Organs-on-a-chip to understand reproduction
J. Julie Kim A *A
Abstract
Organs-on-a-chip technology (OoC) has transformed biomedical research with realistic human organ models for drug testing and disease research. While still in its infancy in reproductive research, OoC holds immense potential. The development and use of OoC platforms specifically for reproductive processes are highlighted here, including a multi-organ platform for studying the female reproductive tract, devices for investigating embryo implantation, and microfluidic systems aimed at improving pre-implantation embryos. These findings emphasize the transformative potential of OoC technology in reproductive biology, advocating for its broader adoption in the field. The potential applications of OoC technology in both human and animal reproduction are vast and significant. By providing detailed and controlled models of reproductive systems, OoC technology is set to transform reproductive health research, steering it towards a more sustainable and ethical future.
Keywords: assisted reproductive technologies, embryo, female reproductive tract, implantation, microphysiological systems, organs-on-a-chip, reproduction.
Introduction
Organs-on-a-chip (OoC) are advanced microfluidic systems designed to mimic the complex structure and function of human organs. By incorporating living cells within precisely engineered environments, these devices allow for detailed study of organ-level physiology and disease processes in a controlled setting. In the field of reproductive physiology, OoC present an advanced tool for exploring reproductive mechanisms, modeling reproductive disorders, and testing new treatments with exceptional accuracy. These systems simulate the dynamic conditions of reproductive tissues, offering a new method to enhance our understanding of fertility, embryonic growth, and overall reproductive health, which can drive forward both basic research and clinical applications. The field of reproductive biology has much to gain in implementing novel technologies given the complexity of reproductive function, ethical limitations, and animal welfare concerns. This review will highlight current advancements, applications, and potential future directions of OoC in the field of reproductive physiology.
The evolution of in vitro systems
History of cell culture
Cell culture, the process of growing cells in a controlled artificial environment, has a rich history marked by significant scientific advancements. The cell theory, which posits that living organisms are composed of cells, was introduced by Matthias Schleiden and Theodor Schwann in the early 19th century, laying the groundwork for cell biology (Vasil 2008). At the beginning of the 20th century, Ross Harrison and Leo Loeb kept tissue or pieces of organs alive in test tubes in liquid medium (Taylor 2014). By the mid-20th century, the use of antibiotics in cell culture greatly reduced contamination issues. The first continuous human cell line, HeLa cells, which were created in 1951 produced from Henrietta Lacks’ cervical cancer cells, revolutionized medical science. Advances in culture media compositions, like Eagle’s Minimal Essential Medium made by Harry Eagle, standardized culture media, thus enhancing the consistency in experiments (Eagle 1959). The next decades brought innovations in developmental biology, drug discovery, and regenerative medicine, with the advent of three-dimensional (3D) cell culture and organoids offering more physiologically relevant models. Cell culture is of paramount importance in science and medicine and continues to drive forward scientific and therapeutic advancements.
In vitro models – the endometrium
In vitro models have become increasingly important for advancing our understanding of tissue function and mechanisms. This is particularly true for the endometrial lining of the uterus which is the first point of contact for a developing embryo to implant and settle in for the duration of the pregnancy. In humans, implantation is successful only if the endometrium has remodeled to a ‘receptive’ state which is driven by ovarian sex hormones (Lessey and Young 2019). This complex process has prompted extensive research, and as a result, the endometrium has been studied outside the body in various ways. To retain key attributes of native endometrial tissue, including tissue architecture, composition, and direct intercellular contact, endometrial explants were used for short-term studies (Schafer et al. 2011). However, substantial tissue degradation occurred rapidly and does not allow for long-term studies. As a result, organotypic endometrial models were created, which usually included the major cell types, stromal and epithelial cells embedded in a matrix to mimic the scaffolding and structure of uterine tissue. The endometrial response to hormones is well replicated in these models, demonstrating the importance of paracrine and endocrine signaling amongst various cell types (Bläuer et al. 2005; Murphy et al. 2022).
A widely adopted 3D culture method of endometrial epithelial cells was developed by two independent groups, Turco et al. (2017) and Boretto et al. (2017). Both luminal and glandular-like endometrial epithelial cells were present in organoids, cultured in Matrigel, and maintained significant endometrial characteristics, including glycogen production and response to hormones (Boretto et al. 2017). The organoids could be maintained for more than 4–6 months and were able to be passaged. Other organoid models have been developed to further mimic the endometrium by including an additional cell type: stromal cells. A scaffold-free multicellular organoid included both primary endometrial epithelial and stromal cell type (Wiwatpanit et al. 2020). Primary epithelial and stromal cells, plated on low-adhesion agarose micromolds, self-organized to reflect the native tissue with one cell layer of polarized epithelial cells surrounding and inner cluster of stromal cells. The organoids expressed functional markers, including estrogen receptor, progesterone receptor, and androgen receptor, and responded to menstrual cycle hormones. Another two-cell organoid was constructed and termed an assembloid, bringing together epithelial organoids in Matrigel, surrounded by a bed of stromal cells on the exterior, which responded to hormones and kinase inhibitors (Rawlings et al. 2021). Organoid technology has changed how researchers study the endometrium and its diseases, with a number of reviews written in recent years (Campo et al. 2020; Alzamil et al. 2021; Cadena et al. 2021; Fitzgerald et al. 2021; Heremans et al. 2021; Song and Fazleabas 2021; Murphy et al. 2022).
Organs-on-a-chip
Along with the development of tissue mimics, came engineered hardware, that provided the platform for cells and organs to behave in a physiological manner. The first organ-on-a-chip was developed in 2010 by researchers at the Wyss Institute for Biologically Inspired Engineering at Harvard University, the lung-on-a-chip. Like lung anatomy and function, the lung-on-a-chip mimicked the air–blood barrier, with mechanical stretching and relaxation to simulate breathing. This technology marked a breakthrough in the field by demonstrating the potential to more accurately model complex organ systems and their responses to various stimuli. This opens new avenues for studying both healthy organ physiology and disease and holds significant promise for more accurate drug testing. As a result, researchers began developing chips for other organs, including the heart, liver, kidney, intestine, and reproductive tract. Funding and interest from both academic institutions and the pharmaceutical industry spurred rapid advancements in the field.
In 2010, the Tissue Chip for Drug Screening initiative was launched as a 5-year collaborative effort between the National Institutes of Health (NIH), the Defense Advanced Research Projects Agency (DARPA), and the U.S. Food and Drug Administration (FDA). Overseen and coordinated by the National Center for Advancing Translational Sciences (NCATS), this program leverages multidisciplinary expertise from various partners to accelerate progress in tissue chip research. The initiative launched the era of tissue chips and continues to envision their use in studying health and disease, conducting clinical trials, exploring health in space, performing drug screening, and addressing the health needs of veterans (source: ncats.nih.gov).
A number of startups and companies have emerged with the goal of bringing OoC technology to the market. Prominent names in this field include Emulate, TissUse, and CN Bio Innovations. These firms have focused on enhancing the technology, increasing production capacity, and incorporating the chips into drug development processes. Regulatory bodies such as the FDA have begun recognizing the potential of OoC devices to improve drug testing and reduce reliance on animal models.
Partnerships between industry, academia, and regulatory bodies aim to establish standards and validate these technologies for various uses. Researchers are now pushing OoC technology forward by investigating multi-organ systems to understand systemic interactions and incorporating new materials and sensors to improve device functionality. These continuous advancements strive to make OoC devices more accessible, reliable, and versatile, holding promise for significant progress in toxicology, pharmacology, and personalized medicine.
Organs-on-a-chip technology in reproduction
Due to ethical concerns on research involving human subjects, particularly regarding the health and safety of both the developing fetus and the mother, investigation of human reproductive processes is a challenge (McKiever et al. 2020). These ethical concerns frequently result in the exclusion of pregnant women from clinical trials creating significant barriers to discovering safe and effective drugs for reproductive system diseases and fetal conditions. OoC hold immense potential by enabling the study of complex reproductive processes, replication of diseases, and evaluation of potential therapies. These devices represent a groundbreaking approach to exploring fertility, embryonic development, and reproductive health, simulating the dynamic environment of reproductive tissues.
The female reproductive tract
As part of the Tissue Chip Program, the EVATAR system, the first multi-organ microphysiological model of the female reproductive tract, was developed through a collaboration between reproductive biologists and engineers (Xiao et al. 2017) (Fig. 1a). To integrate five tissues into a single system, a practical and scalable microfluidic device was engineered. This device consisted of modules, similar to a well in a 24-well plate, with each module large enough to house 3D cultures and explants. Each module had flow ports to facilitate flow of media through all the modules. The flow of media was powered with electromagnetic actuated micropumps and built for precise management of flow rates.
Multiwell microfluidic platforms to study interactions of female reproductive tract tissues. (a) EVATAR, the first microphysiological system of the female reproductive tract was developed to promote interactions between multiple tissue types through microchannels connecting the wells. (b) LATTICE is a plug-and-play microfluidic platform that can accommodate up to eight large tissues or organ models. It builds on the capabilities of EVATAR but with simpler engineering and lower cost. Images of EVATAR and LATTICE were reproduced from Xiao et al. (2017) and Campo et al. (2023), respectively.
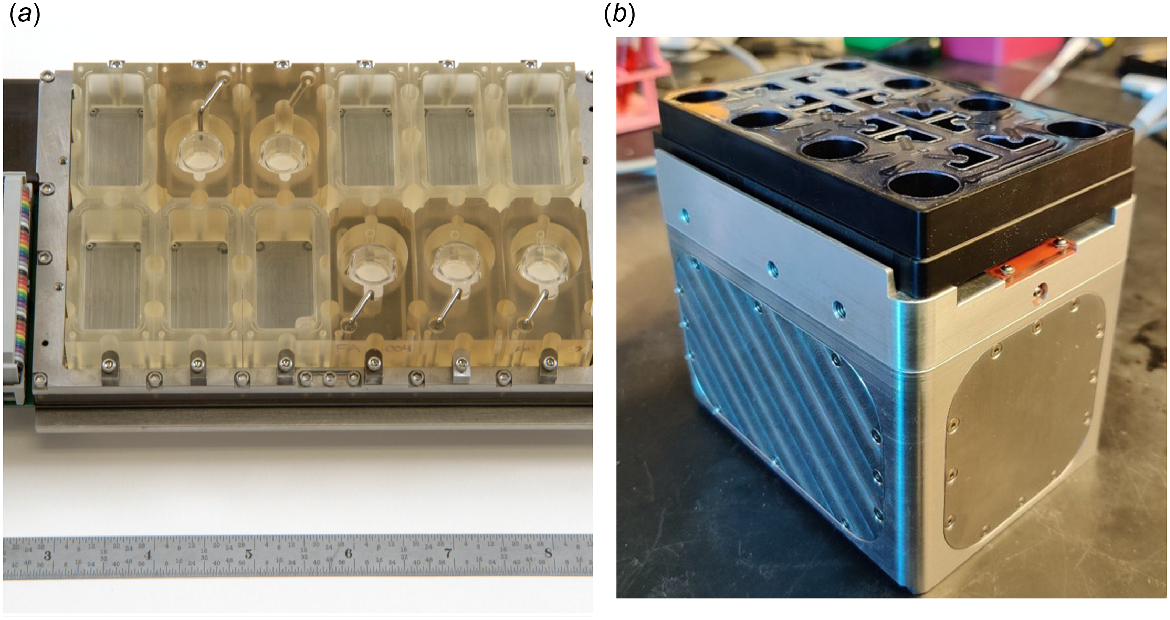
Within this platform, tissue mimics of the ovary, fallopian tube, endometrium, cervix, and non-reproductive tissue (liver) were connected with continuous media flow through each compartment. Mouse ovarian explants were treated with gonadotropic hormones (FSH and LH) to mimic a human menstrual cycle over 28 days. In response, estradiol and progesterone was secreted in a pattern resembling the human 28-day cycle, peaking during the follicular and luteal phases. Human fallopian tube explants, endometrial cells in decellularized scaffolds, human ectocervix explants, and human liver microtissues cultured together in the microfluidic device maintained their integrity and function throughout the 28-day period. These tissues exhibited robust responses to fluctuating levels of ovarian hormones, showing improvements compared to single cultures. Moreover, the use of a shared universal media highlighted the exchange of paracrine factors among the integrated tissues.
The success of this proof-of-concept device propelled the next phase of the study, which was to build a device that was more accessible in engineering and cost as well as to model a disease using the multi-organ format. A second-generation prototype was built named LATTICE (Campo et al. 2023), based on the design of the first platform (Fig. 1b). The device consisted of a power unit and a compatible culture plate with microfluidic channels that facilitated dynamic media mixing and flow between tissue wells. The device was made from biocompatible materials that did not absorb lipophilic compounds like hormones and drugs and the plate dimensions resembled a 24-well culture plate format.
Polycystic ovarian syndrome (PCOS) was the multi-organ reproductive disease chosen to be represented in LATTICE. A hyperandrogenic ovary was used as a source of high androgens, commonly found in women with PCOS, by increasing the FSH/LH ratio added to the mouse ovaries (Campo et al. 2023). Within the microfluidic device, the hyperandrogenic ovary promoted a significant downregulation of cilia beating in the fallopian tube explants. Ultimately, with the inclusion of other tissue types relevant to PCOS like adipose tissue, pancreatic islets, and endometrium, drugs can be screened and their impact on each tissue type can be assessed. As the multi-well platform is able to replenish nutrients and eliminate waste, thereby promoting prolonged physiologic tissue function, interactions between tissues types relevant to reproductive diseases like endometriosis, endometrial cancer, ovarian aging, and ovarian cancer, can be modeled using OoC, obtaining insights into new mechanisms of development and growth in a controlled setting.
Human cervix chips
To study the impact of infection on the lower female reproductive tract, one group developed a six-chamber organ-on-a-chip model (VCD-OOC) that mimicked the female reproductive tract during pregnancy (Tantengco et al. 2022). These chambers housed vaginal epithelial cells, cervical epithelial and stromal cells, and decidual cells. Using this model, this group demonstrated a similar response in the cells to a Ureaplasma parvum infection in the VCD-OOC as found in the animal model of infection.
Another group built a cervix chip to replicate the human cervical epithelial–stromal interface (Izadifar et al. 2024). Similar to the native cervix tissue, the cells in the cervix chip displayed a functional epithelial barrier, producing mucus and responding to hormones. Using this model, this study demonstrated responses to healthy versus dysbiotic microbial infection (specifically Lactobacillus crispatus and Gardnerella vaginalis) including tissue innate immune responses, barrier integrity, cell viability, proteomic profiles, and mucus composition, which were significantly different between the two microbial communities. This human cervix chip was shown to be a physiological representation of the cervix tissue and a useful model to study the cervix and associated diseases.
Senescence in ovarian aging
Cellular senescence has attracted significant attention due to its impact on overall health, with cooperative groups like the Cellular Senescence Network (SenNet) working to create an atlas of senescence across multiple tissues in human health (SenNet Consortium 2022; Suryadevara et al. 2024). Senescent cells are in a state of permanent proliferative arrest, accumulate with biological age, and create a microenvironment that can negatively affect tissue function, also called the bystander effect (Waaijer et al. 2012; Childs et al. 2015). This is due to the secreted factors of senescent cells, termed Senescence-Associated Secretory Phenotype (SASP), which include pro-inflammatory cytokines, chemokines, growth factors, proteases, and lipids. There is a growing body of research associating cellular senescence with ovarian aging (Secomandi et al. 2022; Wu et al. 2024); however, much remains to be learned to fully understand the precise role of senescence and the bystander effect on ovarian function and aging.
Ongoing studies of senescence in the ovary use the LATTICE platform which provides new features well-suited to understanding ovarian aging. Current in vitro methods involve the treatment of single cell type with specific inducers of senescence in static cultures (Huang et al. 2022; Veronesi et al. 2023) with waste accumulation potentially causing artificial responses. LATTICE continuously replenishes media, removing any stress responses that could confound data. The larger wells in the LATTICE plate also allow for the culture of multiple ovarian follicles at time. A customized agarose micromold was made to hold multiple multilayer secondary follicles in a single focal plane, allowing for constant time-lapse monitoring and automated analysis (Zaniker et al. 2024). Finally, the multi-well platform was designed to recapitulate organ–organ interactions in vitro with control of directionality of these interactions. The systemic effects of the aging ovary on other organ systems is an active area of research.
Embryo and fertility research
The OoC technology can enhance current embryo and fertility research by offering more precise, controlled, and scalable models. Studies in embryo implantation face significant challenges due to the complex and dynamic interactions between maternal and fetal tissues, as well as the ethical and practical limitations of in vivo research. One group used a microfluidic device to gain understanding on preeclampsia which is considered to be characterized by inadequate invasion of extravillous placental trophoblast cells into the uterine arteries (Abbas et al. 2017). This device was comprised of three channels with the fetal extravillous trophoblasts (EVTs) in the middle channel and flow of media creating cytokine gradients in the outer channels. This study demonstrated that granulocyte-macrophage colony-stimulating factor, a cytokine that is produced by activated decidual natural killer (NK) cells, stimulated the migration of EVTs with specific directionality.
Another study developed an intricate 3D maternal–fetal interface using OoC technology termed implantation-on-a-chip (Park et al. 2022). In this microengineered model, primary human EVTs isolated from clinical specimens, endometrial stromal fibroblasts from endometrial tissue, endothelial cells to mimic maternal vessels, and uterine NK cells isolated from endometrial tissues, were included in a device consisting of three parallel lanes, each for a specific cell type. The model demonstrated in vivo-like directional migration of EVTs towards microengineered maternal vessels, and showed that decidual cells could impact the invasion process. They were also successful in demonstrating the important role of uterine NK cells in influencing EVT invasion. Novel and important interactions and secreted factors were identified in this study, offering new insights into early human pregnancy dynamics.
Embryo development and screening
Improvements in preimplantation embryo culture, a critical component of assisted reproductive technology (ART), have lagged behind advancements in embryo selection (Vajta et al. 2021). The challenge lies in replicating complex in vivo conditions for in vitro embryo culture. Although human ART data is less conclusive, animal models, particularly bovines, are notably affected by these in vitro conditions. Differences in developmental competence, cryotolerance, and pregnancy outcomes are significant between embryos developed in vitro and those derived in vivo (Abe et al. 2002; Rizos et al. 2002a, 2002b). It was reported that the pregnancy and live birth rates of embryos produced in vitro remained lower compared to in vivo-produced embryos with pregnancy rates at 40.1% and 64.1%, respectively (Ealy et al. 2019). Despite these statistics, over 2 million bovine embryos were collected or produced in 2021, with in vitro-produced embryos surpassing those produced in vivo (Viana 2022).
To improve embryo quality in vitro, an in-depth understanding of the in vivo condition in the local environment of the developing embryo is needed, including the paracrine actions that occur between the embryo and the maternal tissues like the oviduct and the endometrium. Furthermore, biophysical forces during embryo transport in vivo can also be considered. Studies have mimicked the fluid mechanical stimulation of the embryos that occur in the oviduct using dynamic culture systems (Kim et al. 2009; Matsuura et al. 2010; Esteves et al. 2013; Gualtieri et al. 2024). Advanced culture systems such as these hold significant potential for enhancing embryo culture and ART success.
Improving ART in animals has profound impacts across various domains. It enables the selection and propagation of genetically superior animals, to enhance productivity and disease resistance. ART techniques also play a crucial role in conserving endangered species and preserving valuable genetic diversity. Moreover, ART can increase the efficiency of breeding programs, which in turn boosts agricultural productivity and economic returns. Beyond economic benefits, advancements in ART contribute to broader research in reproductive biology and medicine, benefiting animal welfare. By offering alternatives to traditional breeding methods, ART can also improve ethical considerations in animal husbandry, promoting sustainable practices and reducing environmental impact.
Perspectives and future directions
The future potential of OoC technology in reproductive health is immense, promising groundbreaking advancements in our understanding and treatment of reproductive issues. These sophisticated multi-organ chips allow for the simultaneous study of different tissues and organs, accurately mimicking the complex interactions and hormonal dynamics crucial to reproduction. They offer unprecedented insights into reproductive diseases and the testing of new therapies, potentially revolutionizing personalized healthcare by tailoring models specific to each patient’s reproductive system. OoC technology also holds promise for deepening our understanding of early embryonic development and implantation processes. By creating more realistic models of embryo–maternal interactions, researchers can unravel key factors influencing successful pregnancy outcomes and refine ART. This could also illuminate how environmental pollutants and medications affect early pregnancy stages, informing regulatory decisions and healthcare strategies.
In animal reproduction, OoC technology stands to transform veterinary science and breeding practices. Tailored multi-organ chips that replicate diverse animal reproductive systems could vastly improve our understanding of reproduction, leading to enhanced breeding techniques and genetic outcomes in livestock. Innovations in embryo development using OoC could minimize unsuccessful attempts and reduce waste, while offering insights into the effects of environmental factors, medications, and supplements on animal reproduction. Importantly, OoC models provide ethical alternatives to traditional methods and could help evaluate the reproductive impacts of gene editing technologies and epigenetic changes in farm animals. Automated OoC systems also promise to make these technologies more accessible and scalable for both research and clinical applications, potentially revolutionizing reproductive health across species.
Despite the promising potential, challenges hinder the vast application of OoC technology. One significant issue is the steep costs, not just in creating these sophisticated systems but also in maintaining them over time. For many labs, the financial burden can be a serious barrier. Additionally, organs are incredibly complex, with various cell types working together in ways that are difficult to mimic accurately ex vivo. An example is the need for immune and endothelial cells in endometrial organoids as all cell types work together in the endometrium in response to cycling hormones. Moreover, this challenge can affect how well these models perform in drug testing or disease research. Scaling up these platforms to accommodate large-scale studies is another major obstacle. Recognizing these limitations is critical to driving the urgent research and development efforts needed to overcome these challenges, ensuring that OoC become a widely accessible tool in biomedical research.
In conclusion, the potential uses of OoC technology in both human and animal reproduction are vast and significant. By providing detailed and controlled models of reproductive systems, OoC technology stands poised to transform reproductive health research towards a more sustainable and ethical future.
Data availability
All data in this review are published as cited. Specific data can be found in the cited papers.
Acknowledgements
The author acknowledges Drs Francesca Duncan and Hannes Campo for providing the information on ovarian aging and senescence, which is an active and important area of research. Their studies are funded by NIH Common Fund’s SenNet program (UH3CA268105) and the Global Consortium for Reproductive Longevity and Equality postdoctoral grant (GRCLE-2123).
References
Abbas Y, Oefner CM, Polacheck WJ, Gardner L, Farrell L, Sharkey A, Kamm R, Moffett A, Oyen ML (2017) A microfluidics assay to study invasion of human placental trophoblast cells. Journal of The Royal Society Interface 14, 20170131.
| Crossref | Google Scholar |
Abe H, Yamashita S, Satoh T, Hoshi H (2002) Accumulation of cytoplasmic lipid droplets in bovine embryos and cryotolerance of embryos developed in different culture systems using serum-free or serum-containing media. Molecular Reproduction and Development 61, 57-66.
| Crossref | Google Scholar | PubMed |
Alzamil L, Nikolakopoulou K, Turco MY (2021) Organoid systems to study the human female reproductive tract and pregnancy. Cell Death & Differentiation 28, 35-51.
| Crossref | Google Scholar | PubMed |
Bläuer M, Heinonen PK, Martikainen PM, Tomás E, Ylikomi T (2005) A novel organotypic culture model for normal human endometrium: regulation of epithelial cell proliferation by estradiol and medroxyprogesterone acetate. Human Reproduction 20, 864-871.
| Crossref | Google Scholar | PubMed |
Boretto M, Cox B, Noben M, Hendriks N, Fassbender A, Roose H, Amant F, Timmerman D, Tomassetti C, Vanhie A, Meuleman C, Ferrante M, Vankelecom H (2017) Development of organoids from mouse and human endometrium showing endometrial epithelium physiology and long-term expandability. Development 144, 1775-1786.
| Crossref | Google Scholar | PubMed |
Cadena I, Chen A, Arvidson A, Fogg KC (2021) Biomaterial strategies to replicate gynecological tissue. Biomaterials Science 9, 1117-1134.
| Crossref | Google Scholar | PubMed |
Campo H, Murphy A, Yildiz S, Woodruff T, Cervelló I, Kim JJ (2020) Microphysiological Modeling of the Human Endometrium. Tissue Engineering Part A 26, 759-768.
| Crossref | Google Scholar | PubMed |
Campo H, Zha D, Pattarawat P, Colina J, Zhang D, Murphy A, Yoon J, Russo A, Rogers HB, Lee HC, Zhang J, Trotter K, Wagner S, Ingram A, Pavone ME, Dunne SF, Boots CE, Urbanek M, Xiao S, Burdette JE, Woodruff TK, Kim JJ (2023) A new tissue-agnostic microfluidic device to model physiology and disease: the lattice platform. Lab on a Chip 23, 4821-4833.
| Crossref | Google Scholar | PubMed |
Childs BG, Durik M, Baker DJ, van Deursen JM (2015) Cellular senescence in aging and age-related disease: from mechanisms to therapy. Nature Medicine 21, 1424-1435.
| Crossref | Google Scholar | PubMed |
Eagle H (1959) Amino acid metabolism in mammalian cell cultures. Science 130, 432-437.
| Crossref | Google Scholar | PubMed |
Ealy AD, Wooldridge LK, McCoski SR (2019) BOARD INVITED REVIEW: Post-transfer consequences of in vitro-produced embryos in cattle. Journal of Animal Science 97, 2555-2568.
| Crossref | Google Scholar | PubMed |
Esteves TC, van Rossem F, Nordhoff V, Schlatt S, Boiani M, Le Gac S (2013) A microfluidic system supports single mouse embryo culture leading to full-term development. RSC Advances 3, 26451-26458.
| Crossref | Google Scholar |
Fitzgerald HC, Schust DJ, Spencer TE (2021) In vitro models of the human endometrium: evolution and application for women’s health. Biology of Reproduction 104, 282-293.
| Crossref | Google Scholar | PubMed |
Gualtieri R, De Gregorio V, Candela A, Travaglione A, Genovese V, Barbato V, Talevi R (2024) In vitro culture of mammalian embryos: is there room for improvement? Cells 13, 996.
| Crossref | Google Scholar |
Heremans R, Jan Z, Timmerman D, Vankelecom H (2021) Organoids of the female reproductive tract: innovative tools to study desired to unwelcome processes. Frontiers in Cell and Developmental Biology 9, 661472.
| Crossref | Google Scholar | PubMed |
Huang W, Hickson LJ, Eirin A, Kirkland JL, Lerman LO (2022) Cellular senescence: the good, the bad and the unknown. Nature Reviews Nephrology 18, 611-627.
| Crossref | Google Scholar | PubMed |
Izadifar Z, Cotton J, Chen S, Horvath V, Stejskalova A, Gulati A, Logrande NT, Budnik B, Shahriar S, Doherty ER, Xie Y, To T, Gilpin SE, Sesay AM, Goyal G, Lebrilla CB, Ingber DE (2024) Mucus production, host-microbiome interactions, hormone sensitivity, and innate immune responses modeled in human cervix chips. Nature Communications 15, 4578.
| Crossref | Google Scholar | PubMed |
Kim MS, Bae CY, Wee G, Han Y-M, Park J-K (2009) A microfluidic in vitro cultivation system for mechanical stimulation of bovine embryos. Electrophoresis 30, 3276-3282.
| Crossref | Google Scholar | PubMed |
Lessey BA, Young SL (2019) What exactly is endometrial receptivity? Fertility and Sterility 111, 611-617.
| Crossref | Google Scholar | PubMed |
Matsuura K, Hayashi N, Kuroda Y, Takiue C, Hirata R, Takenami M, Aoi Y, Yoshioka N, Habara T, Mukaida T, Naruse K (2010) Improved development of mouse and human embryos using a tilting embryo culture system. Reproductive BioMedicine Online 20, 358-364.
| Crossref | Google Scholar | PubMed |
McKiever M, Frey H, Costantine MM (2020) Challenges in conducting clinical research studies in pregnant women. Journal of Pharmacokinetics and Pharmacodynamics 47, 287-293.
| Crossref | Google Scholar | PubMed |
Murphy AR, Campo H, Kim JJ (2022) Strategies for modelling endometrial diseases. Nature Reviews Endocrinology 18, 727-743.
| Crossref | Google Scholar | PubMed |
Park JY, Mani S, Clair G, Olson HM, Paurus VL, Ansong CK, Blundell C, Young R, Kanter J, Gordon S, Yi AY, Mainigi M, Huh DD (2022) A microphysiological model of human trophoblast invasion during implantation. Nature Communications 13, 1252.
| Crossref | Google Scholar | PubMed |
Rawlings TM, Makwana K, Taylor DM, Molè MA, Fishwick KJ, Tryfonos M, Odendaal J, Hawkes A, Zernicka-Goetz M, Hartshorne GM, Brosens JJ, Lucas ES (2021) Modelling the impact of decidual senescence on embryo implantation in human endometrial assembloids. eLife 10, e69603.
| Crossref | Google Scholar |
Rizos D, Fair T, Papadopoulos S, Boland MP, Lonergan P (2002a) Developmental, qualitative, and ultrastructural differences between ovine and bovine embryos produced in vivo or in vitro. Molecular Reproduction and Development 62, 320-327.
| Crossref | Google Scholar | PubMed |
Rizos D, Ward F, Duffy P, Boland MP, Lonergan P (2002b) Consequences of bovine oocyte maturation, fertilization or early embryo development in vitro versus in vivo: implications for blastocyst yield and blastocyst quality. Molecular Reproduction and Development 61, 234-248.
| Crossref | Google Scholar | PubMed |
Schafer WR, Fischer L, Roth K, Jullig AK, Stuckenschneider JE, Schwartz P, Weimer M, Orlowska-Volk M, Hanjalic-Beck A, Kranz I, Deppert WR, Zahradnik HP (2011) Critical evaluation of human endometrial explants as an ex vivo model system: a molecular approach. Molecular Human Reproduction 17, 255-265.
| Crossref | Google Scholar | PubMed |
Secomandi L, Borghesan M, Velarde M, Demaria M (2022) The role of cellular senescence in female reproductive aging and the potential for senotherapeutic interventions. Human Reproduction Update 28, 172-189.
| Crossref | Google Scholar | PubMed |
SenNet Consortium (2022) NIH SenNet Consortium to map senescent cells throughout the human lifespan to understand physiological health. Nature Aging 2, 1090-1100.
| Crossref | Google Scholar |
Song Y, Fazleabas AT (2021) Endometrial organoids: a rising star for research on endometrial development and associated diseases. Reproductive Sciences 28, 1626-1636.
| Crossref | Google Scholar | PubMed |
Suryadevara V, Hudgins AD, Rajesh A, Pappalardo A, Karpova A, Dey AK, Hertzel A, Agudelo A, Rocha A, Soygur B, Schilling B, Carver CM, Aguayo-Mazzucato C, Baker DJ, Bernlohr DA, Jurk D, Mangarova DB, Quardokus EM, Enninga EAL, Schmidt EL, Chen F, Duncan FE, Cambuli F, Kaur G, Kuchel GA, Lee G, Daldrup-Link HE, Martini H, Phatnani H, Al-Naggar IM, Rahman I, Nie J, Passos JF, Silverstein JC, Campisi J, Wang J, Iwasaki K, Barbosa K, Metis K, Nernekli K, Niedernhofer LJ, Ding L, Wang L, Adams LC, Ruiyang L, Doolittle ML, Teneche MG, Schafer MJ, Xu M, Hajipour M, Boroumand M, Basisty N, Sloan N, Slavov N, Kuksenko O, Robson P, Gomez PT, Vasilikos P, Adams PD, Carapeto P, Zhu Q, Ramasamy R, Perez-Lorenzo R, Fan R, Dong R, Montgomery RR, Shaikh S, Vickovic S, Yin S, Kang S, Suvakov S, Khosla S, Garovic VD, Menon V, Xu Y, Song Y, Suh Y, Dou Z, Neretti N (2024) SenNet recommendations for detecting senescent cells in different tissues. Nature Reviews Molecular Cell Biology 2024.,.
| Crossref | Google Scholar |
Tantengco OAG, Richardson LS, Radnaa E, Kammala AK, Kim S, Medina PMB, Han A, Menon R (2022) Modeling ascending Ureaplasma parvum infection through the female reproductive tract using vagina-cervix-decidua-organ-on-a-chip and feto-maternal interface-organ-on-a-chip. The FASEB Journal 36, e22551.
| Crossref | Google Scholar | PubMed |
Turco MY, Gardner L, Hughes J, Cindrova-Davies T, Gomez MJ, Farrell L, Hollinshead M, Marsh SGE, Brosens JJ, Critchley HO, Simons BD, Hemberger M, Koo B-K, Moffett A, Burton GJ (2017) Long-term, hormone-responsive organoid cultures of human endometrium in a chemically defined medium. Nature Cell Biology 19, 568-577.
| Crossref | Google Scholar | PubMed |
Vajta G, Parmegiani L, Machaty Z, Chen WB, Yakovenko S (2021) Back to the future: optimised microwell culture of individual human preimplantation stage embryos. Journal of Assisted Reproduction and Genetics 38, 2563-2574.
| Crossref | Google Scholar | PubMed |
Vasil IK (2008) A history of plant biotechnology: from the Cell Theory of Schleiden and Schwann to biotech crops. Plant Cell Reports 27, 1423-1440.
| Crossref | Google Scholar | PubMed |
Veronesi F, Contartese D, Di Sarno L, Borsari V, Fini M, Giavaresi G (2023) In vitro models of cell senescence: a systematic review on musculoskeletal tissues and cells. International Journal of Molecular Sciences 24, 15617.
| Crossref | Google Scholar |
Viana JH (2022) 2021 statistics of embryo production and transfer in domestic farm animals. Embryo Technology Newsletter 40, 22-40.
| Google Scholar |
Waaijer MEC, Parish WE, Strongitharm BH, van Heemst D, Slagboom PE, de Craen AJM, Sedivy JM, Westendorp RGJ, Gunn DA, Maier AB (2012) The number of p16INK4a positive cells in human skin reflects biological age. Aging Cell 11, 722-725.
| Crossref | Google Scholar | PubMed |
Wiwatpanit T, Murphy AR, Lu Z, Urbanek M, Burdette JE, Woodruff TK, Kim JJ (2020) Scaffold-free endometrial organoids respond to excess androgens associated with polycystic ovarian syndrome. The Journal of Clinical Endocrinology & Metabolism 105, 769-780.
| Crossref | Google Scholar |
Wu M, Tang W, Chen Y, Xue L, Dai J, Li Y, Zhu X, Wu C, Xiong J, Zhang J, Wu T, Zhou S, Chen D, Sun C, Yu J, Li H, Guo Y, Huang Y, Zhu Q, Wei S, Zhou Z, Wu M, Li Y, Xiang T, Qiao H, Wang S (2024) Spatiotemporal transcriptomic changes of human ovarian aging and the regulatory role of FOXP1. Nature Aging 4, 527-545.
| Crossref | Google Scholar | PubMed |
Xiao S, Coppeta JR, Rogers HB, Isenberg BC, Zhu J, Olalekan SA, Mckinnon KE, Dokic D, Rashedi AS, Haisenleder DJ, Malpani SS, Arnold-Murray CA, Chen K, Jiang M, Bai L, Nguyen CT, Zhang J, Laronda MM, Hope TJ, Maniar KP, Pavone ME, Avram MJ, Sefton EC, Getsios S, Burdette JE, Kim JJ, Borenstein JT, Woodruff TK (2017) A microfluidic culture model of the human reproductive tract and 28-day menstrual cycle. Nature Communications 8, 14584.
| Crossref | Google Scholar | PubMed |
Zaniker EJ, Hashim PH, Gauthier S, Ankrum JA, Campo H, Duncan FE (2024) 3D-printed agarose micromold supports scaffold-free mouse ex vivo follicle growth, ovulation, and luteinization. Bioengineering 11, 719.
| Crossref | Google Scholar |