Effect of bedding application and air change rates on environmental ammonia concentrations for intensively housed beef cattle
I. N. Hanafi


A
B
Abstract
Manure deposition during livestock export voyages contributes to air ammonia levels, potentially affecting human and animal health if not managed. Mitigation strategies may include increased air change rates and application of bedding.
This study examined the effect of bedding application rate (BAR) and air change rate (ACH) on air ammonia (NH3) concentrations and pad properties, including pad surface condition, pH, moisture, and pad ammonium (NH4+) concentrations, for intensively housed beef cattle.
Six 7-day runs were conducted with 72 Bos indicus cross steers (mean liveweight ± s.d. = 338 ± 32 kg) housed in respiration chambers by using a 3 × 3 factorial design. The BARs were set to 0%, 50%, and 100% of the Australian Standards for the Export of Livestock (ASEL), and ACH were varied at 20, 35, and 52. Air NH3 was measured twice daily at three heights. Pad surface condition was collected with the first air NH3 measurement. Video footage captured standing and lying behaviours for each steer. Pad samples were collected on the final day for pad chemical analysis.
The ACH of 20 changes per hour resulted in higher air NH3 concentration than ACH of 35 and 52. Higher BAR led to lower pad pH and moisture, with slightly lower pad NH4+ concentration in 100% and 50% BAR than 0% BAR. Although air NH3 concentration on Day 7 was positively correlated with pad NH4+ concentration, BAR had no marked effect on air NH3 concentration (within the temperature range of this experiment). Drier and firmer pad surfaces were associated with each high BAR and high ACH. Moreover, high BAR increased the frequency of lying behaviour in steers.
These findings indicated that NH3 can be mitigated by optimising air changes to minimise air NH3 concentration and utilising bedding to minimise pad NH4+. This offers practical solutions for intensively housed beef cattle, such as livestock export voyages to improve human and animal welfare onboard.
The study results emphasised the importance of optimising ACH to maintain low air NH3 concentrations in livestock export conditions. Although there was no evidence that BAR affects air NH3 directly, it reduced pad NH4+ and improved pad conditions for overall animal comfort and environmental quality in confined housing with sufficient air changes.
Keywords: air change, ammonia, bedding, livestock export, pad condition, steers, ventilation.
Introduction
Ammonia (NH3) is an irritant gas that can be produced from manure during livestock operations, including livestock transport. Human and animal health can be compromised when exposed to high air NH3 concentrations on a regular basis. The critical value of atmospheric NH3 when transporting cattle, sheep and goats has been set as the human level of time-weighted average exposure tolerance of 25 ppm (17.4 mg/m3) (Costa et al. 2003). However, on the basis of immune responses (as indicated by white blood cell counts and mononuclear cell counts), it has been suggested that the maximum safe level of NH3 exposure for cattle in live export vessels is 20 ppm (13.9 mg/m3) (Tudor et al. 2003; McCarthy and Banhazi 2016). In addition, a study on the physiology and behaviour of sheep showed that sheep exposed to 20.9 mg/m3 (range 17.2–23.7 mg/m3) NH3 displayed discomfort when chewing because of buccal cavity irritation and increased breathing rates (Zhang et al. 2017). A study was conducted in 2007 to simulate a 12-day ship voyage to assess cattle physiological and behavioural response to air NH3 concentration. It showed that 45 ppm (31.3 mg/m3) NH3 increased macrophage activity, lacrimation, and nasal secretion (Phillips et al. 2010).
Ammonia volatilises from manure pads (accumulated animal manure) as a result of nitrogen (N) loss as urinary-N, which contains 69% urea (Bristow et al. 1992). Urinary urea-N is converted to NH3 by microbial decomposition of organic N compounds and urease enzymes in the faeces (Dai and Karring 2014; Liu et al. 2017). In confined cattle housing such as in livestock export ships, NH3 volatilisation is multifactorial and can be influenced by humidity and air temperatures levels (Ngwabie et al. 2011; Pines and Phillips 2011) and also can be affected by bedding (Banney et al. 2009), pad pH, stocking density, and air turnovers (i.e. air change rate per hour or ACH) (Costa et al. 2003).
Bedding on livestock export vessels is mainly utilised as an absorption agent for animal faeces and urine (McCarthy and Banhazi 2016). Bedding may also improve pad condition by reducing the penetrable depth and moisture of the pad (Wilkes et al. 2022) and support animal comfort as measured by lying time and number of lying bouts throughout the day (Tucker et al. 2009). Current Australian Standards for the Export of Livestock ver. 3.3 (ASEL 3.3) (Department of Agriculture, Water and the Environment 2023) require that on long-haul voyages (>10 days), bedding, such as sawdust, rice hulls or similar materials be applied at a rate of 7 tonnes (7000 kg) for every 1000 m2 of cattle pen space. The Australian livestock export industry commonly utilises wood shavings and sawdust (McCarthy and Banhazi 2016) that contain lower moisture of ~30% (Banney et al. 2009) and lower odour activity value than do other materials such as corn cobs, shredded paper, or wood chips (O’Keefe et al. 2013). Even though the bedding application standard does not apply to cattle voyages leaving ports from Brisbane or north of latitude 26°S to Southeast Asia, some exporters carrying heavy cattle (over 380 kg) on short-haul voyages (<10 days) use bedding to minimise lameness incidence (Banney et al. 2009). Moreover, the use of bedding potentially mitigates the humidity issue and minimises risks of slips after washing out (MAMIC 2001) as Australian Maritime Safety Authority (2022) states ‘AMSA recommends that deck washing is conducted at intervals not more than 4 days when carrying cattle and/or buffalo.’
Air ventilation systems can affect the various gas concentrations within the environment (such as NH3) by controlling air changes in animal housing (Ye et al. 2009). Applying higher ventilation rates by increasing air changes per hour to lower the NH3 volatilisation (Kim and Kim 2020) may reduce the risk of disease caused by high NH3 concentrations during sea transport (MAMIC 2001; McCarthy 2005). On livestock export vessels, the ratio of air supply to each specific pen volume is expressed as air changes per hour (ACH), which is the ventilation term used in Australian Maritime Safety Authority (AMSA) Marine Order Part 43 (MO43) regulations. According to AMSA, 20 ACH is required for decks with a ceiling height of 2.3 m or more and 30 ACH for decks with a ceiling height of 1.8 m.
In the current version of ASEL (3.3), recommendations for bedding application rates (BAR) still need a strong empirical study basis and do not include any contingency for how the bedding rate may affect the amount of NH3 volatilised and how ventilation rates might interact in this process under specific BAR. This study aimed to assess the effects of (and interactions between) BAR and ACH on the concentration of air NH3 and pad NH4+ in simulated livestock voyages, with a view to inform refinement of ASEL 3.3 requirements. The study also monitored pad surface condition and investigated pad properties (pH, moisture, and bulk density) and associations between these properties and the concentration of air NH3. Moreover, cattle standing and lying preferences by bedding and air change-rate treatments were also assessed.
Materials and methods
The experiment was undertaken at the Centre for Animal Research and Teaching, UNE, Armidale, NSW, Australia (30°28′57.8″S, 151°38′16.2″E). The conduct of the experiment was approved by the University of New England (UNE) Animal Ethics Committee under the Australian Code for the Care and Use of Animals for Scientific Purposes, 2013 (AEC Approval # 20-058).
Experimental design
The 79-day experiment was a 3 × 3 factorial complete randomised block design with each of the nine combinations of three BAR and three ACH treatments assessed in each of six experimental periods (Runs 1–6). For all runs, the cattle had 14 days of adaptation to feed and facilities, followed by 7 days housed as pairs in large animal-respiration chambers. Nine chambers and 18 steers were to be used in each run, with two steers being housed in each chamber for 7 days. However, in experimental Runs 2 and 6, 10 chambers were required for replication because of the early removal of steer pairs from chambers in previous runs. The BAR treatments and steer pairs were randomly allocated to the chambers within each run.
Animals and animal management
Eighty Bos indicus (Brahman)-cross steers aged 24 months with a mean liveweight (±s.d.) of 338 (±32) kg were transported, in two separate cohorts, 4 h from the property of origin (Department of Primary Industries, Grafton, NSW, Australia) to the Large Animal Facility at the Centre for Animal Research and Teaching of the University of New England (Armidale, NSW Australia). After arrival, the first cohort (Group A and B steers) were housed in a large outdoor pen and transitioned to a shipper pellet ration for a 7-day adaptation period after arriving at the facility. Prior to each run, the steers were randomly allocated into pairs, resulting in nine pairs per group (four groups in total). Each pair in each group underwent a pair adaptation period for 7 days, housed in a 4 m × 2 m pen inside the Large Animal Facility. The second-cohort steers (Groups C and D) arrived on Day 48 and were assigned to the same adaptation and experimental protocols as were Groups A and B. At the start of the feed adaptation period, all steers (Groups A, B, C and D) were inducted to the facility, vaccinated with Webster 7 in 1 Vaccine for Cattle (Virbac Australia, Milperra, NSW, Australia) and treated with Cydectin Pour-On (Virbac Australia). From each cohort of 40 steers, 36 were chosen for the experiment on the basis of temperament. The remaining four spare steers were housed in outdoor pens until they returned to their property of origin.
After each adaptation period, the steer pairs were housed for a 7-day experimental period inside large animal-respiration chambers. The steers were individually assigned numbers, which were sprayed on their backs by using a non-toxic and non-permanent aerosol paint livestock spray (Steadfast Stockmark; Dy-Mark, Darra, Queensland, Australia) for ease of individual identification on the camera footage. The estimated space-allowance area per head in the chamber for an average 338 kg of liveweight was calculated using a k-value of 0.027, giving a 1.26 m2 area per steer (Petherick and Phillips 2009) that met the pen space requirement of ASEL 3.3 (Department of Agriculture, Water and the Environment 2023).
The steers were checked for general health twice daily (AM and PM), standing and lying posture, including demeanour, respiration rates, coat condition, flank (bloat or sunken), eye (weeping mucosa or inflammation), and muzzle (excessively dry or with nasal discharge) conditions. However, for the purpose of this study, only the frequency of standing and lying was analysed.
Seven pairs of the Group A steers used in Run 1 were re-used in Runs 3 and one pair from Group B used in Run 2 was re-used in Run 3. All pairs of the Group B steers used in Run 2 were re-used in Run 4, with the remaining steers in Run 3 and 4 being used for the first time.
Feed provision
During the adaptation period, the steers were introduced to a commercial shipper pellet (9.5 MJ of metabolisable energy (ME) and 12.1% drude protein (CP) per kg of DM; Macco Feeds, Willams, Western Australia; Table 1). The steers were fed with a mix of 50% oaten chaff and 50% pellets for the first 3 days of adaptation. The amount of chaff was reduced to 30% for Days 4–6, and from Day 7 of adaptation onward and during the experimental period, the steers were fed with pellets exclusively. A feed trough (1.04 m × 0.49 m × 0.52 m) was located at the front of the chambers adjacent to water trough. The feed allowance for the entirety of the trial was calculated at 2% of liveweight to follow the ASEL minimum requirement for ‘other classes’ of cattle, and offered in two meals per day.
Chemical property | Composition | |
---|---|---|
Moisture | <12 | |
Ash (% DM) | <13 | |
Crude protein (% DM) | 12.1 | |
Urea (% DM) | <1.2 | |
Acid detergent fibre (% DM) | 18 < 35 | |
Metabolisable energy (MJ/kg DM) | 9.5 |
DM, dry matter.
Fresh water was provided ad libitum by using an automatic nose bowl trough (0.28 m × 0.26 m), located 0.75 m above the floor and secured to the rail at the front of each chamber.
Respiration chambers
Ten chambers were arranged in two rows of five, each with a single full-width entry and exit door opening onto a standard aisle between the two rows. The chambers were sealed in a recessed water-filled trench and could be raised by a pneumatic system to permit hosing out of animal waste at the end of each experiment. The outside chamber dimension was 3.6 m × 2.4 m × 2.4 m (chamber volume of 20.736 m3), and within each chamber, cattle were housed in a smaller internal steel pen structure (3.0 m × 1.8 m × 1.8 m) (Fig. 1).
Respiration chamber layout from the upper view, front view and side view and NH3 measuring height spots (top-2.3 m from the pad (A), middle-1.8 m from the pad (B), bottom-0.3 m from the pad (C)).
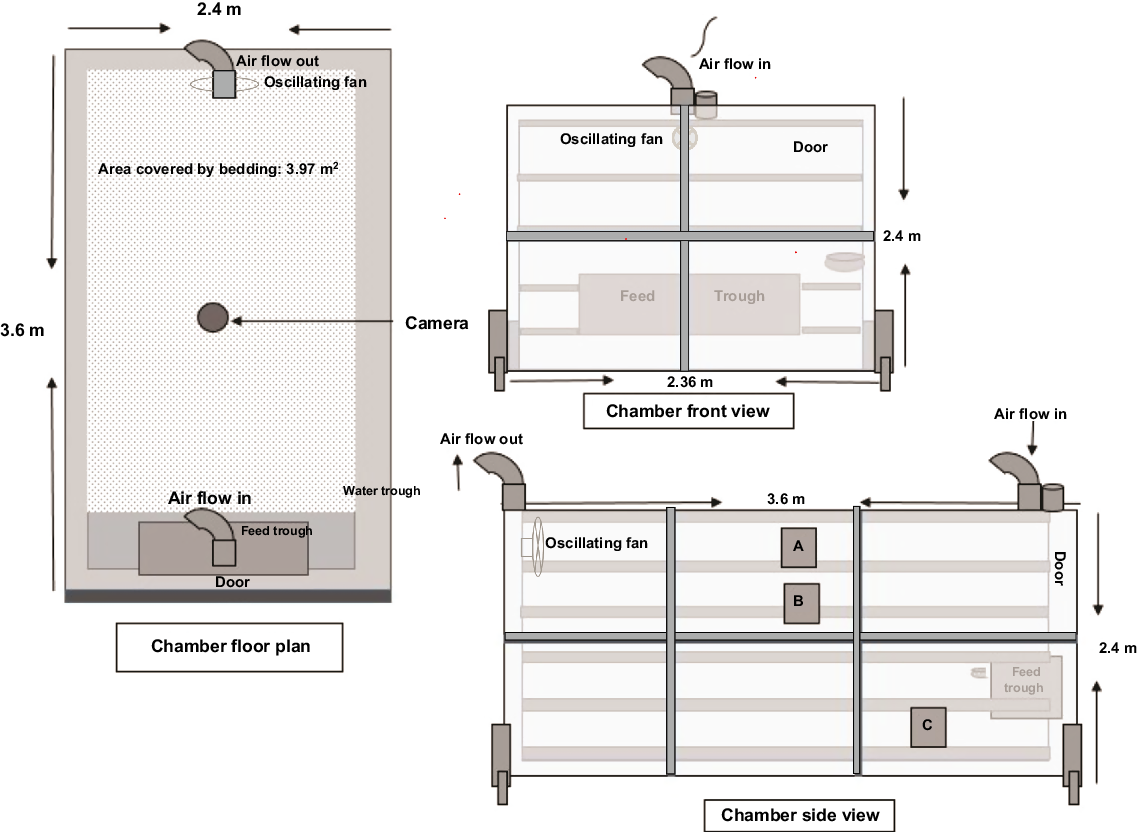
Dry-bulb temperature (TDB) and relative humidity (RH) were recorded twice daily at 07:30 hours (AM) and 14:30 hours (PM) by using an equipped SHT20 I2C temperature and humidity sensor (Core Electronic, Adamstown, NSW, Australia) located at steer head height within each chamber. In total, there were 702 observations of TDB and RH during the trial and the means for TDB and RH are shown in Table 2. The mean values of TDB for PM were 7.1°C higher than those for AM. Conversely, the mean RH for AM was greater than the mean for PM. Wet-bulb temperature (TWB) was calculated from AM and PM TDB and RH (Table 2). The mean AM and PM TDB and RH were equivalent, with the mean of temperature–humidity index (THI) of 62.6 and 71.3 respectively (Table 2).
Variable and time point (number of observations) | Mean ± s.d. | Minimum | Maximum | |
---|---|---|---|---|
TDB (°C) | ||||
AM (n = 378) | 17.4 ± 2.6 | 11.2 | 23.4 | |
PM (n = 324) | 24.5 ± 2.7 | 18.2 | 30.5 | |
TWB (°C) | ||||
AM (n = 378) | 9.7 ± 1.13 | 7.05 | 12.3 | |
PM (n = 324) | 12.8 ± 1.16 | 10.1 | 15.3 | |
THI | ||||
AM (n = 378) | 62.6 ± 4.04 | 53.1 | 72.2 | |
PM (n = 324) | 71.3 ± 3.36 | 62.8 | 78.8 | |
RH (%) | ||||
AM (n = 378) | 73.9 ± 4.2 | 56.1 | 84.7 | |
PM (n = 324) | 53.0 ± 8.6 | 34.0 | 77.1 |
TDB, dry-bulb temperature; TWB, wet-bulb temperature; THI, Temperature–humidity index; RH, relative humidity.
The air was delivered into the chambers by 150-mm-diameter industrial tubing with a rotating baffle that could be closed or opened to increase or decrease the air supplied to the desired air change rate (per hour, ACH). An oscillating fan (diameter of 40 cm) located within the chamber, 2 m above the floor (Fig. 1), continuously mixed the internal air of the chamber. This was to ensure that the air was well-mixed prior, so the air exiting the chamber was representative of that throughout the chamber.
Video footage was recorded using fixed infra-red cameras (MR6822E2 and LR832, Lilin Australia, Lidcombe, NSW, Australia) positioned centrally above each chamber pen for the entire duration of each 7-day experimental period to monitor animal condition and behaviour, particularly standing and lying. The standing and lying frequency data were collected using an instantaneous-scan sampling method every 15 min for Days 1, 3 and 6. Time (h) to when each steer first lay down were measured from 20 min after the steers entered the chamber and the chamber door was closed (Time 0).
Bedding application-rate (BAR) and air change-rate (ACH) treatments
The study methodology was designed in consultation with industry to closely reflect commercial practices, including the average duration between washouts, as well as the type and the amount of bedding used (livecorp.com.au/publication/3OvSabqQOxmKP9KHJUd7ox). Thus, the experimental period is relevant to both short-haul voyages that require bedding for different classes of cattle and long-haul voyages that require the use of bedding, and reflects the average interval between washouts observed in industry. The different bedding levels tested in this study (0%, 50%, and 100% of ASEL) were used to assess how different bedding densities might influence the environment in which the cattle are housed by absorbing excess moisture, for example, after regular washing and thus improving air quality and pad surface condition.
All nine BAR and ACH treatment combinations were allocated across nine chambers within each experimental period. Kiln-dried pine wood shavings were used as the bedding material for this study (DM 91.29%, N content 0.03% DM, pH 8). The BAR treatments were applied at 0, 13.6 and 27.2 kg (equivalent to 0%, 50%, and 100% of the ASEL standard respectively) and spread evenly to cover the 3.97 m2 floor area (not including space required for feed and water trough).
The chamber area in this study was 8.64 m2 and the volume was 20.736 m3. The lowest ACH treatment of 20 ACH was set to meet the minimum Australian Maritime Safety Authority – Marine Order 43 (cargo and cargo handling – livestock) (AMSA MO43) regulation for a 2.4 m chamber-ceiling height. To meet 20 ACH, the volume of air exchange (VAE) was set to 420 m3/h. The 720 m3/h and 1080 m3/h VAE were set to meet 35 ACH (medium) and 52 ACH (high) respectively. Average pen air turnover (PAT), if incoming air enters over the entire area of the chamber for each ACH, was calculated using the corresponding VAE (VAE × 8.64 m2) (Table 3).
Treatment | Air changes per hour-(AMSA MO43 A standard) | Volume of air exchange (m3/h) | Pen air turnover (m/h) | |
---|---|---|---|---|
Treatment 1: low ACH | 20 | 420 | 49 | |
Treatment 2: medium ACH | 35 | 720 | 83 | |
Treatment 3: high ACH | 52 | 1080 | 125 |
Air NH3 measurement and estimated NH3 production
Air NH3 was measured twice daily, in the morning (AM; 0730 hours to 0900 hours) and the afternoon (PM; 1430 hours to 1600 hours) at three different heights (top, middle and bottom; Fig. 1) by using a ToxiRAE Pro gas monitor (PGM-1860; Honeywell International Inc., Charlotte, North Carolina, USA). The sensor’s operating-temperature range was between −20°C and 55°C, and the reading range for NH3 was from 0 to 100 ppm with 0.1 ppm increment. The real-time sensor reading of gaseous concentrations was in parts per million (ppm); these NH3 data were then converted into mg/m3 by using the following formula:
For the top and middle measurements, the sensor was lowered into the chambers by using a hand-held pole (2 m) through the air inlet duct on top of the chamber at two different heights from the pad [2.3 m (top) and 1.8 m (middle) from the floor]. Middle height was approximately standing animal and human head heights. For the bottom measurement, each chamber door was opened slightly, and the sensor was immediately inserted 1.5 m in from the door and held 0.3 m above the pad. The duration for every measure at each height was 2 min. The sensor was reset to 0 ppm before each measurement to prevent sensor drift. The total NH3 produced for each treatment could be approximated using the following formula:
Pad properties
Pad-surface conditions were recorded every morning (AM) at the same time as air NH3 measurement by using four variables. Each of these variables was an ordinal score (three variables) or a nominal categorical variable, where scores or categories were allocated on the basis of visual assessment. This scoring system for pad-surface conditions was developed to assess cattle pads (Supplementary material 1: Daily pad surface conditions scores), because the existing scale was for sheep pads, and these are substantially different in moisture content and other physical and visual characteristics (McCarthy and Banhazi 2016). The data were collected and recorded in the KoBo Collect application (www.kobotoolbox.org) by using scores for each category. Data were collected for four variables: moisture appearance (score 1–8; from dry to flooded respectively), area covered by moisture [score 1–3: no wet areas (0%), small wet patches (<10%), and large wet patches (10% or more)], the assumed moisture source (high humidity, water-trough spill, water-line leak, cattle playing with water, or urine), when moisture was detected in the pad (moisture-area score of >1) and estimated pad depth [score 1–6; from no pad (cm) to >20 cm depth respectively].
Pad sampling was performed on the final day of each experimental period (Day 7), because it was not feasible to conduct it before the steers were discharged from the chambers because of safety reasons, to minimise the disturbance for the steers that can lead to animal stress and to maximise the mass balance of total pad collection. Pad depth on the chamber floor was measured at 25 locations as shown in Fig. 2a. The pad was then considered as being divided into Quarters 1, 2, 3 and 4 (Fig. 2b). Within each quarter, the pad was homogenised, and five grab samples were collected to represent each quarter (2 kg per quarter in total). The pad in each quarter, including the grab samples, was weighed individually and summed to obtain total pad mass (kg) for each chamber. The grab samples from each quarter were split into subsamples and used to measure pH (pH-ORP-Temp. Meter, pH Cube, TPS Pty Ltd, Brendale, Qld, Australia) and then the remainder (250 g) was oven-dried at 105°C for 24 h or until the weight was stable for gravimetric moisture content (AS 1289.2.1.1 method) (Standards Australia 2005). Bulk density was measured using a modified compaction test (AS 1289.5.2.1 method) (Standards Australia 2017) on subsamples stored at 4°C.
Pen set up on Day 7 for 25 spot samples assigned as x, y coordinates (cm) for (a) measuring pad depth and (b) pad sampling location divided into four quarters.
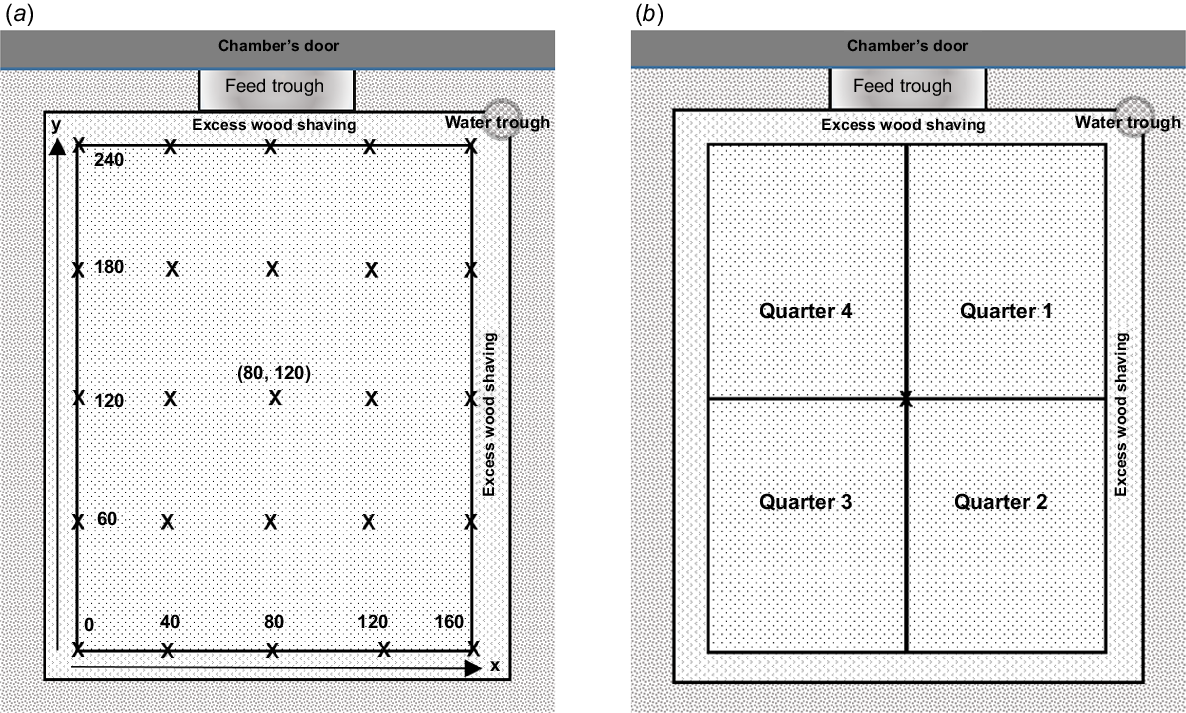
The frozen (−20°C) sample was analysed for pad ammoniacal N (NH3 (aq) and NH4+), which in this study is reported as NH4+ concentration via colorimetric determination through a modified Berthelot reaction (Creevey 2022). Each 1 g subsample was placed in a 50 mL polypropylene centrifuge tube and mixed with 20 mL of 2 M KCl. The mixture was tumbled for 4 h at 15 rpm, refrigerated overnight at 4°C, and then centrifuged at 3000g for 20 min (temperature 20°C) (Allegra X-15R centrifuge, Beckman Coulter USA). The supernatant was transferred to new tubes and stored at −20°C. For the assay, samples were diluted 1:10 with 2 M KCl, added to a 96-well plate in duplicate, and mixed with a working reagent. The absorbance at 650 nm was read after 30 min of incubation at room temperature by using a SPECTROstar Nano plate reader (BMG Labtech, Ortenberg, Germany), and the results were adjusted for blanks before performing a linear regression to determine NH4+ concentrations. The total pad NH4+ mass (g/chamber) was then calculated by multiplying the NH4+ concentration (mg/g pad) by the total pad mass (kg) in the chamber.
A small portion of the pad was outside of the floor surface area accessible by the steers (Fig. 2). This pad portion was also collected for analyses of pad mass and pad NH4+ concentration. This outside portion of the pad was not included in the samples for each chamber quarter.
Statistical analyses
Statistical analyses were performed in Stata (StataCorp, Releases 16 and 18. College Station, TX, USA).
Descriptive results are reported as means ± s.d. Associations with air NH3 concentration over all 7 days were assessed using multilevel mixed-effect linear regression models. Experimental period-chamber identification number combination (n = 54 levels; six runs of nine chambers per run) was fitted as a random effect, with a first-order autoregressive correlation structure for correlations in residuals between time points (Days 1–6 at AM and PM and Day 7 at AM only) within experimental period-chamber identification number combination. Likelihood-ratio test P-values for interaction terms relative to models with only main effects are reported. Predicted means ± s.e.m. from models are reported for each level of the fitted variables.
Air NH3 concentrations were compared by bedding rate (BAR), ACH, measurement height (bottom, middle or top), time of day (AM or PM), and day number, with each variously fitted as fixed effects (all as categorical variables). Estimated differences and associated 95% confidence intervals are reported in Supplementary material Table S1. Interactions between measurement height and day number were assessed by fitting BAR, ACH, measurement height and time of day (AM or PM), along with interaction between day and either measurement height or time of day. Main effects of BAR were assessed with no other fixed effects fitted, as were main effect of ACH.
For each of TDB and RH, associations with air NH3 concentration were assessed adjusted for the other by simultaneously fitting both as continuous variables. Linearity of associations was first assessed by fitting the best 1- and 2-dimension fractional polynomial terms for each of TDB and RH in multivariable multilevel mixed models, with TDB, RH, BAR, ACH, day number and time of day (AM/PM) being fitted simultaneously.
Pad surface-condition variables (moisture appearance, area covered by moisture, and pad-depth score) scored in the morning (AM) of each day over the 7-day experimental periods were each compared by BAR, ACH and day number by using ordered logistic models with all three explanatory variables simultaneously fitted as categorical data, and with standard errors adjusted for clustering of day within experimental period-chamber identification number combination. Pad moisture appearance was refined to four ordinal categories (scores 1 or 2 or 3 combined, 4, 5 or 6 combined, 7 or higher) before analyses. When analysing the ordinal variables describing pad-surface conditions, the data were too sparse to assess interactions between BAR and ACH.
Effects of BAR and ACH on each of five pad properties on Day 7 (pad pH, moisture content, bulk density, NH4+ concentration and total pad NH4+ mass) were assessed with multilevel mixed models with both BAR and ACH (but no other variables) simultaneously fitted as fixed effects.
Associations between each pad property on Day 7 and air NH3 concentration at the AM measurement on Day 7 were assessed in separate univariable multilevel mixed-effects linear regression models with the pad property variable fitted as continuous data as a fixed effect, and with experimental period-chamber identification number combination fitted as a random effect. Pearson’s correlation coefficient and associated 95% confidence intervals and P-values for correlations between pad property variables were also calculated.
Pad depth on Day 7 was modelled using multilevel mixed models with BAR fitted as a fixed effect as a categorical variable, x coordinate (pad depth measured from the left side to right side of the chamber floor) fitted as a continuous variable, and experimental period-chamber identification number combination fitted as a random effect. The curve for the x-coordinate–pad depth relationship was selected as the best fitting 2-dimension fractional polynomials of the x coordinate. Fitted values were plotted pooled across BARs. The same process was repeated for y-coordinates (pad depth measured from the back side to the front side of the chamber floor).
The concentration of NH3 in the pad on Day 7 was compared between samples from outside the quarters area to inside by using a multilevel mixed model with chamber fitted as a random effect. Pad pH, moisture, bulk density and NH4+ concentration on Day 7 were compared between bedding treatments in the same way.
Time taken for each steer to lie for the first time from 20 min after entering the chamber and the chamber door was closed (Time 0) were compared by BAR, by using a multilevel mixed model with chamber fitted as a random effect. The binary variable, lying or standing, was recorded for each steer every 15 min on 3 days (Day 1 from 0730 hours, Days 3 and 6 for all time points). This variable was analysed separately on each day by using generalised estimating equations with binary distribution and logit link and run-steer combination set as the panel variable, with a first-order autoregressive correlation structure for correlations in residuals within run-steer combination between time points. BAR, ACH, and daytime/night-time (where daytime was from 0700 hours to 1800 hours inclusive) were simultaneously fitted as fixed effects, as categorical variables.
Results
Air NH3 concentrations by day and measuring height
Over 2106 observations [six experimental periods × (7 days AM + 6 days PM) × nine chambers × three heights], NH3 concentration (mean ± s.d.) was 3.75 ± 1.66 mg/m3. Air NH3 concentration increased from Day 1 to Day 3, then reached a plateau, with a minimal interaction between day number and measuring height (Fig. 3a). Concentrations were higher in AM than in the PM, with differences of similar magnitude on all days except Day 4 (Fig. 3b). Crude mean air NH3 concentration at the middle height was higher than the means at bottom and top heights, with the latter two being similar (Table 4).
Predicted means for air NH3 concentration by study day number (a) at three measuring heights (top, middle and bottom, 0.3, 1.8, 2.3 m above the pad, respectively) and (b) in the morning (AM) and in the afternoon (PM), with 95% confidence intervals for each predicted mean; bedding application rate (BAR), air changes per hour (ACH), measurement height and time of day (AM or PM) were included as fixed effects, as categorical variables, in both models, along with interaction between day number and measurement height (a) or time of day (b); overall P-values for interaction terms were 0.447 and <0.001 respectively.
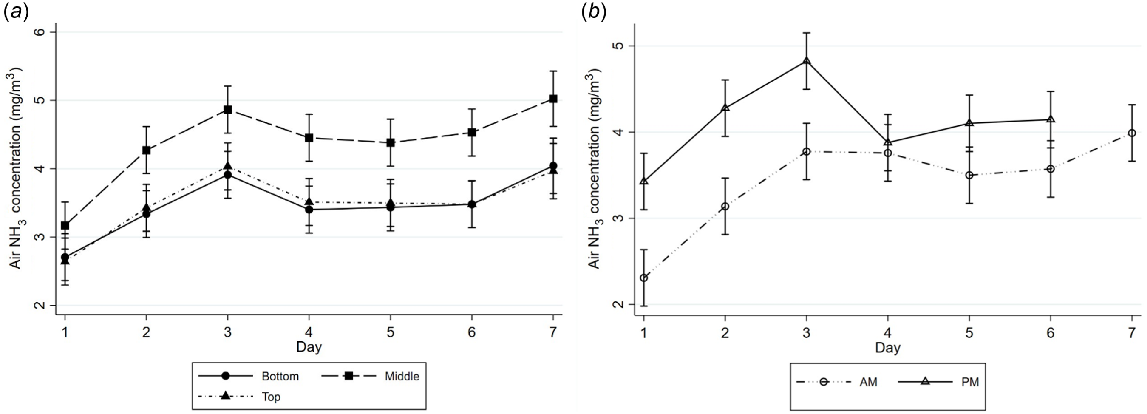
Air NH3 measuring height | Air NH3 concentration (mg/m3) | |||
---|---|---|---|---|
Mean ± s.d. | Minimum | Maximum | ||
Top (2.3 m above the pad) | 3.47 ± 1.444 | 0.70 | 9.76 | |
Middle (1.8 m above the pad) | 4.34 ± 1.888 | 0.70 | 12.54 | |
Bottom (0.3 m above the pad) | 3.43 ± 1.459 | 0.70 | 12.54 |
Determinants of air NH3 concentration
P-values for interaction between BAR and ACH on air NH3 concentration at top, middle, and bottom heights were high (Table 5), but estimates for interaction terms were imprecise (results not shown).
Air NH3 concentration (mg/m3) at three measuring heights | BAR (% ASEL) | ACH (number per hour) | Overall P-value | |||||||
---|---|---|---|---|---|---|---|---|---|---|
0 | 50 | 100 | 20 (low) | 35 (medium) | 52 (high) | Bedding rate (B) | ACH (A) | B × A interaction | ||
Top (2.3 m above the pad) | 3.53 ± 0.18 | 3.34 ± 0.18 | 3.49 ± 0.18 | 4.05 ± 0.18a | 3.21 ± 0.18b | 3.09 ± 0.18b | 0.746 | <0.001 | 0.640 | |
Middle (1.8 m above the pad) | 4.28 ± 0.24 | 4.19 ± 0.24 | 4.38 ± 0.24 | 5.41 ± 0.24a | 3.79 ± 0.24b | 3.66 ± 0.24b | 0.858 | <0.001 | 0.500 | |
Bottom (0.3 m above the pad) | 3.52 ± 0.18 | 3.30 ± 0.18 | 3.42 ± 0.18 | 4.14 ± 0.18a | 3.12 ± 0.18b | 2.99 ± 0.18b | 0.682 | <0.001 | 0.509 |
Means within a row and within a category followed by the same letter or no letter do not differ statistically significantly (at P < 0.05).
Assuming no interaction between BAR and ACH, within measurement heights, predicted that mean air NH3 concentrations (mg/m3) were similar across all BARs (Tables 5, S1). In contrast, within each measurement height, air NH3 concentration was affected by ACH (Tables 5, S1). At the top height, the air NH3 concentration for low ACH was estimated to be 0.84 mg/m3 higher than that for medium ACH, and 0.96 mg/m3 higher than that for high ACH. At the middle height, the air NH3 concentration for low ACH was estimated to be 1.62 mg/m3 higher than that for medium ACH, and 1.76 mg/m3 higher than that for high ACH. Finally, at the bottom height, the air NH3 concentration for low ACH was estimated to be 1.02 mg/m3 higher than that for medium ACH, and 1.16 mg/m3 higher than that for high ACH. Within each height, mean concentrations of air NH3 were similar for medium and high ACH (Tables 5, S1).
The approximated total amounts of NH3 produced for low, medium, and high ACH at the middle height were 0.38 ± 0.2, 0.46 ± 0.2 and 0.66 ± 0.2 kg NH3/chamber over the 7-day experimental period.
There was no evidence of non-linearity of the relationship between TDB and air NH3 concentrations at any height, nor for RH at the bottom height. For RH at top and middle heights, there was evidence of reductions in air NH3 concentration only at RH of >60% but, for simplicity, linear relationships were assumed for each of TDB and RH at all heights. There was evidence for interaction between TDB and RH in their effects on air NH3 concentration at the bottom height but not at the top or middle heights (P-values for interaction terms of <0.001, 0.348 and 0.352 respectively).
At the top and middle heights, there were weak associations between temperature and air NH3 concentration. Estimated effects of TDB adjusted for RH were 0.64 mg/m3 and 0.84 mg/m3 respecteively for each 10°C increase in TDB (P = 0.002 and 0.001 respectively). There was also some evidence for a weak association between RH and air NH3 concentration at the top height. The estimated effect adjusted for TDB was a 0.14 mg/m3 decrease for each 10% point increase in RH (P = 0.043). At the bottom height, interaction between TDB and RH was included in the model and TDB was positively associated with air NH3 concentration at RH of 40%, but no association was evident at 80% (estimated increases in air NH3 concentration for each 10°C increase in TDB 1.51 and 0.00 mg/m3 respectively), and RH was weakly positively associated with air NH3 concentration at 15°C, but negatively associated at 30°C (estimated changes in air NH3 concentration for each 10% point increase in RH 0.17 and −0.40 mg/m3 respectively).
Pad-surface conditions during 7 days of the experimental period
Pad moisture appearance and area covered by moisture over the 7 days of the experiment were each associated with BAR and/or ACH (Table 6). Chambers with 100% and 50% bedding rates and high and medium ACH had higher scores for moisture appearance (where higher scores indicate firmer pads) than did the chambers with no bedding treatment (P < 0.001 and 0.002 respectively) and low ACH (P < 0.001 and 0,002 respectively) where pads were more often sloppier and wetter. Relative to 0% bedding, 0% or <10% of the area as wet patches was more likely for chambers with 100% (P < 0.001) and 50% (P = 0.041) bedding treatments (and 10% or more of area as wet patches less likely). Similarly, increasing ACH reduced the odds of larger moisture-covered areas (Table 6). The presumed sources of moisture were mainly cattle urine and water-trough spills, including when the cattle played with the water (Table S2). Increasing bedding to 100% ASEL resulted in the deeper pad than did 0% ASEL (P < 0.001), whereas the changes of ACH appeared unrelated to the estimated pad depth.
Pad-surface condition | BAR (% ASEL) | ACH (number per hour) | |||||
---|---|---|---|---|---|---|---|
0 | 50 | 100 | 20 (low) | 35 (medium) | 52 (high) | ||
Moisture appearance | |||||||
Odds ratio | Reference category | 5.22 | 12.2 | Reference category | 5.68 | 6.22 | |
95% CI | 1.80–15.15 | 4.59–32.2 | 1.921–16.8 | 2.42–15.9 | |||
P-value | 0.002 | <0.001 | 0.002 | <0.001 | |||
Area covered with moisture | |||||||
Odds ratio | Reference category | 0.37 | 0.10 | Reference category | 0.35 | 0.38 | |
95% CI | 0.14–0.96 | 0.031–0.33 | 0.12–1.06 | 0.14–0.99 | |||
P-value | 0.041 | <0.001 | 0.066 | 0.050 | |||
Pad-depth range | |||||||
Odds ratio | Reference category | 1.39 | 2.37 | Reference category | 1.35 | 1.09 | |
95% CI | 0.74–2.59 | 0.031–0.33 | 0.69–2.62 | 0.53–2.62 | |||
P-value | 0.294 | 0.018 | 0.821 | 0.384 |
Pad depth for the 0% bedding treatment (mean ± s.d.; 3.9 ± 3.30 cm) was lower than 50% (6.1 ± 4.62 cm) and 100% (6.7 ± 4.5 cm). For all bedding treatments, most of the pad was pushed to the side of the quarters, resulting in greater depth than in the centre areas of the quarters (Fig. 4). The small portion of pad not accessible by the steer was mostly dry wood shavings (for the 50% and 100% bedding) and some manure. These outside portions of pads weighed, on average, 115.61 ± 29.30 kg (mean ± s.d.), whereas the pads inside the quarters weighed, on average, 88.94 ± 35.46 kg. The concentration of NH4+ in the pad quarters (predicted mean ± s.e.m 1.11 ± 0.04 mg/g pad) was 0.42 mg/g pad higher (95% CI: 0.33–0.51; P < 0.001) than that in the outside portions of the pad (0.70 ± 0.04 mg/g pad). The total pad NH4+ mass (mean ± s.d., grams per chamber) in the quarters was 100.13 ± 47.78, whereas that in the outside was 80.42 ± 39.86.
Fitted values for pad depth on the chamber floor left to right (x-coordinate as defined in Fig. 1) and back to front (y-coordinate) pooled across bedding application rates; grey band indicates 95% confidence intervals for fitted values.
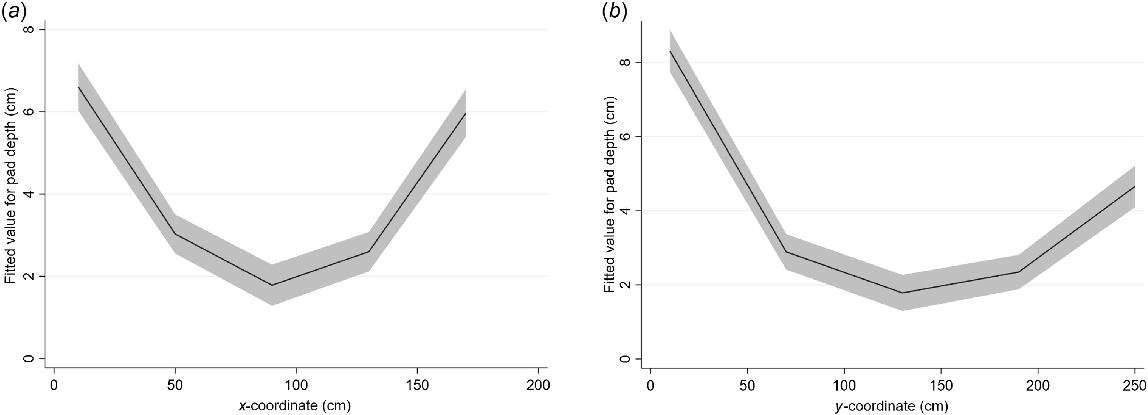
Both pad pH and pad moisture were lower for the 100% bedding treatment (P < 0.001) than for the 50% and 0% bedding (Tables 7, S3). Predicted means for pad bulk density were similar for all BAR. Mean concentration of pad NH4+ (mg/g pad) in the 0% bedding treatment was higher than in the 50% and 100% bedding treatments (predicted means 1.15, 1.01 and 0.95 respectively). However, predicted means for total pad NH4+ content (g/chamber) were similar for all BARs. Pad moisture was lower for the high and medium ACH than for the low ACH. No effects of ACH on other pad properties were evident.
Pad property | BAR (% ASEL) | ACH (number per hour) | Overall P-value | |||||||
---|---|---|---|---|---|---|---|---|---|---|
0 | 50 | 100 | 20 (low) | 35 (medium) | 52 (high) | Bedding rate (B) | ACH (A) | B × A | ||
Pad pH | 7.92 ± 0.37a | 7.69 ± 0.37b | 7.59 ± 0.37b | 7.69 ± 0.37 | 7.79 ± 0.37 | 7.72 ± 0.37 | <0.001 | 0.188 | 0.376 | |
Pad moisture (%) | 80.34 ± 0.59a | 78.66 ± 0.59a,b | 75.34 ± 0.59b | 79.34 ± 0.59a | 77.42 ± 0.59b | 77.58 ± 0.59b | <0.001 | 0.049 | 0.822 | |
Pad bulk density (kg/m3) | 990.0 ± 26.50 | 1002.3 ± 26.50 | 1040.7 ± 26.50 | 997.2 ± 28.40 | 1002.1 ± 28.40 | 1033.8 ± 28.40 | 0.335 | 0.651 | 0.702 | |
Pad NH4 + concentration (mg/g pad), wet basis | 1.18 ± 0.041a | 1.01 ± 0.0.041b | 0.95 ± 0.041b | 1.04 ± 0.041 | 1.05 ± 0.041 | 1.06 ± 0.041 | <0.001 | 0.941 | 0.707 | |
Total pad NH4 + mass (g/chamber), wet basis | 89.6 ± 8.21 | 101.2 ± 8.21 | 91.0 ± 8.21 | 100.4 ± 8.21 | 87.27 ± 8.21 | 94.8 ± 8.21 | 0.415 | 0.602 | 0.899 |
Means within a row and within a category followed by the same letter or no letter do not differ statistically significantly (at P < 0.05).
Our results showed that any correlations between pad moisture and each of pad pH (r = 0.099; 95% CI: −0.091 to 0.283; P = 0.304) and pad NH4+ concentration (r = 0.051; 95% CI: −0.140 to 0.237; P = 0.604) are weak (Table S4). Pad moisture and pad bulk density were negatively correlated (r = −0.383; 95% CI: 0.020–0.382; P = 0.031), and pad moisture and total pad NH4+ mass were positively correlated (r = 0.228; 95% CI: 0.040–0.399; P = 0.018). Pad NH4+ concentration and total pad NH4+ mass were quite closely positively correlated (r = 0.612; 95% CI: 0.478–0.718; P < 0.001).
Associations between pad properties and air NH3 concentration
Air NH3 concentration on Day 7 was positively associated with pad NH4+ concentration (mg/g pad; Table 8). Estimated coefficients for associations between total mass of pad NH4+ in the chamber on Day 7 and air NH3 concentration were also positive, although imprecise (Table 8).
Pad property and statistic | Estimated change in air NH3 concentration (mg/m3) for each extra unit in pad property variable | |||
---|---|---|---|---|
Top height (2.3 m above the pad) | Middle height (1.8 m above the pad) | Bottom height (0.3 m above the pad) | ||
Pad pH | ||||
Coefficient A | 0.16 | 0.59 | 0.43 | |
95% CI | −0.73–1.04 | −0.66–1.83 | −0.31–1.16 | |
P-value | 0.726 | 0.355 | 0.255 | |
Pad moisture (%) | ||||
Coefficient A | −0.004 | −0.02 | 0.00003 | |
95% CI | −0.06–0.05 | −0.01–0.05 | −0.05–0.05 | |
P-value | 0.876 | 0.569 | 0.999 | |
Pad bulk density (kg/m3) | ||||
Coefficient A | −0.001 | −0.001 | −0.001 | |
95% CI | −0.002–0.001 | −0.003–0.001 | −0.002–0.0003 | |
P-value | 0.340 | 0.451 | 0.168 | |
Pad NH4+ concentration (mg/g pad)-wet basis | ||||
Coefficient A | 0.95 | 1.24 | 0.84 | |
95% CI | −0.001–1.89 | −0.11–2.6 | 0.04–1.64 | |
P-value | 0.050 | 0.072 | 0.039 | |
Total pad NH4+ mass (g/chamber)-wet basis | ||||
Coefficient A | 0.002 | 0.001 | 0.0002 | |
95% CI | −0.003–0.001 | −0.006–0.008 | −0.004–0.004 | |
P-value | 0.418 | 0.774 | 0.925 |
Effects of BAR on lying behaviour
Duration of time to when each steer first commenced lying on Day 1 for all runs was shorter for chambers with more bedding. Steers first laid down at 1.14 ± 0.701 h from 20 min after the steers were fed their first meal after entering the chamber and the chamber door was closed (Time 0) for 100% bedding and at 2.06 ± 1.134 h for 50% bedding, compared with steers with no bedding that laid down for the first time at 3.89 ± 1.452 h (P < 0.001 for each of 100% and 50% bedding relative to 0% bedding).
Steers were lying most often throughout the 24-h time period on each of the three sampling days for all runs for the 100% bedding treatment (Fig. 5). On Day 1, odds of lying were 4.5 times higher (95% CI 3.4–5.9) on 100% bedding and 2.1 times higher (95% CI 1.5–2.9) on 50% bedding than in 0% BAR treatment. Effects of bedding were less on Days 3 and 6, but odds of lying were still higher where higher BAR was applied (Day 3 odds-ratio estimates 1.5 (95% CI 1.3–1.8) and 1.3 (95% CI 1.1–1.5) for 100% and 50% bedding respectively; Day 6 odds-ratio estimates 1.3 (95% CI 1.1–1.5) and 1.2 (95% CI 1.0–1.5) for 100% and 50% bedding respectively).
Steers standing and lying frequency (%) in the chambers for 0%, 50% and 100% Australian Standards for the Export of Livestock (ASEL) bedding application rate on Days 1, 3 and 6.
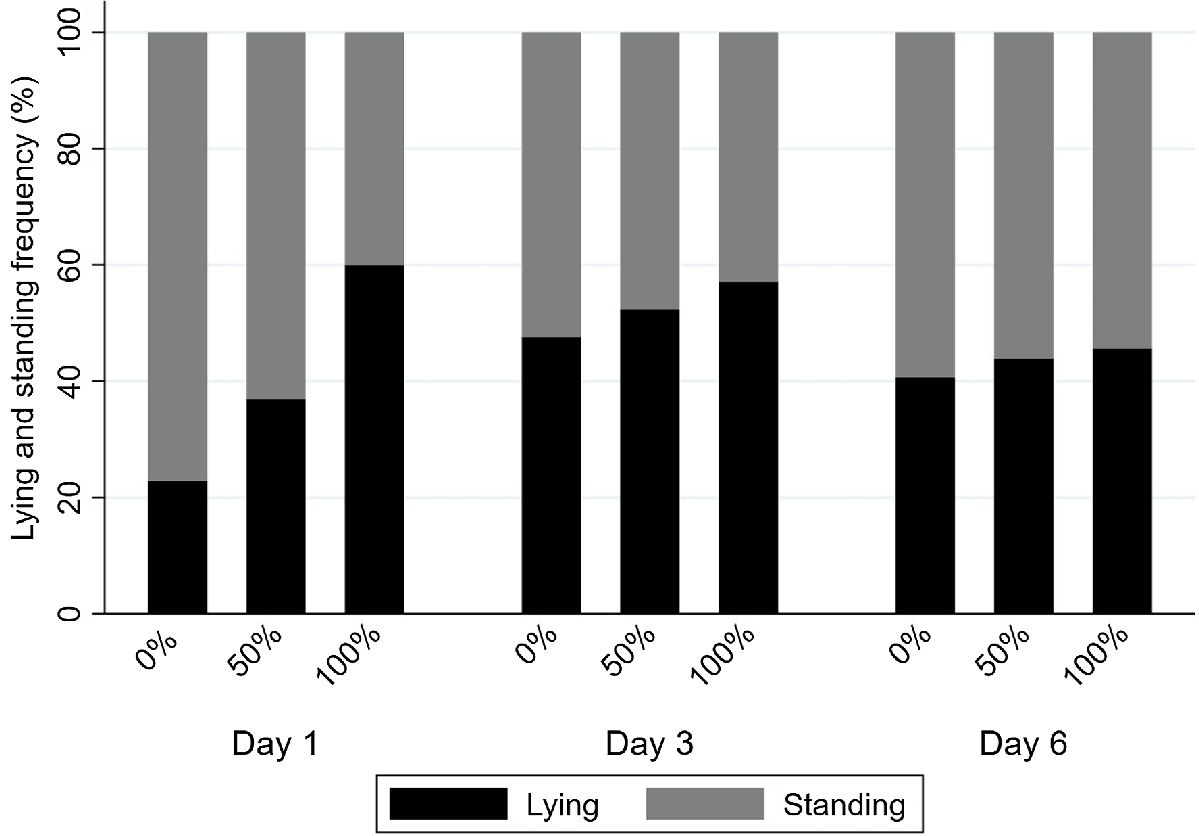
Effects of ACH on lying behaviour were inconsistent among days (results not shown). The steers were more likely to be lying at any particular time point during the night-time period than daytime with odds ratios for lying on Day 1 (odds ratio for night-time relative to daytime 3.3; 95% CI 2.6–4.0) and similar on Days 3 and 6.
Discussion
In general, air NH3 concentration in the chambers increased for each sequential day of the experimental run following the accumulation of deposited manure (urine and faeces) on the floor, most likely because these were sources of urea, and urease enzyme, which resulted in the formation of NH4+ that was volatilised as NH3 gas. Of the 702 air NH3 concentrations measured in the chambers during the study, only 4% were greater than 10 mg/m3, with a maximum concentration of 12.5 mg/m3. This concentration was lower than that reported on ships that could reach 17.07 mg/m3 (Zhang et al. 2017) and even up to 41.1 mg/m3 (Pines and Phillips 2011) and was likely due to the moderate chamber TDB values, ranging from 17°C to 24°C. According to Adegoke and Ku (2023), urease enzyme activity increased exponentially at temperatures greater than 25°C releasing NH3 in the gaseous phase. Given that our TDB remained in the lower end of this range, it is likely to have led to slower urease activity, and, consequently, lower air NH3 concentrations. These findings suggested that the lower temperatures in the current study might inhibit the full potential of NH3 volatilisation, which would likely increase under the higher temperatures typical of live-export conditions. The relationship between TDB and the rate of volatilisation of NH3 has been previously demonstrated by Sommer and Olesen (1991) and Sommer et al. (1991), which showed that the rate of loss of NH3 in the cattle slurry through volatilisation was related to TDB increase.
In the current study, the air NH3 concentration was lower in the morning, and more concentrated in the afternoons. In the afternoon, when the TDB increased, the RH in the chamber decreased, which was probably related to a high water-vapour deficit (VPD) that can cause drier air (Runkle 2021). It is likely that this condition contributed to more rapid moisture evaporation from the manure pad, leaving less liquid available to retain NH3 as NH4+, and potentially increase NH3 volatilisation from the pad surface. This result was consistent with Whitehead and Raistrick (1991) finding, which noted that greater urea hydrolysis and nitrification were noticeable when the air was warmer and drier.
In the present study, we found no evidence of an interaction between BAR and ACH in their effects on air NH3 concentration, suggesting that the relationship between these variables might be independent under the given experimental conditions. It appeared that increasing BAR and adjusting it with high ACH was not mutually simulating the reduction of air NH3 concentration as was hypothesised, indicating that each variable may utilise its impacts separately. This condition might be explained by the different mechanism of action between BAR and ACH in influencing air NH3. Bedding primarily affected the initial source of air NH3 by influencing the concentration of NH4+ on the pad level. In contrast, ACH affected the removal of NH3 gas once it volatilised from the pad surface to the higher measuring point. However, the results showed that the total pad NH4+ mass per chamber was not significantly different for each BAR treatment. Therefore, under this similar condition, the concentration of air NH3 at any measuring height was dependent mostly on the amount of air flow in the chamber.
When considering individual variables, there was a relationship between ACH and air NH3 concentration in the chamber at each measuring height, but no marked effects for pad pH, bulk density, and pad NH3. Contrastingly, air NH3 concentration was similar across BAR treatments for all measuring heights; however, there were differences in pad pH, pad moisture, and pad NH3 concentration related to the BAR treatments.
ACH was strongly associated with air NH3 concentration (mg/m3) of all BAR treatments and at any measuring height. Lower air NH3 concentrations were observed in the chambers with medium (35) and high (52) ACH than with low ACH (20) for all measuring heights. Meanwhile, air NH3 concentrations were similar at high and medium ACH. Air change rate for the high ACH was 1.6 times greater than that for the low ACH. Therefore, under steady-state conditions, if volatilisation rates were unchanged and NH3 concentration in the air was the same throughout the chamber (so that NH3 concentration in exiting air was the same as that at any point in the chamber), air NH3 concentrations at 52 ACH would be expected to be 38% of those at 20 ACH. In fact, the observed mean NH3 concentrations at 52 ACH at top, middle and bottom heights respectively, were 76%, 68% and 72% of those at 20 ACH. This difference was potentially a result of the higher volatilisation rates at the higher ACH (Kim and Kim 2020; Kumar et al. 2022; Kamp et al. 2024), supported by the calculated total NH3 (using the mean concentration of NH3 at the middle height), which showed that the high ACH produced 1.7 times more NH3 than did the low ACH. Furthermore, although the deposition rate of N by the cattle would be similar for both treatments and there was no observable difference in measured pad NH4+ concentration among the different ACH treatments, the low ACH produced 0.283 kg less air NH3 over the 7 days, than did the high ACH. To determine why this may be the case, first consideration was that the urease enzyme hydrolyses urea into carbonic acid (H2CO3) and NH3, which remains in equilibrium with bicarbonate (HCO3−) and ammonium (NH4+) (Dai and Karring 2014). Given that the pad pH in this study was ~7.8, most N would be expected to exist in the NH4+ form, but the Berthelot method measures ammoniacal N and does not appear to distinguish between NH3 and NH4+ (Creevey 2022; Alwael et al. 2024). One plausible explanation for less air NH3 being produced under low ACH is a slower rate of urea hydrolysis, leaving more N in the form of urea-N, which had not yet undergone hydrolysis. However, further experimental investigation would be necessary to confirm this hypothesis. There may also be additional factors beyond pad pH and NH4+ concentration, such as airflow dynamics, that need to be considered.
In the current study, pad moisture was lower for the high and medium ACH than the low ACH. However, even though the oscillating fans mixed the air to create a homogenous downward airflow, with empty chambers, the measured velocity of the air at pad surface was 0 and we assume that it would be the same if two animals were in each of these small spaces. According to Li (2023), when the ventilation-duct system is positioned in the upper room space of a confined space, the supply air runs down along vertical walls or columns to the floor, then shifts horizontally, forming a thin, evenly spread layer across the floor, moving with low velocity, and it is possible that that horizontal velocity was higher at higher air change rates.
Another possibility is that the rate of removal of NH3 from the chamber does not increase in proportion to the increase in air change rate, for example, because of differences in the vertical profile of NH3 concentrations from top to bottom. However, our results did not support this because the ratios of means for top and middle heights relative to the bottom level were similar within each air change rate. In summary, these results indicated that even though the rate of ACH increases the volatilisation of NH3 from the pad surface, the higher ACH still reduces air NH3 concentration. The negative association between ACH and air NH3 concentration supports the results of previous studies, which demonstrated that NH3 concentrations are influenced by air movement (Sheffield et al. 2007; Saha et al. 2010). This finding in the current study suggests that increasing the ACH rate in the cattle pen lowers the concentration of air NH3. Additionally, the risk of disease on board might be reduced because the concentration of air NH3 is lower at an increased ACH rate (MAMIC 2001).
Even though high and medium ACH reduced the air NH3 more than did the low ACH, we found no evidence that ACH influences pad NH4+ concentrations. The concentration of NH4+ in the pad is influenced more by the chemical and biological processes within the bedding and manure, such as urea hydrolysis by urease-producing bacteria. Air changes less directly affect these processes, owing to airflow mainly being in contact with the pad’s surface rather than effectively penetrating the pad layer to influence these reactions. Increasing the airflow rate in the chamber over the pad surface increased the pad surface conditions, which were drier, firmer, and deeper under high and medium ACH than under low ACH. These results showed that the rate of ACH was associated with pad moisture. Applying higher ACH rates and bedding (50% and 100% ASEL) in the chamber improved the pad condition, with the pad being drier, denser, and less moist.
The opposite was found for effects of BAR treatments on air NH3 and pad NH4+. The pads were a mixture of manure (faeces + urine) and spilled drinking water for no-bedding treatment, and manure, spilled water and wood shavings for 50% and 100% ASEL bedding treatments. When bedding was applied to the chamber, the pad was built and formed deeper and denser layers. The bedding material in the pad sample has reduced pad pH, moisture, and pad NH4+ concentration (mg/g pad) for higher bedding-rate treatments relative to 0% bedding, but bulk density and total pad NH4+ mass (g/chamber) appeared similar for all bedding treatments. The higher concentration of NH4+ per gram of pad in the no-bedding treatment was due to the pad consisting entirely of manure containing abundant organic matter and nitrogenous compounds, including ionised NH4+. The absence of bedding material in this treatment to mix with or dilute the manure presumably resulted in a more concentrated N content and a lower pH. The differences in pad NH4+ concentration between no bedding, 50% and 100% bedding were modest, probably owing to the slight pH range, such that BAR had no marked effect on air NH3 concentration. Nevertheless, these results indicated that a higher bedding application rate increased the ability of bedding to absorb manure and other fluid waste and keep the pad in low pH and drier condition.
Another reason for the lack of influence of BAR on air NH3 concentration could be attributed to the narrow range of pad pH across bedding treatments. Even though lower pH values and higher moisture values than with dry bedding (pH 8 and 91% DM) were observed in the pad on Day 7. The small variation in pad pH among BAR treatments, ranging from 7.5 to 7.9 when the bedding became saturated with manure, was insufficient to result in significant differences in pad NH4+ or air NH3 concentration. Additionally, the increase in pad moisture after 7 days (75–80% moisture), particularly for the 50% and 100% BAR treatments, was a consequence of the bedding absorbing urine and water. In this study, no correlation was evident for pad pH and pad moisture directly affecting the air NH3 concentration. This result was in contrast with the previous study that showed pad pH and DM content influence NH3 volatilisation (Sommer and Olesen 1991; Olesen and Sommer 1993).
This study showed no evidence that air NH3 concentration changes by BAR treatments, which could be due to pad distribution and animal activities in the chamber. In the current study, the surface area was the same in all chambers (3.97 m2). Thus, the size of the area contaminated by the excreta was also similar. This result is supported by Webb et al. (2005), who stated that when estimating NH3 emissions, the surface area of the facilities should be considered.
Fig. 5 shows that the pad formation in the chamber was deeper on the side of the quarter than in the centre. The pad was spread on the side of the chamber rather than the centre because of the steer activities (standing, lying and locomoting) within the central areas of the chambers. This increased animal activity in the centre of the pen area pushed the bedding and some of the manure to the side, resulting in a deeper pad depth and lower NH4+ concentrations than in the centre sampling points. As a result, more pad NH4+ was trapped in the undisturbed bedding layers on the side of the pen than in the centre, which the steers frequently disturbed. During the measurement of air NH3 concentrations, the sensor was lowered into the centre of the chambers (Fig. 1). Thus, if there was minimal lateral air movement, the recorded air NH3 would mainly have reflected concentrations in air that had escaped from the pad layer in the centre of the chamber floor instead of from the sides. As a result, the total measured air NH3 concentrations would be expected to have been more influenced by the pad NH4+ concentration (mg/g) than of the total NH4+ mass in the pad. The relationships between pad NH4+ concentration (mg/g pad) and air NH3 concentration (mg/m3) in the chamber are shown in Table 8, where the air NH3 in the chamber increased with the concentration of NH4+ in the pad.
The activities of the steers in the chambers may have contributed to the differences in air NH3 concentrations among measuring heights, where the highest concentration was at the middle height. The study of Bleizgys and Naujokienė (2023) showed that NH3 emission from the pad surface decreased as a result of the decrease of temperature differences between the manure layer and surface and moisture evaporation. In the current study, the air NH3 at the bottom height was potentially lower owing to the prevention of volatilisation of NH3 from the top layer of the pad when the steers were lying down, covering the pad surface. However, when air NH3 was measured in the chambers, the steers would stand up and disturb the pad, leading to the potential flux of NH3 from the pad surface. Because NH3 gas is lighter than air, the volatilisation of air NH3 would be expected to rise to the next vertical measuring spot, middle height, where the concentration of air NH3 was found to be the highest. At this height, the animals may have created a ‘temporary blanket effect’, with their bodies trapping NH3 between the pad surface and the animals’ underbelly/frame, concentrating the gas in this zone before reaching the top height once the steers had moved. This may also explain why not all air NH3 reached the top height. These patterns by height were consistent for every BAR and ACH treatment combination.
Air NH3 was generally observed at higher concentrations at the middle height, which was approximately head height for a standing human or steer. However, most measured concentrations of air NH3 in this study were below 25 ppm or 17 mg/m3, which is the level recommended for the livestock export industry in Australia (MAMIC 2001; Phillips et al. 2010). Therefore, the exposure of the air NH3 in this study did not exceed the critical exposure limit to be considered toxic for humans and animals. Nevertheless, it is essential to note that these values were measured under moderate temperature conditions and may not fully represent typical conditions on live-export ships. Consequently, although the study provides valuable data, caution is needed when applying this model in actual livestock-export scenarios under warmer conditions.
When bedding was applied in the chamber, the pad was deeper and denser BAR affected the physical characteristics of the pad. The results demonstrated that pad with 100% BAR was significantly deeper than that with 50% or 0% BAR. The deeper pad was due not only to the amount of bedding applied but also to the ability of bedding particles to trap moisture and maintain pad weight. The negative correlation between pad moisture and pad bulk density suggested that as the moisture increased, the bulk density decreased. When bedding was still dry or less moist, presumably the bulk density was lower because of air space between bedding particles. In this study, because the bedding was mixed with manure, the addition of moisture in the pad generated pore-water pressures, causing the pad to be less compacted (Etim Udom and Ehilegbu 2018) and the bond between soil particles was weakened (Salman and Kiss 2018). The pad condition appeared to deteriorate as the study progressed, indicating that the ability of bedding to absorb manure was reduced over time, and additional bedding or new bedding applications could be required (Cowley et al. 2019).
Although BAR treatments showed no evidence of affecting air NH3 concentration, bedding increased frequency of lying in this study. First, the preference of the steers to lie on the bedding compared with no-bedding treatment was shown by the shorter time it took for steers to commence lying on Day 1 than in the no-bedding (0%) chambers. From an animal-welfare perspective, being able to provide a surface that increased lying and subsequent resting behaviour may reduce the animals’ stress, fatigue, and potential for injury. Second, the drier pad conditions and low pad moisture, as a result of the application of bedding, resulted in the steers lying at more observation timepoints (indicating that they spent more time lying) when more bedding was applied (50% and 100% ASEL) than under the no-bedding treatment (not including feeding and sampling times). Presumably, the steers spent more time standing with no bedding to potentially avoid the excreta that was covering the pen floor. However, on Day 7 of observation in the experiment, most dry bedding was pushed to the side of all chambers and could not be utilised by the animals (Fig. 4). For each consecutive day, the pad was thinner, and the absorbing ability was reduced; thus, a wetter pad was formed. Despite this, the odds of steers lying on Days 3 and 6 were still higher where bedding was provided (50% and 100% ASEL); however, the reduced strengths of association between BAR and lying frequency later in the study period were presumably due to increased pad moisture over time. These results support those of the previous studies in that cattle prefer to lie down on clean, dry, and uncontaminated bedding (Crafter et al. 2006; Tucker et al. 2009; O’Connor et al. 2019; Tait et al. 2023).
Conclusions
The findings of this study emphasise the vital role of bedding application rates (BAR) on minimising potential pad NH4+ and air change rates (ACH) in reducing air NH3 concentration within a confined setting of live-export context. Air NH3 concentrations in the current study were mostly lower than the concentrations previously reported on livestock-export ships (Pines and Phillips 2011; Zhang et al. 2017) and we suggest that this was due to the modest ambient temperature and RH in the chambers. The lowest air change rate (ACH) in this study aligned with AMSA’s minimum requirements. Under conditions such as those in this experiment, increasing ACH to more than double the standard effectively reduced air NH3 concentration by 0.75–1.88 mg/m3 (95% CI; P < 0.001). Additionally, the bedding applications used in this study reduced pad pH, pad moisture and pad NH4+ concentration and improved overall pad conditions.
Results from this study highlight the importance of strategic bedding management for those livestock-export voyages that require bedding application. Daily assessment of the pad physical conditions can inform potential washout times and need for provision of new bedding where the existing bedding has deteriorated or when the air NH3 concentrations have risen to levels that could be harmful to both human and animals as a potential effect of pad NH4+ accumulation. Future work should aim to identify the effects of BAR and ACH under other climatic conditions, including higher TDB and RH. The results of this study will assist in predicting air NH3 and pad NH4+ concentrations in simulated livestock-export conditions and help inform optimal BAR and ACH to help improve cattle welfare when exported from Australian ports.
For practical applications, this suggests that to minimise air NH₃ concentrations in confined animal housing, focusing on optimising ACH might be more effective than adjusting BAR. However, BAR should not be neglected because it plays a crucial role in pad management, which could have other welfare and environmental implications. Although BAR may not directly affect air NH3, it remains important for managing other pad-related factors, which can influence animal comfort and welfare, as well as long-term environmental conditions within the housing system. Proper bedding management can contribute to a healthier environment by controlling moisture, pad consistency and by potentially reducing NH3 volatilisation at the source.
Data availability
The data supporting the conclusions is confidential and will be released only given permission by the funding bodies.
Declaration of funding
This study was funded by Meat and Livestock Australia Pty Ltd, North Sydney, NSW, Australia, LiveCorp North Sydney, NSW, Australia, and the Australian Government, Canberra, ACT, Australia (W.LIV.0299).
Author contributions
L. T., F. C. and J. W.: research funding acquisition and original draft editing. L. T.: project administration. L. T., F. C., and I. H.: conceptualisation and methodology. I. H., J. W. and J. M.: data curation and formal analysis. I. H.: original draft preparation. I. H., L. T., J. W., S. C. and F. C.: sample and data collection. I. H., L. T., J. W., and F. C.: experimental conduct. I. H., J. M., J. W., L. T., and F. C.: interpretation of data. All authors approved the final version of the paper.
Acknowledgements
We acknowledge the Department of Primary Industries, Grafton, the owners of the steers and whose cooperation made this study a success. We also thank the University of New England, Centre for Animal Research and Teaching, for the provision of facilities and technical support. Thanks go you to Amelia de Almeida, Brendan Doyle, Haylee Herriot, and Mal Kul for their assistance with experimental conduct and sampling days and other University of New England Ruminant Research Group members for assistance on Day 7 sampling days.
References
Adegoke TO, Ku H-H (2023) Temperature response of ammonia emission from sandy loam soil amended with manure compost and urea. Environmental Technology & Innovation 31, 103226.
| Crossref | Google Scholar |
Alwael H, Alharthi AS, Dabi MM, Oubaha M, El-Shahawi MS (2024) A highly sensitive electrochemical sensing probe incorporating classical Berthelot’s reaction and glassy carbon electrode for measuring ultra-trace levels of ammonia/NH4+ in water. Electrochemistry Communications 162, 107686.
| Crossref | Google Scholar |
Australian Maritime Safety Authority (2022) Deck washing guidance for livestock vessels carrying cattle and/or buffalo. Available at https://www.amsa.gov.au/vessels-operators/cargoes-and-dangerous-goods/deck-washing-guidance-livestock-vessels-carrying
Bleizgys R, Naujokienė V (2023) Ammonia emissions from cattle manure under variable moisture exchange between the manure and the environment. Agronomy 13, 1555.
| Crossref | Google Scholar |
Bristow AW, Whitehead DC, Cockburn JE (1992) Nitrogenous constituents in the urine of cattle, sheep and goats. Journal of the Science of Food and Agriculture 59, 387-394.
| Crossref | Google Scholar |
Cowley F, Tait A, Wilkes J, Taylor P, Hegarty R, McGilchrist P (2019) B.FLT.0244: Graded levels of woodchip during wet feedlot conditions. Meat and Livestock Australia Limited. Available at https://hdl.handle.net/1959.11/29138
Dai X, Karring H (2014) A determination and comparison of urease activity in feces and fresh manure from pig and cattle in relation to ammonia production and pH changes. PLoS ONE 9, e110402.
| Crossref | Google Scholar | PubMed |
Etim Udom B, Ehilegbu J (2018) Critical moisture content, bulk density relationships and compaction of cultivated and uncultivated soils in the humid tropics. Asian Soil Research Journal 1, 1-9.
| Crossref | Google Scholar |
Kamp JN, Hafner SD, Huijsmans J, van Boheemen K, Götze H, Pacholski A, Pedersen J (2024) Comparison of two micrometeorological and three enclosure methods for measuring ammonia emission after slurry application in two field experiments. Agricultural and Forest Meteorology 354, 110077.
| Crossref | Google Scholar |
Kim M-S, Kim J-G (2020) Effects of thickness of solid media, ventilation rate, and chamber volume on ammonia emission from liquid fertilizers using dynamic chamber-capture system (DCS). Agriculture 10, 226.
| Crossref | Google Scholar |
Kumar N, Gedam P, Gupta SK (2022) Investigating the dynamics of ammonia volatilisation and the role of additives in thermal digestion of food waste. Journal of Environmental Management 323, 116312.
| Crossref | Google Scholar | PubMed |
Liu Z, Liu Y, Murphy JP, Maghirang R (2017) Ammonia and methane emission factors from cattle operations expressed as losses of dietary nutrients or energy. Agriculture 7, 16.
| Crossref | Google Scholar |
McCarthy M, Banhazi T (2016) Bedding management and air quality on livestock vessel-a literature review. Meat and Livestock Australia. Report No. W.LIV.0290, Sydney, NSW, Australia. Available at http://www.livecorp.com.au/LC/files/4e/4ebf1750-693c-4182-bc47-71fe027294e3.pdf
Ngwabie NM, Jeppsson K-H, Gustafsson G, Nimmermark S (2011) Effects of animal activity and air temperature on methane and ammonia emissions from a naturally ventilated building for dairy cows. Atmospheric Environment 45, 6760-6768.
| Crossref | Google Scholar |
Olesen JE, Sommer SG (1993) Modelling effects of wind speed and surface cover on ammonia volatilization from stored pig slurry. Atmospheric Environment. Part A. General Topics 27, 2567-2574.
| Crossref | Google Scholar |
O’Connor C, Dowling S, Cave V, Webster J (2019) Cow lying behaviour and bedding quality changes during five weeks on a stand-off pad. Animals 9, 257.
| Crossref | Google Scholar |
Petherick JC, Phillips CJC (2009) Space allowances for confined livestock and their determination from allometric principles. Applied Animal Behaviour Science 117, 1-12.
| Crossref | Google Scholar |
Phillips CJC, Pines MK, Latter M, Muller T, Petherick JC, Norman ST, Gaughan JB (2010) The physiological and behavioral responses of steers to gaseous ammonia in simulated long-distance transport by ship. Journal of Animal Science 88, 3579-3589.
| Crossref | Google Scholar |
Pines MK, Phillips CJC (2011) Accumulation of ammonia and other potentially noxious gases on live export shipments from Australia to the Middle East. Journal of Environmental Monitoring 13, 2798-2807.
| Crossref | Google Scholar | PubMed |
Runkle E (2021) Water vapor-pressure deficit. Available at https://gpnmag.com/article/water-vapor-pressure-deficit/
Saha CK, Zhang G, Ni J-Q (2010) Airflow and concentration characterisation and ammonia mass transfer modelling in wind tunnel studies. Biosystems Engineering 107, 328-340.
| Crossref | Google Scholar |
Salman ND, Kiss P (2018) Survey: effect of bulk density and moisture content of soil on the penetration resistance and penetration depth. Institute for Process Engineering 17, 109-118.
| Google Scholar |
Sommer SG, Olesen JE (1991) Effects of dry matter content and temperature on ammonia loss from surface-applied cattle slurry. Journal of Environmental Quality 20, 679-683.
| Crossref | Google Scholar |
Sommer SG, Olesen JE, Christensen BT (1991) Effects of temperature, wind speed and air humidity on ammonia volatilization from surface applied cattle slurry. The Journal of Agricultural Science 117, 91-100.
| Crossref | Google Scholar |
Tait LA, Taylor PS, Wilkes J, McGilchrist P, Reid J, Cowley FC (2023) Effect of woodchip bedding during wet conditions on feedlot cattle productivity, behaviour and relative adrenal gland weight. Animal 17, 101032.
| Crossref | Google Scholar |
Tucker CB, Weary DM, von Keyserlingk MAG, Beauchemin KA (2009) Cow comfort in tie-stalls: increased depth of shavings or straw bedding increases lying time. Journal of Dairy Science 92, 2684-2690.
| Crossref | Google Scholar | PubMed |
Webb J, Menzi H, Pain BF, Misselbrook TH, Dämmgen U, Hendriks H, Döhler H (2005) Managing ammonia emissions from livestock production in Europe. Environmental Pollution 135, 399-406.
| Crossref | Google Scholar | PubMed |
Whitehead DC, Raistrick N (1991) Effects of some environmental factors on ammonia volatilization from simulated livestock urine applied to soil. Biology and Fertility of Soils 11, 279-284.
| Crossref | Google Scholar |
Wilkes J, Tait AL, Flavel RJ, Turnell J, Cowley FC (2022) Physical and chemical characteristics of feedlot pen substrate bedded with woodchip under wet climatic conditions. The Journal of Agricultural Science 160, 413-422.
| Crossref | Google Scholar |
Ye Z, Zhang G, Seo I-H, Kai P, Saha CK, Wang C, Li B (2009) Airflow characteristics at the surface of manure in a storage pit affected by ventilation rate, floor slat opening, and headspace height. Biosystems Engineering 104, 97-105.
| Crossref | Google Scholar |
Zhang Y, Lisle AT, Phillips CJC (2017) Development of an effective sampling strategy for ammonia, temperature and relative humidity measurement during sheep transport by ship. Biosystems Engineering 155, 12-23.
| Crossref | Google Scholar |