercial operating conditions and with mitigation strategies applied.Crossref | GoogleScholarGoogle Scholar |
Wiedemann SG, Yan M-J, Murphy CM (2016b) Resource use and environmental impacts from Australian export lamb production: a life cycle assessment. Animal Production Science 56, 1070–1080.
| Resource use and environmental impacts from Australian export lamb production: a life cycle assessment.Crossref | GoogleScholarGoogle Scholar |
Wiedemann SG, McGahan EJ, Murphy CM (2017) Resource use and environmental impacts from Australian chicken meat production. Journal of Cleaner Production 140, 675–684.
| Resource use and environmental impacts from Australian chicken meat production.Crossref | GoogleScholarGoogle Scholar |
Williams A, Audsley E, Sandars D, Jones R, Whitmore A, Glendining M, Dailey G, Williams A, Audsley E, Sandars D (2006) Determining the Environmental Burdens and Resource Use in the Production of Agricultural and Horticultural Commodities: Defra Project Report No: IS0205. Department of the Environment, Food and Rural Affairs (DEFRA), UK. Available at http://randd.defra.gov.uk/Default.aspx
Willis S (2003) The use of Soybean meal and full fat Soybean meal by the animal feed industry. In ‘12th Australian Soybean Conference’. (Department of Primary Industries: Qld, Australia) Available at http://australianoilseeds.com/__data/assets/file/0019/1198/Sarah_Willis-The_Use_of_Soybean_Meal_and_Full_Fat_Soybean_Meal_by_the_Animal_Feed_Industry.pdf
Wiltshire J, Tucker G, Williams A, Foster C, Wynn S, Thorn R, Chadwick D (2009) Supplementary Technical Report to ‘Scenario building to test and inform the development of a BSI method for assessing GHG emissions from food’. Final report to Defra on research project FO0404, London, UK.
Zeltner E, Maurer V (2009) Welfare of organic poultry. In ‘Poultry Welfare Symposium’, 18–22 May 2009, Cervia, Italy. pp. 104–112.