Maternal aging reduces female fecundity and alters offspring phenotype in a sex-specific manner
Macarena B. Gonzalez



A
# These authors contributed equally to this paper
Handling Editor: Richard Lea
Abstract
The age of childbearing in women has increased, with more babies born to women over 30 years old than to those in their 20s. However, increasing maternal age is associated with a range of pregnancy and perinatal complications, such as reduced chance of conception, and higher risk of miscarriage or fetal death. Further, epidemiological studies indicate that advanced maternal age is also linked to a higher incidence of metabolic and neurodevelopmental disorders in offspring, such as Type 1 diabetes and autism spectrum disorder (ASD).
Mature female mice recapitulate many of the fertility characteristics seen in older women, such as reduced egg number and quality, providing a robust experimental model. This study examined fertility and offspring phenotypes in female mice at the onset of reproductive aging.
Firstly, fecundity in mice was measured from 3 to 18 months of age. Secondly, reproductive outcomes in aged female mice (12 months old) were compared to those of young females (3 months of age). Growth of the offspring was assessed, as well as metabolism, behaviour, and immune function in adulthood.
Female aging reduced pregnancy rate, litter size and pup survival to weaning. Maternal age did not affect adult offspring immune function; however, female offspring had higher body weights, and male littermates presented dysregulated glucose tolerance and hyperactivity.
Maternal age affects offspring survival and health in a sex-specific manner.
These findings expand our understanding of maternal programming of offspring health, particularly the effects of increased age at pregnancy.
Keywords: developmental programming, fertility, litter size, maternal age, offspring, parental age, reproduction, sexual dimorphism.
Introduction
The age of childbearing has increased in the last several decades so that it is now common, globally, for women to have children in their mid- to late-30s (Fitzpatrick et al. 2017; Shan et al. 2018; Morse 2022; AIHW 2023). As such, it is becoming apparent that advanced reproductive age is detrimental for female fertility and offspring health. Reduced numbers of ovarian follicles and chromosomal aneuploidy in eggs are the primary causes of reduced fecundity in females of advanced reproductive age. Less well understood are the phenotypic changes in resulting offspring that occur with increased maternal age.
With every 5-year increase in maternal age, the risk of Type 1 diabetes in the child increases by an average of 5% (Cardwell et al. 2010). Conversely, the incidence of atopic or Th2 immune conditions in children (such as atopic dermatitis, dietary allergies and asthma), are reduced with increasing maternal age (Svanes et al. 1998; Dioun et al. 2003; Lu et al. 2020). Thus, a hypothesis has emerged that maternal age influences maturation of the offspring immune system, with a bias towards Th1 for the Th1/Th2 immune balance (Bingley et al. 2000). Maternal age has also been linked to a higher incidence of neurodevelopmental disorders in offspring. Children born to women at or over 35 years old had a 30% higher risk of developing autism spectrum disorder (ASD) compared to those born to mothers younger than 29 years old, with this risk increasing with advancing maternal age (Sandin et al. 2012). The association was stronger in studies with a higher proportion of male offspring, suggesting a sex-specific effect.
Aging is a natural, slow and complex biological process leading to a gradual functional decline in several physiological systems. However, aging affects the female reproductive system, particularly the ovary, earlier than other major organs, approximately midway through the lifespan in women. Clinically, women ≥ 35 years old are considered of ‘advanced maternal age’, and experience diminished fecundity (Warburton 2005), due to reduced numbers of ovarian follicles and oocytes, as well as increased incidence of early pregnancy loss. Similarly, female mice at 48–57 weeks old (reproductive equivalent of 38–45 years in women (Dutta and Sengupta 2016) have fewer growing follicles and corpora lutea, and reduced ovulation, compared to younger mice at 6–12 weeks of age (Mara et al. 2020). Egg quality is also reduced in reproductively aged mice (Bertoldo et al. 2020; Dogan et al. 2020), which is linked to abnormal pre-implantation embryo development and a higher risk of fetal loss (Woods et al. 2017; Cimadomo et al. 2018; Bertoldo et al. 2020; Li et al. 2020). These observations establish that female mice are a robust model of human reproductive aging.
The rate of chromosomal defects, such as aneuploidy, increases in women of advanced maternal age, and this is believed to be the underlying cause of the higher rates of early miscarriage and some congenital defects seen in their pregnancies (reviewed in Glick et al. (2021)). Aneuploidy in eggs also increases with ovarian aging in mice, albeit the timing may be strain dependent (Zuccotti et al. 1998; Camlin et al. 2017). Other studies have noted that the decrease in female mouse fecundity seen with age can also occur in the absence of reductions in oocyte stores or number of early implantation sites (Biggers et al. 1962; Finn 1963).
These observations agree with the fact that many of the pregnancy and offspring health complications seen in pregnancies from women of advanced maternal age, such as fetal growth restriction, preterm birth, stillbirth, and perinatal death (reviewed in Lean et al. (2017)) are not due to chromosomal abnormalities. This suggests that aging of the female reproductive system directly affects germinal cell development during oogenesis (pre-fertilisation), as well as embryonic development during pregnancy (post-fertilisation). Maternal age alters oocyte gene expression and biochemical aspects (i.e. mitochondrial dysfunction) that are essential for oocyte quality in both humans (Reyes et al. 2017; Zhang et al. 2020) and mice (Hamatani et al. 2004; Bertoldo et al. 2020). The periconception environment of the egg is also affected in women of advanced reproductive age, with follicular fluid presenting distinct metabolomic (Dogan et al. 2020), hormonal (Pacella et al. 2012) and inflammatory (Piccinni et al. 2021) profiles. Similar age-dependent changes have also been documented in oviducts of bovine aging models (Tanaka et al. 2016). The uterine environment also presents signs of tissue dysfunction and inflammation with aging (Carnevale and Ginther 1992; Elmes et al. 2015; Simmen et al. 2015) that are linked to reduced pregnancy rates and a higher chance of pregnancy loss (Carnevale and Ginther 1992; Cano et al. 1995). Fetuses from older female mice presented more morphological abnormalities related to growth retardation and cardiovascular, brain and neural tube defects than fetuses from younger mice (Woods et al. 2017). Further, most of the developmental abnormalities seen in embryos from older mothers were reversed by transferring the embryos into the uterus of younger mice (Woods et al. 2017), demonstrating the importance of the post-implantation maternal environment for normal embryogenesis and fetal health.
The mechanisms by which maternal aging alters offspring development and adult phenotypes are not understood. Children from older mothers present altered methylation patterns in genes associated with the development of type 2 diabetes and neuropsychiatric disorders, and the control of inflammation and allergies (Yeung et al. 2024). Thus, at least some phenotypes are likely explained by molecular and epigenetic mechanisms. More intriguingly, older women present an overall, abnormal inflammatory state during pregnancy (Elovitz et al. 2011), and this has been shown to alter brain development (Graham et al. 2018; Rudolph et al. 2018; Rasmussen et al. 2019; Ramirez et al. 2020) and offspring behaviour (Graham et al. 2018; Sunwoo et al. 2018). Thus, it is possible that inflammatory signals such as cytokines act directly on developing offspring tissues or indirectly via influencing epigenetic modifications to control offspring phenotypes.
Because of this emerging data on changes to the oocyte and female reproductive tract with aging that are a potential cause of alterations to offspring phenotypes, it is important to understand the extent to which maternal age at birth affects the programming of offspring health later in life. In this study, we characterised fecundity and offspring phenotypes in female mice at the onset of reproductive aging. Firstly, we examined reproductive outcomes in breeder pairs over time to define the timing of fertility decline. We then directly compared pregnancy outcomes in female mice that were either young (3 months old) or of advanced maternal age (12 months old). Further, we assessed the growth, metabolic function, behaviour, and immune system in their adult offspring.
Materials and methods
Fecundity assessments
Female fecundity across the reproductive lifespan was analysed from litter size and pup weaning data collected from breeder pairs in a mouse colony, located at and managed by the Laboratory Animal Services, The University of Adelaide. Breeder pairs (one male and one female) were housed together at ~2.5 months of age and until a maximum of 18 months of age. Data was collected on number of litters, litter size at birth and number of pups weaned 3 weeks post-birth.
Offspring generation and body weight measurement
Offspring were produced by mating either young or reproductively aged female mice to young male mice. For this, young (8-week-old, nulliparous) and reproductively aged (40-week-old multiparous (i.e. ‘ex-breeder’)) C57BL/6J female mice, were obtained from the Animal Resources Centre (Canning Vale, WA, Australia). Female mice were housed in groups of five to seven until the females were 52 weeks of age (12 months old, equivalent to 41 years of age in women (Dutta and Sengupta 2016)), and the young controls were 12 weeks old (3 months old). Male mice were C57BL/6J at 8–12 weeks old and proven fertile. Females were housed with a male (1:1) for 1 week, separated, and then weighed 1 week later; with pregnancy indicated by a ≥ 3 g increase in body weight. After birth, the offspring remained with the dam until weaning at 3 weeks of age. Upon weaning, offspring were individually identified and housed in groups of five to seven with offspring from other mothers of the same type. Offspring body weights were measured weekly on week 3–10 postnatal, and then at 12 and 16 weeks postnatal. At 12 weeks of age, the offspring underwent behaviour testing. Glucose tolerance tests (GTT), and insulin tolerance tests (ITT), were conducted 2 weeks later and tissues were collected at 16 weeks of age.
Glucose and insulin tolerance test
Prior to the glucose tolerance test (GTT) at 13–14 weeks old, the offspring were fasted for 5–6 h, then injected intraperitoneally with 2 g glucose per kg of body weight in 0.9% NaCl solution. The same animals underwent insulin tolerance test (ITT) 1 week later at 14–15 weeks old. Mice received an intraperitoneal injection of 0.75 IU of Actrapid® Penfill® (Novo Nordisk Pharmaceuticals Pty Ltd., North Sydney, NSW, Australia) per kg of body weight in 0.9% NaCl solution. For both GTT and ITT, blood glucose was measured in tail tip blood samples at 0, 15, 30, 60 and 120 min post challenge using Accu-check Performa glucose meter (Roche Diabetes Care, North Ryde, NSW, Australia).
Blood collection and organ weight measurement
Tissues were collected from the offspring at 16 weeks old. For this, mice were fasted for 3 h, then blood was collected from a subset of animals by cardiac puncture for immune cell analysis. Briefly, mice were anaesthetised with 125–250 mg/kg 2,2,2, tribromoethanol (Sigma-Aldrich Pty Ltd, North Ryde BC, NSW, Australia) via intraperitoneal injection; and approximately 500 μL blood was collected from the heart using an 18G needle connected to a heparinised (10 USP unit/mL) syringe. The heparin-blood mixture was gently mixed and immediately used for immune cell analysis. Mice were humanely killed by cervical dislocation followed by whole body weight and organ measurement, namely: abdominal fat pad, spleen, liver, kidneys, heart and reproductive organs (testis or uterus plus ovaries).
Immune cell staining and flow cytometry
Reagents for immunophenotyping assays were acquired from Becton Dickinson Pty Ltd. (see Supplementary Table S1). 200 μL of heparinised blood was transferred into a 1.7 mL Eppendorf tube containing antibody cocktail (see Table S2), mixed and incubated at 4°C for 30 min. Samples were then transferred into 15 mL tubes and red blood cells (RBC) were lysed by adding 5 mL RBC lysis buffer (150 mM NH4Cl, 10 mM NaHCO3 and 1 mM EDTA), mixing and incubation at room temperature for 10 min. The sample was then centrifuged at 300g at room temperature for 5 min, the supernatant discarded, and the RBC lysis process repeated. After the second centrifugation, the cells were resuspended with 100 μL 0.5% bovine serum albumin (BSA)-supplemented phosphate buffered saline and transferred into a 5 mL test tube. The sample was analysed by flow cytometry and the proportion of immune cell populations were quantified using LSRFortessa X-20 Cell Analyser and FACSDiva analysis software (BD Biosciences, San Diego, CA, USA). The gating strategy for immune cell types was based on previous established methods (Hensel et al. 2019), and is shown in Supplementary Fig. S1. The specific immune cell proportion is calculated from number of each specific immune cell type divided by total number of cells multiplied by 100. Validation using blood samples taken from young and old mothers showed that the gating strategy employed successfully quantified the proportion of blood immune cells (Fig. S1b).
Behavioural testing
Behavioural testing was performed on one female and one male offspring per litter, at 12 weeks old. The tests conducted were open field and sociability as previously described (Loring et al. 2021), performed consistently in that order and between 11:00 and 14:00 hours each assessment day. Briefly, open field test is used to quantify locomotor activity and anxiety-like behaviour under stress-inducing conditions. For this, the mouse was placed in the southwest corner of a 40 cm × 40 cm plexiglass box with overhead light, for 5 min and movement into the outermost (darker) zone of the box is monitored (see Fig. S3a). Specifically, number of entries into, time spent within, and travel distance and speed in the demarked outer zone are recorded. Sociability test is employed to investigate social and autistic-like behaviours. For this, the mouse is placed in the centre of a rectangular plexiglass box consisting of three-chambers (each 20 cm × 40 cm), connected by up-sliding doors which are opened right before the start of recording. The sociability test consists of 3–5 min observations referred to as habituation, sociability and preference for social novelty (see Fig. S3b). At the end of each observation, the tested mouse is herded to the centre chamber and the door is closed. For habituation, only the test mouse was present in the maze. To measure sociability, a stranger mouse (age- and sex-matched) in a wire cage was placed in the left chamber. To measure preference for social novelty, two mice were placed at the left (familiar) and right chamber (stranger). The number and duration of the interactions were recorded manually when the tested mouse sniffed, interacted with, or climbed on the occupied cages. All testing was recorded and analysed using ANY-maze (Stoelting Co., Wood Dale, IL, USA).
Statistical analysis
Data are represented as mean ± s.e. of mean (s.e.m.) or mean ± s.d. as indicated. Statistical analysis was performed by either GraphPad Prism 9 (GraphPad Software LLC, San Diego, CA, USA), or IBM SPSS Statistics for Windows ver. 28.0.0.0 (IBM Corp, Armonk, NY, USA). The data was checked for Gaussian distribution (normality) and homoscedasticity (equal variance), to confirm that the assumptions of a linear model were met. The effect of age on litter size throughout female reproductive lifespan was analysed using a repeated measure mixed model analysis, followed by post hoc pairwise comparisons to determine differences in litter size between two consecutive months. The effect of age on litter size, pregnancy rate, livebirth rate, newborn death, pup survival, and maternal immune cell distribution were analysed by unpaired Student’s t-test or Fisher’s Exact test as indicated. Pup sex ratio was analysed using a two-tailed binomial test to compare each group’s observed sex ratio to the expected distribution of 0.5 for both sexes. Offspring postnatal growth trajectory, glucose metabolism, organ weight and immune cell phenotype were calculated by a multilevel, repeated measures mixed model analysis, with post hoc Fisher’s l.s.d. To understand possible predictors of the dependent variables studied, some analysis used either litter size (postnatal weight gain and behavioural testing) or body weight (glucose metabolism, organ weight and peripheral blood immune cell composition) as covariates at the time of the analysis. Litter (mother ID) was also introduced into the model to check for random effects over the covariance structure of the data, as per Golub and Sobin (2020).
Ethical statement and mouse husbandry
All procedures were approved by the University of Adelaide Animal Ethics Committee and were conducted in accordance with the Australian Code of Practice for the Care and Use of Animals for Scientific Purposes. Animals were maintained at 24°C on a 12-h light, 12-h dark cycle, with Envigo standard rodent diet (Teklad Global Rodent Diet® #0915: 19% (w/w) crude protein, 9% (w/w) fat, 45% (w/w) carbohydrate; Madison, WI, USA) and water available ad libitum.
Results
Parental reproductive aging is characterised by smaller litters and higher newborn death
Reproductive performance in mice within a breeding colony was monitored and showed a significant effect of the age of the parents on litter size. Month to month pairwise comparisons revealed that the first significant decrease in litter size occurred when the mice reached 11 months old, presenting an average litter size of 4.7 ± 2.3 pups that was lower than the average of 5.5 ± 3.0 pups at 10 months old (Fig. 1a). Thus, we used this information to classify mice as either young (< 11 months old) or old (≥ 11 months old). When offspring survival to the age of weaning (21 days old) was analysed using this classification, it was found that pup survival was reduced when the dams were older (Fig. 1b). Pup mortality rate was almost doubled when the mice were older compared to when they were young (27.5% vs 14.4% respectively; Fig. 1b). These data indicate that the effects of aging on mouse breeder pairs’ reproductive success are first seen at 11 months old and can be identified by smaller litters, as well as reduced pup survival.
Fecundity declines with parental age. (a) Litter size (i.e. number of pups born) over the lifespan of continuously housed male and female (1:1) breeder pairs. Black data points indicate ages at which mice were considered ‘young’, while grey data points indicate the ages after which there was a first significant reduction in litter size compared to the previous month, and mice were then considered ‘old’ (N = 48 female breeders; *P < 0.05 compared to 10-month-old time point). (b) Pup survival to weaning at 21 days post-birth in litters from female breeders at < 11 months old (black bar) compared to those born when females were ≥ 11 months old (grey bar) (N = 2063 pups from < 11 months, 592 pups from ≥ 11 months; ****P < 0.0001). Data was analysed using repeated measure mixed model with post hoc paired t-test comparisons test (a) or Fisher’s Exact test (b).
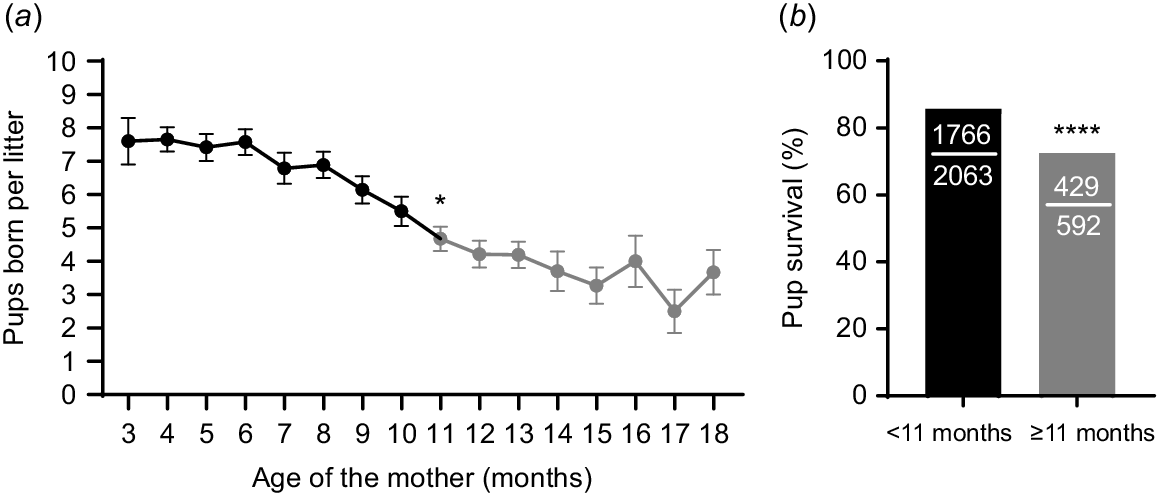
Aging impairs female fecundity and offspring survival
We next assessed the effects of reproductive aging on fecundity, pup survival and offspring phenotypes using C57BL6/J female mice. Reproductively old female mice (12 month old and multiparous) and young females (3 months old and nulliparous) were each paired (1:1) with 8–12 weeks old stud males to assess pregnancy and birth rates (Fig. 2a). Older females became pregnant at a rate that was not statistically different to that of young females; however, only 34.3% of pregnant old females gave birth to live pups, compared to 100% of pregnant young females (Table 1). The older females also had smaller litters, averaging 2.6 ± 1.3 pups per litter, compared to 6.9 ± 2.3 pups per litter in younger mice (Table 1). In addition, fewer of the offspring from older females survived to weaning, compared to young females (79.3% vs 96.9%, respectively; Table 1). Interestingly, offspring from young females were born at a sex ratio which was female-biased; while litters from older females had balanced sex ratios that were not different from the expected 50:50. It is not clear whether these sex ratio differences were present in newborns or due to sex-specific differences in pup death. These results show that early reproductive decline impairs female reproductive success and offspring survival.
Female offspring from older mothers are heavier. (a) Young (3 months) and reproductively aged (12 months) female mice were paired with young male mice to produce offspring. Offspring were assessed for body weight up to 16 weeks of age, and underwent behavioural, glucose and insulin tolerance, and immune cell phenotyping at the indicated ages. Body weight over time (from 3 to 16 weeks of age) for female (b) and male offspring (c) born to young females (black line) or older females (grey line). N = 77 pups from 11 young, 27 pups from 6 old dams/litters. Data are presented as mean ± s.e.m. Statistical analysis was a multilevel, repeated measures mixed model using litter size at birth as a covariate with post hoc Fisher’s Least Significant Difference test. *P < 0.05, **P < 0.01, ***P < 0.001.
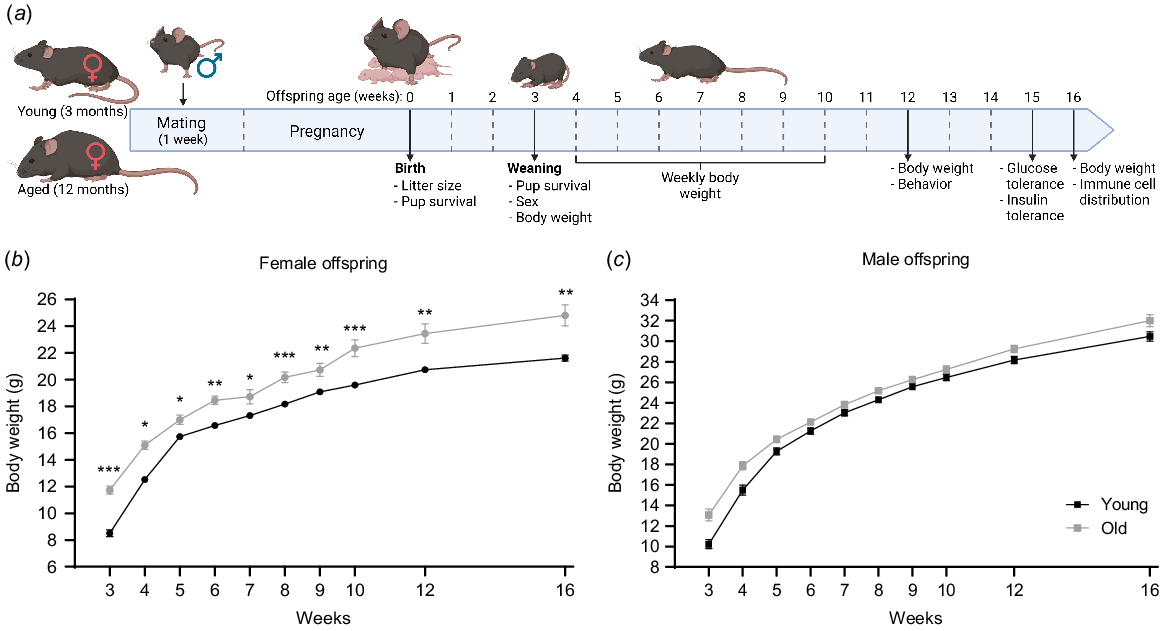
Maternal age | Young (3 months) | Old (12 months) | |
---|---|---|---|
Total number of females (n) | 18 | 52 | |
Number of pregnant females (%) | 14 (77.8) | 35 (67.3) | |
Number of females with live birth (%) | 14 (100) | 12 (34.3)^^^^ | |
Litter size at birth (mean ± s.d.) | 6.9 ± 2.3 | 2.6 ± 1.3**** | |
Pup survival (%) | 93/96 (96.9) | 23/29 (79.3)^^ | |
Sex ratio (F:M) | 0.7:0.3^ | 0.4:0.6 |
Litter size was counted at birth and offspring survival and sex ratio were determined at weaning (21 days of age).
****P < 0.001 using unpaired Student’s t-test.
^P < 0.05, ^^P < 0.01 and ^^^^P < 0.001 using a binomial test.
Maternal age at conception alters growth trajectory in female offspring
Postnatal growth of offspring was examined by measuring body weight from 3 to 16 weeks of age (Fig. 2a). Female offspring of old mothers were on average 2.3 g heavier than those born to young mothers, a difference that was statistically significant even accounting for the differences in litter size as a co-variate (Fig. 2b). In contrast, male offspring from older females had a growth trajectory that was not different from those born to young females (Fig. 2c). At 16 weeks of age, final body weight was recorded, and key organs collected and weighed, namely reproductive tract (uterus plus ovaries in females), testis (males), abdominal fat pad, spleen, liver, kidneys and heart. Female offspring born to older mothers were on average 3.54 g heavier than those from young, presenting 52% heavier abdominal fat pads (0.79 g vs 0.38 g respectively), and 19% lighter kidneys (0.32 g vs 0.26 g respectively) than those from young (Table S3). However, these relative differences in organ weights disappeared when adjusting for body weight as a co-variate, since the female offspring from older mothers are heavier overall (Table S3). Male offspring from both groups had similar body weights at 16 weeks of age; however, when organ weight was adjusted to body weight as a co-variate, males born to old mothers had 17% lighter abdominal fat pads and 5% heavier kidneys than those from young (Table S3).
At the same time, blood was collected for analysis of immune cell distribution in serum (see gating strategy in Fig. S1a). The older dams had lower CD4+ T cell and higher neutrophil proportions in their peripheral blood immune cell populations (Table S4), as expected (Levenson et al. 2020). There were no differences in the proportions of specific immune cell types in the male offspring from old mothers compared to young mothers (Table 2). However, female offspring of older mothers presented 9.3% higher proportion of circulating B cells (Table 2). Together, these findings indicate that the age of the mother at conception has a sex-distinct influence on offspring growth, but only a modest effect on adult offspring immune cell distribution.
Female offspring | ||||
---|---|---|---|---|
Maternal age | Young | Old | P-value | |
N pups (N mothers) | 16 (11) | 9 (9) | ||
Cell type | Cell proportion ± s.d. | Cell proportion ± s.d. | ||
CD4+ T cells | 7.79 ± 2.20 | 8.50 ± 1.80 | 0.7 | |
CD8+ T cells | 4.24 ± 1.77 | 3.87 ± 1.43 | 0.8 | |
B cells | 41.30 ± 5.95 | 45.16 ± 4.75 | 0.04* | |
NK cells | 1.06 ± 0.53 | 0.90 ± 0.37 | 0.1 | |
Monocytes | 3.28 ± 3.51 | 1.19 ± 0.83 | 0.3 | |
Neutrophils | 2.11 ± 1.02 | 3.10 ± 1.76 | 0.9 |
Male offspring | ||||
---|---|---|---|---|
Maternal age | Young | Old | P-value | |
N pups (N mothers) | 15 (11) | 10 (9) | ||
Cell type | Cell proportion ± s.d. | Cell proportion ± s.d. | ||
CD4+ T cells | 7.57 ± 1.50 | 7.98 ± 1.90 | 0.8 | |
CD8+ T cells | 4.49 ± 3.45 | 4.55 ± 2.15 | 0.9 | |
B cells | 43.23 ± 9.81 | 48.77 ± 5.19 | 0.9 | |
NK cells | 1.02 ± 0.52 | 1.16 ± 0.50 | 0.8 | |
Monocytes | 2.30 ± 3.08 | 2.17 ± 1.47 | 0.5 | |
Neutrophils | 5.71 ± 5.21 | 6.06 ± 4.78 | 0.2 |
s.d. = standard deviation, N = total number of pups (number of dams/litters) analysed per group at 16 weeks old, * indicates P-value < 0.05 by multilevel mixed model, using body weight as a covariate with post hoc Fisher’s Least Significant Difference test.
Maternal age alters glucose tolerance in male offspring
The offspring underwent metabolic assessments, namely glucose tolerance tests (GTT) at 14 weeks of age and insulin tolerance tests (ITT) at 15 weeks old (Fig. 2a). The age of the mother had no effect on female offspring glucose tolerance or insulin sensitivity (Figs 3a, b and S2a, b). Differences were apparent, however, in male offspring. Circulating glucose levels in male offspring from older mothers were on average 2.63 mmol/mL lower at 15, 30 and 60 min post-glucose challenge (Fig. 3c). This was confirmed by a lower area under the curve result for male offspring of older females (Fig. S2c). During the insulin tolerance test, the male offspring of older mothers exhibited an average 1.5 mmol/mL lower baseline (fasting) levels (0 min) compared to those from young (Fig. 3d). However, the relative response to insulin and the area under the curve result were not different between the two groups (Fig. 3d and S2d). These results suggest that maternal aging influences glucose tolerance in the male offspring.
Maternal aging alters glucose tolerance in male offspring. Blood glucose level at 0, 15, 30, 60 and 120 min post-glucose challenge in female (a) and male (c) offspring at 15 weeks old. Blood glucose level at 0, 15, 30, 60 and 120 min post-insulin challenge in female (b) and male offspring (d) at 15 weeks old. N = 77 pups from 11 young, 27 pups from 6 old dams/litters. Data are presented as mean ± s.e.m. Statistical analysis was multilevel, repeated measures mixed model, using body weight as a covariate with post hoc Fisher’s Least Significant Difference test. **P < 0.01, ***P < 0.001.
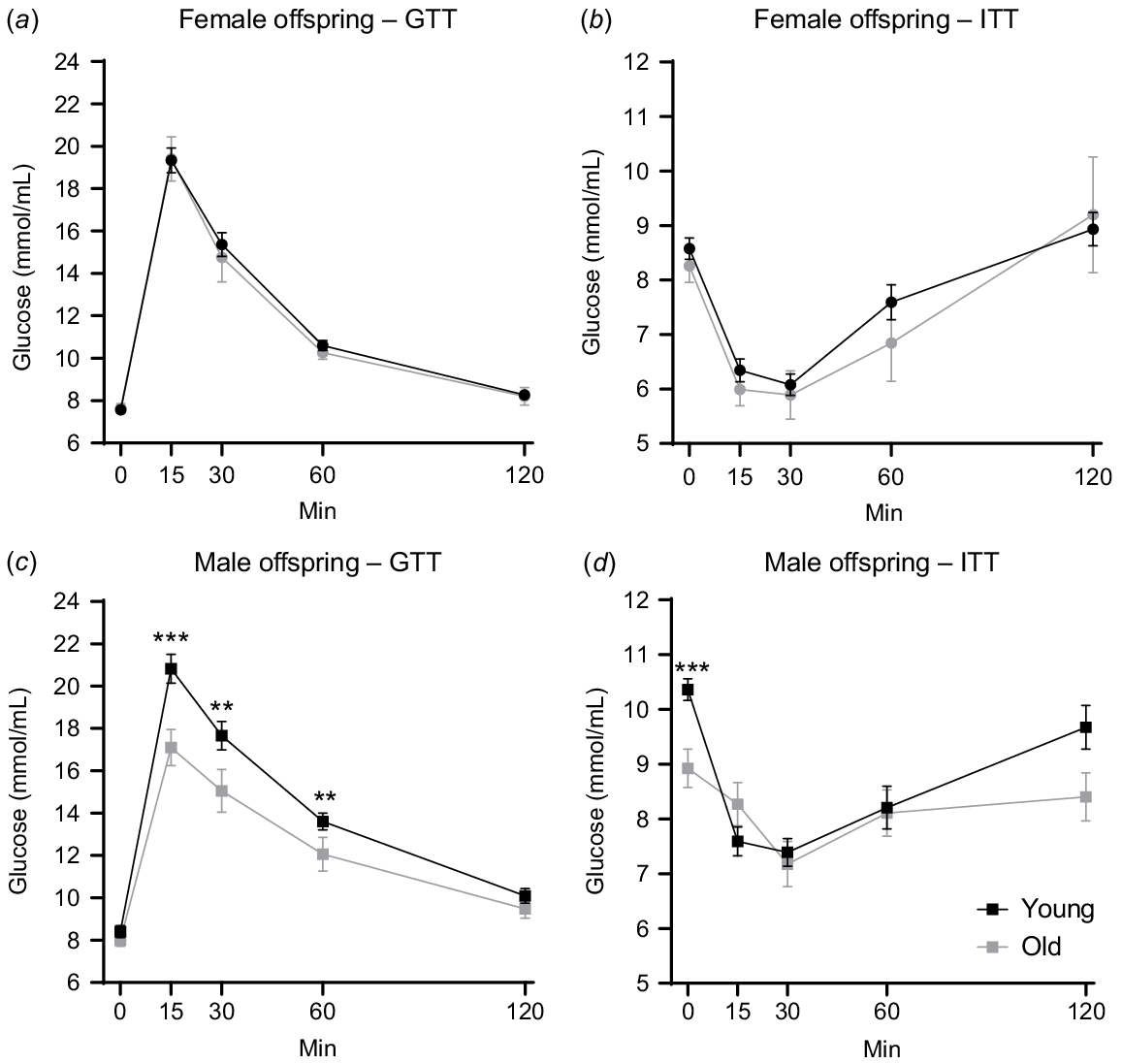
Offspring from older mothers display hyperactivity
At 12 weeks of age, the offspring underwent behavioural testing to assess neurodevelopment for anxiety-like and autistic-like behaviour (Fig. 2a). In open field tests, mice with anxiety-like phenotypes spend most of the time in the outer (darker) zone (see Fig. S3a). Female offspring from both groups displayed similar behaviours during the open field assessment (Fig. 4a–d). In contrast, male offspring born to old females travelled farther (14.87 cm vs 9.68 cm; Fig. 4g) and faster (0.056 m/s vs 0.036 m/s; Fig. 4h) in the outer zone compared to those born to young mothers. The offspring from older versus younger mothers did not exhibit any differences in behaviour during the sociability tests (Fig. S3b–n). Taken together, these results indicate that male offspring from older mothers showed hyperactivity, a marker of anxiety-like behaviour.
Maternal aging increases anxiety markers in male offspring. Open field test assessments in female (a–d) and male (e–h) offspring at 12 weeks old. Number of entries to- (a, e), time spent in- (b, f), distance travelled in- (c, g) and average speed in- outer zone (d, h) recorded. N = 13 pups from seven young, eight pups from 4 old dams/litters (one female and one male offspring per litter). Data are represented as mean ± s.e.m. Statistical analysis was a multilevel mixed model, using litter size as a covariate with post hoc Fisher’s Least Significant Difference test. *P < 0.05; **P < 0.01.
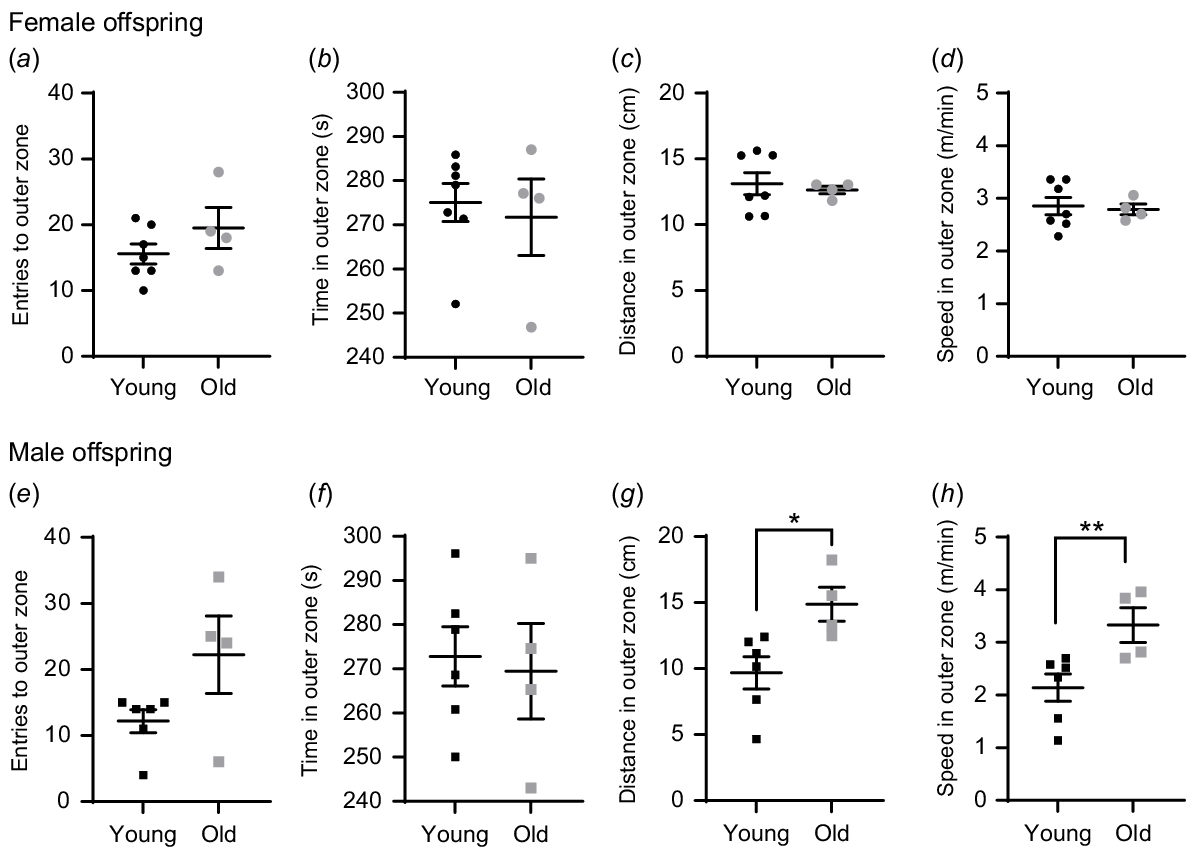
Discussion
There is increasing understanding that offspring phenotypes are influenced by maternal physiology, and this includes maternal age. Our study shows that fecundity is reduced around 11–12 months in multiparous female mice, with lower rates of pup survival to weaning. Young adult offspring from older females also presented altered phenotypes, with females having higher body weight and proportion of B cells, while males showed altered organ weight, glucose performance and hyperactivity behaviour.
Aging has been linked to the abnormal function or physiological failure of several body systems, affecting morbidity and mortality later in life. However, less is known about the onset of reproductive aging, which happens midway through the lifespan and is characterised by a decline in reproductive function, which we have identified to first be apparent after 11 months old in continuously breeding mice. We found that breeding pairs with females that were at or older than 11 months of age had smaller litters and lower pup survival, consistent with previous findings (Sampino et al. 2017; Mao et al. 2018; Levenson et al. 2020). Although increased paternal age is also known to negatively affect pup survival (García-Palomares et al. 2009; Gonzalez et al. 2024), we show that this decline in live offspring is specifically due to maternal aging since an independent cohort of female mice mated with young males exhibited the same loss of pups. Others have reported that fetuses from older females present morphological abnormalities related to growth retardation and cardiovascular, brain and neural tube defects (Woods et al. 2017). Fetuses with moderate to severe abnormalities are less likely to survive through pregnancy and the neonatal period, which can explain observations of decreased litter size and increased newborn death. On the other hand, those pups with milder defects may survive to adulthood and only display subtle physiological abnormalities later in life.
Curiously, litters born to older females had near-balanced sex ratio, whilst those born to younger females had more female than male pups. This could be the result of increased incidence of detrimental congenital issues in the pups or a difference in maternal behaviours in older dams. Timing of mating affects litter sex ratio in some strains, with early matings producing a higher proportion of females (Jimenez et al. 2001). Stresses to the mother’s environment can also reduce the proportion of male offspring. (Lane and Hyde 1973; Krackow 1997). The basis of maternal age-related differences in offspring sex ratio and whether these observations are relevant to humans requires further investigation.
Growing evidence shows that parental aging alters offspring development, growth, metabolism, health and behaviour (Velazquez et al. 2016; Sampino et al. 2017; Woods et al. 2017; Han et al. 2018; Mao et al. 2018; Gonzalez et al. 2024). We found that female offspring born to older females were heavier compared to those from young females, and this was independent of smaller litter size, which is associated with increased birth weight. A sex-specific effect of advanced maternal age on female body weight was also found in offspring born from embryo transfer (Velazquez et al. 2016). In that study, they also found that female offspring had higher blood glucose level post-glucose challenge, while our findings showed that it was the male offspring from old mothers that exhibited lower fasting and post-glucose challenge circulating glucose levels. In addition to this, post-mortem organ weight analysis showed that male offspring born to older females had lower abdominal fat, but higher kidney weight compared to their counterparts, while in Velazquez et al. (2016), female offspring from old mothers had lower spleen weight compared to those from young mothers. Our study used C57BL6/J × C57Bl6/J offspring; however, Velazquez et al. used CBA × C57Bl6/J embryos, which could explain the sex differences in glucose performance and organ weight. Different strains of mice have variable responses to glucose performance tests (Berglund et al. 2008; Montgomery et al. 2013), plus CBA male mice (as in the progenitor used in the Velazquez study) show deterioration of their glucose tolerance relatively earlier in life, around 5–8 months old (Leiter 1988). Further, the reproductively aged mice in our study were multiparous. This may also explain distinct observations between studies, as maternal parity status can affect offspring body weight in mice (Rebholz et al. 2012), which would have downstream consequences on metabolic parameters. Cumulatively, these data from our work and others show that maternal age influences offspring growth and metabolism, which deserves future investigation in humans.
Similar to others (Levenson et al. 2020), we found that older mothers had lower levels of CD4+ T cells, which are important to promote maternal–fetal tolerance during pregnancy (Aluvihare et al. 2004; Kahn and Baltimore 2010; Green et al. 2023). It is speculated that immune phenotypes in offspring of older mothers may be caused by a more inflammatory uterine environment. While Levenson et al. found altered immune function in infant mice born to older mothers (Levenson et al. 2020), we show a small increase in the proportion of B cells in the adult female offspring. It is possible that the altered immune responses are more acute in the perinatal stages, and then normalise over time, or that an altered immune state only becomes apparent under distinct stress conditions.
Lastly, we document altered behaviour in the offspring of older mothers. The male offspring exhibited mild anxiety-like behaviours, showing increased locomotor activity or hyperactivity, suggesting an impact of maternal aging on neurodevelopment. Similarly, others have reported advanced maternal age induced anxiety-like behaviour in mice (Sampino et al. 2017) and rats (Han et al. 2018). In addition, adult offspring from old mothers have impaired spatial learning and social behaviour (Han et al. 2018; Mao et al. 2018). Although not statistically significant, we observed a trend towards altered sociability in female offspring of older mothers that was not apparent with males. Future studies should explore whether aging or stress exacerbates either immune or behavioural phenotypes in offspring of older dams.
Overall, our study confirms that maternal age influences offspring phenotypes. Specifically, advanced maternal age altered offspring development at various stages of life, influencing early growth patterns, as well as metabolism and behaviour in adulthood. The mechanisms by which maternal age influences offspring physiology remain to be determined and are an active area of investigation that is highly relevant to humans, as women increasingly choose to have children in their 30s and early 40s. These mechanisms are likely to be multi-factorial and range from intrinsic epigenetic changes within oocytes during ovarian aging, to the higher maternal body weight and changed systemic metabolism that occurs with increased age. Our results contribute to the body of evidence that demonstrates maternal developmental programming of offspring physiology. Documenting the range and relative extent of phenotypes is essential for understanding the mechanisms by which advanced age in mothers differentially affects male and female offspring.
Data availability
No datasets were generated during this study. Data is available upon reasonable request to the corresponding author.
Declaration of funding
This study was supported by funds from The National Health and Medical Research Council (NHMRC; project ID: APP1165633).
References
Aluvihare VR, Kallikourdis M, Betz AG (2004) Regulatory T cells mediate maternal tolerance to the fetus. Nature Immunology 5(3), 266-271.
| Crossref | Google Scholar | PubMed |
AIHW (2023) Australia’s mothers and babies. Available at https://www.aihw.gov.au/reports/mothers-babies/australias-mothers-babies/contents/overview-and-demographics/maternal-age
Berglund ED, Li CY, Poffenberger G, Ayala JE, Fueger PT, Willis SE, Jewell MM, Powers AC, Wasserman DH (2008) Glucose metabolism in vivo in four commonly used inbred mouse strains. Diabetes 57(7), 1790-1799.
| Crossref | Google Scholar | PubMed |
Bertoldo MJ, Listijono DR, Ho W-HJ, Riepsamen AH, Goss DM, Richani D, Jin XL, Mahbub S, Campbell JM, Habibalahi A, Loh W-GN, Youngson NA, Maniam J, Wong ASA, Selesniemi K, Bustamante S, Li C, Zhao Y, Marinova MB, Kim L-J, Lau L, Wu RM, Mikolaizak AS, Araki T, Le Couteur DG, Turner N, Morris MJ, Walters KA, Goldys E, O’Neill C, Gilchrist RB, Sinclair DA, Homer HA, Wu LE (2020) NAD+ repletion rescues female fertility during reproductive aging. Cell Reports 30(6), 1670-1681.e7.
| Crossref | Google Scholar |
Biggers JD, Finn CA, Mc LA (1962) Long-term reproductive performance of female mice. II. Variation of litter size with parity. Reproduction 3(3), 313-330.
| Crossref | Google Scholar | PubMed |
Bingley PJ, Douek IF, Rogers CA, Gale EA (2000) Influence of maternal age at delivery and birth order on risk of type 1 diabetes in childhood: prospective population based family study. BMJ 321(7258), 420-424.
| Crossref | Google Scholar | PubMed |
Camlin NJ, McLaughlin EA, Holt JE (2017) The use of C57Bl/6 × CBA F1 hybrid cross as a model for human age-related oocyte aneuploidy. Molecular Reproduction and Development 84(1), 6-7.
| Crossref | Google Scholar | PubMed |
Cano F, Simón C, Remohí J, Pellicer A (1995) Effect of aging on the female reproductive system: evidence for a role of uterine senescence in the decline in female fecundity. Fertility and Sterility 64(3), 584-589.
| Crossref | Google Scholar | PubMed |
Cardwell CR, Stene LC, Joner G, Bulsara MK, Cinek O, Rosenbauer J, Ludvigsson J, Jané M, Svensson J, Goldacre MJ, Waldhoer T, Jarosz-Chobot P, Gimeno SGA, Chuang L-M, Parslow RC, Wadsworth EJK, Chetwynd A, Pozzilli P, Brigis G, Urbonaitė B, Šipetić S, Schober E, Devoti G, Ionescu-Tirgoviste C, de Beaufort CE, Stoyanov D, Buschard K, Patterson CC (2010) Maternal age at birth and childhood type 1 diabetes: a pooled analysis of 30 observational studies. Diabetes 59(2), 486-494.
| Crossref | Google Scholar | PubMed |
Carnevale EM, Ginther OJ (1992) Relationships of age to uterine function and reproductive efficiency in mares. Theriogenology 37(5), 1101-1115.
| Crossref | Google Scholar | PubMed |
Cimadomo D, Fabozzi G, Vaiarelli A, Ubaldi N, Ubaldi FM, Rienzi L (2018) Impact of maternal age on oocyte and embryo competence. Frontiers in Endocrinology 9, 327.
| Crossref | Google Scholar |
Dioun AF, Harris SK, Hibberd PL (2003) Is maternal age at delivery related to childhood food allergy? Pediatric Allergy and Immunology 14(4), 307-311.
| Crossref | Google Scholar | PubMed |
Dogan B, Karaer A, Tuncay G, Tecellioglu N, Mumcu A (2020) High-resolution 1H-NMR spectroscopy indicates variations in metabolomics profile of follicular fluid from women with advanced maternal age. Journal of Assisted Reproduction and Genetics 37(2), 321-330.
| Crossref | Google Scholar | PubMed |
Dutta S, Sengupta P (2016) Men and mice: relating their ages. Life Sciences 152, 244-248.
| Crossref | Google Scholar | PubMed |
Elmes M, Szyszka A, Pauliat C, Clifford B, Daniel Z, Cheng Z, Wathes C, McMullen S (2015) Maternal age effects on myometrial expression of contractile proteins, uterine gene expression, and contractile activity during labor in the rat. Physiological Reports 3(4), e12305.
| Crossref | Google Scholar |
Elovitz MA, Brown AG, Breen K, Anton L, Maubert M, Burd I (2011) Intrauterine inflammation, insufficient to induce parturition, still evokes fetal and neonatal brain injury. International Journal of Developmental Neuroscience 29(6), 663-671.
| Crossref | Google Scholar | PubMed |
Finn CA (1963) Reproductive capacity and litter size in mice: effect of age and environment. Reproduction 6(2), 205-214.
| Crossref | Google Scholar | PubMed |
Fitzpatrick KE, Tuffnell D, Kurinczuk JJ, Knight M (2017) Pregnancy at very advanced maternal age: a UK population-based cohort study. BJOG 124(7), 1097-1106.
| Crossref | Google Scholar | PubMed |
García-Palomares S, Pertusa JF, Miñarro J, García-Pérez MA, Hermenegildo C, Rausell F, Cano A, Tarín JJ (2009) Long-term effects of delayed fatherhood in mice on postnatal development and behavioral traits of offspring. Biology of Reproduction 80(2), 337-342.
| Crossref | Google Scholar | PubMed |
Glick I, Kadish E, Rottenstreich M (2021) Management of pregnancy in women of advanced maternal age: improving outcomes for mother and baby. International Journal of Women’s Health 13, 751-759.
| Crossref | Google Scholar |
Golub MS, Sobin CA (2020) Statistical modeling with litter as a random effect in mixed models to manage “intralitter likeness”. Neurotoxicology and Teratology 77, 106841.
| Crossref | Google Scholar | PubMed |
Gonzalez MB, Campugan CA, Connaughton HS, Andreas E, Winstanley YE, Williams EJ, Dorian CL, Robertson SA, Shoubridge C, Robker RL (2024) Reproductive ageing: BGP-15 mitigates adverse impacts of aging on sperm quality, fertility, and offspring health in male mice. Reproduction 168(3), e240105.
| Crossref | Google Scholar | PubMed |
Graham AM, Rasmussen JM, Rudolph MD, Heim CM, Gilmore JH, Styner M, Potkin SG, Entringer S, Wadhwa PD, Fair DA, Buss C (2018) Maternal systemic interleukin-6 during pregnancy is associated with newborn amygdala phenotypes and subsequent behavior at 2 years of age. Biological Psychiatry 83(2), 109-119.
| Crossref | Google Scholar | PubMed |
Green ES, Moldenhauer LM, Groome HM, Sharkey DJ, Chin PY, Care AS, Robker RL, McColl SR, Robertson SA (2023) Regulatory T cells are paramount effectors in progesterone regulation of embryo implantation and fetal growth. JCI Insight 8(11), e162995.
| Crossref | Google Scholar |
Hamatani T, Falco G, Carter MG, Akutsu H, Stagg CA, Sharov AA, Dudekula DB, VanBuren V, Ko MSH (2004) Age-associated alteration of gene expression patterns in mouse oocytes. Human Molecular Genetics 13(19), 2263-2278.
| Crossref | Google Scholar | PubMed |
Han W, Dong X, Song X, Cheng L, Xie L, Chen H, Jiang L (2018) Effects of advanced maternal age on cognitive and emotional development in offspring rats. Behavioural Brain Research 353, 218-226.
| Crossref | Google Scholar | PubMed |
Hensel JA, Khattar V, Ashton R, Ponnazhagan S (2019) Characterization of immune cell subtypes in three commonly used mouse strains reveals gender and strain-specific variations. Laboratory Investigation 99(1), 93-106.
| Crossref | Google Scholar | PubMed |
Jimenez A, Gutierrez-Adan A, Fuente Jdl, Pintado B (2001) Abstracts for poster presentation-sexing-relationship between time to synchrony of embryo transfer and sex ratio in mice. Theriogenology 55(1), 500.
| Google Scholar |
Kahn DA, Baltimore D (2010) Pregnancy induces a fetal antigen-specific maternal T regulatory cell response that contributes to tolerance. Proceedings of the National Academy of Sciences of the United States of America 107(20), 9299-9304.
| Crossref | Google Scholar | PubMed |
Krackow S (1997) Effects of mating dynamics and crowding on sex ratio variance in mice. Reproduction 110(1), 87-90.
| Crossref | Google Scholar | PubMed |
Lane EA, Hyde TS (1973) Effect of maternal stress on fertility and sex ratio: a pilot study with rats. Journal of Abnormal Psychology 82(1), 78.
| Crossref | Google Scholar | PubMed |
Lean SC, Derricott H, Jones RL, Heazell AEP (2017) Advanced maternal age and adverse pregnancy outcomes: a systematic review and meta-analysis. PLoS ONE 12(10), e0186287.
| Crossref | Google Scholar | PubMed |
Leiter EH (1988) Control of spontaneous glucose intolerance, hyperinsulinemia, and islet hyperplasia in nonobese C3H.SW male mice by Y-linked locus and adrenal gland. Metabolism 37(7), 689-696.
| Crossref | Google Scholar | PubMed |
Levenson D, Romero R, Garcia-Flores V, Miller D, Xu Y, Sahi A, Hassan SS, Gomez-Lopez N (2020) The effects of advanced maternal age on T-cell subsets at the maternal–fetal interface prior to term labor and in the offspring: a mouse study. Clinical and Experimental Immunology 201(1), 58-75.
| Crossref | Google Scholar | PubMed |
Li C, He X, Huang Z, Han L, Wu X, Li L, Xin Y, Ge J, Sha J, Yin Z, Wang Q (2020) Melatonin ameliorates the advanced maternal age-associated meiotic defects in oocytes through the SIRT2-dependent H4K16 deacetylation pathway. Aging 12(2), 1610-1623.
| Crossref | Google Scholar | PubMed |
Loring KE, Mattiske T, Lee K, Zysk A, Jackson MR, Noebels JL, Shoubridge C (2021) Early 17β-estradiol treatment reduces seizures but not abnormal behaviour in mice with expanded polyalanine tracts in the Aristaless related homeobox gene (ARX). Neurobiology of Disease 153, 105329.
| Crossref | Google Scholar | PubMed |
Lu H-Y, Chiu C-W, Kao P-H, Tsai Z-T, Gau C-C, Lee W-F, Wu C-Y, Lan Y-T, Hung C-C, Chang F-Y, Huang Y-W, Huang H-Y, Chang-Chien J, Tsai H-J, Yao T-C (2020) Association between maternal age at delivery and allergic rhinitis in schoolchildren: a population-based study. World Allergy Organization Journal 13(6), 100127.
| Crossref | Google Scholar | PubMed |
Mao W-J, Wu Z-Y, Yang Z-H, Xu Y-W, Wang S-Q (2018) Advanced maternal age impairs spatial learning capacity in young adult mouse offspring. American Journal of Translational Research 10(3), 975-988.
| Google Scholar | PubMed |
Mara JN, Zhou LT, Larmore M, Johnson B, Ayiku R, Amargant F, Pritchard MT, Duncan FE (2020) Ovulation and ovarian wound healing are impaired with advanced reproductive age. Aging 12(10), 9686-9713.
| Crossref | Google Scholar | PubMed |
Montgomery MK, Hallahan NL, Brown SH, Liu M, Mitchell TW, Cooney GJ, Turner N (2013) Mouse strain-dependent variation in obesity and glucose homeostasis in response to high-fat feeding. Diabetologia 56(5), 1129-1139.
| Crossref | Google Scholar | PubMed |
Morse A (2022) Fertility rates: declined for younger women, increased for older women. In ‘America counts: stories behind the numbers’. (National Centre for Health Statistics – United States Census Bureau: Washington, DC). Available at https://www.census.gov/library/stories/2022/04/fertility-rates-declined-for-younger-women-increased-for-older-women.html
Pacella L, Zander-Fox DL, Armstrong DT, Lane M (2012) Women with reduced ovarian reserve or advanced maternal age have an altered follicular environment. Fertility and Sterility 98(4), 986-994.e2.
| Crossref | Google Scholar | PubMed |
Piccinni M-P, Vicenti R, Logiodice F, Fabbri R, Kullolli O, Pallecchi M, Paradisi R, Danza G, Macciocca M, Lombardelli L, Seracchioli R (2021) Description of the follicular fluid cytokine and hormone profiles in human physiological natural cycles. The Journal of Clinical Endocrinology & Metabolism 106(2), e721-e738.
| Crossref | Google Scholar | PubMed |
Ramirez JSB, Graham AM, Thompson JR, Zhu JY, Sturgeon D, Bagley JL, Thomas E, Papadakis S, Bah M, Perrone A, Earl E, Miranda-Dominguez O, Feczko E, Fombonne EJ, Amaral DG, Nigg JT, Sullivan EL, Fair DA (2020) Maternal interleukin-6 is associated with macaque offspring amygdala development and behavior. Cerebral Cortex 30(3), 1573-1585.
| Crossref | Google Scholar | PubMed |
Rasmussen JM, Graham AM, Entringer S, Gilmore JH, Styner M, Fair DA, Wadhwa PD, Buss C (2019) Maternal interleukin-6 concentration during pregnancy is associated with variation in frontolimbic white matter and cognitive development in early life. NeuroImage 185, 825-835.
| Crossref | Google Scholar | PubMed |
Rebholz SL, Jones T, Burke KT, Jaeschke A, Tso P, D’Alessio DA, Woollett LA (2012) Multiparity leads to obesity and inflammation in mothers and obesity in male offspring. American Journal of Physiology – Endocrinology and Metabolism 302(4), E449-E457.
| Crossref | Google Scholar | PubMed |
Reyes JM, Silva E, Chitwood JL, Schoolcraft WB, Krisher RL, Ross PJ (2017) Differing molecular response of young and advanced maternal age human oocytes to IVM. Human Reproduction 32(11), 2199-2208.
| Crossref | Google Scholar | PubMed |
Rudolph MD, Graham AM, Feczko E, Miranda-Dominguez O, Rasmussen JM, Nardos R, Entringer S, Wadhwa PD, Buss C, Fair DA (2018) Maternal IL-6 during pregnancy can be estimated from newborn brain connectivity and predicts future working memory in offspring. Nature Neuroscience 21(5), 765-772.
| Crossref | Google Scholar | PubMed |
Sampino S, Stankiewicz AM, Zacchini F, Goscik J, Szostak A, Swiergiel AH, Drago G, Modlinski JA, Ptak GE (2017) Pregnancy at advanced maternal age affects behavior and hippocampal gene expression in mouse offspring. The Journals of Gerontology: Series A 72(11), 1465-1473.
| Crossref | Google Scholar |
Sandin S, Hultman CM, Kolevzon A, Gross R, MacCabe JH, Reichenberg A (2012) Advancing maternal age is associated with increasing risk for autism: a review and meta-analysis. Journal of the American Academy of Child & Adolescent Psychiatry 51(5), 477 486.e1.
| Crossref | Google Scholar |
Shan D, Qiu P-Y, Wu Y-X, Chen Q, Li A-L, Ramadoss S, Wang R-R, Hu Y-Y (2018) Pregnancy outcomes in women of advanced maternal age: a retrospective cohort study from China. Scientific Reports 8(1), 12239.
| Crossref | Google Scholar | PubMed |
Simmen RCM, Heard ME, Simmen AM, Montales MTM, Marji M, Scanlon S, Pabona JMP (2015) The Krüppel-like factors in female reproductive system pathologies. Journal of Molecular Endocrinology 54(2), R89-R101.
| Crossref | Google Scholar | PubMed |
Sunwoo J-S, Jeon D, Lee S-T, Moon J, Yu J-S, Park D-K, Bae J-Y, Lee DY, Kim S, Jung K-H, Park K-I, Jung K-Y, Kim M, Lee SK, Chu K (2018) Maternal immune activation alters brain microRNA expression in mouse offspring. Annals of Clinical and Translational Neurology 5(10), 1264-1276.
| Crossref | Google Scholar | PubMed |
Svanes C, Omenaas E, Heuch J, Irgens L, Gulsvik A (1998) Birth characteristics and asthma symptoms in young adults: results from a population-based cohort study in Norway. European Respiratory Journal 12(6), 1366-1370.
| Crossref | Google Scholar | PubMed |
Tanaka H, Ohtsu A, Shiratsuki S, Kawahara-Miki R, Iwata H, Kuwayama T, Shirasuna K (2016) Age-dependent changes in inflammation and extracellular matrix in bovine oviduct epithelial cells during the post-ovulatory phase. Molecular Reproduction and Development 83(9), 815-826.
| Crossref | Google Scholar | PubMed |
Velazquez MA, Smith CGC, Smyth NR, Osmond C, Fleming TP (2016) Advanced maternal age causes adverse programming of mouse blastocysts leading to altered growth and impaired cardiometabolic health in post-natal life. Human Reproduction 31(9), 1970-1980.
| Crossref | Google Scholar | PubMed |
Warburton D (2005) Biological aging and the etiology of aneuploidy. Cytogenetic and Genome Research 111(3–4), 266-272.
| Crossref | Google Scholar | PubMed |
Woods L, Perez-Garcia V, Kieckbusch J, Wang X, DeMayo F, Colucci F, Hemberger M (2017) Decidualisation and placentation defects are a major cause of age-related reproductive decline. Nature Communications 8(1), 352.
| Crossref | Google Scholar | PubMed |
Yeung E, Biedrzycki RJ, Gómez Herrera LC, Issarapu P, Dou J, Marques IF, Mansuri SR, Page CM, Harbs J, Khodasevich D, Poisel E, Niu Z, Allard C, Casey E, Berstein FM, Mancano G, Elliott HR, Richmond R, He Y, Ronkainen J, Sebert S, Bell EM, Sharp G, Mumford SL, Schisterman EF, Chandak GR, Fall CHD, Sahariah SA, Silver MJ, Prentice AM, Bouchard L, Domellof M, West C, Holland N, Cardenas A, Eskenazi B, Zillich L, Witt SH, Send T, Breton C, Bakulski KM, Fallin MD, Schmidt RJ, Stein DJ, Zar HJ, Jaddoe VWV, Wright J, Grazuleviciene R, Gutzkow KB, Sunyer J, Huels A, Vrijheid M, Harlid S, London S, Hivert M-F, Felix J, Bustamante M, Guan W (2024) Maternal age is related to offspring DNA methylation: a meta-analysis of results from the PACE consortium. Aging Cell 23(8), e14194.
| Crossref | Google Scholar | PubMed |
Zhang J-J, Liu X, Chen L, Zhang S, Zhang X, Hao C, Miao Y-L (2020) Advanced maternal age alters expression of maternal effect genes that are essential for human oocyte quality. Aging 12(4), 3950-3961.
| Crossref | Google Scholar | PubMed |
Zuccotti M, Boiani M, Garagna S, Redi CA (1998) Analysis of aneuploidy rate in antral and ovulated mouse oocytes during female aging. Molecular Reproduction and Development 50(3), 305-312.
| Crossref | Google Scholar |