Expanding our understanding of nitrogen dynamics after fire: how severe fire and aridity reduce ecosystem nitrogen retention
Maxwell Kay Strain


A
Abstract
Fires release large pulses of nitrogen (N), which can be taken up by recovering plants and microbes or exported to streams where it can threaten water quality.
The amount of N exported depends on the balance between N mineralisation and rates of N uptake after fire. Burn severity and soil moisture interact to drive these rates, but their effects can be difficult to predict.
To understand how soil moisture and burn severity influence post-fire N cycling and retention in a dryland watershed, we quantified changes in plant biomass, plant N content, soil microbial biomass, inorganic N pools, and net N mineralisation for 2 years after fire. We compared sites that were unburned with those that burned at moderate or high severity, capturing variation in soil moisture within each severity category.
Severe fire limited N uptake by plants. Dry conditions after fire limited both plant and microbial N uptake.
When fire is severe or when soils are relatively dry after fire, recovering plants and microbes are less likely to take up post-fire N and therefore, N in these sites is more susceptible to export.
Keywords: burn severity, ecosystem processes, post-fire impacts, soil biogeochemistry, dryland ecosystems, nitrogen cycling, water quality, N saturation.
Introduction
In drylands, post-fire nitrogen (N) that is lost to streams can become a pollutant, and as such, pose a major threat to water quality and stream health (Delwiche 2010; Rust et al. 2019; Gustine et al. 2022). The amount of N that is exported from burned slopes to streams depends on the relative balance between processes that make N available and those that take up N (Hanan et al. 2017; Gustine et al. 2022). Fire intensity and post-fire soil moisture are key drivers of these processes, particularly in the first few years after fire (Rhoades et al. 2011; Hanan et al. 2016). While direct measurements of fire intensity are not often available, burn severity – or the degree to which plant biomass, litter, and soil organic matter are combusted (Keeley 2009) – provides a convenient post hoc approach for characterising fire. Burn severity can also correlate with how much N is volatilised or oxidised from aboveground biomass, and the extent to which ammonium-rich ash is deposited on the soil surface (Debano and Conrad 1978; Raison 1979), which can in turn influence post-fire N cycling. Recent modelling research suggests that after severe fire, dry soil conditions can slow plant recovery and increase N export (Hanan et al. 2017). However, there is a lack of empirical research evaluating how burn severity and post-fire soil moisture interact to drive ecosystem N uptake.
The amount of N converted from live biomass and soil organic matter to inorganic N (i.e. N mineralised by fire) tends to increase with fire severity (Glass et al. 2008). However, severe fire can also reduce plant and microbial biomass, thereby slowing rates of post-fire N uptake (Neary et al. 1999). While many plants native to semiarid shrublands of the western United States have long co-existed with fire and have developed traits that allow them to survive or quickly repopulate after low or mixed severity fire (Keeley and Pausas 2022), sufficiently severe fire can leave little or no surviving vegetation on a landscape. This may constrain seed dispersal, plant productivity, and carbon (C) inputs for recovering microbes, despite high N availability (Midgley et al. 2011; Thomson et al. 2011; Adkins et al. 2020). Severe fire can also kill soil microbes, particularly those closest to the surface, where soil heating is the greatest (Hart et al. 2005; Cairney and Bastias 2007). While microbes in deeper layers may survive, sustained recovery of microbial biomass depends on the amount of soil organic C and soil moisture in the years following fire (Dooley and Treseder 2012).
In drylands, water limitation constrains ecosystem productivity by reducing stomatal conductance and photosynthesis in plants (McDowell et al. 2008), by limiting microbial access to substrates, and by slowing microbially-mediated nutrient cycling (Schimel 2018). For example, soil moisture moderates net mineralisation and nitrification rates, and therefore how much N is available to recovering plants to be taken up or exported to streams (Stanford and Epstein 1974; Stark and Firestone 1995). If conditions are dry following fire, plant roots may become limited in their ability to access soil N. The relative balance between N uptake and export after fire can also depend on whether the timing of precipitation corresponds with peak plant growth (Homyak et al. 2014; Ren et al. 2024a, 2024b). For instance, when soils are rewetted following extended dry periods, soil microbes may rapidly mineralise N (Birch 1964), which may become available to plants. However, if plants are not actively growing and taking up N (due to phenology or dry soils), or if storms intense enough that ash is eroded, excess N may instead be flushed to streams (Homyak et al. 2014; Hanan et al. 2017).
Projecting the extent to which inorganic N deposited by fire is taken up or exported is further complicated by whether an ecosystem is N limited or N saturated. While many drylands are N limited (Hooper and Johnson 1999), fire releases a large pulse of inorganic N that may exceed demand by the recovering plant and microbial communities. When N availability exceeds the capacity for uptake, N saturation can occur, leading to elevated N export to streams (Fenn et al. 1996). However, even when drylands are N limited, intermittent rainfall can promote loss of N to streams if plants are dormant or growing slowly due to dry soils (Homyak et al. 2014). To account for this phenomenon, Lovett and Goodale (2011) distinguish between kinetic N saturation, which is temporary (e.g. at the onset of snowmelt or during intermittent storms), and capacity N saturation, which is long-term. Kinetic N saturation occurs when potential N uptake is high, but available N is greater than what can be taken up at a given point in time. Capacity N saturation, alternatively, tends to occur when an ecosystem has reached peak biomass, where potential uptake is relatively low. We hypothesise that in N-limited watersheds, kinetic saturation will occur after severe fire, as fire simultaneously increases N availability and slows uptake by plants and microbes. However, it remains unclear how fire severity and soil aridityinteract to influence N cycling and uptake.
While fire severity determines the proportion of vegetation and soil organic matter consumed (and how much N is volatilised or released with ash), severe fire also constrains plant and microbial productivity. Soil moisture can also have opposing effects on N uptake – while moist soils can increase plant and microbial access to N, it can also lead to leaching. Thus, it can be difficult to predict how fire severity and soil moisture interact. To understand how burn severity and soil moisture influence N uptake and retention following fire, we quantified N cycling (e.g. net N mineralisation and net nitrification) and N uptake (e.g. microbial and plant N immobilisation rates) and how they varied with burn severity and post-fire soil moisture in a semiarid shrubland. We focused on the first 2 years post-fire, when the greatest changes in biogeochemical cycling and export occur (Verkaik et al. 2013). This research seeks to disentangle how burn severity and post-fire soil moisture interact to drive N uptake in a semiarid watershed, while also providing empirical data that can be used to evaluate previous findings from modelling studies. Finally, we use our findings to extend the Lovett and Goodale (2011) conceptual model for N saturation to include wildfire, an intrinsic component of N dynamics in drylands.
Methods
Site description
This study was conducted in Lamoille Canyon, a glacially carved feature of the Ruby-Humboldt Mountain range in Eastern Nevada, USA. The Range Two fire burned approximately 37.2 km2 of Lamoille Canyon in October 2018 (Fig. 1). This is a semiarid watershed with mean annual temperatures ranging from 0 to 15°C, and a mean annual precipitation of 0.78 m, 82% of which falls as snow (National Water and Climate Center et al. 2024). In the upper portions of the canyon a seasonal snowpack typically lasts from November to April (National Water and Climate Center et al. 2024). Snowpack in the first year of study (2019) lasted from late November to mid-May 2019. The following winter, snowpack was present from 23 November 2019 to 23 April 2020 (National Water and Climate Center et al. 2024). At our site average historic snow depth (2010–2021) was 1.15 m, and average accumulated precipitation between 2010 and 2021 was 0.78 m. The year the fire occurred (2018), peak snow depth was 1.14 m, and accumulated precipitation was 0.59 m. The first year following fire (2019) was wetter than average, with a peak snow depth of 1.8 m and accumulated precipitation of 1.04 m. This was followed by a drier than average year (2020), where peak snow depth was 0.86 and 0.44 m of accumulated precipitation (National Water and Climate Center et al. 2024).
Field site overview (panel a) and sampling method overview (panels b and c). Panel a: the red polygon represents the 2018 Range Two fire. Dots represent upslope sites that burned severely (orange), moderately (yellow), and unburned sampling locations (green). Each site is associated with three transects that measured plant and soil characteristics, as well as measurements of N cycling and uptake. Panel b: each site included three 9 m transects that comprised three randomly placed 1 m2 vegetation quadrats and nine 10 cm depth soil cores aggregated into one sample (n = 9 per severity category; 27 total). Panel c: Vegetation sampling occurred annually and included cover, biomass, and carbon and nitrogen (C/N) content of each species, which was aggregated to functional groups for analysis. Soil sampling occurred seasonally and included gravimetric water content, microbial biomass and inorganic N pools and cycling rates.
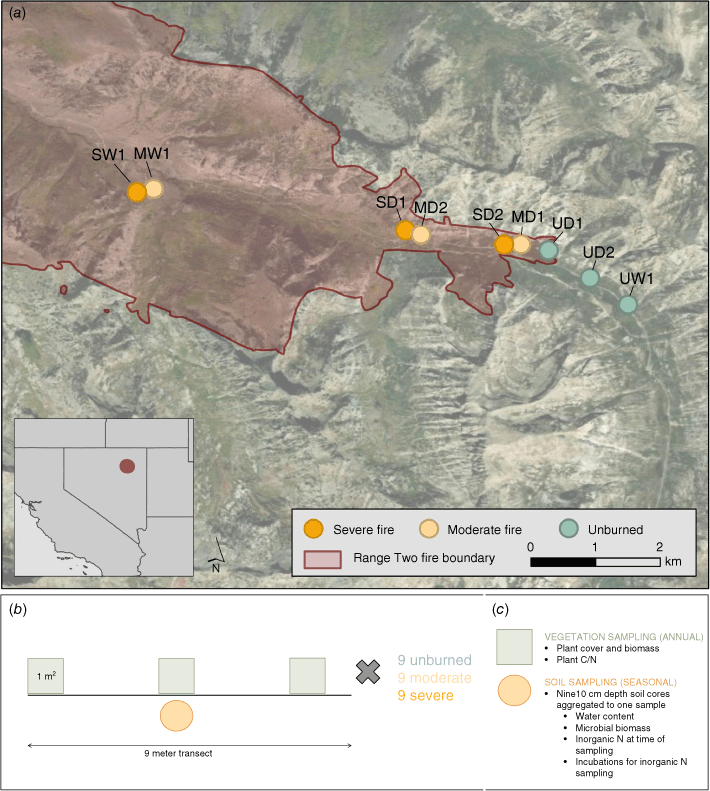
Elevation in the watershed ranges from 2072 to 2590 m with slopes ranging from 15 to 75% (Soil Survey Staff, Natural Resources Conservation Service United States Department of Agriculture 2024). Lower elevations and dry south-facing slopes are dominated by curlleaf mountain mahogany (Cercocarpus ledifolius), while low-lying riparian areas and north-facing slopes contain stands of quaking aspen (Populus tremuloides; Lewis 1971). The dominant overstory plant, curlleaf mountain mahogany, is an N-fixer and can fix up to 13 kg N/ha annually, though N fixation after fire can be minimal if soil N is high (Lepper and Fleschner 1977; Hobbs and Schimel 1984). The soils are Mollisols, with texture of the A horizon as loam or sandy clay loam (Soil Survey Staff, Natural Resources Conservation Service United States Department of Agriculture). Organic horizon depths ranged from 3 to 10 cm deep, and depth depended on the site slope. Rates of N deposition at this site are relatively low, and ranged from 4 to 6 kg N/ha in 2019 and 2020 (NADP Program Office 2024). These rates are relatively low in comparison to some parts of the eastern United States, which can receive as much as 8 kg N/ha annually.
Field sampling
All field sampling was conducted in the first 2 years following fire. Six sites were established within Lamoille Canyon in July 2019. To sample a larger portion of the watershed, three additional sites were established in July 2020. Site selection was based on the following criteria: (1) accessibility, (2), overstory comprised of curlleaf mountain mahogany, and (3) located at least 30 m elevation above the riparian zone, which was dominated by quaking aspen. Unburned sites were established upslope of any burned areas so that N inputs from burned areas did not influence unburned sites. A burn severity classification (unburned, moderate, severe) was given to each site based on total vegetation cover and soil charring during July vegetation sampling at the time of site establishment according to Parsons et al. (2010). We classified burn severity at a given site as severe if curlleaf mountain mahogany had no remaining leaves, the herbaceous and smaller shrub community was fully combusted, and ash was mostly white where present. Moderately burned sites may have fully burned curlleaf mountain mahogany with fine twigs still present, and rarely had leaves present. In moderately burned sites, fine fuels were fully consumed, and smaller shrub stems were charred, with some root crowns present, with a soil surface covered in mostly black or gray ash where ash was present. Burn severity at a given site was also confirmed with differenced normalized burn ratio (dNBR) values calculated prior to site visits in the lab using Landsat 8 imagery (Supplementary Fig. S1) and the MTBS database (USDA Forest Service/U.S. Geological Survey).
Within each site, we established three transects. Transects included one soil sampling location from nine soil cores and three 1 m× 1 m vegetation sampling quadrats (Fig. 1). Soil samples were taken seasonally, and vegetation measurements were taken annually (Fig. 1). Because N-fixers such as curlleaf mountain mahogany and ceanothus (Ceanothus spp.) were present at our field site, soil samples were taken in interspaces, if discernible, and at least 0.5 m away from the nearest shrub in unburned transects. At each vegetation quadrat, we recorded all species present, percent plant cover, and biomass of each plant species greater than 2 g in mass, or less than 1 m tall. Biomass was subsampled for shrubs taller than 1 m by recording total cover of the plant, estimating percent of total biomass taken, and weighing wet weight of the biomass. When samples were dried for 5 days at 65°C in the lab, we estimated the total dry biomass of the original subsampled plant by multiplying the subsampled dry biomass by 100 and dividing by the percent of total biomass in the field. Percent cover of litter and rocks were recorded where present. In summer and fall, each transect was associated with one soil sampling location where nine soil cores were composted (2.5 cm diameter by 10 cm depth in the mineral soil layer). Because the organic soil layers were often entirely combusted in the fire or lost to erosion in our steep study area, we focused on the mineral horizons in both burned and unburned sites. All samples were immediately brought back to the laboratory for analysis. Soil samples collected in the field were kept cool using dry ice.
Laboratory analyses
All soil samples were homogenised and sieved at 4 mm. Sieved and homogenised samples were kept refrigerated for the duration of the analyses detailed below. Bulk density was calculated by dividing the mass of each soil sample by the number of cores taken and the volume of the soil corer. Water content and water holding capacity were determined for each sample by weighing 10 g subsamples of mineral soil and saturating each subsample with deionised water before weighing at full saturation. Subsamples were then dried at 60°C for 72 h before reweighing to calculate the dry weight. Water holding capacity was calculated as the difference between the saturated weight and the dry weight divided by the wet weight. For each sampling period, gravimetric water content was calculated for each soil sample as the difference between the field wet weight of the sample and the oven dry weight divided by the dry weight. This gravimetric water content was averaged for each transect across the period of study to get a time-averaged soil moisture (Table 1).
Location | Severity | Latitude | Longitude | Wet/dry classification by median (for visualisation) | Time-averaged soil moisture | Years sampled | |
---|---|---|---|---|---|---|---|
UW1 | Unburned | 40.6177146 | −115.37339858 | Wet | 0.22 | 2019, 2020 | |
UD1 | Unburned | 40.6491795 | −115.38707748 | Dry | 0.1 | 2019, 2020 | |
UD2 | Unburned | 40.6454961 | −115.3789636 | Dry | 0.03 | 2020 | |
MW1 | Moderate | 40.656856 | −115.440975 | Wet | 0.29 | 2019, 2020 | |
MD1 | Moderate | 40.64960492 | −115.38757561 | Dry | 0.19 | 2019, 2020 | |
MD2 | Moderate | 40.6515087 | −115.40338333 | Dry | 0.01 | 2020 | |
SW1 | Severe | 40.65752483 | −115.44168016 | Wet | 0.36 | 2019, 2020 | |
SD1 | Severe | 40.65210603 | −115.40418331 | Dry | 0.19 | 2019, 2020 | |
SD2 | Severe | 40.6498138 | −115.38916015 | Dry | 0.01 | 2020 |
Wet/dry classification is determined by whether time averaged gravimetric water content was above or below the median. Time-averaged soil moisture was mean water content for soil moisture taken in July 2019, October 2019, and July 2020.
To quantify the N content for each plant species in each vegetation sampling quadrat, plant leaf and stem samples were dried at 65°C for 5 days before being ground to a fine powder using a mill (Thomas Scientific Wiley Mill 4). For each ground sample, a scintillation vial of homogenised sample was dried for 2 days in an oven at 45°C and analysed for and total N using an elemental analyser (Costech 4010). During elemental analysis, every 10th sample included a duplicate sample, followed by one continuing calibration verification (CCV) standard and four continuing calibration blanks (CCB). Samples in a set of 10 were reanalysed if duplicate, CCB, or CCV values were more than 15% outside of expected values. Within a transect, biomass and total N content for each species were aggregated into functional groups (forb, shrub, graminoid; Supplementary Tables S2, S3). Species-level biomass, percent aboveground C and N for each transect and year can be found in supplementary materials (Supplementary Table S2). To calculate aboveground plant N uptake per transect, we calculated total aboveground plant N by species before summing all N in a transect. For each sample, average percent N per gram for each species was multiplied by total grams of dry plant biomass of the species in that transect. We consider changes in plant N per meter across time to reflect rates of plant N uptake.
Inorganic N measurements were conducted immediately after fall and summer field collection on fresh soil samples to determine ammonium and nitrate concentrations at the time of collection. For fall 2019 and summer 2020, field moist and wetted (to 40% water holding capacity) incubations were conducted to evaluate net N mineralisation and net nitrification. These incubations were designed to examine the potential rate of N cycling under field conditions and following rewetting (Brady et al. 2022). Both sets of incubations were conducted for 1 week in 50 mL uncapped centrifuge tubes placed inside a sealed 1 L Mason jar. A small amount (<5 mL) of deionised water was added to the bottom of all incubation jars to maintain humidity during the incubation period. At the end of incubation, inorganic N was extracted by shaking subsamples for 2 h in 2 M KCl solution and then vacuum filtering them through glass microfibre filters (Pall Gelmann Type A/E 0.1 μm). Extracts were then frozen until colorimetric analysis could be performed. Frozen samples were thawed within 6 months of sampling and analysed in triplicate for and using a microplate reader (Tecan MNano+). Each plate of 25 samples included a minimum of two blank samples of ultrapure deionised water, where each blank was run in triplicate. Sample trays were re-run if blanks were greater than 10% outside of the expected value from the standard curve.
Substrate induced respiration (SIR), a proxy for microbial biomass, was estimated on fresh soil samples (West and Sparling 1986; Fierer 2003). From here forward, we will describe SIR as soil microbial biomass. 12 g of yeast per liter in solution was added to each sample so that 20 mg of sterilised yeast solution was delivered for each gram of sample material before shaking for 2 h. For each 10 g soil sample, carbon dioxide (CO2) respiration was measured every 2 h for 6 h using an infrared gas analyser (LI-COR 8100A IRGA). Each sample was run in triplicate on the gas analyser, and for every 25 gas samples, one duplicate, three ambient samples, and three blank samples were run. Samples were shaken at 180 rpm on a reciprocal shaker in between measurements to ensure homogeneity of the soil slurry and gas samples. Changes in CO2 concentration in the headspace across the sampling period reflects microbial carbon in the soil. To avoid introducing errors via upscaling, soil results are reported gravimetrically (μg per gram dry soil) as opposed to grams per meter.
Statistical analyses
All analyses were performed using generalised linear models. Burn severity was used as a categorical variable (unburned, moderate, severe) for all tests. Replication for all analyses was at the transect level (Fig. 1), where one mineral soil sample is associated with three 1 m2 vegetation quadrats. Site was included as a random effect for all models, except one: inorganic N at time of sampling. For this model, variation due to site did not explain any variation, so we removed site as a random effect. When analyses included multiple years of data, year was included as a fixed effect. To assess whether normal distributions were an appropriate fit for modelling response variables, Shapiro–Wilk tests were used. For like model comparison, we used the Akaike Information Criteria (AIC). Analyses were performed in R (R Core Team 2021, R version 4.1.2).
We were interested in how burn severity and soil moisture influenced plant biomass (dry g biomass per m2). A lognormal distribution was used to model dry biomass at the time of sampling. We used burn severity (categorical) and mean transect soil moisture (numeric) across the period of study as predictor variables. In a separate model, we tested whether change in dry biomass was different between functional groups (e.g. shrubs, grasses, forbs). We tested how the same variables predicted plant species richness (number of plant species per m2) as well as plant N concentrations. We used a normal distribution to model change in plant biomass between functional groups. A lognormal distribution was used for plant biomass, plant species richness, and plant N.
For soil biogeochemical analyses and N mineralisation, we investigated the effects of burn severity and soil moisture on inorganic N at the time of sampling. We used burn severity (categorical) and mean transect soil moisture (numeric) across the period of study to predict inorganic N ( per gram dry soil) at each transect. A lognormal distribution was used to model the inorganic N data. We tested whether net N mineralisation rates (μg N mineralised and nitrified per gram dry soil per day) were predicted by time-averaged soil moisture across years and by categorical burn severity. A normal distribution was used for this model. Finally, we analysed the effects of burn severity and time-averaged soil moisture on microbial biomass (μg C per gram dry soil).
For visualisation purposes, sites were divided into relatively moist and relatively dry categories within each severity class based on the median temporally averaged soil moisture to account for seasonal and longer-term trends in post-fire soil moisture (Table 1). Time-averaged soil moisture is determined by a mean gravimetric soil moisture taken in July 2019, October 2020, and July 2020. Dry sites are defined as a mean gravimetric water content of less than or equal to 0.19 g water per gram dry soil (group mean = 0.10 g water per gram dry soil); wet sites are defined by a time-averaged soil moisture greater than 0.19 g water per gram dry soil (group mean = 0.29 g water per gram dry soil).
Results
Plant biomass and biogeochemistry
Plant biomass was significantly lower in both moderately burned and severely burned transects compared to unburned transects when controlling for year and soil moisture, but this effect was much larger in severely burned transects (Table 2, Supplementary Fig. S2). Plant biomass was positively associated with time-averaged soil moisture (Table 2, Supplementary Fig. S2). We found a significant positive interaction with plant biomass and year in moderately burned transects, but not in severely burned transects (Fig. 2, Table 2). Plant biomass increased especially between years in moderate severity transects (Fig. 2, Table 2). Species richness (number of species per m2) was not different between transects that were unburned and transects that experienced moderate burn severity, but severely burned transects had lower diversity (Table 2, Supplementary Fig. S3). Year since fire and water content did not have significant effects on species diversity (Table 2).
Response | Distribution | Predictor | Effect (s.e.) | P-value | Proportion of variance due to site | |
---|---|---|---|---|---|---|
Plant biomass (dry g per m2) | Lognormal | Moderate burn severity | −1.72 ± 0.700 | 0.03 | 0.299 | |
Plant biomass (g dry per m2) | Lognormal | Severe burn severity | −3.45 ± 0.710 | >0.001 | 0.299 | |
Plant biomass (g dry per m2) | Lognormal | Years since fire | 0.013 ± 0.473 | 0.79 | 0.299 | |
Plant biomass (g dry per m2) | Lognormal | Time-averaged soil moisture (0.1 g water per gram dry soil) | 0.431 ± 0.210 | 0.079 | 0.299 | |
Plant biomass (g dry per m2) | Lognormal | Interaction between year and moderate burn severity | 1.98 ± 0.665 | 0.005 | 0.299 | |
Plant biomass (g dry per m2) | Lognormal | Interaction between year and severe burn severity | 0.961 ± 0.679 | 0.166 | 0.299 | |
Number of species per m2 | Normal | Moderate burn severity | 2.35 ± 1.99 | 0.300 | 0.358 | |
Number of species per m2 | Normal | Severe burn severity | −8.33 ± 1.96 | 0.011 | 0.358 | |
Number of species per m2 | Normal | Time-averaged soil moisture (0.1 g water per gram dry soil) | −1.02 ± 0.898 | 0.318 | 0.358 | |
Number of species per m2 | Normal | Year since fire | 0.679 ± 0.457 | 0.146 | 0.358 | |
Plant N (grams N per m2) | Lognormal | Moderate burn severity | −1.28 ± 0.574 | 0.047 | 0.29 | |
Plant N (grams N per m2) | Lognormal | Severe burn severity | −2.27 ± 0.580 | 0.002 | 0.29 | |
Plant N (grams N per m2) | Lognormal | Year since fire | 0.159 ± 0.039 | 0.686 | 0.29 | |
Plant N (grams N per m2) | Lognormal | Interaction between year and moderate burn severity | 1.70 ± 0.552 | 0.004 | 0.29 | |
Plant N (grams N per m2) | Lognormal | Interaction between year and severe burn severity | 0.584 ± 0.552 | 0.297 | 0.29 |
Change in total plant biomass across years for wet and dry transects (top) and separated by functional group (bottom) for transects sampled in both 2019 and 2020 (n = 18). Box plots showing median, 25th percentile and 75th percentile values for change in grams of dry plant biomass per meter for each water content category (wet/dry) based on time-averaged soil moisture, functional group (grasses, forbs, shrubs), and burn severity (unburned/moderate/severe). The blue dashed line indicates zero change across years.
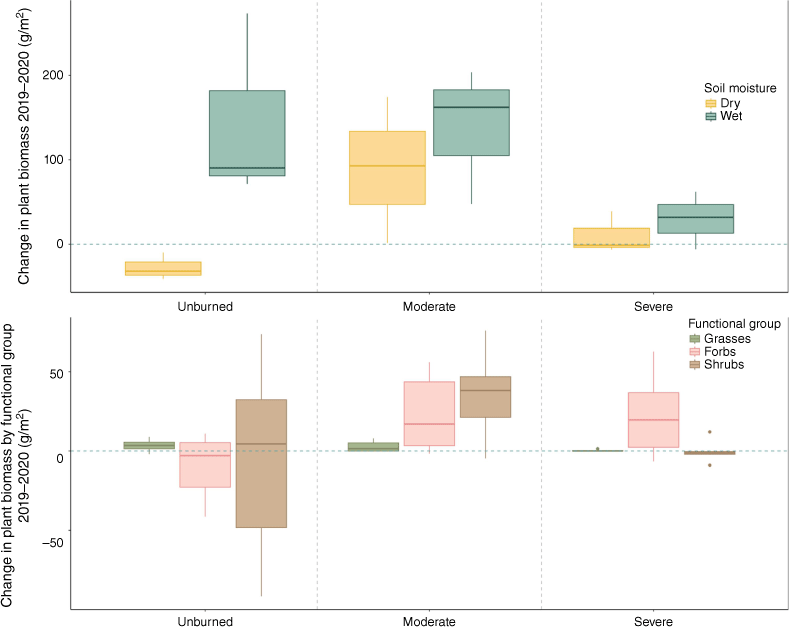
Plant N was lower in both moderately burned and severely burned transects compared to unburned transects, though this effect was much larger and significance was greater in severely burned transects (Table 2, Fig. 3). Plant N was positively associated with time-averaged soil moisture over the course of the study (Table 2, Supplementary Fig. S2). Importantly, there was a significant positive interaction between year and severity in moderately burned transects, but not in severely burned transects, indicating greater increases in plant N across time for moderately burned areas (Fig. 3, Table 2).
Plant and microbial N uptake for the first year following fire. Mean net change in aboveground plant N across years varied by water content and by severity (top). Daily inorganic N mineralisation during the 2019 growing season (bottom; n = 18) based on 1 week field moist mineralisation rates. Aboveground plant N content is calculated by %N in stems and leaves, multiplied by dry mass of the plant. The blue dashed line indicates zero change across years in the top panel. In the bottom panel, the blue horizontal line represents equilibrium (no net immobilisation or mineralisation). Box plots showing median, 25th percentile and 75th percentile values for change in grams plant N for each water content condition (wet/dry) based on time-averaged soil moisture and burn severity (unburned/moderate/severe) for the transects that were sampled both years (n = 18), and for mineral soil incubations for categorised time-averaged soil moisture (wet/dry) and severity (unburned/moderate/severe).
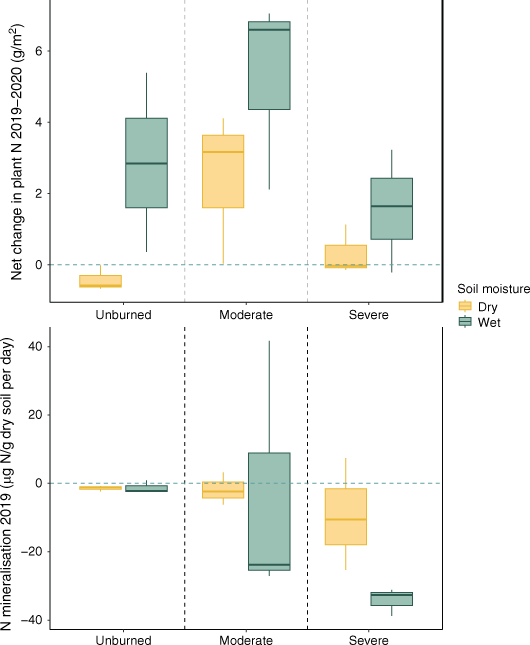
Soil biogeochemical cycling
Inorganic N at time of sampling was positively associated with time-averaged soil moisture when controlling for year and burn severity (Table 3). Compared to unburned soils, inorganic N pools were significantly higher in both moderately burned soils and severely burned soils, though this effect was higher in the severely burned soils (Fig. 4, Table 3). Year had a negative effect on inorganic N pool size.
Response | Distribution | Predictor | Effect (s.e.) | P-value | Proportion of variance due to site | |
---|---|---|---|---|---|---|
Inorganic N at time of sampling (μg N per gram dry soil) | Lognormal | Moderate burn severity | 1.44 ± 0.452 | 0.021 | 0.356 | |
Inorganic N at time of sampling (μg N per gram dry soil) | Lognormal | Severe burn severity | 2.26 ± 0.457 | 0.0033 | 0.356 | |
Inorganic N at time of sampling (μg N per gram dry soil) | Lognormal | Time-averaged soil moisture (0.1 g water per gram dry soil) | 0.363 ± 0.166 | 0.067 | 0.356 | |
Inorganic N at time of sampling (μg N per gram dry soil) | Lognormal | Years since fire | −0.714 ± 0.208 | 0.001 | 0.356 | |
Inorganic N mineralisation in field moist soil (μg N per gram dry soil per day) | Normal | Moderate burn severity | 0.053 ± 4.37 | 0.99 | N/A; see statistical methods | |
Inorganic N mineralisation in field moist soil (μg N per gram dry soil per day) | normal | Severe burn severity | −9.17 ± 4.46 | 0.044 | N/A; see statistical methods | |
Inorganic N mineralisation in field moist soil (μg N per gram dry soil per day) | Normal | Years since fire | 7.25 ± 3.87 | 0.068 | N/A; see statistical methods | |
Estimated microbial biomass (μg C per gram dry soil) | Normal | Moderate burn severity | −1.83 ± 1.56 | 0.316 | 0.120 | |
Estimated microbial biomass (μg C per gram dry soil) | Normal | Severe burn severity | −4.66 ± 1.59 | 0.049 | 0.120 | |
Estimated microbial biomass (μg C per gram dry soil) | Normal | Time-averaged soil moisture (0.1 g water per gram dry soil) | 1.37 ± 0.673 | 0.075 | 0.120 | |
Estimated microbial biomass (μg C per gram dry soil) | Normal | Years since fire | 15.6 ± 3.49 | <0.0001 | 0.120 | |
Estimated microbial biomass (μg C per gram dry soil) | Normal | Time-averaged soil moisture (0.1 g water per gram dry soil) | 2.31 ± 0.642 | 0.001 | 0.120 |
Total inorganic N at time of sampling by time-averaged soil moisture (wet/dry), severity (unburned/moderate/severe) for each year. Box plots showing median, 25th percentile and 75th percentile values for total inorganic N at time of growing season (summer) sampling for 2019 (left, n = 18) and 2020 (right, n = 27). Yellow boxplots represent transects from relatively dry sites, while blue boxplots show transects from relatively wet sites.
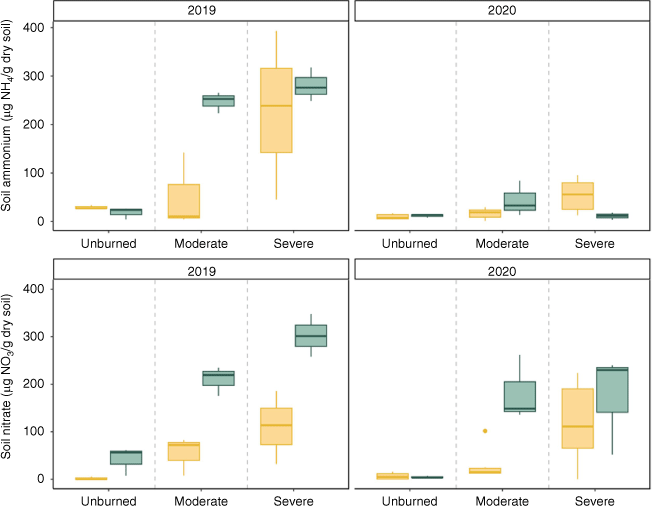
Field moist net N mineralisation rates were positively associated with time-averaged soil moisture (Fig. 3, Table 3). While moderately burned soils did not differ from unburned soils in their net N mineralisation rates, severely burned transects were associated with greater immobilisation (Fig. 3, Table 3). Year since fire had a positive effect on N mineralisation rates (Table 3, Supplementary Table S1).
Estimated microbial biomass (using SIR as a proxy) was positively associated with time since fire and time-averaged soil moisture (Fig. 5, Table 3). Microbial biomass was lower in soils that burned at high severity compared to unburned soils but did not significantly differ between soils moderately burned and unburned soils when controlling for year (Table 3). In the first year following fire, we observed a negative relationship between microbial biomass and burn severity in dry transects and a positive relationship between burn severity and microbial biomass in wet transects.
Box plots showing median, 25th percentile and 75th percentile values for soil microbial biomass (using SIR as a proxy) at time of growing season (summer) sampling for 2019 (left, n = 18) and 2020 (right, n = 27). Colour indicates wet/dry based on time averaged soil moisture for each severity (unburned/moderate/severe).
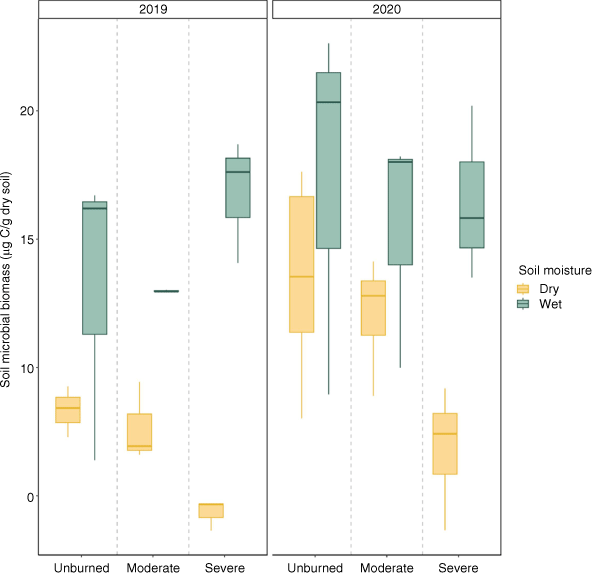
Discussion
The severity and extent of drought and wildfire are increasing in the western United States (Abatzoglou and Williams 2016; Zhang et al. 2021) and globally (Jones et al. 2022). Modelling studies have suggested that dry conditions following fire limit ecosystems’ ability to take up inorganic N deposited by fire, which can increase N export to streams (Hanan et al. 2017). However, empirical studies are needed to corroborate these findings (Hanan et al. 2022). We examined how burn severity and soil moisture influence N cycling and uptake across a fire scar that contained a mixture of moderately and severely burned patches. Our findings show that plant N uptake is reduced after severe fire, and that N uptake by both plants and microbes was muted when relatively dry conditions followed fire (Figs 3, 5).
The role of burn severity
Burn severity is positively associated with mineral soil inorganic N following fire, and negatively associated with how quickly plants and soil microbes regenerate and take up post-fire N. Because burn severity is defined in part by the consumption of plant biomass, it is associated with conversion of biomass to inorganic N (Raison 1979; Kauffman et al. 1993; Keeley 2009). Thus, in lower severity fires, less inorganic N deposited by fire may lead to greater competition for N amongst surviving plants and soil microbes. This can promote tight N cycling (Brady et al. 2022), with little N being exported over the course of the first few growing seasons post-fire (Delwiche 2010).
Burn severity increases inorganic N availability through greater combustion of plant biomass, litter, and soil organic matter and subsequent deposition of ash (Turner et al. 2007; Keeley 2009). Our results corroborate this; we found that burned soils had mean inorganic N concentrations eight times the mean concentration in unburned transects (Fig. 4). When fire was severe, inorganic N pools were larger compared to those in transects that burned at moderate burn severity, but plant communities experienced slower recovery and N uptake (Figs 3, 4). Resprouting shrubs such as chokecherry (Prunus virginiana) resprouted quickly after moderate burn severity and were associated with greater N content than shrubs in unburned transects (Figs 2, 3). In contrast, resprouting shrubs in severely burned transects were suppressed, possibly because the fire was sufficiently severe to kill resprouting plants. Where they survived, higher N content in resprouting shrubs likely contributed to the decrease in inorganic N pools in moderately burned transects over the course of the study. Though in severely burned transects, lower N uptake by recovering shrubs indicates inorganic N may instead be exported (Fig. 4). Driven primarily by limits to plant productivity, areas that burned at high severity had both the highest post-fire N availability (Fig. 4) and the lowest plant N uptake (Fig. 3). This combination may result in greater N loss when dry conditions follow fire (e.g. due to topography or dry years following fire), which can further constrain productivity.
We observed a non-monotonic relationship between burn severity and plant N uptake. This relationship is characterized by high rates of N uptake by resprouting shrubs and forbs in moderately burned areas, and suppressed N uptake in severely burned areas. It is unclear if this pattern would remain if obligate seeders (i.e., shrubs that do not resprout) were the dominant cover type. Modelling studies show N uptake by obligate-seeding species after fire is lower than resprouting shrubs, which can grow quickly to take up post-fire N if not killed (Hanan et al. 2017). Empirical studies where the dominant cover type is not resprouting corroborate this finding: for example biomass and therefore N uptake can decrease with burn severity in sagebrush steppe (Wood et al. 2019). Similar to our findings, studies in sagebrush steppe can show that when burn severity is not severe and where herbaceous species are abundant, recovery can be robust (Weiner et al. 2016). We found that while forbs exhibited equal biomass between severely burned and moderately burned transects (Fig. 2), plant uptake of N was much lower in transects that burned at high severity (Fig. 3). It is possible that in the absence of resprouting shrubs, forbs and perennial grasses may exhibit a similar non-monotonic relationship with burn severity and N uptake as reported here, though further investigation is needed.
The role of post-fire soil moisture
In arid and semi-arid systems, N uptake often depends on water availability (Austin et al. 2004). We observed that dry conditions after fire muted responses in plant and microbial N uptake, though the relationships between burn severity and plant N uptake were preserved (e.g. transects that burned at high severity exhibited lowered plant N uptake; Fig. 3).
In each burn severity category, plants and microbes in dry sites took up less N than their wetter counterparts (Fig. 3). Likely, dry soils disconnected recovering plant roots from available water and N, which are tightly coupled in drylands like our study area (Austin et al. 2004). In addition to limiting productivity of surviving plants, dry soils following fire can also reduce recruitment of obligate seeding dryland shrubs (Urza et al. 2021). In the first year after fire, we observed that both microbial biomass and microbial N uptake increased with burn severity in wet transects (Fig. 3, 5). The inverse was true, however, for microbial biomass when conditions were dry (Fig. 5). While it is possible that soil microbes in dry and severely burned sites lacked access to post fire substrate, it is also possible that despite high N availability (Fig. 4), these microbes lacked C inputs from the plant community (Dooley and Treseder 2012). Dry conditions likely separated microbes from soil substrates due to lack of water in the soil pores, limited plant productivity, and subsequently, limited plant inputs to the soil (Schimel 2018; Adkins et al. 2020).
In contrast, moderate burn severity and relatively moist soils likely created a soil environment in which N was rapidly taken up by plants and soil microbes due to hydrologic connectivity between plant roots and fire-deposited N (Figs 3, 5). These relationships may also have been influenced by pre-fire soil moisture, which in turn can influence plant productivity and contributions to soil organic matter (Plaza et al. 2018). Furthermore, some plants present at our sites such as curlleaf mountain mahogany and ceanothus can fix N, and may have had greater cover in wetter sites before fire (Lepper and Fleschner 1977; Klemmedson and Wienhold 1992). These factors could, in part, explain why wetter sites had higher ammonium concentrations immediately after fire compared to their dry counterparts in the same severity category (Fig. 4, Supplementary S1). However, the most common N fixing species in unburned sites, curlleaf mountain mahogany, experienced poor recruitment after fire and likely did not significantly influence post-fire N concentrations. Soil moisture differences in our sites were likely due to topographical differences (e.g. slope and elevation), though seven of our sites shared aspect, and all sites had similar plant community compositions (Supplementary Tables S2, S3). While these small-scale topographical differences led to greater soil moisture and differences in fire recovery, this approach would be strengthened by application to multiple fires, aspects, and plant communities.
Our findings suggest that patterns of N uptake are driven primarily by responses to the plant community, which were suppressed in severely burned areas compared to their unburned and moderately burned counterparts, resulting in less N retention over time (Fig. 3). Under dry conditions, both plants and microbes were suppressed (Fig. 3, 5), even when N was available in the soil (Fig. 4). Overall, trends of N uptake were driven by plant recovery, which represented a larger portion of N uptake. Assuming a 90-day growing season, in dry conditions uptake by plants in moderately burned transects was 2.43 g N per m2, while microbes took up 0.204 g N per m2. In contrast, plants in severely burned transects took up an average of 5.25 g N per m2, while microbes took up 0.387 g N per m2. Increased soil microbial N uptake in moderate burn severity and moist conditions has been empirically observed in soils (Brady et al. 2022) as well as less N export to streams under moderate severity (Delwiche 2010), also implying greater N is retained after moderate severity. One important difference between these studies and our own is that we found plant and microbial productivity increased with soil moisture in all sites, not just burned areas, which likely reflects water limitation of our more arid system. Drylands are observed to be more responsive to changes in soil moisture relative to more mesic systems (Poulter et al. 2014), and we see that this responsiveness in both burned and unburned sites of our study area (Figs 2, 5).
Soil inorganic N concentrations declined with time since fire for all transects (Fig. 4) but where fire was severe and when conditions were dry, decreases in inorganic N were less likely to be associated with increases in plant and microbial biomass (Figs 2, 5). The observed decline with time could be in part due to less N mineralised in 2020, due to relatively drier conditions compared to 2019 (Supplementary Table S1). However, this decline in N concentrations may also be due to increased plant and microbial demand as post-fire recovery occurs. Since we observed less demand by the plant and microbial communities in severely burned transects, it is also possible that decreases in inorganic N availability was due to runoff and leaching. Our study area is characterised by wet winters and dry summers, and our field sites experienced an unusually heavy winter after fire, with 1.04 m of accumulated precipitation (compared to 0.78 m average accumulation). Snowmelt and runoff, along with steep slopes, likely led to winter erosion and loss of N after fire that we were unable to capture. This may explain why our burned sites had little ash cover in the summer following the severe fire. Likely, these losses represent an important portion of the ecosystem’s N budget post-fire.
Fire alters ecosystem N dynamics by depositing inorganic N on the soil surface. However, unlike atmospheric N deposition, wildfire simultaneously limits the ability of plant communities to take up N. In the first season after fire, we found that N concentrations in mineral soils were equivalent to 0.89 kg N/ha in sites that burned at moderate severity, and 3.22 kg/ha in sites that burned at high severity (as opposed to 0.16 kg N/ha in unburned mineral soils). These ash-induced N inputs are comparable to 6–12 months of atmospheric N deposition in the region (i.e. 4–6 kg N/ha; NADP Program Office 2024). We also note that the mineral N concentrations we observed only represent the portion of ash N inputs that made their way into the mineral horizon. Furthermore, while we observed elevated nitrate in wet transects for both years of our study, nitrate concentrations were lower in dry transects. While increases in ammonium may follow fire quickly due to ash deposition, subsequent pulses of nitrate following fire can lag up to 3 years (Covington and Sackett 1992). A longer study may capture more complex temporal dynamics. While we did not quantify ash N inputs directly, N inputs from ash have been found to be substantially higher in shrublands than the concentrations we report here – for example, in chaparral ash has been estimated to contribute 21 kg N/ha following severe fire (Christensen and Muller 1975 as applied in Hanan et al. 2017). These large N inputs are likely to be lost to streams via leaching if severe fire or dry conditions constrain N uptake.
Modelling studies in semi-arid shrublands such as chaparral have also found that dry conditions after fire constrain productivity (Meng et al. 2015) and increase hydrologic export of N when storms occur (Hanan et al. 2017; Ren et al. 2024a). We found that where fire was severe and plant productivity was limited due to mortality and dry conditions, N availability exceeded the capacity for uptake. Conditions such as these can promote N export to streams (Fenn et al. 2008; Gustine et al. 2022).
A hypothesised framework for how burn severity and soil moisture drive N export
Drylands are often N-limited (Hooper and Johnson 1999). Fire deposits a large pulse of inorganic N on the soil surface by converting plant biomass, litter, and soil organic matter into bioavailable N in the form of ash (Gustine et al. 2022). If the amount of inorganic N deposited by fire exceeds the ability of the burned ecosystem to take it up, N saturation may occur. Traditional frameworks to assess N saturation tend to consider longer-term N balance (i.e. across multiple seasons or years) and assume synchronicity between plant growth and N availability (Aber et al. 1989). However, assessing N saturation in drylands can be difficult, as the timing of precipitation and N availability may not coincide with the plant growing season (Lovett and Goodale 2011). This asynchrony between N availability and N uptake in drylands often results in seasonal pulses of N to streams despite longer-term N limitation (Homyak et al. 2014). Following fires (which typically occur during the dry season), pulses that occur with the onset of the rainy season may be exacerbated due to additional N availability through ash deposition (Hanan et al. 2017). When plant uptake is constrained outside of seasonality due to severe fire or drought, the N that is available can exceed the uptake capacity of the ecosystem, and kinetic N-saturation may occur.
We propose an extension to the Lovett and Goodale (2011) conceptual model for N saturation to include fire and the role of fire severity. In our hypothesised framework, N loss after fire is determined in large part by (a) the amount of inorganic N deposited by fire (b) N mineralisation following fire, and (c) rates of plant and microbial N uptake (Fig. 6, Aber 1992). While the amount of N deposited by fire increases with severity, uptake of this N is determined by fire severity and soil moisture. We found that high severity transects experienced lower plant N uptake, and therefore, reduced capacity for N uptake following fire. We hypothesise that there is a burn severity threshold at which plants become limited in their ability to take up N released by fire, leading to a fire induced kinetic N saturation. Because dry conditions further constrain N uptake, even at lower fire severities, we further hypothesise that dry conditions after fire reduce the burn severity required to achieve fire induced kinetic N-saturation (Fig. 6). Fire induced kinetic N-saturation is a major concern for both N loss and ecosystem recovery (Rust et al. 2019). This hypothesis can have important implications for the timing of prescribed fire and post-fire management, as a fire that occurs before a dry growing season may result in longer term N losses than one that occurs before a relatively wetter growing season (Meixner et al. 2006; Delwiche 2010).
When conditions are dry after fire, the point at which ecosystems switch from N limitation to N saturation occurs at lower severities. We expand upon a framework developed by Lovett and Goodale (2011) to understand controls on N retention and export following fire. N released by fire (grey curve) increases with burn severity as fire mineralises N. Driven primarily by plant uptake, potential N uptake is highest after moderate severity fire. N limitation (blue area in panel a, yellow area in panel b) occurs when potential uptake of N is greater than N mineralised by fire. N export (orange area) occurs when N uptake is less than N availability. When conditions are wet after fire (panel a) N uptake is greater than when conditions are dry (panel b). When comparing these two scenarios (panel c), when conditions are dry after fire the point at which N limitation transitions to N export (grey dashed lines) occurs at lower burn severities, leading to less N export after fire. As we did not measure N dynamics in low severity fire, low severity is hypothetical in this framework.
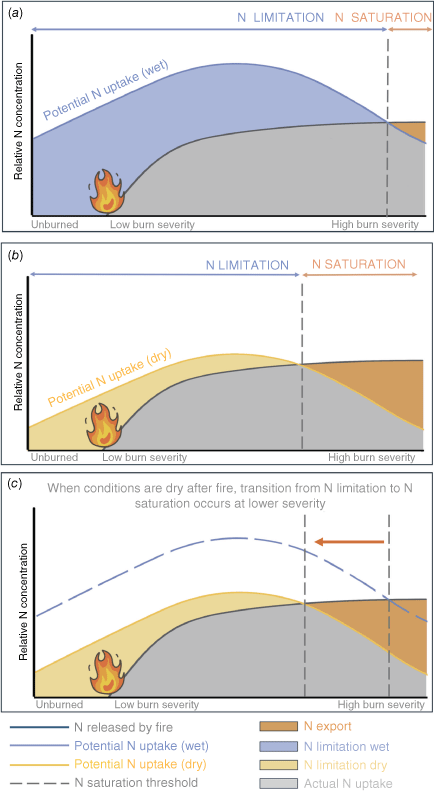
Areas of future research
While we propose an expanded conceptual framework to understand N export following fire in drylands, further research is needed to understand how relationships between fire, drought, and N dynamics can vary. A few key areas for future research are outlined below.
We found that inorganic N in mineral soils increased with burn severity (Fig. 4), and plant N uptake was suppressed after severe fire and when conditions were dry (Fig. 3). It is unclear how the non-monotonic pattern of plant uptake we observed (i.e. where N uptake is greatest in moderate burn severity and suppressed in high burn severity) is influenced by atmospheric N deposition, which provides steady N inputs but does not constrain plant uptake. N deposition is occurring at our study site at rates between 4 and 6 kg N/ha (NADP Program Office 2024). Some studies suggest that atmospheric N deposition may reduce N limitation at lower burn severities, increasing N export (Braakhekke et al. 2017), though the effects of N deposition on dryland N pools can be context dependent (Osborne et al. 2022).
We considered the effects of time-averaged soil moisture on N dynamics. However, climate forecasts in this region predict changes in precipitation variability, (Keyser and LeRoy Westerling 2017; Pendergrass et al. 2017), which can influence biogeochemical cycling and fire severity in complex ways. It is unclear how interannual changes in plant biomass will influence rates of N uptake before and after fire.
Our observed patterns of N uptake in severely burned transects were largely driven by the lack of resprouting shrubs and forb growth (Fig. 2, Supplementary Figs S4 and S5). It is uncertain whether the N uptake patterns we observed will hold true in drylands dominated by shrubs that do not resprout after fire, such as sagebrush steppe. However, in sagebrush, herbaceous species have been observed to exhibit similar responses to fire as observed with resprouting shrubs in this study (e.g. Weiner et al. 2016). Therefore, N uptake where resprouting shrubs are absent may depend on the recovering herbaceous community.
We measured rates of N cycling and uptake over 2 years, though N transformations such as nitrification can sometimes lag one or more years after fire (Covington and Sackett 1992). The timing of post-fire nitrification responses depends, in part, on precipitation patterns (Hanan et al. 2016; Goodridge et al. 2018). While the first 2 years following fire can capture important post-fire dynamics (Verkaik et al. 2013), our hypothesised framework could be strengthened by testing with multiple fires across larger temporal and spatial scales.
If greater N is lost when dry conditions follow fire, it is possible that N losses will result in N-limitation and constrain long-term productivity (Vitousek et al. 2022). While terrestrial N availability is declining globally (Mason et al. 2022), in part due to changes in disturbance regimes such as fire, more research is needed to understand these dynamics regionally.
Conclusions
While inorganic N deposited by fire increased with burn severity (Fig. 4), we observed that severely burned areas exhibited lower plant N uptake compared to transects that experienced moderate burn severity (Fig. 3). This non-monotonic relationship between burn severity and plant N stores likely occurred because there was more surviving vegetation to rapidly take up ash-deposited N in moderately burned sites (Xu et al. 2022). Compared to moderate burn severity, high burn severity was associated with lower plant biomass and plant N uptake - especially in dry conditions (Figs 2, 5). These patterns contrast with severe transects’ association with the highest N availability and greater microbial N uptake immediately after fire (Figs 3, 4). It appears that in these severely burned areas, soil microbes are accessing and immobilising soil N, but the plant community is slow to grow and take up N. Interestingly, we observed that microbial biomass increased with severity in wet transects, but decreased with severity in dry transects (Fig. 5). It is likely that plant mortality in dry and severely burned areas limits C inputs to the soil, which in tandem with direct C loss from soils after fire, leads to slower microbial recovery. Driven primarily by patterns of plant uptake, N uptake is limited in severely burned and dry areas, and therefore N not taken up in these areas is more vulnerable to export via leaching and erosion (Adkins et al. 2020; Gill et al. 2022).
We found that after fire in a semiarid shrubland, severe fire limited plant recovery and therefore plant uptake of post-fire N. Low soil moisture constrained N uptake after fire in both plants and microbes, which may leave post-fire N particularly vulnerable to export during intermittent storms when dry conditions follow fire. Following these relationships, we provide a conceptual framework to suggest that dry conditions are more likely to lead to fire induced kinetic N saturation at lower burn severities. N saturation and subsequent N loss not only threatens water quality and public health, but may result in subsequent N limitation and slow plant recovery in following years. As the extent of wildfire and drought are both increasing in the western United States and globally, extra care must be taken to consider the role of drought and water limitation when assessing post-fire N dynamics.
Data availability
The data that support this study are available in Open Science Forum at DOI:10.17605/OSF.IO/JE7QM.
Declaration of funding
This research was supported in part by the intramural research program of the U.S. Department of Agriculture, National Institute of Food and Agriculture, through the Nevada Agricultural Experiment Station (HATCH, accession number 1018368). The findings and conclusions in this preliminary publication have not been formally disseminated by the U. S. Department of Agriculture and should not be construed to represent any agency determination or policy.
Acknowledgements
We thank the reviewers for their valuable input and detailed feedback during the review process. This article greatly benefited from discussion with and comments from Seren Bagcilar, Ash Cale, and Elena Cox. This research was made possible by funding from the University of Nevada, and land access from Lions Club of Elko, Nevada, and U.S. Forest Service. Thank you to Kevin Shoemaker for statistical advice, to Paul Verburg for instrument access, and to B. T. Harter for GIS assistance. Raymond Lopez and Hannah Hoyos assisted with lab work on this project.
Author contributions
M. Kay Strain conceptualised, investigated, analysed, and wrote original draft. Erin J. Hanan acquired funding, revised, and edited original drafts, and provided project administration. Mary K. Brady helped with conceptualisation and investigation of this project.
References
Abatzoglou JT, Williams AP (2016) Impact of anthropogenic climate change on wildfire across western US forests. Proceedings of the National Academy of Sciences 113, 11770-11775.
| Crossref | Google Scholar | PubMed |
Aber JD (1992) Nitrogen cycling and nitrogen saturation in temperate forest ecosystems. Trends in Ecology & Evolution 7, 220-224.
| Crossref | Google Scholar | PubMed |
Aber JD, Nadelhoffer KJ, Steudler P, Melillo JM (1989) Nitrogen saturation in Northern forest ecosystems. BioScience 39, 378-386.
| Crossref | Google Scholar |
Adkins J, Docherty KM, Gutknecht JLM, Miesel JR (2020) How do soil microbial communities respond to fire in the intermediate term? Investigating direct and indirect effects associated with fire occurrence and burn severity. Science of The Total Environment 745, 140957.
| Crossref | Google Scholar | PubMed |
Austin AT, Yahdjian L, Stark JM, Belnap J, Porporato A, Norton U, Ravetta DA, Schaeffer SM (2004) Water pulses and biogeochemical cycles in arid and semiarid ecosystems. Oecologia 141, 221-235.
| Crossref | Google Scholar | PubMed |
Birch HF (1964) Mineralisation of plant nitrogen following alternate wet and dry conditions. Plant and Soil 20, 43-49.
| Crossref | Google Scholar |
Braakhekke MC, Rebel KT, Dekker SC, Smith B, Beusen AHW, Wassen MJ (2017) Nitrogen leaching from natural ecosystems under global change: a modelling study. Earth System Dynamics 8, 1121-1139.
| Crossref | Google Scholar |
Brady MK, Hanan EJ, Dickinson MB, Miesel JR, Wade L, Greenberg J (2022) How interactions between wildfire and seasonal soil moisture fluxes drive nitrogen cycling in Northern Sierra Nevada forests. International Journal of Wildland Fire 31, 786-798.
| Crossref | Google Scholar |
Cairney JWG, Bastias BA (2007) Influences of fire on forest soil fungal communities. Canadian Journal of Forest Research 37, 207-215.
| Crossref | Google Scholar |
Christensen NL, Muller CH (1975) Effects of fire on factors controlling plant growth in Adenostoma Chaparral. Ecological Monographs 45, 29-55.
| Crossref | Google Scholar |
Covington WW, Sackett SS (1992) Soil mineral nitrogen changes following prescribed burning in ponderosa pine. Forest Ecology and Management 54, 175-191.
| Crossref | Google Scholar |
Debano LF, Conrad CE (1978) The effect of fire on nutrients in a chaparral ecosystem. Ecology 59, 489-497.
| Crossref | Google Scholar |
Delwiche J (2010) After the fire, follow the nitrogen. JFSP Briefs 80, 1-6.
| Google Scholar |
Dooley SR, Treseder KK (2012) The effect of fire on microbial biomass: a meta-analysis of field studies. Biogeochemistry 109, 49-61.
| Crossref | Google Scholar |
Fenn ME, Poth MA, Johnson DW (1996) Evidence for nitrogen saturation in the San Bernardino Mountains in southern California. Forest Ecology and Management 82, 211-230.
| Crossref | Google Scholar |
Fenn ME, Jovan S, Yuan F, Geiser L, Meixner T, Gimeno BS (2008) Empirical and simulated critical loads for nitrogen deposition in California mixed conifer forests. Environmental Pollution 155, 492-511.
| Crossref | Google Scholar | PubMed |
Gill NS, Turner MG, Brown CD, Glassman SI, Haire SL, Hansen WD, Pansing ER, St Clair SB, Tomback DF (2022) Limitations to propagule dispersal will constrain post-fire recovery of plants and fungi in Western coniferous forests. BioScience 72, 347-364.
| Crossref | Google Scholar |
Glass DW, Johnson DW, Blank RR, Miller WW (2008) Factors affecting mineral nitrgen transformations by soil heating: a laboratory-simulated fire study. Soil Science 173, 387-400.
| Crossref | Google Scholar |
Goodridge BM, Hanan EJ, Aguilera R, Wetherley EB, Chen Y-J, D’Antonio CM, Melack JM (2018) Retention of nitrogen following wildfire in a chaparral ecosystem. Ecosystems 21, 1608-1622.
| Crossref | Google Scholar |
Gustine RN, Hanan EJ, Robichaud PR, Elliot WJ (2022) From burned slopes to streams: how wildfire affects nitrogen cycling and retention in forests and fire-prone watersheds. Biogeochemistry 157, 51-68.
| Crossref | Google Scholar |
Hanan EJ, D’Antonio CM, Roberts DA, Schimel JP (2016) Factors regulating nitrogen retention during the early stages of recovery from fire in coastal chaparral ecosystems. Ecosystems 19, 910-926.
| Crossref | Google Scholar |
Hanan EJ, Tague CN, Schimel JP (2017) Nitrogen cycling and export in California chaparral: the role of climate in shaping ecosystem responses to fire. Ecological Monographs 87, 76-90.
| Crossref | Google Scholar |
Hanan EJ, Kennedy MC, Ren J, Johnson MC, Smith AMS (2022) Missing climate feedbacks in fire models: limitations and uncertainties in fuel loadings and the role of decomposition in fine fuel accumulation. Journal of Advances in Modeling Earth Systems 14, e2021MS002818.
| Crossref | Google Scholar |
Hart SC, DeLuca TH, Newman GS, MacKenzie MD, Boyle SI (2005) Post-fire vegetative dynamics as drivers of microbial community structure and function in forest soils. Forest Ecology and Management 220, 166-184.
| Crossref | Google Scholar |
Hobbs NT, Schimel DS (1984) Fire effects on nitrogen mineralization and fixation in mountain shrub and grassland communities. Journal of Range Management 37, 402-405.
| Crossref | Google Scholar |
Homyak PM, Sickman JO, Miller AE, Melack JM, Meixner T, Schimel JP (2014) Assessing nitrogen-saturation in a seasonally dry chaparral watershed: limitations of traditional indicators of N-saturation. Ecosystems 17, 1286-1305.
| Crossref | Google Scholar |
Hooper DU, Johnson L (1999) Nitrogen limitation in dryland ecosystems: responses to geographical and temporal variation in precipitation. Biogeochemistry 46, 247-293.
| Crossref | Google Scholar |
Jones MW, Abatzoglou JT, Veraverbeke S, Andela N, Lasslop G, Forkel M, Smith AJP, Burton C, Betts RA, Van Der Werf GR, Sitch S, Canadell JG, Santín C, Kolden C, Doerr SH, Le Quéré C (2022) Global and regional trends and drivers of fire under climate change. Reviews of Geophysics 60, e2020RG000726.
| Crossref | Google Scholar |
Kauffman JB, Sanford RL, Cummings DL, Salcedo IH, Sampaio EVSB (1993) Biomass and nutrient dynamics associated with slash fires in neotropical dry forests. Ecology 74, 140-151.
| Crossref | Google Scholar |
Keeley JE (2009) Fire intensity, fire severity and burn severity: a brief review and suggested usage. International Journal of Wildland Fire 18, 116-126.
| Crossref | Google Scholar |
Keeley JE, Pausas JG (2022) Evolutionary ecology of fire. Annual Review of Ecology, Evolution, and Systematics 53, 203-225.
| Crossref | Google Scholar |
Keyser A, LeRoy Westerling A (2017) Climate drives inter-annual variability in probability of high severity fire occurrence in the western United States. Environmental Research Letters 12, 065003.
| Crossref | Google Scholar |
Klemmedson JO, Wienhold BJ (1992) Aspect and species influences on nitrogen and phosphorus accumulation in Arizona chaparral soil‐plant systems. Arid Soil Research and Rehabilitation 6, 105-116.
| Crossref | Google Scholar |
Lepper MG, Fleschner M (1977) Nitrogen fixation by Cercocarpus ledifolius (Rosaceae) in pioneer habitats. Oecologia 27, 333-338.
| Crossref | Google Scholar | PubMed |
Lovett GM, Goodale CL (2011) A new conceptual model of nitrogen saturation based on experimental nitrogen addition to an oak forest. Ecosystems 14, 615-631.
| Crossref | Google Scholar |
Mason RE, Craine JM, Lany NK, Jonard M, Ollinger SV, Groffman PM, Fulweiler RW, Angerer J, Read QD, Reich PB, Templer PH, Elmore AJ (2022) Evidence, causes, and consequences of declining nitrogen availability in terrestrial ecosystems. Science 376, eabh3767.
| Crossref | Google Scholar | PubMed |
McDowell N, Pockman WT, Allen CD, Breshears DD, Cobb N, Kolb T, Plaut J, Sperry J, West A, Williams DG, Yepez EA (2008) Mechanisms of plant survival and mortality during drought: why do some plants survive while others succumb to drought? New Phytologist 178, 719-739.
| Crossref | Google Scholar | PubMed |
Meixner T, Fenn ME, Wohlgemuth P, Oxford M, Riggan P (2006) N saturation symptoms in chaparral catchments are not reversed by prescribed fire. Environmental Science & Technology 40, 2887-2894.
| Crossref | Google Scholar | PubMed |
Meng R, Dennison PE, Huang C, Moritz MA, D’Antonio C (2015) Effects of fire severity and post-fire climate on short-term vegetation recovery of mixed-conifer and red fir forests in the Sierra Nevada Mountains of California. Remote Sensing of Environment 171, 311-325.
| Crossref | Google Scholar |
Midgley JJ, Kruger LM, Skelton R (2011) How do fires kill plants? The hydraulic death hypothesis and Cape Proteaceae “fire-resisters”. South African Journal of Botany 77, 381-386.
| Crossref | Google Scholar |
NADP Program Office (2024) ‘National Atmospheric Deposition Program.’ (Wisconsin State Laboratory of Hygiene: Madison, WI, USA) Available at https://nadp.slh.wisc.edu/
National Water and Climate Center, NRCS, USDA (2024) Air and water database. Available at https://wcc.sc.egov.usda.gov/reportGenerator/
Neary DG, Klopatek CC, DeBano LF, Ffolliott PF (1999) Fire effects on belowground sustainability: a review and synthesis. Forest Ecology and Management 122, 51-71.
| Crossref | Google Scholar |
Osborne BB, Roybal CM, Reibold R, Collier CD, Geiger E, Phillips ML, Weintraub MN, Reed SC (2022) Biogeochemical and ecosystem properties in three adjacent semi‐arid grasslands are resistant to nitrogen deposition but sensitive to edaphic variability. Journal of Ecology 110, 1615-1631.
| Crossref | Google Scholar |
Parsons A, Robichaud P, Lewis S, Napper C (2010) Field Guide for Mapping Post-fire Soil Burn Severity. General Technical Report, RMRS-GTR-243. Available at https://www.fs.usda.gov/rm/pubs/rmrs_gtr243.pdf
Pendergrass AG, Knutti R, Lehner F, Deser C, Sanderson BM (2017) Precipitation variability increases in a warmer climate. Scientific Reports 7, 17966.
| Crossref | Google Scholar | PubMed |
Plaza C, Zaccone C, Sawicka K, Méndez AM, Tarquis A, Gascó G, Heuvelink GBM, Schuur EAG, Maestre FT (2018) Soil resources and element stocks in drylands to face global issues. Scientific Reports 8, 13788.
| Crossref | Google Scholar | PubMed |
Poulter B, Frank D, Ciais P, Myneni RB, Andela N, Bi J, Broquet G, Canadell JG, Chevallier F, Liu YY, Running SW, Sitch S, Van Der Werf GR (2014) Contribution of semi-arid ecosystems to interannual variability of the global carbon cycle. Nature 509, 600-603.
| Crossref | Google Scholar | PubMed |
Raison RJ (1979) Modification of the soil environment by vegetation fires, with particular reference to nitrogen transformations: a review. Plant and Soil 51, 73-108.
| Crossref | Google Scholar |
R Core Team (2021) R: A Language and Environment for Statistical Computing. Available at https://www.R-project.org/
Ren J, Hanan EJ, D’Odorico P, Tague C, Schimel JP, Homyak PM (2024a) Dryland watersheds in flux: how nitrogen deposition and changing precipitation regimes shape nitrogen export. Earth’s Future 12, e2023EF004120.
| Crossref | Google Scholar |
Ren J, Hanan EJ, Greene A, Tague C, Krichels AH, Burke WD, Schimel JP, Homyak PM (2024b) Simulating the role of biogeochemical hotspots in driving nitrogen export from dryland watersheds. Water Resources Research 60, e2023WR036008.
| Crossref | Google Scholar |
Rhoades CC, Entwhistle D, Butler D (2011) The influence of wildfire extent and severity on streamwater chemistry, sediment and temperature following the Hayman Fire, Colorado. International Journal of Wildland Fire 20, 430-442.
| Crossref | Google Scholar |
Rust AJ, Saxe S, McCray J, Rhoades CC, Hogue TS (2019) Evaluating the factors responsible for post-fire water quality response in forests of the western USA. International Journal of Wildland Fire 28, 769-784.
| Crossref | Google Scholar |
Schimel JP (2018) Life in dry soils: effects of drought on soil microbial communities and processes. Annual Review of Ecology, Evolution, and Systematics 49, 409-432.
| Crossref | Google Scholar |
Stanford G, Epstein E (1974) Nitrogen mineralization-water relations in soils. Soil Science Society of America Journal 38, 103-107.
| Crossref | Google Scholar |
Stark JM, Firestone MK (1995) Mechanisms for soil moisture effects on activity of nitrifying bacteria. Applied and Environmental Microbiology 61, 218-221.
| Crossref | Google Scholar | PubMed |
Thomson FJ, Moles AT, Auld TD, Kingsford RT (2011) Seed dispersal distance is more strongly correlated with plant height than with seed mass: dispersal distance and seed mass. Journal of Ecology 99, 1299-1307.
| Crossref | Google Scholar |
Turner MG, Smithwick EAH, Metzger KL, Tinker DB, Romme WH (2007) Inorganic nitrogen availability after severe stand-replacing fire in the Greater Yellowstone ecosystem. Proceedings of the National Academy of Sciences 104, 4782-4789.
| Crossref | Google Scholar | PubMed |
Urza AK, Weisberg PJ, Board D, Chambers JC, Kitchen SG, Roundy BA (2021) Episodic occurrence of favourable weather constrains recovery of a cold desert shrubland after fire. Journal of Applied Ecology 58, 1776-1789.
| Crossref | Google Scholar |
USDA Forest Service/U.S. Geological Survey (2024) Monitoring Trends in Burn Severity (MTBS) Data Access: Fire Level Geospatial Data. Available at http://mtbs.gov/direct-download [accessed 29 June 2019]
Verkaik I, Rieradevall M, Cooper SD, Melack JM, Dudley TL, Prat N (2013) Fire as a disturbance in mediterranean climate streams. Hydrobiologia 719, 353-382.
| Crossref | Google Scholar |
Vitousek PM, Treseder KK, Howarth RW, Menge DNL (2022) A “toy model” analysis of causes of nitrogen limitation in terrestrial ecosystems. Biogeochemistry 160, 381-394.
| Crossref | Google Scholar |
Weiner NI, Strand EK, Bunting SC, Smith AMS (2016) Duff distribution influences fire severity and post-fire vegetation recovery in sagebrush steppe. Ecosystems 19, 1196-1209.
| Crossref | Google Scholar |
West AW, Sparling GP (1986) Modifications to the substrate-induced respiration method to permit measurement of microbial biomass in soils of differing water contents. Journal of Microbiological Methods 5, 177-189.
| Crossref | Google Scholar |
Wood DJA, Seipel T, Irvine KM, Rew LJ, Stoy PC (2019) Fire and development influences on sagebrush community plant groups across a climate gradient in northern Nevada. Ecosphere 10, e02990.
| Crossref | Google Scholar |
Xu W, Elberling B, Ambus PL (2022) Pyrogenic organic matter as a nitrogen source to microbes and plants following fire in an Arctic heath tundra. Soil Biology and Biochemistry 170, 108699.
| Crossref | Google Scholar |
Zhang F, Biederman JA, Dannenberg MP, Yan D, Reed SC, Smith WK (2021) Five decades of observed daily precipitation reveal longer and more variable drought events across much of the Western United States. Geophysical Research Letters 48, e2020GL092293.
| Crossref | Google Scholar |