Fire regime impacts on soil microbes, soil organic carbon and ground cover in an Australian tropical savanna
Marissa H. Blunden
A
B
C
D
Abstract
Soil microbes drive the carbon cycle, yet are understudied in relation to long-term fire regimes in tropical savannas.
Explore the impact of fire regime on soil microbes and organic carbon.
We sampled topsoils (0–10 cm) of a tropical savanna near Darwin, Australia, where the frequency and season of fire had been experimentally managed for 17 years. We measured the effects of fire regime on microbial abundance, fungal-to-bacterial (F:B) ratio, soil physicochemistry (organic carbon, total nitrogen, C:N ratio, pH) and vegetative ground cover (grasses, leaf litter).
Microbial abundance was most influenced by fire season, minimally affected by fire frequency and reduced by both grass and litter cover; the magnitude of grass cover effect differed among paleoecological groups (i.e. ancient and modern). Soil organic carbon was not affected by fire treatments, nor was the F:B ratio.
Our data indicate that soil organic carbon, microbial abundance and F:B ratio are slow to change in a tropical savanna despite 17 years of imposed fire regimes.
Soil microbes in savanna ecosystems may have evolved resilience to variable fire regimes and the potential for soil carbon sequestration in Australia from fire suppression is likely limited within human timescales.
Keywords: Australia, bacteria, carbon sequestration, F:B ratio, fire management, fungal-to-bacterial ratio, fungi, microbial abundance, paleoecological grass groups, tropical savanna.
Introduction
Tropical savannas cover approximately 20% of the world’s land surface (Ramankutty and Foley 1999). They experience highly seasonal precipitation, with distinct annual wet and dry seasons (Hutley and Setterfield 2008). Savanna structure is shaped by frequent fires and herbivory, promoting a low tree density and moderate-to-high abundance of C4 grasses (Van Langevelde et al. 2003). Savannas store high proportions of total ecosystem carbon in their soils (~80%; Chen et al. 2003; Dalal and Allen 2008) with grasses in African and North American savannas contributing more, relative to abundance, than woody vegetation (Dass et al. 2018; Coetsee et al. 2023; Zhou et al. 2023). Owing to their low tree density, savannas have been suggested as targets for sequestering carbon in plant biomass and soil organic matter via the suppression of burning and grazing (Grace et al. 2006; Bastin et al. 2019). Aside from the biodiversity impacts of afforesting a distinct grassy biome (Abreu et al. 2017; Bond et al. 2019), net ecosystem carbon gains are difficult to predict owing to the context-specific responses of soil microorganisms, particularly bacteria and fungi (Dooley and Treseder 2012; Pellegrini et al. 2022).
The Earth’s carbon cycle is largely controlled by the metabolic activity of microorganisms (Gougoulias et al. 2014; Liang 2020). This is particularly the case in the pedosphere, which holds the largest terrestrial pool of carbon globally (Jackson et al. 2017). Soil microbes consume inputs from organic (Cotrufo et al. 2013) and inorganic (Uroz et al. 2009) origins from which they produce biomass and extracellular exudates that can accumulate to increase carbon stores. However, microbes also respire carbon-based compounds (notably CO2 and CH4) into the atmosphere, thereby mediating carbon loss from the soil (Bradford et al. 2016; Liu et al. 2022). As such, increasing microbial carbon accumulation relative to carbon loss through respiration may aid the mitigation of climate change (Tao et al. 2023). Although potentially promising, the manipulation of soil microbes requires greater understanding of the processes that affect them (Fierer and Walsh 2023).
Fire affects soil microbiota by causing direct heat-induced mortality and by indirectly altering soil moisture availability, nutrient flows, aboveground vegetation and soil pH (Mataix-Solera et al. 2009; Dooley and Treseder 2012). High fire temperatures can cause surface soil heating beyond the survival threshold of soil microbes, resulting in their direct mortality (Pingree and Kobziar 2019). Indirectly, fires affect soil microbes through altering soil moisture by evaporating water from upper soil layers and removing litter cover, increasing susceptibility to desiccation stress (Manzoni et al. 2012). Nutrient flows are affected, for example, by the combustion of plant litter, a major source of nutrients for saprotrophic microbes (Marañón-Jiménez et al. 2021), and by damage to live plants, reducing resource allocation to belowground biomass and rhizodeposits that feed associated soil microbes (Jones et al. 2009; Teixeira et al. 2022). Fire also increases the inputs of partially combusted pyrogenic materials, which are highly resistant to microbial consumption, therefore potentially contributing to more stable soil organic carbon (SOC) stores (Bruun et al. 2008; Schneider et al. 2011); charcoal-rich soils can, however, positively impact microbial diversity and biomass (Kolb et al. 2009; Lasota et al. 2021).
Global trends suggest that even single fire events reduce microbial biomass and that this effect is amplified by increasing fire intensity (Liu et al. 2023b). Fungi are generally more affected than bacteria by fire (Dooley and Treseder 2012; Pressler et al. 2019), and seem to be driven more by vegetation changes than changes in soil physicochemistry (Vermeire et al. 2021). However, the response of soil microbes to longer-term fire regimes has received little attention. Similarly, soil microbial community structure has been understudied in response to fire regime, but dominance of fungi over bacteria has been linked with systems of limited disturbance (Van Der Heijden et al. 2008; Štursová et al. 2014), and increased carbon-use efficiency and carbon stores (Bailey et al. 2002; Jastrow et al. 2007; Malik et al. 2016).
Although savannas occur globally, they have different origins and ecological histories on each continent (Edwards et al. 2010), requiring an understanding of local conditions to inform their management (Estes and Vermeij 2022). Australian savannas evolved recently (possibly less than ~1 million years ago (Bryceson and Morgan 2022)) compared to savannas on other continents. Today they are comprised of a combination of plant and animal lineages that migrated into the country from southeast Asia, coexisting with unique and ancient Australian-origin taxa. Australian savannas are characterised by a dominant ground layer of tall, flammable, modern-origin grasses intermixed with smaller, ancient-origin endemic grasses (Bryceson et al. 2023). Grass lineages from southeast Asia co-evolved with frequent fire, compared with ancient Australian-origin grasses (Bryceson and Morgan 2022). Understanding local drivers of the microbiome in the context of Australia’s paleoecological distinctiveness is vital before applying principles and practices developed for savannas on other continents.
In this study, we explored the response of soil microbes and SOC to manipulated fire regimes in a long-term experiment in tropical savanna near Darwin, Australia. We measured fungal and bacteria abundance, fungal-to-bacterial (F:B) ratio (a broad measure of community structure), soil physicochemical properties (SOC, total nitrogen, C:N ratio, pH) and ground cover vegetation (grasses, leaf litter). Grasses were categorised according to their historic distributions to understand how species origin may affect below-ground processes. We hypothesised that (i) both fungal and bacterial abundance would be highest in less burnt savanna owing to the diversity and availability of nutrient inputs; (ii) the less burnt savanna would have higher F:B ratio owing to fungal sensitivity to fire and alkaline environments; (iii) SOC would be unaffected by fire regime owing to the low saturation threshold of Australian savanna’s sandy soils and the high rainfall and temperatures of the tropical climate causing rapid decomposition and increased respiration, and (iv) modern native grasses and ancient endemic grasses would have distinct impacts on soil microbial communities.
Methods
Experimental site
The study was conducted at an experimental fire regime site located at the Territory Wildlife Park in Berry Springs, Northern Territory, Australia (12°41′42.25″S, 130°58′50.36″E), 30 km south-east of Darwin (Fig. 1).
Left: Aerial view of plots showing the randomised block design and north to south transect arrangement. Treatments are fire applied every 1–5 years in the early (E) or late (L) dry season. U, unburnt. Right: the location of the experimental site at the Territory Wildlife Park, Northern Territory. Images from Google Earth.
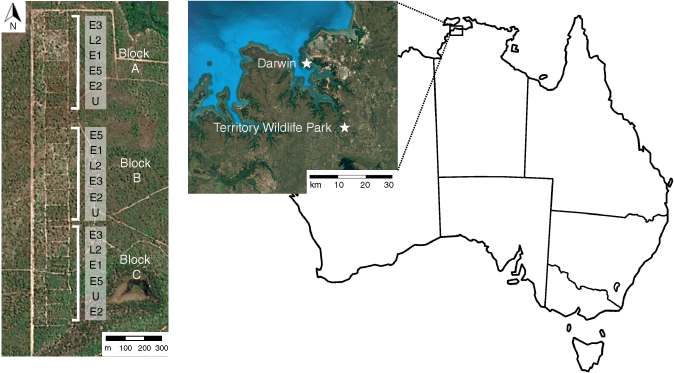
The climate at the study site is tropical monsoonal with highly seasonal rainfall; ~90% of rain falls as monsoonal events in the wet season between November and April. Mean annual precipitation is 1722 mm (Territory Wildlife Park Station, station no. 014264, Bureau of Meteorology, Commonwealth of Australia). The dry season is warm, with minimal precipitation during which plant productivity and soil respiration slow (Richards et al. 2012). The study site has fairly shallow (50–100 cm deep), gravelly red soils over laterite classified as petroferric red Kandosols, characteristic of the Kay land system, with minimal slopes (<5%) (Isbell 2002). They are largely developed from deeply weathered sandstones, siltstones and shales (Wood et al. 1985). Australian Kandosols are most closely related to Inceptisols from the USDA soil taxonomy classification system (Hughes et al. 2018).
Indigenous fire management has been practised in northern Australia from at least 11,000 years ago, altering the pre-anthropogenic pattern of large-scale, infrequent, late dry-season fires ignited by lightning (Bird et al. 2024). Historic Indigenous practices are likely to have consisted of frequent, small-scale, low-intensity fires resulting in a pyrodiverse mosaic of burnt patches, with some areas left unburnt supporting fire-sensitive plant communities (Yibarbuk et al. 2001; Perry et al. 2018; Bowman et al. 2022).
At the start of the fire experiment, the site was defined as mixed eucalypt tropical savanna woodland (as per Specht 1970) dominated by Eucalyptus tetrodonta F. Muell., Eucalyptus miniata A. Cunn. ex Shauer and Corymbia bleeseri (Blakely) K.D. Hill & L.A.S. Johnson. Fire treatments have led to changes in tree, mid-canopy shrub and grass cover (Fig. 2; Levick et al. 2019), with increased dominance of the annual native grass species Sarga intrans F. Muell. ex Benth. in the more frequently burned plots as one of the most distinct changes (S. R. Bryceson and J. W. Morgan, unpubl. data). Changes to vegetation structure at the field experiment have been demonstrated to impact invertebrates including ant diversity (Brassard et al. 2023) and dung beetle abundance (Carvalho et al. 2020). The unburnt plots have been previously reported to have the highest cover of litter (Scott 2008) and the highest soil respiration rate (Richards et al. 2012).
Characteristic examples of the two extremes of vegetation response to fire regime at the study site near Darwin, Australia: (a) an unburnt (>30 years) plot with a leaf litter layer, dense mid-storey and low abundance of grasses; and (b) an E2 (early dry season, biennial fire regime) plot with the ground layer dominated by the native annual grass Sarga intrans and a reduced mid-storey. Photos June, 2023 by MHB.
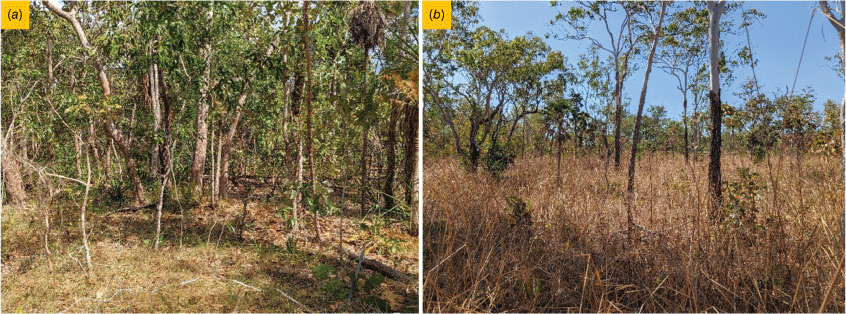
The burning experiment is arranged in a randomised block design along a north–south transect (Fig. 1). The site consists of three replicate blocks of six 1 ha treatment plots, totalling 18 experimental plots. Plots are separated by 4–10 m buffers of cleared vegetation, and blocks are separated by 40 m unburned vegetated buffers. The treatments are prescribed burns in the early dry season (June) at 1, 2, 3 and 5 yearly intervals (E1, E2, E3, E5), one late dry-season (October) burn every 2 years (L2) and an unburnt treatment (U). Fires are applied by drip torch along the northern, eastern and southern perimeters of plots, and coarse woody debris is left to smoulder for up to 3 days, after which fires are extinguished. At the time of sampling, time-since-last-fire (TSF) for E2, E3 and E5 regimes was 2 years, E1 was 1 year and the L2 was 8 months. The unburnt plots had not experienced fire for at least 14 years prior to the start of the experiment in 2004.
Soil sampling
To measure microbial abundance, SOC and pH, soils were sampled prior to the scheduled burns of June 2021. Soil cores to 10 cm were extracted with an auger along two permanent transects located in each plot. One core was extracted from each transect at 20 m intervals, totalling six soil samples per plot (n = 18 per treatment, except for the unburnt and E5 regime where n = 12). Soil samples were kept in sealed Falcon tubes at −4°C until processing, except for approximately 3 days of transport in insulated cooler bags. Cores were processed and analysed individually for further downstream analyses.
Vegetation
We explored the effect of cover of grasses and leaf litter on soil microbial abundance owing to their influential impact on soil physicochemistry and microbes. Litter was used as a proxy of woody vegetation at the most microbially relevant scale for surface soil, due to the influence of litter on microbial decomposition (Liu et al. 2023a). The percentage cover of grasses and leaf litter was quantified in 10 × 10 m contiguous quadrats along the permanent transect lines in each plot in June 2021. The most common nine grass species recorded across the site were used in this analysis (annuals Sarga intrans, Pseudopogonatherum contortum, Schizachyrium fragile, Thaumastochloa spp., and perennials S. plumosum, Heteropogon triticeus, Chrysopogon fallax, Eriachne triseta and E. avenaceae). Percentage cover for each species of grass was recorded and aggregated to calculate total overlapping percentage grass cover. Annuals and perennials occurred in different proportions across the site (Supplementary Table S1). Grass genera were classified according to their paleoecological origins, as modern (‘Sunda’ species from the Southeast Asian continental shelf) or ancient (‘Sahul’ species from the Australian–Papua New Guinea continent) according to Bryceson and Morgan (2022).
Quantitative PCR (qPCR)
Quantitative polymerase chain reaction (qPCR) was used to quantify genetic copy number of bacteria and fungi as a measure of abundance, as well as to calculate F:B ratio. Standard curves were created using gDNA extracted from Aspergillus niger and Escherichia coli. gDNA was extracted using the ZR Fungal/Bacterial DNA MiniPrep™ kit (Zymo Research). Target regions of the bacterial 16S rRNA gene were amplified using the primer pair 1114f and 1275r (Denman and McSweeney 2006). For fungi, ITS1f (Vilgalys and Hester 1990) and 5.8S (Gardes and Bruns 1993) were used, targeting the ITS1 region (Fierer et al. 2005). Each 25 μL PCR reaction contained: 12.5 μL of KAPA2G Fast HotStart, 1.25 μL of forward primer (10 μM), 1.25 μL of reverse primer (10 μM), 8 μL of Milli-Q H2O, and 2 μL of DNA template (40 ng/μL). Cycle conditions were experimentally optimised for use with the Taq polymerase KAPA2G Fast HotStart (Sigma Aldrich) and the Biometra TProfessional Trio Thermocycler (Analytik Jena). For bacterial 16S amplification, PCR cycle settings were: initial denaturation at 95°C for 180 s, followed by 40 cycles of 95°C for 10 s, 60°C for 10 s and 72°C for 1 s, with a final extension of 72°C for 60 s. Cycle settings to amplify fungal ITS region were identical to the bacterial PCR setting except annealing temperature was reduced from 60 to 52°C. To purify amplicons for qPCR standards, amplified DNA was run on a 1.5% agarose gel and extracted using a QIAquick Gel Extraction Mini Kit (QIAGEN). Standards were created by serially diluting the purified amplicons 1 in 10 up to 10-fold with Milli-Q H2O.
DNA was extracted from soil samples using the DNeasy PowerSoil Pro Kit (QIAGEN) as per manufacturer’s instructions. The DNA concentration of each sample was quantified using the Qubit dsDNA HS or BR Assay Kit (Thermo Fisher Scientific Q33231). Extracted DNA was diluted to 5 ng/μL with Milli-Q H2O. qPCR on field samples was conducted with a CFX Connect Real-Time PCR Detection System (BioRad) using the same primers described above. Each 10 μL reaction contained: 1 μL Milli-Q H2O, 5 μL SensiFAST™ SYBR and Fluorescein Mix (Bioline), 1 μL of forward primer (10 μM), 1 μL of reverse primer (10 μM) and 2 μL template DNA. Each qPCR assay included 10-fold diluted standards (10−1 to 10−10) in triplicate and three no-template controls. There were six biological replicates for each plot, except for two blocks with only two per plot. For bacteria, qPCR cycle conditions followed the protocol developed by Klevenhusen et al. (2011): an initial denaturation at 95°C for 20 s, followed by 40 cycles of 95°C for 3 s and 61.5°C for 30 s, followed by a melt curve increasing 1°C every 30 s from 60 to 95°C. The protocol by Wolfe et al. (2010) was followed for fungi: initial denaturation of 95°C for 600 s, followed by 35 cycles of 95°C for 30 s, 55°C for 30 s and 72°C for 60 s, with a final extension of 72°C for 300 s. qPCR fluorescence detection thresholds were fitted for highest efficiency score and R2 value. Efficiency scores were between 91 and 101, with R2 values greater than 0.991. Starting quantities were reverse-normalised by their original dilution factor to give absolute abundance of copy number per soil sample.
Soil physicochemistry
For measurement of percentage SOC, total nitrogen (TN) and pH, 5 g of each soil sample (collected from transects in each plot for microbial abundance) was dried for 86 h at 30°C, sieved to 1 mm and ground to a fine powder using a ball mill (Retsch MM400, Hanau, Germany) at 30 repititions per second for 5 min. A total of 30 mg of each sample was analysed for SOC and TN with a dry combustion CHNS elemental analyser (Perkin Elmer 2400 Series II, USA). Dry combustion at 925°C combusts all carbon and nitrogen including carbonates and charcoal; however, the value obtained for carbon was taken to be only of organic origin as Kandosols are typified by their lack of carbonates (Isbell 2002). For pH, 1 g of soil was added to 5 mL 0.01 M CaCl2, shaken for 1 h and left to sit for 1 h, allowing sediment to settle (Minasny et al. 2011). Soil pH measurements were conducted using a Hanna HI 2211 pH meter (Hanna Instruments, Inc.).
Statistical analysis
The statistical analyses used in this study are fully reproducible and accessible at https://github.com/Applied-and-Environmental-Microbiology/burning-for-biodiversity. All statistical analyses were performed using R v4.2.2.
To evaluate the effect of fire treatment on microbial abundance, F:B ratio and SOC, we employed linear and generalised-linear mixed models using lme4 v1.1.31 with the unburnt treatment set as the reference level (Bates et al. 2015). Continuous variables (F:B ratio, organic carbon) were fitted using a Gaussian error distribution with block as a random effect. Counts (bacterial and fungal abundances) were fitted on a Poisson error distribution with samples nested in block as random effects. We tested and visualised the assumptions of residual normality, homogeneity and dispersion using DHARMa v0.4.6 (Hartig 2022). Differences in effect sizes between treatments were assessed using parameters v0.21.3 (Lüdecke et al. 2020). We calculated marginal means and pairwise comparisons of treatments using emmeans v1.8.2 (Lenth 2023), and P-values were Tukey-adjusted. To explore the effect that TSF might have on microbial abundance, F:B ratio and SOC, we employed the same method as above but with TSF as the explanatory variable.
To further investigate how fire treatment and soil chemistry influenced soil microbial abundance and F:B ratio, we fitted structural equation models (SEMs) using piecewiseSEM v2.3.0 (Lefcheck 2016). This model independently evaluated fungal and bacterial abundances, as well as F:B ratio, based on our a priori SEM (Supplementary Fig. S1). Owing to the high variability in dependent variables, multicollinearity in independent variables and the fairly small sample size of our dataset, we followed a recommended model selection approach to reduce the likelihood of false negatives (Garrido et al. 2021). To evaluate the performance of candidate SEMs, we reduced our a priori model using a backward stepwise approach, and selected best-fit models based on AICc (Akaike Information Criterion corrected for sample size) and model relative weight (wi), which represents the difference between the AICc of each model and the best model, normalised across all candidate models so that the weights sum to one. We then selected the optimal model based on Δwi ≥55% and ΔAICc ≤2 (Garrido et al. 2021). Information on candidate and best-fit models can be found in Supplementary material Results.
Results
Fire regime affected bacterial and fungal abundance, but the pattern was not clearly related to fire frequency (Fig. 3a, b). Rather, we found moderate evidence that fungal abundance in the E2 treatment was lower than in the L2 treatment (Fig. 3b), demonstrating a seasonal effect on microbial abundance. Fire treatment had a net positive impact on bacterial abundance. There was moderate evidence that treatments E1 (z = 2.02; P = 0.043) and L2 (z = 2.13; P = 0.034) had higher bacterial abundance compared with the unburnt treatment (Fig. 3a). A pairwise comparison revealed strong to moderate evidence that the bacterial abundance in the E2 treatment was lower than in the E1, L2 and E5 treatments (Fig. 3a). By contrast, the fire treatments generally had a neutral effect on fungal abundance (Fig. 3b). However, there was also moderate evidence that the E2 burns negatively impacted fungal abundance (z = −2.43; P = 0.015). There was no evidence that fire influenced F:B ratio (Fig. 3c) or SOC (Fig. 3d). TSF did not influence microbial abundance, SOC or TN, but we found moderate evidence that it influenced F:B ratio and strong evidence of an influence on C:N ratio (Supplementary Results).
Impact of fire treatments on soil properties of an Australian tropical savanna: (a) bacterial gene abundance (millions), (b) fungal gene abundance (millions), (c) fungal-to-bacterial ratio, (d) soil organic carbon (%). Left panels show raw observed values per treatment (n [E1, L2, E2, E3] = 18; n [E5, U] = 12); boxes show s.e. around marginal means with 95% confidence intervals (CIs). The right panels represent effect size means compared with the unburnt treatment; bars show 95% CIs; brackets show statistical differences based on Tukey-adjusted P-values. Model intercepts with 95% CIs are as follows: (a) = 4.6 million ± 1.2 million, (b) = 6.3 million ± 1.5 million, (c) = 1.4 ± 0.41, (d) = 1.54 ± 0.31. Abbreviations: U, unburnt treatment; E, early season burn; L, late season burn; numbers represent burn frequency in years.
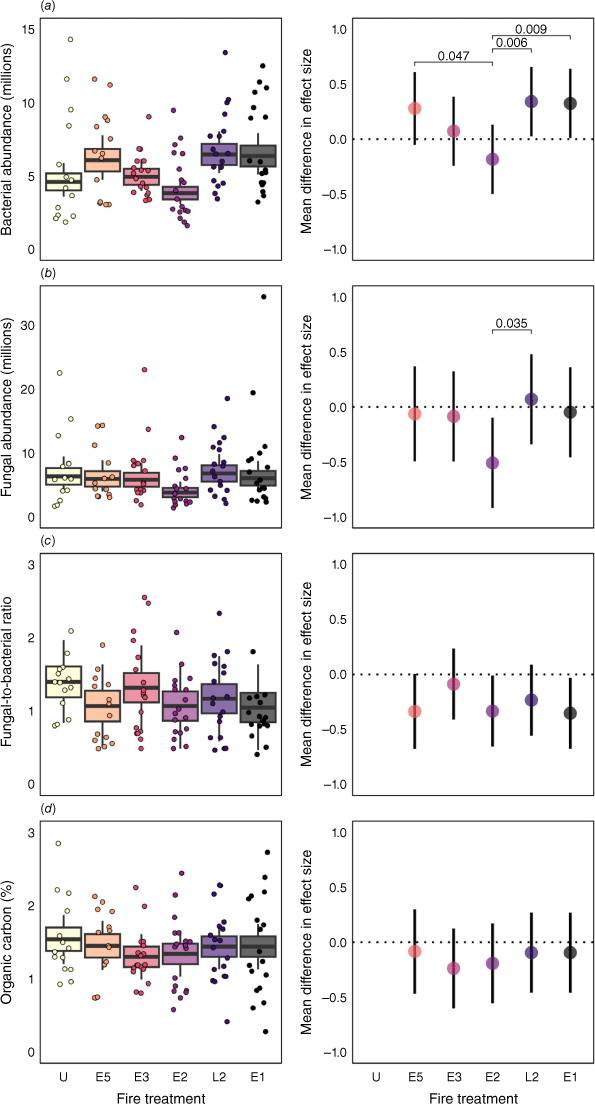
The structural equation model (Fig. 4a) showed that, compared with the unburnt plots, there was a significant effect of fire treatment on ancient grass cover, modern grass cover, leaf litter cover and the C:N ratio. There was a muted effect of total grass cover on microbial abundance (Supplementary Fig. S2). However, when grasses were analysed paleoecologically (ancient and modern), we found markedly different responses of the two groups to fire (Fig. 4). Specifically, ancient grass cover declined with increasing fire frequency, with the least cover in the E1 regime (Fig. 4b). In contrast, modern grass cover increased with fire frequency, with the greatest cover in the L2 regime (Fig. 4c). Litter cover (Fig. 4d) and C:N ratio (Fig. 4e) also declined with more frequent burning; overall, however, the C:N ratio was lowest in the E5 regime.
Structural equation model illustrating the impact of fire on the soil properties of an Australian tropical savanna: (a) the best-fit structural equation model displaying mean effect sizes (ß) of significant variables, with positive effects shown in blue and negative effects in red. Pairwise comparison of the effect size means (points) and Tukey adjusted P-values (brackets) for (b) ancient grasses, (c) modern grasses, (d) leaf litter and (e) carbon-to-nitrogen ratio. Bars represent 95% CIs. Model intercepts with 95% CIs are as follows: (b) = 22.00 ± 7.61 and (c) = 4.55 ± 0.16. Abbreviations: U, unburnt treatment; E, early season burn; L, late season burn; numbers represent burn frequency in years.
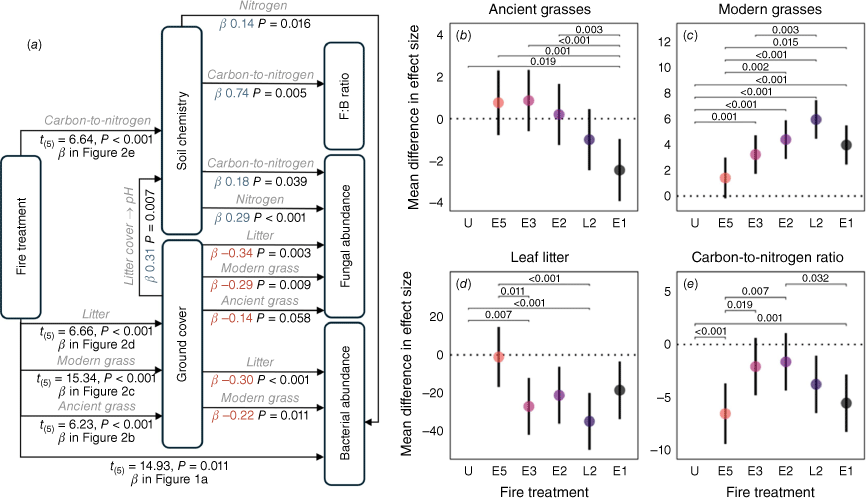
Of all the ground cover and soil physicochemical interactions, there was strong evidence that litter cover increased pH. Grass and litter cover reduced microbial abundance overall. There was only weak evidence that ancient grass cover reduced fungal abundance, but moderate evidence that modern grasses reduced bacterial abundance and strong evidence for reducing fungal abundance. Litter cover also reduced both bacterial and fungal abundance, with strong evidence for both and a greater effect size than for modern grasses.
Fungal abundance was increased in the presence of higher TN, with strong evidence, but there was also moderate evidence that fungal abundance increased with higher C:N ratios. There was moderate evidence that bacterial abundance was greater with higher TN. The C:N ratio had a strong positive effect on the F:B ratio. Mean C:N ratio per regime ranged from 15.49 (E5) to 22 (U), and mean pH ranged from 4.55 (U) to 4.76 (E2) (Supplementary Results).
The effect of fire treatment on fungal abundance was eliminated from the SEM during the model selection process, indicating little to no evidence that fire treatment affects fungal abundance directly; rather, fire treatment likely affects fungal abundance indirectly through soil chemistry and vegetation cover pathways (Supplementary Fig. S2).
Discussion
This study examined the impacts of fire regime on soil microbial abundance, F:B ratio and SOC, as well as exploring soil physicochemical parameters and vegetative ground cover as mediators of the total effect of fire regime on soil microbes. In doing so, we wanted to further understand the interaction between fire regimes, soil microbial communities and carbon cycling dynamics to help inform fire and carbon management of Australian savannas. Overall, our results indicate apparent resilience in microbial community structure and SOC to medium-term (17 years) implementation of different fire regimes. Bacterial and fungal abundances were similarly affected by fire regime and were most responsive to changes in season of fire, and abundance effects were mediated by fire regime impacts to vegetative ground cover.
Fire season affects microbial abundance but no effect of regime on F:B ratio
Microbial abundances under all fire regimes were not significantly different from the unburnt regime, yet for both taxa there was strong evidence of the L2 regime having higher abundance than the E2 regime. The highest microbial abundance under late dry season burning may be a function of both soil moisture availability and seasonal plant productivity, in turn affecting the mortality and recovery of microbiota after fire. The L2 plots are burned at the end of the dry season when microbial activity is likely to be lowest owing to the stress of limited soil moisture (Chen et al. 2002; Manzoni et al. 2012; Cruz-Paredes et al. 2021). Richards et al. (2012) reported a significant reduction in soil respiration in the dry season compared with the wet season at the experimental site, most pronounced in litter patches in unburnt plots.
Desiccation stress is likely to cause selection for resilient microbes that have developed adaptations including spore formation and thickened cell walls through the production of peptidoglycan (Bogati and Walczak 2022). This can result in seasonal community composition turnover, which has been shown in fungal and bacterial communities between wet and dry season in monsoonal grassy shrublands (Umair et al. 2020). Mechanisms utilised by microbiota tolerant of desiccation stress may be similar to survival traits exhibited by pyrophilous (fire-adapted) microbes (Enright et al. 2022). The microbial taxa dominant in the late dry season may thus be particularly tolerant of both desiccation and fire. Further, immediate mortality of soil microbes when burning in the late dry season is less likely owing to the greater sensitivity of microbes to wet heat than dry heat (Mataix-Solera et al. 2009). Finally, the L2 plots are burned late in October, just before the onset of the wet season. The monsoonal rains in tropical systems trigger seasonal plant growth (Hutley and Setterfield 2008; Richards et al. 2012) and the associated increase in root exudates and soil moisture availability shortly after the fires may contribute to faster and more stable recovery of microbiota under this regime.
Fire regime did not significantly affect the F:B ratio after 17 years of fire treatments. This is a novel result compared with the general sensitivity of F:B ratio to fire events globally (Zhang et al. 2015; Muñoz-Rojas et al. 2016; Wang et al. 2016; Zhou et al. 2019; Schill et al. 2023); however, previous studies were of ecosystems that had rarely been exposed to fire whereas Australian savannas have experienced moderately frequent fires since at least 11,000 years (Bird et al. 2024). In studies of fungal responses to fire regime in similarly fire-adapted northern hemisphere savannas, the demonstrated lack of response indicates a legacy of fire-tolerant soil microbial communities adapted to varying fire regimes over long periods of time (Hansen et al. 2019; Vermeire et al. 2021). Further, no change in F:B ratio may indicate that there is little change in the carbon use efficiency of the soil microbial community (Malik et al. 2016). These communities appear to be resilient at a coarse level, but further analysis of taxonomic and metabolic diversity is recommended to thoroughly determine the impact of fire regime on microbial carbon cycling.
Soil microbial abundance differs according to grass paleoecology in Australian savannas
Our model shows that cover abundance of modern grasses increased with fire frequency. Without the palaeoecological lens of grass species diversity, we would have underreported the influence of grass on microbial abundance. Here, the response of modern grasses to frequent fires may have contributed to the overall effect of reducing microbial abundance more than ancient grasses. We also note that the proportion of modern annual grasses increased with fire frequency, which, given their shallow rooting systems, lends to the hypothesis that soil carbon may decline with high fire frequencies (1–2 years) owing to the promotion of modern annual grasses. This contrasts findings from Africa that demonstrate the increased allocation of carbon to belowground resources (roots) by grass species (Coetsee et al. 2023; Zhou et al 2023), where African savannas are rich in endemic perennial C4 grasses (Bond 2016).
Litter had the strongest effect in reducing microbial abundance. This was initially surprising given the assumption that more surface inputs and diversity of litter would increase resources available for soil microbes, therefore leading to higher microbial abundance (Bardgett and Van Der Putten 2014; Liu et al. 2023a). However, in a pyrophilic savanna that appears to foster microbial communities resilient to variations to fire regime in the mid-term, it may be that the native soil microbes are suppressed by increases in vegetation components resulting from either too much or too little fire. For example, leaf litter and modern grasses both reduced microbial abundance, and these components are promoted by reduced burning and increased burning, respectively. Therefore, we suggest that over greater lengths of time, changes to fire regime will affect soil microbial abundance driven by the changes to vegetation.
Soil organic carbon sequestration likely limited in Australian savannas
Soil organic carbon to 10 cm was not affected by 17 years of varied fire regimes. This finding supports previous work by Richards et al. (2011) who used CENTURY modelling and field data to predict that there may be no change in SOC associated with fire regime for up to 100 years. Richards et al. (2011) suggested that SOC sequestration down to 30 cm would be most likely detectable if low-intensity fires occurred every 5 years.
Although changes to fire regime could eventually lead to increases in SOC over long time periods, it is necessary to consider overall system changes and the potential vulnerability of vegetation-associated carbon stocks to fire. Reduced fire frequency can result in greater net ecosystem carbon owing to increases in woody biomass (Levick et al. 2019; Pellegrini et al. 2022). However, accumulation of carbon in vegetation is more vulnerable to loss through fire (Coetsee et al. 2010). In contrast, low-biomass species such as grasses preferentially store carbon belowground (Dass et al. 2018; Coetsee et al. 2023) and therefore less carbon is susceptible to loss by fire. However, not all grasses are equally flammable, and the broad implementation of highly frequent fires may be causing fire–grass feedback loops that increase the current risk of fire by selecting for highly pyrophilic invasive (Rossiter-Rachor et al. 2009) and native species (Elliott et al. 2009), particularly of the Andropogoneae tribe (Bryceson et al. 2023).
In any case, reducing northern Australia – or any landscape – to its carbon capture potential should not overshadow intrinsic biodiversity and cultural values (Burgess et al. 2009; Abreu et al. 2017). In the Americas, Africa and Asia, the conversion of savanna to forest promotes woody encroachment (Venter et al. 2018) and threatens a distinctive biome with endemic suites of species (Abreu et al. 2017). Conversely, woody encroachment in Australia is considered low compared with Africa (Stevens et al. 2017) and frequent fire poses risks to ancient endemic taxa that evolved in less flammable ecosystems (Bryceson et al. 2023). Although fire is important for maintaining the tree–grass structure of savannas, many Australian ecological savanna studies have called for a reduction in burning frequency (Russell-Smith et al. 2012; Woinarski and Legge 2013; Corey et al. 2019; Andersen 2020; Evans and Russell-Smith 2020; Radford et al. 2020; Davies et al. 2023), and although globally, grassy ecosystems appear to have experienced a significant reduction in fires since the year 2000 (Chen et al. 2023), much of the land in northern Australia is still burned every 1–2 years (Russell-Smith et al. 2020). It is crucial to manage ecosystems in ways that both support intrinsic values as well as take commensurable opportunities to increase carbon storage.
Microbial diversity, soil texture and carbon fractions recommended for future research
Future work can build on our findings with next-generation sequencing, such as metabarcoding or metagenomics, to determine the effect of fire regime on soil microbial diversity, which can have strong impacts on microbial carbon cycling (Domeignoz-Horta et al. 2020). Further, although we found no change in total SOC here, different fractions of carbon may have shifted. Specifically, the relatively labile particulate fraction tends to be replaced by more stable mineral-associated and pyrogenic forms of carbon in response to fire, which has implications for the degradability and stability of soil carbon (Pellegrini et al. 2022). For example, pyrogenic carbon (Haig et al. 2024) is particularly resistant to microbial consumption and can further influence carbon cycling by altering microbial community structure and suppressing methane (CH₄) production (Xin et al. 2023). Finally, even with a moderate increase in SOC, the opportunity for SOC sequestration across much of northern Australia may be limited owing to the prevalence of coarse-textured, sandy soils with a low carbon saturation threshold and reduced surface area for the adsorption of soil organic matter (Six et al. 2002; Cotrufo et al. 2019). However, at time of writing, no such comprehensive analysis of Australian soil texture or mineralogy was found and it is recommended as a direction for future work.
Conclusions
This study was a preliminary exploration into the impacts of fire regime on soil microbes, soil organic carbon and vegetative ground cover to aid fire management in Northern Australian tropical savannas. Our results demonstrate resilience in SOC to medium-term (17 years) implementation of different fire regimes. SOC in Australian savannas is slow to change and is unlikely to offer serious potential as a carbon sink, especially when weighing up the resulting risks to biodiversity and cultural values of this unique biome. Bacterial and fungal abundance were most responsive to changes in season of fire, indicating that this factor is an important influence on pedospheric carbon dynamics in Australian savannas. Further, the effects of fire on soil microbes were directed by changes in vegetative ground cover. The influence of total grasses vs paleoecologically classified grass groups highlights the importance of both vegetation and their evolutionary history to belowground microbial carbon cycling. The indirect impacts of fire regime through vegetation changes on soil microbes will be a necessary component of future analysis of fire and soil microbial dynamics.
Data availability
The statistical analyses used in this study are fully reproducible and accessible at: https://github.com/Applied-and-Environmental-Microbiology/burning-for-biodiversity.
Acknowledgements
We acknowledge that our work was conducted on the unceded traditional lands of the Kungarakany, Larrakia and Wurrundjeri-Woi Wurrung peoples. M. H. B., L. F. and S. R. B. were supported by the National University Research Training Scheme and Research Training Program Stipend Scholarships. Thank you to Merinda Day-Smith for collecting the soil samples and to Anya Shindler, Jian Jin, Josh Vido, Jayson Rose, Elise Henderson, Chunya Zhu, Courtney Taylor and Caixan Tang for their help with laboratory work and statistical analysis.
Author contributions
J. L. W. and J. W. M. designed the study, M. H. B. conducted the lab experiments with help from G. J. C., S. R. B. collected the vegetation data, L. F. and M. H. B. conducted the statistical analyses and M. H. B. prepared the manuscript. All authors contributed to the editing and writing of the manuscript.
References
Abreu RCR, Hoffmann WA, Vasconcelos HL, Pilon NA, Rossatto DR, Durigan G (2017) The biodiversity cost of carbon sequestration in tropical savanna. Science Advances 3, e1701284.
| Crossref | Google Scholar | PubMed |
Andersen AN (2020) Faunal responses to fire in Australian tropical savannas: insights from field experiments and their lessons for conservation management. Diversity and Distributions 27, 828-843.
| Crossref | Google Scholar |
Bailey VL, Smith JL, Bolton H (2002) Fungal-to-bacterial ratios in soils investigated for enhanced C sequestration. Soil Biology & Biochemistry 34, 997-1007.
| Crossref | Google Scholar |
Bardgett RD, Van Der Putten WH (2014) Belowground biodiversity and ecosystem functioning. Nature 515, 505-511.
| Crossref | Google Scholar | PubMed |
Bastin J-F, Finegold Y, Garcia C, Mollicone D, Rezende M, Routh D, Zohner CM, Crowther TW (2019) The global tree restoration potential. Science 365, 76-79.
| Crossref | Google Scholar | PubMed |
Bates D, Mächler M, Bolker B, Walker S (2015) Fitting linear mixed-effects models using lme4. Journal of Statistical Software 67, 1-48.
| Crossref | Google Scholar |
Bird MI, Brand M, Comley R, Fu X, Hadeen X, Jacobs Z, Rowe C, Wurster CM, Zwart C, Bradshaw CJA (2024) Late Pleistocene emergence of an anthropogenic fire regime in Australia’s tropical savannahs. Nature Geoscience 17, 233-240.
| Crossref | Google Scholar |
Bogati K, Walczak M (2022) The impact of drought stress on soil microbial community, enzyme activities and plants. Agronomy 12, 189.
| Crossref | Google Scholar |
Bond WJ (2016) Ancient grasslands at risk. Science 351, 120-122.
| Crossref | Google Scholar | PubMed |
Bond WJ, Stevens N, Midgley GF, Lehmann CER (2019) The trouble with trees: afforestation plans for Africa. Trends in Ecology and Evolution 34, 963-965.
| Crossref | Google Scholar | PubMed |
Bowman DMJS, Williamson GJ, Johnston FH, Bowman CJW, Murphy BP, Roos CI, Trauernicht C, Rostron J, Prior LD (2022) Population collapse of a Gondwanan conifer follows the loss of Indigenous fire regimes in a northern Australian savanna. Scientific Reports 12, 9081.
| Crossref | Google Scholar | PubMed |
Bradford MA, Wieder WR, Bonan GB, Fierer N, Raymond PA, Crowther TW (2016) Managing uncertainty in soil carbon feedbacks to climate change. Nature Climate Change 6, 751-758.
| Crossref | Google Scholar |
Brassard F, Pettit MJ, Murphy BP, Andersen AN (2023) Fire influences ant diversity by modifying vegetation structure in an Australian tropical savanna. Ecology 104, e4143.
| Crossref | Google Scholar | PubMed |
Bruun S, Jensen ES, Jensen LS (2008) Microbial mineralization and assimilation of black carbon: dependency on degree of thermal alteration. Organic Geochemistry 39, 839-845.
| Crossref | Google Scholar |
Bryceson SR, Morgan JW (2022) The Australasian grass flora in a global context. Journal of Systematics and Evolution 60, 675-690.
| Crossref | Google Scholar |
Bryceson SR, Hemming KTM, Duncan RP, Morgan JW (2023) The contemporary distribution of grasses in Australia: a process of immigration, dispersal and shifting dominance. Journal of Biogeography 50, 1639-1652.
| Crossref | Google Scholar |
Burgess CP, Johnston FH, Berry HL, McDonnell J, Yibarbuk D, Gunabarra C, Mileran A, Bailie RS (2009) Healthy country, healthy people: the relationship between Indigenous health status and ‘caring for country’. Medical Journal of Australia 190, 567-572.
| Crossref | Google Scholar | PubMed |
Carvalho RL, Weir T, Vasconcelos HL, Andersen AN (2020) Dung beetles of an Australian tropical savanna: species composition, food preferences and responses to experimental fire regimes. Austral Ecology 45, 958-967.
| Crossref | Google Scholar |
Chen X, Eamus D, Hutley LB (2002) Seasonal patterns of soil carbon dioxide efflux from a wet-dry tropical savanna of northern Australia. Australian Journal of Botany 50, 43-51.
| Crossref | Google Scholar |
Chen X, Hutley LB, Eamus D (2003) Carbon balance of a tropical savanna of northern Australia. Oecologia 137, 405-416.
| Crossref | Google Scholar | PubMed |
Chen Y, Hall J, Van Wees D, Andela N, Hantson S, Giglio L, Van Der Werf GR, Morton DC, Randerson JT (2023) Multi-decadal trends and variability in burned area from the fifth version of the Global Fire Emissions Database (GFED5). Earth System Science Data 15, 5227-5259.
| Crossref | Google Scholar |
Coetsee C, Bond WJ, February EC (2010) Frequent fire affects soil nitrogen and carbon in an African savanna by changing woody cover. Oecologia 162, 1027-1034.
| Crossref | Google Scholar | PubMed |
Coetsee C, February EC, Wigley BJ, Kleyn L, Strydom T, Hedin LO, Watson H, Attore F, Pellegrini A (2023) Soil organic carbon is buffered by grass inputs regardless of woody cover or fire frequency in an African savanna. Journal of Ecology 111, 2483-2495.
| Crossref | Google Scholar |
Corey B, Andersen AN, Legge S, Woinarski JCZ, Radford IJ, Perry JJ (2019) Better biodiversity accounting is needed to prevent bioperversity and maximize co-benefits from savanna burning. Conservation Letters 13, e12685.
| Crossref | Google Scholar |
Cotrufo MF, Wallenstein MD, Boot CM, Denef K, Paul E (2013) The Microbial Efficiency-Matrix Stabilization (MEMS) framework integrates plant litter decomposition with soil organic matter stabilization: do labile plant inputs form stable soil organic matter? Global Change Biology 19, 988-995.
| Crossref | Google Scholar | PubMed |
Cotrufo MF, Ranalli MG, Haddix ML, Six J, Lugato E (2019) Soil carbon storage informed by particulate and mineral-associated organic matter. Nature Geoscience 12, 989-994.
| Crossref | Google Scholar |
Cruz-Paredes C, Tájmel D, Rousk J (2021) Can moisture affect temperature dependences of microbial growth and respiration? Soil Biology and Biochemistry 156, 108223.
| Crossref | Google Scholar |
Dalal RC, Allen DE (2008) Greenhouse gas fluxes from natural ecosystems. Australian Journal of Botany 56, 369-407.
| Crossref | Google Scholar |
Dass P, Houlton BZ, Wang Y, Warlind D (2018) Grasslands may be more reliable carbon sinks than forests in California. Environmental Research Letters 13, 074027.
| Crossref | Google Scholar |
Davies HF, Visintin C, Murphy BP, Ritchie EG, Banks SC, Davies ID, Bowman DMJS (2023) Pyrodiversity trade-offs: a simulation study of the effects of fire size and dispersal ability on native mammal populations in northern Australian savannas. Biological Conservation 282, 110077.
| Crossref | Google Scholar |
Denman SE, McSweeney CS (2006) Development of a real-time PCR assay for monitoring anaerobic fungal and cellulolytic bacterial populations within the rumen. FEMS Microbiology Ecology 58, 572-582.
| Crossref | Google Scholar | PubMed |
Domeignoz-Horta LA, Pold G, Liu XJA, Frey SD, Melillo JM, DeAngelis KM (2020) Microbial diversity drives carbon use efficiency in a model soil. Nature Communications 11, 3684.
| Crossref | Google Scholar | PubMed |
Dooley SR, Treseder KK (2012) The effect of fire on microbial biomass: a meta-analysis of field studies. Biogeochemistry 109, 49-61.
| Crossref | Google Scholar |
Edwards EJ, Osborne CP, Strömberg CAE, Smith SA (2010) The origins of C4 grasslands: integrating evolutionary and ecosystem science. Science 328, 587-591.
| Crossref | Google Scholar | PubMed |
Elliott LP, Franklin DC, Bowman DMJS (2009) Frequency and season of fires varies with distance from settlement and grass composition in Eucalyptus miniata savannas of the Darwin region of northern Australia. International Journal of Wildland Fire 18, 61-70.
| Crossref | Google Scholar |
Enright DJ, Frangioso KM, Isobe K, Rizzo DM, Glassman SI (2022) Mega-fire in redwood tanoak forest reduces bacterial and fungal richness and selects for pyrophilous taxa that are phylogenetically conserved. Molecular Ecology 31, 2475-2493.
| Crossref | Google Scholar | PubMed |
Estes JA, Vermeij GJ (2022) History’s legacy: why future progress in ecology demands a view of the past. Ecology 103, e3788.
| Crossref | Google Scholar | PubMed |
Evans J, Russell-Smith J (2020) Delivering effective savanna fire management for defined biodiversity conservation outcomes: an Arnhem Land case study. International Journal of Wildland Fire 29, 386-400.
| Crossref | Google Scholar |
Fierer N, Walsh CM (2023) Can we manipulate the soil microbiome to promote carbon sequestration in croplands? PLoS Biology 21, e3002207.
| Crossref | Google Scholar | PubMed |
Fierer N, Jackson JA, Vilgalys R, Jackson RB (2005) Assessment of soil microbial community structure by use of taxon-specific quantitative PCR assays. Applied and Environmental Microbiology 71, 4117-4120.
| Crossref | Google Scholar | PubMed |
Gardes M, Bruns TD (1993) ITS primers with enhanced specificity for basidiomycetes-application to the identification of mycorrhizae and rusts. Molecular Ecology 2, 113-118.
| Crossref | Google Scholar | PubMed |
Garrido M, Hansen SK, Yaari R, Hawlena H (2021) A model selection approach to structural equation modelling: a critical evaluation and a road map for ecologists. Methods in Ecology and Evolution 13, 42-53.
| Crossref | Google Scholar |
Gougoulias C, Clark JM, Shaw LJ (2014) The role of soil microbes in the global carbon cycle: tracking the below-ground microbial processing of plant-derived carbon for manipulating carbon dynamics in agricultural systems. Journal of the Science of Food and Agriculture 94, 2362-2371.
| Crossref | Google Scholar | PubMed |
Grace J, José JS, Meir P, Miranda HS, Montes RA (2006) Productivity and carbon fluxes of tropical savannas. Journal of Biogeography 33, 387-400.
| Crossref | Google Scholar |
Haig J, Sanderman J, Zwart C, Smith C, Bird MI (2024) Impact of fire return interval on pyrogenic carbon stocks in a tropical savanna, North Queensland, Australia. International Journal of Wildland Fire 33, WF24006.
| Crossref | Google Scholar |
Hansen PM, Semenova-Nelsen TA, Platt WJ, Sikes BA (2019) Recurrent fires do not affect the abundance of soil fungi in a frequently burned pine savanna. Fungal Ecology 42, 100852.
| Crossref | Google Scholar | PubMed |
Hartig F (2022) DHARMa: residual diagnostics for hierarchical (multi-level/mixed) regression models. R package version 0.4.6, Available at https://CRAN.R-project.org/package=DHARMa
Hughes P, McBratney AB, Minasny B, Huang J, Micheli E, Hempel J, Jones E (2018) Comparisons between USDA soil taxonomy and the Australian Soil Classification system II: comparison of order, suborder and great group taxa. Geoderma 322, 48-55.
| Crossref | Google Scholar |
Hutley LB, Setterfield SA (2008) Savanna. In ‘Encyclopedia of ecology’. (Eds SE Jørgensen, BD Fath) pp. 3143–3154. (Academic Press: Oxford) 10.1016/B978-008045405-4.00358-X
Jackson RB, Lajtha K, Crow SE, Hugelius G, Kramer MG, Piñeiro G (2017) The ecology of soil carbon: pools, vulnerabilities, and biotic and abiotic controls. Annual Review of Ecology, Evolution, and Systematics 48, 419-445.
| Crossref | Google Scholar |
Jastrow JD, Amonette JE, Bailey VL (2007) Mechanisms controlling soil carbon turnover and their potential application for enhancing carbon sequestration. Climatic Change 80, 5-23.
| Crossref | Google Scholar |
Jones DL, Nguyen C, Finlay RD (2009) Carbon flow in the rhizosphere: carbon trading at the soil–root interface. Plant and Soil 321, 5-33.
| Crossref | Google Scholar |
Klevenhusen F, Meile L, Kreuzer M, Soliva CR (2011) Effects of monolaurin on ruminal methanogens and selected bacterial species from cattle, as determined with the rumen simulation technique. Anaerobe 17(5), 232-238.
| Crossref | Google Scholar |
Kolb SE, Fermanich KJ, Dornbush ME (2009) Effect of charcoal quantity on microbial biomass and activity in temperate soils. Soil Science Society of America Journal 73, 1173-1181.
| Crossref | Google Scholar |
Lasota J, Błońska E, Babiak T, Piaszczyk W, Stępniewska H, Jankowiak R, Boroń P, Lenart-Boroń A (2021) Effect of charcoal on the properties, enzyme activities and microbial diversity of temperate pine forest soils. Forests 12, 1488.
| Crossref | Google Scholar |
Lefcheck JS (2016) piecewiseSEM: piecewise structural equation modelling in R for ecology, evolution, and systematics. Methods in Ecology and Evolution 7, 573-579.
| Crossref | Google Scholar |
Lenth R (2023) emmeans: estimated marginal means, aka least-squares means. R package version 1.8.5. Available at https://CRAN.R-project.org/package=emmeans
Levick SR, Richards AE, Cook GD, Schatz J, Guderle M, Williams RJ, Subedi P, Trumbore SE, Andersen AN (2019) Rapid response of habitat structure and above-ground carbon storage to altered fire regimes in tropical savanna. Biogeosciences 16, 1493-1503.
| Crossref | Google Scholar |
Liang C (2020) Soil microbial carbon pump: mechanism and appraisal. Soil Ecology Letters 2, 241-254.
| Crossref | Google Scholar |
Liu LY, Xie GJ, Ding J, Liu BF, Xing DF, Ren NQ, Wang Q (2022) Microbial methane emissions from the non-methanogenesis processes: a critical review. Science of The Total Environment 806, 151362.
| Crossref | Google Scholar | PubMed |
Liu S, Plaza C, Ochoa-Hueso R, Trivedi C, Wang J, Trivedi P, Zhou G, Piñeiro J, Martins CSC, Singh BK, Delgado-Baquerizo M (2023a) Litter and soil biodiversity jointly drive ecosystem functions. Global Change Biology 29, 6276-6285.
| Crossref | Google Scholar | PubMed |
Liu W, Zhang Z, Li J, Wen Y, Liu F, Zhang W, Liu H, Ren C, Han X (2023b) Effects of fire on the soil microbial metabolic quotient: a global meta-analysis. Catena 224, 106957.
| Crossref | Google Scholar |
Lüdecke D, Ben-Shachar M, Patil I, Makowski D (2020) Extracting, computing and exploring the parameters of statistical models using R. Journal of Open Source Software 5, 2445.
| Crossref | Google Scholar |
Malik AA, Chowdhury S, Schlager V, Oliver A, Puissant J, Vazquez PGM, Jehmlich N, von Bergen M, Griffiths RI, Gleixner G (2016) Soil fungal:bacterial ratios are linked to altered carbon cycling. Frontiers in Microbiology 7, 1247.
| Crossref | Google Scholar | PubMed |
Manzoni S, Schimel JP, Porporato A (2012) Responses of soil microbial communities to water stress: results from a meta-analysis. Ecology 93, 930-938.
| Crossref | Google Scholar | PubMed |
Marañón-Jiménez S, Radujković D, Verbruggen E, Grau O, Cuntz M, Peñuelas J, Richter A, Schrumpf M, Rebmann C (2021) Shifts in the abundances of saprotrophic and ectomycorrhizal fungi with altered leaf litter inputs. Frontiers in Plant Science 12, 682142.
| Crossref | Google Scholar | PubMed |
Mataix-Solera J, Guerrero C, García-Orenes F, Bárcenas GM, Torres MP (2009) Forest fire effects on soil microbiology. In ‘Fire Effects on soils and restoration strategies’. (Eds R John Raison, PK Khanna, KLS Jacobsen, J Romanya, I Serrasolses) pp. 133–175. (CRC Press) 10.1201/9781439843338-c5
Minasny B, Mcbratney AB, Brough DM, Jacquier D (2011) Models relating soil pH measurements in water and calcium chloride that incorporate electrolyte concentration. European Journal of Soil Science 62, 728-732.
| Crossref | Google Scholar |
Muñoz-Rojas M, Erickson TE, Martini D, Dixon KW, Merritt DJ (2016) Soil physicochemical and microbiological indicators of short, medium and long term post-fire recovery in semi-arid ecosystems. Ecological Indicators 63, 14-22.
| Crossref | Google Scholar |
Pellegrini AFA, Harden J, Georgiou K, Hemes KS, Malhotra A, Nolan CJ, Jackson RB (2022) Fire effects on the persistence of soil organic matter and long-term carbon storage. Nature Geoscience 15, 5-13.
| Crossref | Google Scholar |
Perry JJ, Sinclair M, Wikmunea H, Wolmby S, Martin D, Martin B (2018) The divergence of traditional Aboriginal and contemporary fire management practices on Wik traditional lands, Cape York Peninsula, Northern Australia. Ecological Management and Restoration 19, 24-31.
| Crossref | Google Scholar |
Pingree MRA, Kobziar LN (2019) The myth of the biological threshold: A review of biological responses to soil heating associated with wildland fire. Forest Ecology and Management 432, 1022-1029.
| Crossref | Google Scholar |
Pressler Y, Moore JC, Cotrufo MF (2019) Belowground community responses to fire: meta-analysis reveals contrasting responses of soil microorganisms and mesofauna. Oikos 128, 309-327.
| Crossref | Google Scholar |
Radford IJ, Woolley L-A, Corey B, Vigilante T, Hatherley E, Fairman R, Carnes K, Start AN, Wunambal Gaambera Aboriginal Corporation (2020) Prescribed burning benefits threatened mammals in northern Australia. Biodiversity and Conservation 29, 2985-3007.
| Crossref | Google Scholar |
Ramankutty N, Foley JA (1999) Estimating historical changes in global land cover: croplands from 1700 to 1992. Global Biogeochemical Cycles 13, 997-1027.
| Crossref | Google Scholar |
Richards AE, Cook GD, Lynch BT (2011) Optimal fire regimes for soil carbon storage in tropical savannas of northern Australia. Ecosystems 14, 503-518.
| Crossref | Google Scholar |
Richards AE, Dathe J, Cook GD (2012) Fire interacts with season to influence soil respiration in tropical savannas. Soil Biology and Biochemistry 53, 90-98.
| Crossref | Google Scholar |
Rossiter-Rachor NA, Setterfield SA, Douglas MM, Hutley LB, Cook GD, Schmidt S (2009) Invasive Andropogon gayanus (gamba grass) is an ecosystem transformer of nitrogen relations in Australian savanna. Ecological Applications 19, 1546-1560.
| Crossref | Google Scholar | PubMed |
Russell-Smith J, Edwards AC, Price OF (2012) Simplifying the savanna: the trajectory of fire-sensitive vegetation mosaics in northern Australia. Journal of Biogeography 39, 1303-1317.
| Crossref | Google Scholar |
Russell-Smith J, McCaw L, Leavesley A (2020) Adaptive prescribed burning in Australia for the early 21st Century – context, status, challenges. International Journal of Wildland Fire 29, 305-313.
| Crossref | Google Scholar |
Schill ML, Baird R, Brown SP, Veach AM (2023) Wildfire severity alters soil microbial exoenzyme production and fungal abundances in the Southern Appalachian Mountains. Pedosphere 34, 865-878.
| Crossref | Google Scholar |
Schneider MPW, Lehmann J, Schmidt MWI (2011) Charcoal quality does not change over a century in a tropical agro-ecosystem. Soil Biology and Biochemistry 43, 1992-1994.
| Crossref | Google Scholar |
Six J, Conant RT, Paul EA, Paustian K (2002) Stabilization mechanisms of soil organic matter: implications for C-saturation of soils. Plant and Soil 241, 155-176.
| Crossref | Google Scholar |
Stevens N, Lehmann CER, Murphy BP, Durigan G (2017) Savanna woody encroachment is widespread across three continents. Global Change Biology 23, 235-244.
| Crossref | Google Scholar | PubMed |
Štursová M, Šnajdr J, Cajthaml T, Bárta J, Šantrůčková H, Baldrian P (2014) When the forest dies: the response of forest soil fungi to a bark beetle-induced tree dieback. The ISME Journal 8, 1920-1931.
| Crossref | Google Scholar | PubMed |
Tao F, Huang Y, Hungate BA, Manzoni S, Frey SD, Schmidt MWI, Reichstein M, Carvalhais N, Ciais P, Jiang L, Lehmann J, Wang YP, Houlton BZ, Ahrens B, Mishra U, Hugelius G, Hocking TD, Lu X, Shi Z, Viatkin K, Vargas R, Yigini Y, Omuto C, Malik AA, Peralta G, Cuevas-Corona R, Di Paolo LE, Luotto I, Liao C, Liang YS, Saynes VS, Huang X, Luo Y (2023) Microbial carbon use efficiency promotes global soil carbon storage. Nature 618, 981-985.
| Crossref | Google Scholar | PubMed |
Teixeira J, Souza L, Le Stradic S, Fidelis A (2022) Fire promotes functional plant diversity and modifies soil carbon dynamics in tropical savanna. Science of the Total Environment 812, 152317.
| Crossref | Google Scholar | PubMed |
Umair M, Ningxiao S, Du H, Hui N, Altaf M, Du B, Yin S, Liu C (2020) Bacterial communities are more sensitive to water addition than fungal communities due to higher soil K and Na in a degraded karst ecosystem of southwestern China. Frontiers in Microbiology 11, 562546.
| Crossref | Google Scholar | PubMed |
Uroz S, Calvaruso C, Turpault MP, Frey-Klett P (2009) Mineral weathering by bacteria: ecology, actors and mechanisms. Trends Microbiol 17, 378-387.
| Crossref | Google Scholar | PubMed |
Van Der Heijden MGA, Bardgett RD, Van Straalen NM (2008) The unseen majority: soil microbes as drivers of plant diversity and productivity in terrestrial ecosystems. Ecology Letters 11, 296-310.
| Crossref | Google Scholar | PubMed |
Van Langevelde F, Van De Vijver C, Kumar L, Van De Koppel J, De Ridder N, Van Andel J, Skidmore AK, Hearne JW, Stroosnijder L, Bond WJ, Prins HHT, Rietkerk M (2003) Effects of fire and herbivory on the stability of savanna ecosystems. Ecology 84, 337-350.
| Crossref | Google Scholar |
Venter ZS, Cramer MD, Hawkins HJ (2018) Drivers of woody plant encroachment over Africa. Nature Communications 9, 2272.
| Crossref | Google Scholar | PubMed |
Vermeire ML, Thoresen J, Lennard K, Vikram S, Kirkman K, Swemmer AM, Te Beest M, Siebert F, Gordijn P, Venter Z, Brunel C, Wolfaard G, Krumins JA, Cramer MD, Hawkins HJ (2021) Fire and herbivory drive fungal and bacterial communities through distinct above- and belowground mechanisms. Science of the Total Environment 785, 147189.
| Crossref | Google Scholar | PubMed |
Vilgalys R, Hester M (1990) Rapid genetic identification and mapping of enzymatically amplified ribosomal DNA from several Cryptococcus species. Journal of Bacteriology 172, 4238-4246.
| Crossref | Google Scholar | PubMed |
Wang C, Wang G, Wang Y, Rafique R, Ma L, Hu L, Luo Y (2016) Fire alters vegetation and soil microbial community in Alpine meadow. Land Degradation and Development 27, 1379-1390.
| Crossref | Google Scholar |
Woinarski JCZ, Legge S (2013) The impacts of fire on birds in Australia’s tropical savannas. Emu - Austral Ornithology 113, 319-352.
| Crossref | Google Scholar |
Wolfe BE, Richard F, Cross HB, Pringle A (2010) Distribution and abundance of the introduced ectomycorrhizal fungus Amanita phalloides in North America. New Phytologist 185(3), 803-816.
| Crossref | Google Scholar |
Xin D, Li W, Choi J, Yu YH, Chiu PC (2023) Pyrogenic black carbon suppresses microbial methane production by serving as a terminal electron acceptor. Environmental Science and Technology 57, 20605-20614.
| Crossref | Google Scholar | PubMed |
Yibarbuk D, Whitehead PJ, Russell-Smith J, Jackson D, Godjuwa C, Fisher A, Cooke P, Choquenot D, Bowman DMJS (2001) Fire ecology and Aboriginal land management in central Arnhem Land, northern Australia: a tradition of ecosystem management. Journal of Biogeography 28, 325-343.
| Crossref | Google Scholar |
Zhang L, Liu L, Pan K, Li W, Wang Y, Deng M, Xia J, Yang X (2015) Post-wildfire soil and plant foliar nutrient ratios and soil fungi: bacterial ratios in alpine meadows on the southeastern Qinghai–Tibet Plateau. International Journal of Wildland Fire 24, 933-939.
| Crossref | Google Scholar |
Zhou X, Sun H, Pumpanen J, Sietiö O-M, Heinonsalo J, Köster K, Berninger F (2019) The impact of wildfire on microbial C:N:P stoichiometry and the fungal-to-bacterial ratio in permafrost soil. Biogeochemistry 142, 1-17.
| Crossref | Google Scholar |
Zhou Y, Bomfim B, Bond WJ, Boutton TW, Case MF, Coetsee C, Davies AB, February EC, Gray EF, Silva LCR, Wright JL, Staver AC (2023) Soil carbon in tropical savannas mostly derived from grasses. Nature Geoscience 16, 710-716.
| Crossref | Google Scholar |