Waterbird assemblages of Australia’s largest east-coast wetland complex: environmental determinants of spatial and temporal distribution
Richard G. Pearson
A
Abstract
The extensive Burdekin–Townsville seasonal freshwater wetlands in the Queensland tropics (adjacent to an intertidal Ramsar site) support important waterbird assemblages within and downstream of the Burdekin–Haughton irrigation area. Little evidence is available of the environmental drivers of these assemblages, possibly including the extensive development of irrigated agriculture.
We described the composition and dynamics of freshwater waterbird assemblages to identify their environmental determinants and underpin conservation management.
We used up to four decades’ worth of records of waterbird assemblages, and collected biophysical data from 57 sites and water quality data for 32 sites. We predicted that these measured variables would determine assemblage composition, thereby elucidating the environmental drivers of the assemblages.
In total, 100 waterbird and shorebird species were recorded. Individual samples were strongly nested. Species richness increased with area but density decreased. Richness had a humped relationship with water depth. Linear modelling identified mostly weak relationships between species, assemblages and environmental variables (e.g. nutrients). Abundances mostly increased during the dry season but irrigation tailwater reduced seasonal variation in some wetlands. No apparent change in common species’ assemblages was evident over four decades.
The wetlands provide extensive, diverse habitats for rich waterbird assemblages, despite impacts of agricultural development. Impacts on waterbirds appear to be buffered by the extent and complexity of the wetland mosaic that includes modified wetlands.
Holistic, active management is required to ensure the long-term conservation of habitats for waterbirds and other biota in the Burdekin–Townsville seasonal freshwater wetlands. Inclusion of these freshwater wetlands in the Ramsar site would be beneficial.
Keywords: agricultural impacts, environmental drivers, floodplain wetlands, management, temporal change, tropics, waterbirds, water quality.
Introduction
Globally, freshwater ecosystems have high biodiversity relative to size but are disproportionately threatened by human activity (Tickner et al. 2020) that results in extensive losses of wetland area (Davidson 2014; Fluet-Chouinard et al. 2023) and climate change (Grieger et al. 2021; Osland et al. 2022). Ecosystem services that wetlands provide are important and highly valued (Whiteoak and Binney 2012; de Groot et al. 2018; Pearson et al. 2021), especially in coastal wetlands (Davidson et al. 2019), but suffer from limited information on environmental drivers of community structure and function. Although Australia is the driest inhabited continent, the country has extensive coastal wetlands that are subject to a variety of environmental pressures (Bino et al. 2016; Grieger et al. 2021). Laurance et al. (2011) included coastal floodplains and wetlands as one of the ‘10 Australian ecosystems most vulnerable to tipping points’, partly because of the restricted and fragmented nature of these ecosystems but also environmental constraints and proximity to areas in which land-use change is implemented. Threats include sea-level rise, plant invasion, hydrological change, habitat loss, pollution and altered fire regimes.
Wetlands may be clustered, patchy or dispersed habitats varying greatly in size and permanence. Areas of intensive land development are typically located on coastal floodplains, making the associated wetlands particularly vulnerable to land-use impacts owing to a combination of upstream (development) and downstream (rising sea level) pressures (Bernhardt 2022). For example, in the Great Barrier Reef catchment in north-eastern Queensland, impacts on wetlands accrue from agriculture, infrastructure and water management, especially through changes in hydrological processes, habitat loss, water-quality deterioration and weed invasion (GBRMPA 2013; Davis et al. 2014; Adame et al. 2019; Pearson et al. 2019), although there is little direct evidence concerning waterbirds. Wetlands are an important focus of catchment management because of the role these ecosystems play in local hydrological processes, connectivity to downstream environments, ecosystem services and biodiversity, and appropriate management is required to sustain these values (Adame et al. 2019).
Waterbirds are distinct components of the high levels of biodiversity that wetlands support, such that they have been used to provide a globally applicable indication of wetland condition in Australia (Kingsford 1999), Argentina (Sica et al. 2020) and the United States (Stevens and Conway 2020), for example. Australia’s waterbirds can be highly nomadic, reflecting the variability of the climate, and exploit wetlands as these become available (Halse et al. 2005; Kingsford et al. 2010), although groundwater-fed coastal wetlands may provide longer-duration or permanent habitat. All wetlands may be threatened by loss of habitat as a result of developments of water resources, flood mitigation and irrigation (Davis et al. 2014; Tibby et al. 2019; Caley et al. 2022). There is a need to understand the values of wetlands and factors that determine condition to guide practical management strategies (Grieger et al. 2021).
We assembled data from a series of studies of waterbird assemblages in the coastal floodplain wetlands of the Burdekin River and associated drainages. This floodplain system and the contiguous irrigation area are the largest on the Australian east coast (GBRMPA 2013; Davis et al. 2014). The floodplain abuts the intertidal Bowling Green Bay Ramsar wetlands and other wetlands of national importance that are listed in the National Directory of Important Wetlands (DIWA) (Australian Government 2024). We aimed to determine the major drivers of waterbird assemblages and any change in these due to agricultural development on the floodplain, especially from expansion of the irrigation area following the 1987 completion of the Burdekin Falls dam. The dam supplies irrigation water via artificial and natural channels, leading to tailwater supply to drains, natural channels and wetlands. Changes in hydrological processes, extensive land clearing and agricultural expansion have led to enhanced concentrations of nutrients and sediments, and have facilitated weed invasion and other impacts (GBRMPA 2013; Davis et al. 2014).
We used data derived from two studies of multiple sites in the 1990s and early 2000s, broad-scale records from the 1990s to 2021, and regular surveys of two important sites between 2012 and 2021. We aimed to identify major factors affecting waterbird assemblages in this complex wetland environment to underpin conservation management planning and activity. Specifically, we hypothesised that:
waterbird assemblages are generally similar among the wetlands surveyed;
waterbird assemblages vary spatially with wetland character, as defined by 32 measured variables encompassing the timing of sampling (year, month and season), wetland dimensions, hydrological processes, agricultural or urban disturbance, weed growth and water quality (e.g. Ma et al. 2010);
abundance of waterbird populations and assemblage composition vary seasonally, with maxima in the wet season (Kingsford et al. 2010);
assemblages vary annually according to antecedent rainfall (Kingsford et al. 2010; Kingsford 2013; Caley et al. 2022); and
assemblages have changed over several decades as a result of floodplain development for agriculture (clearing, levelling, irrigation supply and drainage) (Davis et al. 2014).
Methods
We focused on the waterbird assemblages (Anseriformes, Podicepiformes, Gruiformes, Pelecaniformes and Charadriiformes) of the Burdekin–Townsville wetlands (Fig. 1, Supplementary material Table S1). Common and scientific names are provided in Table 1 (more common species) and Table S2 (all species); otherwise, we only use common names. We also refer to the Charadriiformes as shorebirds, following convention (e.g. Driscoll et al. 2012).
Google Earth© image of the Burdekin–Townsville study area indicating the locations of sampling sites (Survey 1, white dots; Survey 2, white diamonds), Ramsar tidal wetlands, main towns and rivers, and other points of interest. Sugarcane is coloured mid-green. Forest is dark green and grassland/woodland is pale green or brown. Inset shows location in north-eastern Queensland (approximately 19.5°S, 147.2°E). W, Wongaloo; HL, Horseshoe Lagoon.
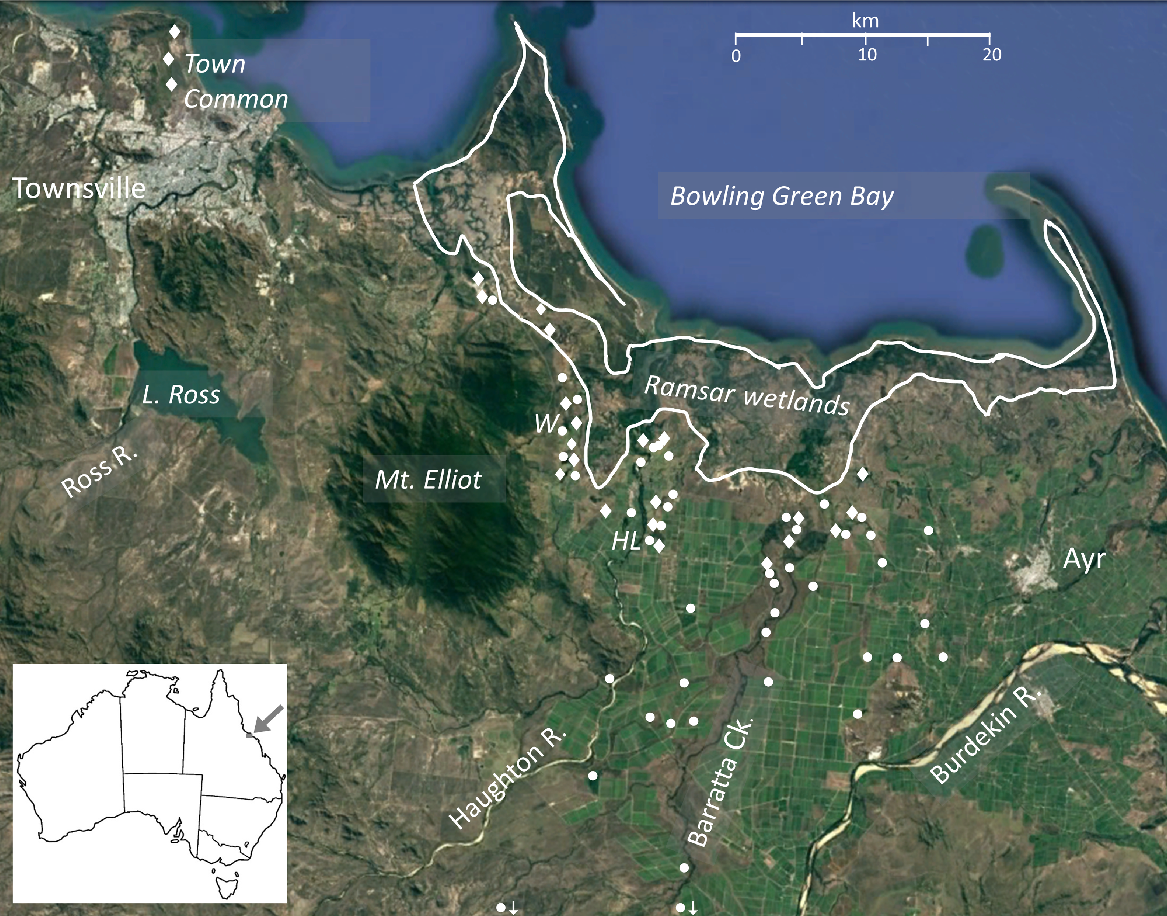
Surveys and no. of samples (times × sites) | Diet | |||||||||
---|---|---|---|---|---|---|---|---|---|---|
BDATA | WONG | HL | UNSW | DRISC | S1 and 2 | S1 and 2 | ||||
(4235) | (234) | (26) | (121) | (4) | (198) | Mass | ||||
Magpie Goose | Anseranas semipalmata | 43.7 | 44.2 | 42.3 | 38.3 | 48.6 | 33.9 | 4193 | SFCA | |
Pacific Black Duck | Anas superciliosa | 9.30 | 9.85 | 2.25 | 6.61 | 14.2 | 14.0 | 758 | SFA | |
Plumed Whistling-Duck | Dendrocygna eytoni | 0.07 | 0 | 0 | 32.2 | 1.18 | 18.6 | 697 | SFAA | |
Hardhead | Aythya australis | 2.51 | 2.94 | 36.5 | 0.81 | 2.82 | 8.48 | 377 | SFA | |
Yellow-billed Spoonbill | Platalea flavipes | 0.24 | 0.27 | 0 | 0.12 | 0.05 | 3.04 | 291 | A | |
Brolga | Antigone rubicunda | 2.78 | 1.35 | 0.01 | 1.32 | 0.43 | 0.74 | 237 | SFCTA | |
Royal Spoonbill | Platalea regia | 1.08 | 1.11 | 0.13 | 0.50 | 2.65 | 2.52 | 223 | A | |
Australian Pelican | Pelecanus conspicillatus | 1.27 | 1.35 | 1.35 | 0.62 | 0.38 | 0.65 | 178 | TA | |
Australian White Ibis | Threskiornis moluccus | 0.36 | 0.38 | 0.06 | 0.27 | 0.18 | 1.65 | 153 | TA | |
Straw-necked Ibis | Threskiornis spinicollis | 0.74 | 0.85 | 0.01 | 0.09 | 0.02 | 2.00 | 138 | T | |
Black Swan | Cygnus atratus | 9.51 | 10.6 | 5.12 | 6.71 | 5.18 | 0.31 | 91 | F | |
Little Black Cormorant | Phalacrocorax sulcirostris | 0.31 | 0.30 | 1.41 | 0.11 | 1.99 | 1.27 | 56 | A | |
Great Egret | Ardea alba | 0.59 | 0.56 | 0.13 | 0.11 | 1.83 | 0.71 | 34 | TA | |
Black-winged Stilt | Himantopus leucocephalus | 3.80 | 3.94 | 0.04 | 0.19 | 2.18 | 3.54 | 32 | A | |
Masked Lapwing | Vanellus miles | 0.70 | 0.72 | 0.33 | 0.33 | 0.90 | 1.91 | 31 | TA | |
Australasian Darter | Anhinga novaehollandiae | 0.05 | 0.06 | 1.29 | 0.04 | 0.47 | 0.33 | 26 | A | |
Pied Cormorant | Phalacrocorax varius | 0.01 | 0.02 | 0.15 | 0.07 | 0.01 | 0.01 | 20 | A | |
Grey Teal | Anas gracilis | 18.4 | 17.6 | 0.43 | 4.38 | 0.88 | 0.65 | 16 | SK | |
Australian Pelican | Pelecanus conspicillatus | 1.27 | 1.35 | 1.35 | 0.62 | 0.38 | 0.65 | 16 | TA | |
Australasian Grebe | Tachybaptus novaehollandiae | 0.09 | 0.10 | 1.67 | 0 | 1.34 | 1.55 | 15 | A | |
White-faced Heron | Egretta novaehollandiae | 0.12 | 0.09 | 0.02 | 0.20 | 0.15 | 0.51 | 15 | TA | |
Black-necked Stork | Ephippiorhynchus asiaticus | 0.08 | 0.09 | 0.03 | 0.02 | 0.07 | 0.05 | 7 | A | |
Whiskered Tern | Chlidonias hybrida | 0.23 | 0.21 | 2.87 | 0.31 | 1.92 | 1.32 | 6 | TA | |
Intermediate Egret | Ardea intermedia | 0.57 | 0.31 | 0.09 | 0 | 3.30 | 0.28 | 6 | TA | |
Little Pied Cormorant | Microcarbo melanoleucos | 0.09 | 0.10 | 0.34 | 0.01 | 0.33 | 0.16 | 6 | A | |
Black-fronted Dotterel | Elseyornis melanops | 0.07 | 0.08 | 0.01 | 0 | 0.06 | 0.96 | 2 | TA | |
Comb-crested Jacana | Irediparra gallinacea | 0.08 | 0.06 | 0.77 | 0 | 0.77 | 0.09 | 1.1 | STA | |
Glossy Ibis | Plegadis falcinellus | 0.42 | 0.36 | 0.02 | 0.10 | 0.19 | 0.04 | 1.1 | SA | |
Little Egret | Egretta garzetta | 0.19 | 0.20 | 0.02 | 0 | 0.29 | 0.04 | 0.7 | TA | |
Cattle Egret | Bubulcus ibis | 0.88 | 0.79 | 0.13 | 0 | 0.67 | 0.04 | 0.6 | T | |
Wandering Whistling-Duck | Dendrocygna arcuata | 0.36 | 0.04 | 0.02 | 6.23 | 3.75 | 0.04 | 0.5 | SFA | |
Cotton Pygmy-goose | Nettapus coromandelianus | 0 | 0 | 0.48 | 0 | 0.35 | <0.1 | <0.5 | SFA | |
Eurasian Coot | Fulica atra | 0 | 0 | 1.98 | 0 | 0 | <0.1 | <0.5 | F | |
Great Cormorant | Phalacrocorax carbo | 0 | 0 | 0.04 | 0 | 0 | <0.1 | <0.5 | A | |
Red-capped Plover | Charadrius ruficapillus | 0.04 | 0.05 | 0 | 0 | 0 | <0.1 | <0.5 | A | |
White-necked Heron | Ardea pacifica | 0.29 | 0.32 | 0.01 | 0 | 0.14 | <0.1 | <0.5 | TA | |
Total richness (number of species on database (Table S2) | 100 | 61 | 40 | 30 | 43 | 47 |
Surveys include: BirdLife Australia (2022) (BDATA), Wongaloo (WONG) and Horseshoe Lagoon (HL); University of New South Wales (UNSW); Driscoll et al. (2012) (DRISC); and this study (Surveys 1 and 2). Numbers of samples (in parentheses) include times and sites. See Table S2 for full species list. Diet summary from Garnett et al. (2015): S, seeds; F, foliage; C, corms and tubers; T and A, respectively, terrestrial and aquatic invertebrates and vertebrates.
Sampling area and sites
The climate of the study area is seasonal tropical, with mean minimum and maximum temperatures at Ayr (Fig. 1) of 21.9°C (June) and 35.3°C (February) respectively (Australian Bureau of Meteorology data, 1952–2022). Rainfall is also seasonal, with a summer wet season (here defined as January to April), spring dry season (September to December) and a transitional period overlapping the wet and dry seasons during the period of decline in freshwater flows (May to August) (Fig. S1). Annual rainfall in Ayr (mean 1887–2022, 1056 mm) is highly variable (e.g. during the study period, 366 mm in 1991 and 1363 mm in 2021).
The study was located in the ‘Burdekin–Townsville Coastal Aggregation’ (Queensland Government 2024), and on the floodplain of the Burdekin–Haughton Water Supply System (BHWSS) and irrigation scheme. This area covers ~1140 km2, including 93 km2 of freshwater wetlands. The aggregation extends from Ayr north-eastwards to the coast and north-westwards to Townsville and includes the Burdekin–Haughton irrigation area (800 km2) which, despite wetland losses, still includes many wetlands. This area also includes the mostly intertidal Bowling Green Bay Ramsar wetlands (GBRMPA 2013), which were excluded from our study (Fig. 1). This complex represents one of the largest concentrations of high-value freshwater, estuarine and marine wetlands in Australia (Davis et al. 2014; NQ Dry Tropics 2016).
The floodplain is fed naturally by shallow aquifers, local rainfall and runoff, and overbank flows of the Burdekin River (rarely; Alexander et al. 1999) and Haughton River, resulting in the formation of numerous seasonal swamps and shallow lakes, deep perennial riverine lagoons and extensive coastal sedgelands (Fig. S1) (Tait and Veitch 2007; GBRMPA 2013; Queensland Government 2024). The water table is close to ground level on a large proportion of the floodplain. Patches of woodland characterised by Melaleuca, Pandanus and Livistona species occur on raised ground and, along the natural drainage channels, species-rich gallery forest or mangrove forest is prevalent, except where channels have been cleared or burned. The wetlands are of significant conservation value, providing habitat for diverse plant, invertebrate and fish assemblages (Connolly et al. 2012; Pearson et al. 2019), and many bird species, some of which occur in nationally significant numbers (Driscoll et al. 2012) or are variously threatened (e.g. 17 species of shore or wetland species (Table S2; Garnett and Baker 2021)).
Agricultural development on the floodplain historically depended on rainfall and water derived from wetlands and groundwater (from the 1870s), and subsequently directly from the Burdekin River. The Burdekin Falls dam was completed in 1987 and currently supplies both the subsequently developed irrigation area and groundwater recharge. Downstream of the dam, water is delivered via the river, from which it is pumped into irrigation channels and the Haughton River, and distributed as required to crops and to recharge pits on the lower floodplain. Irrigation runoff enters drains and eventually natural waterways, thereby boosting groundwater levels and providing water to many wetlands during the dry season (Queensland Government 2018). The dam facilitated growth of the irrigation area by ~40,000 ha to a total of 80,000 ha, providing ~25% of Australia’s sugar crop (Fig. S2) (GBRMPA 2013). The dam overflows during most years but this does not affect the frequency of rare overbank flooding to the floodplain of the BHWSS (Alexander et al. 1999; Cooper et al. 2016; Queensland Government 2018).
Development of the irrigation area for sugarcane production involved clearing of vegetation, filling of shallow wetlands, levelling of land and establishment of irrigation infrastructure. According to GBRMPA (2013), this has led to as much as 57% loss of original lacustrine wetlands and 40% loss of palustrine wetlands. Conversely, extensive development of irrigation channels and drains (387 km of channels and 366 km of drains; Sunwater 2024), weirs, balancing storages and ponded pastures has maintained the total area of wetlands at ~106% of the pre-clearance area. There were 3.8× and 1.8× increases in the areas of highly modified lacustrine and palustrine wetlands since pre-clearing of farmland respectively (Queensland Government 2024) (Table S3).
Impacts or threats to wetlands (Table S4) include changes to hydrological processes, with potentially positive or negative effects on waterbirds depending on the associated reliance on seasonal or permanent wetlands; changes to water quality that may increase productivity but deleteriously alter aquatic assemblages; loss of riparian vegetation that provides nesting or roosting sites; establishment of invasive weeds, including water hyacinth (Eichhornia crassipes), para grass (Urochloa mutica) and hymenachne (Hymenachne amplexicaulis) (the latter two species having been introduced to ponded pastures) that may choke waterways and impede reaeration (reducing dissolved oxygen saturation), thereby precluding access by many fish species; and damage by feral pigs, Sus scrofa (Connolly et al. 2012; Perna et al. 2012; GBRMPA 2013; Davis et al. 2014; NQ Dry Tropics 2016; Waltham et al. 2020).
In our surveys, freshwater wetland sites were located (i) upstream of the Ramsar site and within the BHWSS, including (ii) along Barratta Creek and (iii) Horseshoe Lagoon Conservation Park (0.8 km2); (iv) at Wongaloo Conservation Park (18 km2), adjacent to the BHWSS; and (v) at the Townsville Town Common conservation area (40 km2) (Tait and Veitch 2007) (Fig. 1). The Wongaloo and Townsville Town Common wetlands comprise mainly seasonal vegetated and open-water swamps but do not receive irrigation drainage and have no sugarcane growing in the catchments. Wongaloo is subject to partial cattle grazing. Horseshoe Lagoon is an open-water habitat situated within the agricultural area and artificially perennial because it receives irrigation tailwater.
Data collection
We use the terms ‘survey’ to describe an organised series of samples (or datasets) and ‘sample’ to describe a single census or count at a single site and time. Datasets from seven surveys and several univariate and multivariate analyses were used in this study to address the five hypotheses (Table S5) as described below.
We undertook two separate surveys: (1) comprising two dry-season and two wet-season sets of samples from each of 32 sites in 1991–1993; and (2) comprising three samples from each of 25 sites in June, July and August 2003. Sampling sites were selected from an initial listing of over 400 individual wetlands according to representativeness and accessibility, from upstream to downstream and east to west on the floodplain (Fig. 1; Table S1). These ranged from 0.1 to 400 ha in area and included only discrete, freshwater/slightly brackish waterbodies such as riverine lagoons and extensive swamps. Identification and counts of waterbirds were made using binoculars and telescope from vantage points around entire wetlands or around defined portions of more extensive waterbodies. Counts were expressed as absolute numbers per sample and as density (numbers of waterbirds per ha sampled). Density and presence/absence data were used for most analyses.
We also accessed other datasets from (3) the Birdata Atlas database (BirdLife Australia 2022) for the whole study area for 1972–2021, for 2012–2021 at (4) Wongaloo and (5) Horseshoe Lagoon (Fig. 1), during which multiple surveys were undertaken each year; (6) an aerial survey by the University of New South Wales (Kingsford and Porter 2009; Kingsford et al. 2020); and (7) sites ground-truthed during an aerial survey by Driscoll et al. (2012). Breeding activity (presence of accompanied juvenile birds) was noted during surveys of Wongaloo but was not otherwise consistently recorded. The abundance of cryptic species, especially crakes and rails (Gruiformes: Rallidae) was likely underestimated in all these surveys and occurrence of rarer species may have been missed because of limitations in numbers of sampling sites and times.
In Surveys 1 and 2, we recorded up to 32 environmental variables for each sample as potential influences on waterbird assemblages (Table S6). We recorded, for each sample (site and time), the area surveyed; estimated maximum water depth; riparian native tree diversity (number of species along ~50 m of representative wetland perimeter) as a measure of condition; whether the wetland dried during the study period; distance of the wetland from the coast and agricultural influences (using Google Earth for the year sampled) as possible proxies for agricultural or saline disturbance; species richness of aquatic plants at access points; antecedent annual rainfall at Ayr; and antecedent discharge for Barratta Creek. Additionally, for each sample in Survey 1, we further characterised each site by measuring a standard suite of water quality variables in situ (temperature, conductivity, pH and dissolved oxygen (using Yellow Springs Instruments meters) and collected water samples from 20 to 30 cm below the water surface directly into sample bottles and preserved these for subsequent analysis. Bottles were selected in accordance with requirements for preservation specified by Australian Standard 2301.1. Samples for nutrient analyses were filtered using Sartorius 0.45-μm cellulose acetate filters, stored in gamma-sterilised plastic tubes and snap-frozen upon arrival at the laboratory (a commercial water analysis laboratory in the Australian Centre for Tropical Freshwater Research (currently TropWater) at James Cook University). Preservation procedures and analysis followed standard methods (APHA 2017). Variables measured included concentrations of calcium, chlorophyll a, potassium, magnesium, sodium, ammonium, nitrate plus nitrite, phosphate, total nitrogen, total phosphorus and total suspended solids. Water quality may not directly affect waterbirds but has a strong bearing on habitat quality and productivity for food organisms (plants, invertebrates and fish), and may therefore be of indirect influence. We were particularly interested in any effects on productivity through nutrient input (positive) and suspended sediments (negative), and indicators of saline conditions (suggesting coastal encroachment). Low levels of dissolved oxygen could indicate limitations on bird food organisms (fish and invertebrates). Other measures formed part of a normal water quality scan that typically accompanies most studies of aquatic ecology.
Statistical analysis
Species counts and densities were log10(x + 1) transformed to normalise data for analysis, except for some graphical display of abundance. Our analyses included only samples from wetlands that had not dried unless otherwise indicated. Surveys and the analyses applied to the data are listed in Table S5.
We compared assemblage composition among the data sources using Pearson correlation analysis to check for overall agreement. Initial observations suggested that many wetland species select a broad range wetland habitats, such that we predicted that assemblages would be nested subsets of the species pool rather than substantially different – that is, assemblage composition at species-poor sites would be a subset of those at species-rich sites (Patterson and Atmar 1986), forming part of a large metacommunity along a habitat gradient (essentially, wetland size). The degree of nestedness in Surveys 1 and 2 was compared with a null model to determine whether the samples formed a nested hierarchy or were random (Joppa et al. 2010) by calculating the ‘nestedness metric based on overlap and decreasing fill’ (NODF) metric and associated statistics (Almeida-Neto and Ulrich 2011), using the online software at http://ecosoft.alwaysdata.net/.
Overall waterbird abundance, density and species richness in Surveys 1 and 2 were compared with area, depth and riparian condition using linear regression in SigmaPlot 12.5 (Systat Software, Inc., San Jose, CA, USA). We described broad trophic relationships by grouping species into functional groups determined by the predominant diet (vegetation, including seeds, tubers, etc.; invertebrates; and fish) (Barker and Vestjens 1989; Garnett et al. 2015) to provide an outline of major trophic dependencies. Biomass of individual species and functional groups was calculated as mean density × mean individual mass (from Garnett et al. 2015) for Surveys 1 and 2.
We used the Primer package (Primer ver. 7.0.23 and Permanova+ from Quest Research Limited, Massey University, Auckland, New Zealand) for multivariate analysis. We investigated the influence of normalised environmental variables on the bird assemblages in Survey 1 with distance-based linear modelling (DistLM), using the Bray–Curtis similarity measure on proportional data, given the variation in data sources. DistLM is essentially a multiple regression procedure that uses a similarity matrix of sites based on species’ densities. This seeks the most significant relationships between assemblages and environmental variables by progressively modelling the matrix against the most influential variable, taking the residuals of that relationship, modelling the next most influential variable etc. thereby taking into account correlations between variables (Anderson et al. 2008). Thirty-two environmental variables were associated with each sample (Table S6), all of which could affect bird assemblages directly or indirectly. We used multiple regression analysis in Statistix (ver. 10, Analytical Software, Tallahassee, FL, USA) to investigate the influence of the environmental variables on individual species’ densities, total density and species richness.
We included the season, month and year of the variables in the DistLM and multiple regression analyses of Survey 1 data for analysis of short- to medium-term temporal change. We used Atlas count records to identify monthly changes over a full year graphically and via repeated measures ANOVA between seasons for the aggregated data over 7 years (2015–2021) of mean total density, mean total number of species and mean abundance of more common species, using Statistix for the Wongaloo and Horseshoe Lagoon sites. We assigned three nominal seasons, based on rainfall and hydrological characteristics: Wet, Transitional and Dry, representing January–April, May–August and September–December respectively. We similarly analysed changes in the three broad functional (diet) groups. We described short-term temporal changes in abundance during wetland drying graphically for Survey 2 (transitional period only). We also examined the effects of drying on behavioural groupings (foraging methods including aerial, swimming/diving and wading) of the waterbirds that essentially relate to the depth of the water body and associated decline.
We illustrated long-term (decadal) change in assemblages using nonmetric multi-dimension scaling (NMDS) in Primer. We ordinated the annual mean proportional composition of assemblages over three decades for the five surveys that had suitable data (Table S5). We ordinated change in presence/absence data over four decades for all datasets. The effects of time, antecedent rainfall and flow on the proportional data were tested using DistLM. Antecedent rainfall and flow were represented by total annual rainfall at Ayr and discharge in Barratta Creek for the current and previous years to provide an indication of wet and dry years that may influence assemblages. Data were sourced from the Australian Bureau of Meteorology and Queensland Government Water Monitoring Portal (https://water-monitoring.information.qld.gov.au/) station 119101A (West Barratta Creek) respectively. Differences between a priori groups of samples were investigated using Analysis of Similarities (ANOSIM) in Primer.
Results
Hypothesis 1. Waterbird assemblages are generally similar among the wetlands surveyed
Across all datasets, 100 waterbird (including shorebird) species were recorded (Table S2), of which 44 species occurred in five or more of the datasets. Twenty-six of the remaining species were shorebirds that inhabited the intertidal zone in Australia that was not included in this study. Forty-seven species were recorded during Surveys 1 and 2 (Table 1). There were surprisingly strong correlations among the datasets that recorded abundance, given the different approaches used: for all comparisons, r ≥ 0.7, except Horseshoe Lagoon, for which r = 0.55; in all cases, P < 0.001. Nevertheless, individual datasets recorded differences in assemblages: for example, Horseshoe Lagoon and Wongaloo showed strong similarities in common species such as Pacific Black Duck and Black Swan but large differences between cormorants and Hardhead, probably due to the consistently deeper habitat in the former as a result of irrigation drainage.
In Surveys 1 and 2, 24 species occurred in more than 10% of samples, with 12 of these occurring in at least 25% of the samples (Table 1). The most frequently recorded species (among times and sites) were Masked Lapwing, Pacific Black Duck, Australasian Darter and Great Egret, while the most numerically abundant species were Magpie Goose, Pacific Black Duck and Plumed Whistling-Duck. Biomass of individual species followed a similar pattern to that of abundance, with the goose and two duck species predominant. Collective mean biomass of functional groups was estimated at 5992 kg.ha−1, 1249 kg.ha−1 and 370 kg.ha−1 respectively for vegetation, invertebrate and fish consumers.
Nestedness analysis showed that samples from Surveys 1 and 2 were highly nested (NODF index = 67.4, Z-score = 18.85, relative nestedness = 0.43, P <0.0001), indicating that all samples were nested within the entire metacommunity, with no separate groupings.
Hypothesis 2. Wetland bird assemblages vary spatially with wetland character
Abundance of waterbirds increased with wetland area but density decreased (Fig. 2a, b). Species richness increased with abundance and consequently with area (Fig. 2c). Richness increased with maximum depth to a peak at ~3.0 m, after which it declined (Fig. 2d). Richness declined with higher riparian diversity, although the relationship was weak (Fig. 2e). Reflecting the overall data, density of functional groups was negatively correlated with wetland size (r = −0.414, −0.420, −0.366 respectively for vegetation, invertebrate and fish consumers with P < 0.01 in each case). However, there were no clear relationships with depth, riparian condition or macrophyte diversity (r < 0.20, P > 0.05).
Results of regression analyses of data from Surveys 1 and 2 to demonstrate relatonships between: (a) waterbird abundance and wetland area; (b) waterbird density and wetland area; (c) waterbird species richness and wetland area; (d) waterbird species richness and wetland depth; and (e) waterbird species richness and wetland riparian integrity. Regression lines and associated r2 values are indicated, based on linear models, except for peak model in (d). Symbols (some overlapping) represent all samples (sites and times: black circles, Survey 1; grey triangles, Survey 2).
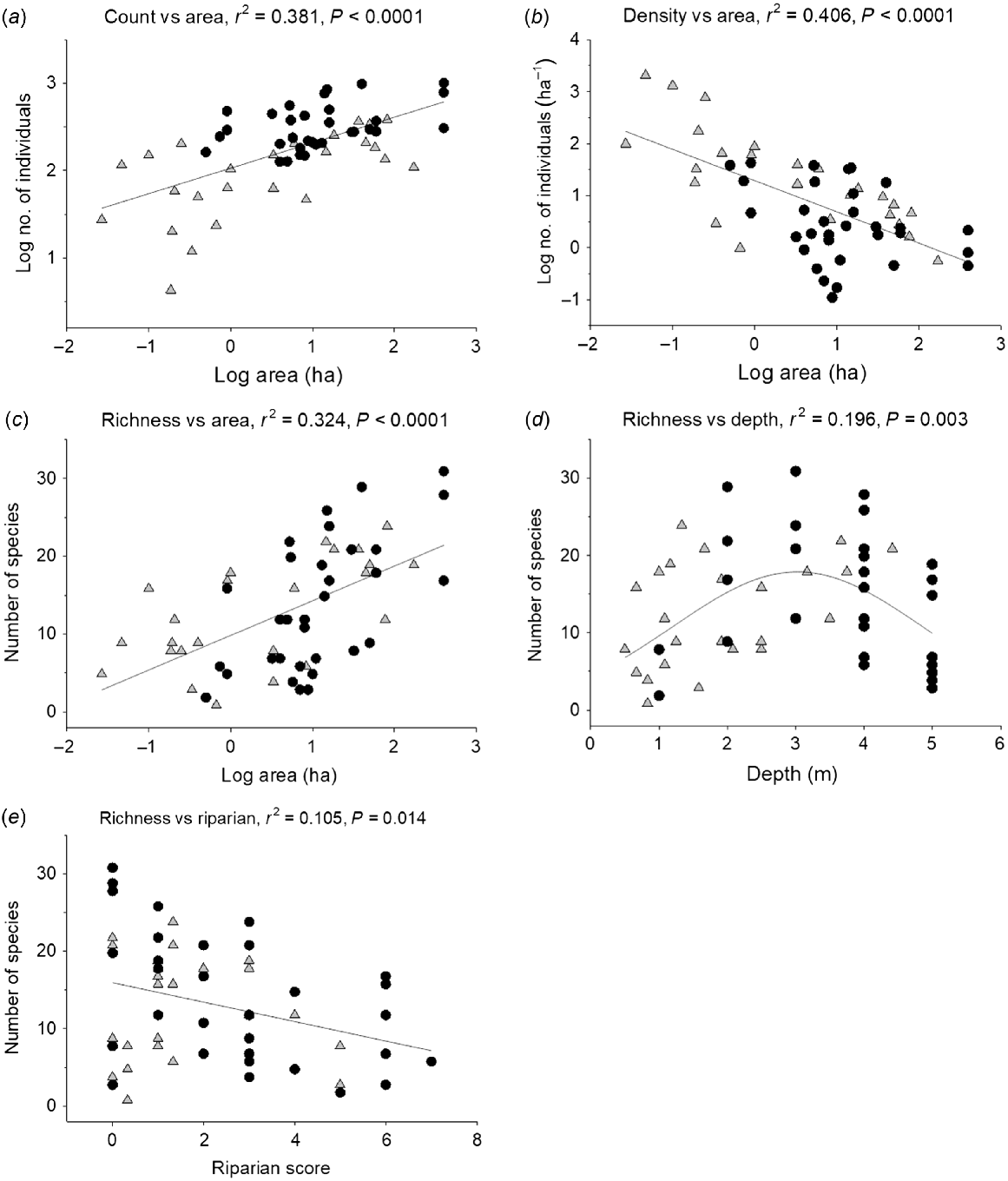
Multiple regressions of densities of common species in Survey 1 tested models with temporal, locational, site and water-quality characteristics. Models with r2 > 0.25 and P < 0.05 (Table 2) included those for total density, species richness and density of 15 individual species. Total density was negatively related to sodium (Na) concentration. Species richness showed positive relationships with wetland area and phosphate concentration. All 15 individual species had significant relationships with water-quality variables, with some indicators of productivity being particularly evident (chlorophyll a and the nutrients ammonium (NH4) and phosphate (PO4), with four, eight and four positive instances respectively) (Table S3). Na (four instances) was negative whereas distance from the coast (two instances) was positive. Other variables, with three or fewer instances, differed among species as to whether these were positive or negative influences. Several variables had no significant relationship. These included most of the direct seasonal indicators, although temperature, drying and instantaneous discharge had minor influence (together, five instances). Correlation analysis between seasonal and water-quality variables yielded none with r2 > 0.08.
Richness, total density and species identity | Predictor variables | t | P | Model F | Model P | Model r2 | |
---|---|---|---|---|---|---|---|
Richness | Wetland area | 4.04 | 0.0001 | 5.13 | <0.0001 | 0.695 | |
Phosphate (PO4) | 2.92 | 0.0047 | |||||
Total density | Sodium (Na) | −2.8 | 0.0066 | 1.96 | 0.0097 | 0.465 | |
Little Egret | Phosphate (PO4) | 6.05 | <0.0001 | 20.3 | <0.0001 | 0.900 | |
Total phosphorus (TP) | −3.02 | 0.0035 | |||||
Dissolved oxygen (DO2) | 2.46 | 0.0165 | |||||
Conductivity | 2.23 | 0.0291 | |||||
Km from coast | 2.07 | 0.0416 | |||||
Wandering Whistling-Duck | Ammonium (NH4) | 4.47 | <0.0001 | 5.5 | <0.0001 | 0.710 | |
Phaeophytin | −4.11 | 0.0001 | |||||
Total suspended solids (TSS) | 2.93 | 0.0046 | |||||
DO2 | −2.75 | 0.0076 | |||||
Nitrate + nitrite (NOX) | 2.34 | 0.0219 | |||||
Magnesium (Mg) | 2.11 | 0.0381 | |||||
Magpie Goose | Na | −3.7 | 0.0004 | 3.04 | <0.0001 | 0.575 | |
Calcium (Ca) | 2.67 | 0.0094 | |||||
Discharge | −2.35 | 0.0218 | |||||
NOX | 2.3 | 0.0246 | |||||
Wetland area | 2.14 | 0.0359 | |||||
Black-winged Stilt | PO4 | 4.69 | <0.0001 | 2.99 | 0.0001 | 0.571 | |
TP | −4.71 | <0.0001 | |||||
Potassium (K) | 2.6 | 0.0113 | |||||
NH4 | 2.05 | 0.044 | |||||
Km from coast | 2.02 | 0.0471 | |||||
NOX | −2.01 | 0.0481 | |||||
Plumed Whistling-Duck | Chlorophyll a | 3.21 | 0.002 | 2.74 | 0.0002 | 0.549 | |
K | −2.54 | 0.0132 | |||||
Macrophytes | −2.23 | 0.0291 | |||||
NH4 | 1.99 | 0.0502 | |||||
Masked Lapwing | Depth | −2.64 | 0.01 | 2.34 | 0.0015 | 0.510 | |
NH4 | 2.41 | 0.0186 | |||||
Conductivity | −2.39 | 0.0195 | |||||
K | 2.3 | 0.0244 | |||||
TP | −2.18 | 0.0328 | |||||
Yellow-billed Spoonbill | PO4 | 3.83 | 0.0003 | 2.24 | 0.0024 | 0.499 | |
Phaeophytin | −3.41 | 0.0011 | |||||
NH4 | 3.23 | 0.0019 | |||||
Depth | −2.58 | 0.0118 | |||||
TSS | −2.1 | 0.0394 | |||||
Comb-crested Jacana | Na | −3.03 | 0.0034 | 2 | 0.008 | 0.470 | |
TSS | 2.9 | 0.005 | |||||
NH4 | 2.87 | 0.0053 | |||||
PO4 | 2.34 | 0.0222 | |||||
TN | −2.1 | 0.0395 | |||||
DO2 | −1.99 | 0.0504 | |||||
Australasian Darter | Temperature | −2.93 | 0.0045 | 1.91 | 0.0119 | 0.460 | |
Wetland area | −2.22 | 0.0292 | |||||
Drying | −2.17 | 0.0336 | |||||
Cattle Egret | TP | 3.98 | 0.0002 | 1.83 | 0.0174 | 0.449 | |
Chlorophyll a | 1.97 | 0.0521 | |||||
Australasian Grebe | Riparian | −2.82 | 0.0063 | 1.75 | 0.0256 | 0.438 | |
Wetland area | −2.64 | 0.0101 | |||||
Na | −2.15 | 0.0352 | |||||
Drying | −2.08 | 0.0409 | |||||
Australian Pelican | Conductivity | 2.68 | 0.0091 | 1.71 | 0.0307 | 0.432 | |
Chlorophyll a | 2.13 | 0.037 | |||||
Great Egret | Chlorophyll a | 3.94 | 0.0002 | 1.68 | 0.0354 | 0.428 | |
NH4 | 3.43 | 0.001 | |||||
K | −3.1 | 0.0028 | |||||
Phaeophytin | −2.72 | 0.0082 | |||||
Temperature | 2.52 | 0.0138 | |||||
TSS | −2.28 | 0.0256 | |||||
Wetland area | −2.02 | 0.0472 | |||||
Intermediate Egret | Ca | 3.28 | 0.0016 | 1.64 | 0.0417 | 0.422 | |
Mg | −2.19 | 0.0314 | |||||
Pacific Black Duck | Na | −2.73 | 0.0079 | 1.61 | 0.0476 | 0.418 | |
NH4 | 2.61 | 0.011 | |||||
Mg | 2.38 | 0.0201 |
d.f. = 32, 72. Species ordered by r2. Explanation of the variables is provided in Table S6.
DistLM was likewise applied to Survey 1 bird assemblage data and environmental variables, producing a model that included all 32 variables that together explained 46.8% of the variation (r2 = 0.468), although for no individual variable was r2 greater than 0.060 (Table 3).
Variable | d.f. | Pseudo-F | P | r2 | Cumulative r2 × 100% | |
---|---|---|---|---|---|---|
Wetland area | 103 | 6.596 | 0.001 | 6.02 | 6.02 | |
PO4 | 102 | 4.583 | 0.001 | 4.04 | 10.06 | |
Depth | 101 | 3.801 | 0.002 | 3.26 | 13.32 | |
Distance from sugarcane fields | 100 | 3.804 | 0.002 | 3.18 | 16.5 | |
Drying | 99 | 3.887 | 0.001 | 3.15 | 19.65 | |
Riparian species richness | 98 | 2.600 | 0.007 | 2.08 | 21.73 | |
Total (all 32 variables in model) | 72 | 46.83 |
Only variables with P < 0.05 are shown.
Hypothesis 3. Abundance of waterbird populations and assemblage composition vary seasonally, with maxima in the wet season
At Wongaloo, overall abundance, abundance of functional groups, and abundance and percent frequency of occurrence of many common species varied between seasons, particularly with increases in the dry season (Figs 3, S3 and S4; Table S7). The seasonal pattern was not clear at Horseshoe Lagoon, except that the Black Swan appeared to be more abundant in the drier part of the year. At Wongaloo, individual species showed varied patterns of occurrence frequency, with many species present in all months, some more prevalent in the dry season and a few migratory waders such as the Marsh Sandpiper being recorded more frequently in the summer wet season (Fig. S3). In Survey 2, patterns of occurrence frequency across three sites that dried over the 3-month period showed the expected gradual loss of species, with deepwater species leaving first, followed by waders (Table S8). Notably, Brolga remained during drying, feeding on sedge tubers. Evidence of breeding of different species at Wongaloo suggested that breeding occurred mainly during the transitional period and into the dry season (Table S9).
Monthly relative abundance of common species (% of aggregated totals over 2015–2021), total abundance and total number of species over the same period at (a) Horseshoe Lagoon and (b) Wongaloo. Differences with P < 0.05 (Tukey test following repeated measures ANOVA) between Wet (January–April), Transition (May–August) and Dry (September–December) seasons (defined by vertical dashed lines) are indicated by letters a and b. Seasons were offset one month to the right for Hardhead analysis. ANOVA results are shown in Table S4. ‘ns’, no sample.
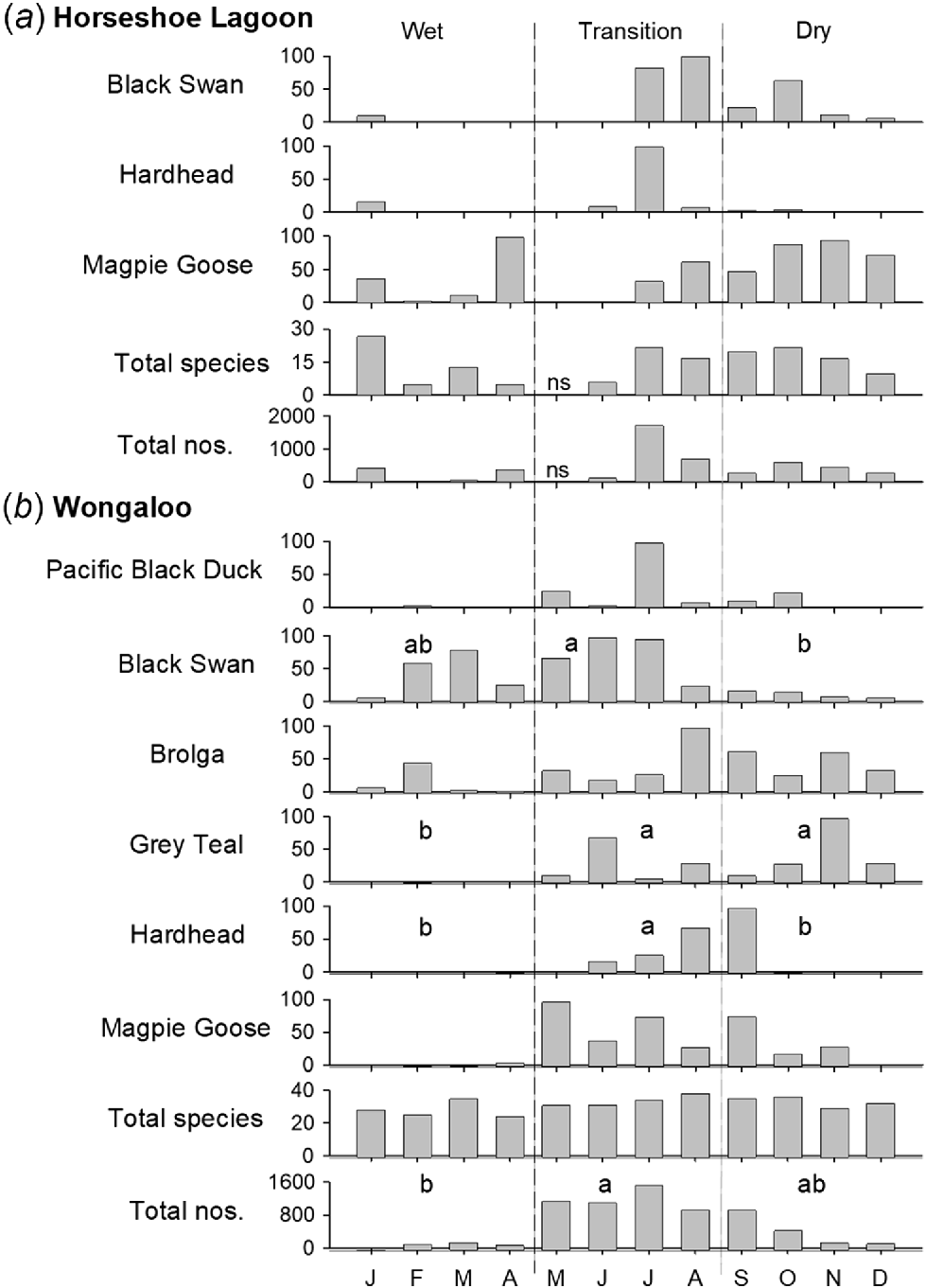
Hypotheses 4 and 5. Assemblages vary annually according to antecedent rainfall and assemblages have changed over several decades
In DistLM, for proportional (density) data, no relationship was evident for assemblages across years (Pseudo-F = 1.975, P = 0.098), with antecedent annual rainfall (Pseudo-F = 0.240, P = 0.875) or flow (Pseudo-F = 0.383, P = 0.840); r2 for the model = 0.052. Similarly, for presence/absence data, no relationship was evident for assemblages across years (Pseudo-F = 1.687, P = 0.165), with antecedent annual rainfall (Pseudo-F = 0.137, P = 0.933) or flow (Pseudo-F = 0. 401, P = 0.788); r2 for the model = 0.059.
The NMDS of proportional abundance of species in assemblages over three decades also showed little evidence of temporal change but indicated, three distinct groupings of surveys: at Wongaloo, Horseshoe Lagoon and the Burdekin-Townsville floodplain (Fig. 4a). ANOSIM demonstrated significance of the overall model (global R = 0.772, P = 0.001), and pairwise differences between all group pairs (Burdekin–Townsville floodplain (BT) and Horseshoe Lagoon (HL), R = 0. 940, P = 0.005; BT and Wongaloo (W), R = 0.68, P = 0.006; HL and W, R = 0. 884, P = 0.001).
Temporal distribution of annual data aggregated for identified samples, ordinated using multidimensional scaling plot of datasets: (a) over three decades (1991–2021) – proportional mean abundance (i.e. % composition) within a survey; and (b) over four decades (1979–2021) – presence/absence data. Samples are labelled by survey or site: A – BirdLife Atlas; HL – Horseshoe Lagoon (Atlas data); BT – Burdekin–Townsville floodplain (this study); W – Wongaloo (Atlas data); DR – Driscoll et al. (2012) ground surveys; UN – University of New South Wales aerial surveys); and by year (1979–2021).
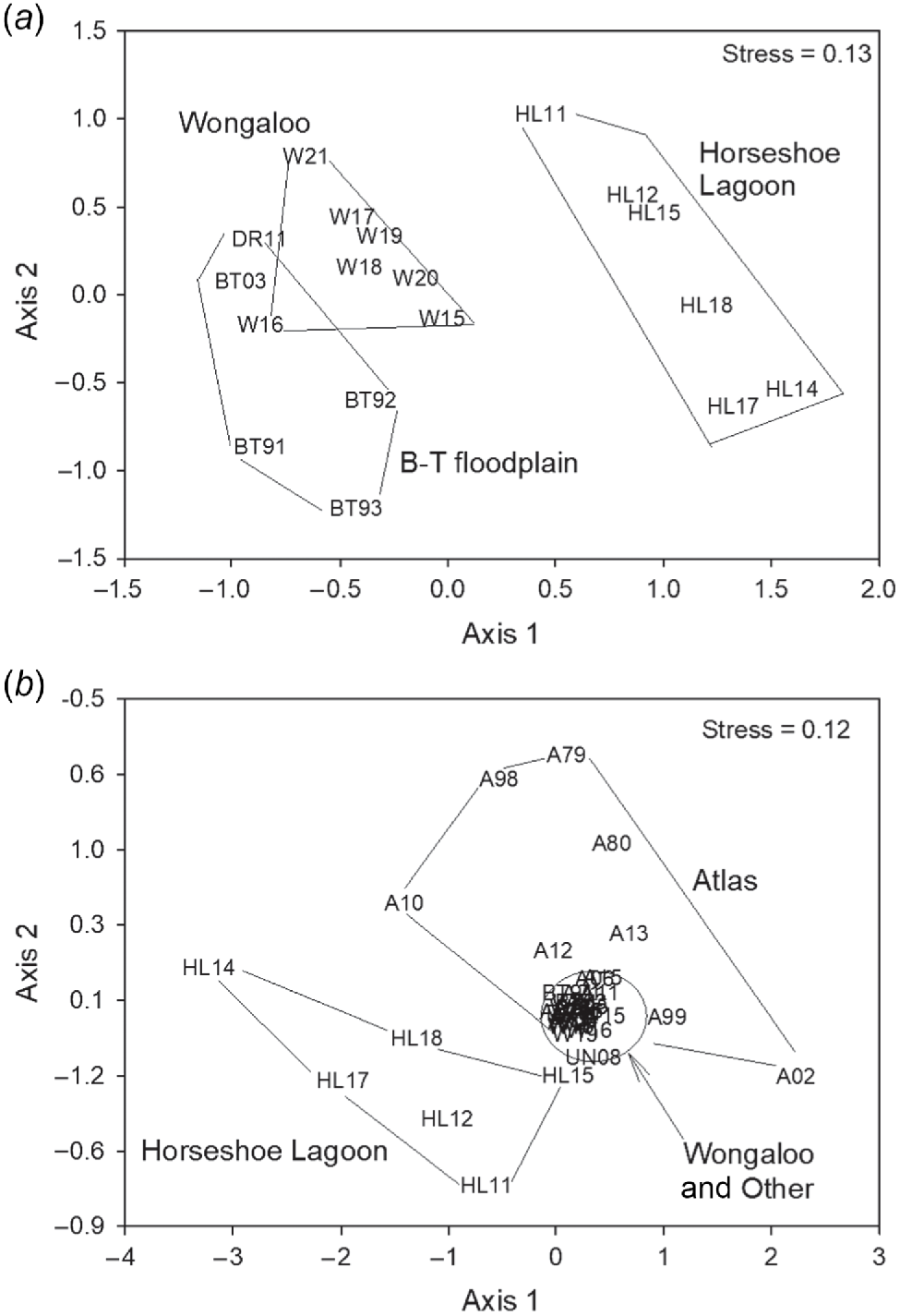
In the NMDS of presence/absence data over four decades, again there was little evidence of temporal change, but two distinct groupings emerged: Horseshoe Lagoon and a combination of the three other groups (Atlas, Wongaloo and Other) (Fig. 4b). ANOSIM demonstrated non-significance of the overall model (global R = 0. 124, P = 0. 143), but pairwise comparisons found the Horseshoe Lagoon samples to be separate from those in Atlas and Wongaloo (R = 0.530, P = 0.001), and the ‘Other’ grouping, which included samples from the remaining surveys (global R = 0.569, P = 0.002). In these analyses, the Wongaloo site, which was not affected by intensive agriculture, notably grouped with Atlas and Other sites, some of which were subject to agricultural impacts.
Discussion
Hypothesis 1. Waterbird assemblages are generally similar among the wetlands surveyed
The Burdekin–Townsville freshwater wetlands provide extensive diverse habitats for a rich, and abundant waterbird fauna, estimated at 10 times the total waterbird abundance (excluding shorebirds) from the contiguous Bowling Green Bay Ramsar wetlands (Driscoll et al. 2012). In that study, four species occurred in internationally significant numbers (Cotton Pygmy Goose, Eastern Great Egret, Pacific Black Duck and Royal Spoonbill) and six further species in nationally significant numbers (Black Swan, Hardhead, Plumed Whistling Duck, Wandering Whistling-Duck, Intermediate Egret and Little Black Cormorant). The Ramsar wetlands support globally significant populations of shorebirds (Driscoll et al. 2012), some of which occurred in lower numbers in our study area. Our data correlate strongly with these and other records, despite differences in sampling design and extent, and the three- or four-decade span of the data. The most abundant species by numbers and biomass were vegetation feeders (Magpie Goose and Plumed Whistling-Duck) or generalist vegetation/invertebrate feeders (Pacific Black Duck) (Barker and Vestjens 1989; Garnett et al. 2015), which greatly exceed the biomass of specialist invertebrate feeders and piscivores, reflecting the expected trophic pattern of productivity. The populations of the grazers, Magpie Goose and Plumed Whistling-Duck, were likely boosted by the terrestrial food supply in well-watered grassland, including sugarcane headlands. Estimates of population size (Driscoll et al. 2012) produced one of the largest waterbird counts of eastern Australian wetlands when compared with 20 other important sites (Kingsford and Porter 2009).
Nestedness analysis found that individual assemblages were subsets of the entire set of samples, with no major habitat-based divisions. Nearly all species were represented in the larger wetlands, explaining the high level of nestedness. However, the inclusion of the Ramsar site would probably have shown a separation between the waterbird- and shorebird-dominated assemblages (Driscoll et al. 2012). Therefore, the broad requirements of many waterbird species are likely accommodated by the different wetland types, especially the larger ones, although some sites show distinct signatures in the ordinations. For example, samples from Horseshoe Lagoon were separated from the remaining samples, likely due to its perennial nature resulting from irrigation drainage.
Hypothesis 2. Wetland bird assemblages vary with wetland character
Abundance and species richness of waterbirds and functional groups increased with wetland area, as expected from the species richness/area relationship generally, and reflected by observations globally, such as in Tunisia (Hamza and Selmi 2018), Amazonia (Cintra 2019) and China (Li et al. 2019). Sites with high species richness were large, with open water. Habitats included large, small, shallow, deep, permanent and temporary types, together providing most of the habitats required by the waterbirds. However, overall densities decreased with increasing area, as expected if the number of waterbirds was fixed. Additionally, the area of a particular habitat does not necessarily increase linearly with wetland size. Population size may also be limited by breeding success locally or further afield but this seems unlikely given the extent of the wetland/grassland complex for ground-nesting waterbirds and patchy woodland, gallery forest and extensive mangroves for those nesting in trees. Apart from the shorebird migrants, many of the common wetland species recorded are known or expected to breed within the wetland complex. The wetlands are also likely to provide a dry-season refuge for waterbirds whose breeding sites are elsewhere, as some of the seasonal data suggest. Waterbirds are likely to be subject to predation by native fauna and feral pigs but the impact is unknown.
The hump-shaped relationship of species richness with depth probably occurred because suitable habitat for many species was proportionately diminished in deeper waters. This was very clear for riverine lagoons that only had small areas of shallow water. Depth is a critical issue: for example, a global review indicates that a wide diversity of depths boosted diversity, with shorebirds restricted to less than 15 cm depth, dabbling ducks and large waders to about 25 cm and diving birds using much deeper waters (Ma et al. 2010). In the study area, dabbling ducks also occurred in deeper water in which submerged plants reached the surface. The Burdekin–Townsville complex provides large areas of shallow and deeper waters, thereby providing suitable feeding habitat for waders, dabblers and diving birds.
A negative relationship between bird species richness and riparian integrity was weak, possibly because (i) riparian condition declined with increasing area, as riparian vegetation was less well defined in the larger wetlands or (ii) waterbirds essentially prefer more open areas. Nevertheless, the local riparian vegetation and discrete woodland patches are important as breeding and roosting sites for several species. Total density, species richness and density of common species correlated with environmental variables (model r2 > 0.250), including those relating to water quality, and especially indicators of productivity. There were no negative relationships with water quality, possibly because deep riverine lagoons, where weed infestation can cause poor water quality (Waltham et al. 2020), do not normally support many waterbirds. However, even deep lagoons can be attractive to Magpie Geese that feed on the invasive water hyacinth, and to predators such as Comb-crested Jacana and egrets that feed on animals found among hyacinth plants.
DistLM analysis of the assemblages explained ~47% of the variation in the data in a model that included all the environmental variables, each of which was very weakly associated with the bird resemblance matrix, again indicating little evidence of strong reactions to human disturbance. Therefore, although agriculture, pollution and invasive species are implicated in the degradation of wetlands in southern Queensland (Bino et al. 2016), and in our study area the same is true for aquatic invertebrates and fish (Connolly et al. 2012; Pearson et al. 2019; Tibby et al. 2019; Waltham et al. 2020), the picture was less clear for the bird assemblages. Whether or not waterbirds are indirectly affected by such impacts is unknown. Water resource development has had a significant physical impact on the Burdekin–Townsville wetlands, as elsewhere (Kingsford and Porter 2009), yet substantial assemblages continued to use the wetlands.
The weak influence of anthropogenic factors on waterbird assemblages has been reported elsewhere (Daniel et al. 2021; Khaliq et al. 2019). In the Burdekin–Townsville area, the sheer extent and diversity of the mosaic of wetlands may absorb or buffer impacts and while this may not be true of highly modified wetlands such as Horseshoe Lagoon, even this wetland provided suitable habitat for many species. Additionally, waterbird assemblages may benefit from increased wetland habitat introduced by irrigation delivery and drainage channels, ponded pastures and artificial storages, possibly offsetting wetland loss due to land clearing and levelling, as may be the case for farm dams and rice fields (Hamilton et al. 2017; Herring et al. 2019). Nevertheless, active management is required for the whole ecosystem, including control of groundwater levels and salinity, with sea-level rise a developing threat in the region (GBRMPA 2019).
Hypothesis 2 was therefore largely supported with regard to morphology but, despite signals of the impacts of agriculture, the impacts were less substantial than expected for the bird assemblages. Cumulative impacts on waterbird assemblages may not yet be evident, although this is unlikely, given that three decades have passed since the major expansion of sugarcane cultivation (see below).
Hypothesis 3. Abundance and assemblage composition vary seasonally
A seasonal pattern was not clear at Horseshoe Lagoon, in which water levels were maintained by irrigation runoff. At Wongaloo, many common species varied seasonally in abundance and occurrence frequency, typically with a dry-season peak or no peak, except that migratory shorebirds mostly occurred during the wet season. Differences between years were inconsistent, indicating that no major trend or driver of change was operating. Our finding of reduction in abundance of some species during the wet season is likely due to local dispersal as habitats expanded or movement to different areas for breeding, as occurs in the Australian Northern Territory (Morton et al. 1990a, 1990b, 1993a, 1993b). We found that waterbirds subsequently returned to the wetlands for a great deal of the dry season, trailing off as wetlands dried and diminished in extent, in which case there was a succession of waterbirds moving away, as expected. Seasonal movements were not as clear as in drier country (Halse et al. 2005; Kingsford et al. 2010), contrasting with the greater seasonality that is typical of inland Australia, where replenished habitat is exploited during and following the wet season (Kingsford et al. 2017; Caley et al. 2022) or following infrequent wet years (Halse et al. 2005).
Therefore our second hypothesis was supported, except that maximum abundances occurred after and not during the wet season.
Hypothesis 4. Assemblages vary among years according to antecedent rainfall
This hypothesis was not supported because there was little evidence that antecedent rainfall or flow were implicated in interannual variation among assemblages or individual species. As noted above, the Burdekin–Townsville wetlands are generally seasonal, although many are sustained through the dry season by normal or rising groundwater levels and irrigation tailwater that reduce interannual variability, in contrast with a large proportion of the continent.
Hypothesis 5. Assemblages have changed over several decades as a result of floodplain development
We summarised a substantial quantity of data but acknowledge that the different sources from different times may preclude substantiation of any conclusions. For this reason we were conservative in our approach and cautious in our interpretation. We found no evidence for bird assemblage change over the entire study period (three decades of proportional data, four decades of presence/absence data), despite the extensive agricultural development of the study area. Cumulative impacts were possibly not yet evident, except that it was approximately three decades since the major expansion of sugarcane growing. Undoubtedly, many wetlands were lost to or degraded by agricultural development (GBRMPA 2013; Davis et al. 2014; Waltham et al. 2020) but overall this loss appeared to be mitigated by the extent and diversity of remaining or novel wetlands, including modified and artificial wetlands as noted above. Using a rich dataset, Colloff et al. (2015) likewise demonstrated mixed trajectories or only minor decline in integrity of wetlands in the Murray–Darling area. We had limited data from before the expansion of intensive agriculture involving land clearing, levelling and irrigation development. However, the inter-decadal samples were collected in a similar timeframe to the agricultural development but no association was found. Additionally, some sites (e.g. Wongaloo and adjacent sites) were unaffected by agricultural development, providing control sites that corroborate our conclusion by grouping with our survey sites in multivariate analyses.
We should note that the bird assemblage data that we record are insufficient to track gradual trajectories in the occurrence of rarer species, for which targeted surveys would be required. For example, the Australian Painted Snipe declined nationally during the study period with a recent Australian population estimate of only 340 (Rogers et al. 2021). Lavery and Seton (1974) recorded this species as ‘uncommon’ for our study area, while the Birdata database has only two records. Our broad surveys were inadequate to assess population size or change for this or other rare species.
Conclusion and conservation implications
The Burdekin–Townsville wetlands provide extensive, diverse habitats for a rich waterbird assemblage. Unlike many wetlands globally, the Burdekin–Townsville wetlands do not suffer a loss of water caused by irrigation agriculture (Lemly et al. 2000) as water is sourced from outside the system (the Burdekin River and Dam), and irrigation infrastructure (channels and storages) and tailwater supplement many wetlands (e.g. Horseshoe Lagoon and natural channels) either directly or through groundwater. Counts of six waterbird species (Black Swan, Hardhead, Plumed and Wandering Whistling-Ducks, Intermediate Egret and Little Black Cormorant) are nationally significant and total waterbird counts rank highly for eastern Australia, affirming the critical conservation importance of these wetlands (Kingsford and Porter 2009; Driscoll et al. 2012).
Despite data restrictions, the apparent absence of change in waterbird assemblages over three or four decades is noteworthy and indicates that the current extent and diversity of natural and artificial wetlands may provide a buffer against ecosystem impacts. If this is the case, they may similarly provide some protection against future changes such as those due to climate warming. Nevertheless, increasing salinity due to rising sea level in the Burdekin–Townsville coastal zone is likely to change wetland assemblages substantially with no upstream refuge available (Bernhardt 2022; Osland et al. 2022). Regular monitoring at a variety of sites, comparable to that undertaken at Wongaloo, is advisable to underpin appropriate water management, particularly for conservation goals. Additionally, special attention is required to identify and assess important breeding sites and mitigate threats to them.
Sica et al. (2020) suggested that conservation of many small rather than few large wetlands is preferable. Maintaining the full diversity of wetlands, from the smaller deep riverine lagoons to the expansive palustrine/lacustrine systems, would appear to be important for sustaining the full diversity of habitats through the seasons (Būhning-Gaese 1997) for the Burdekin–Townsville waterbirds. Ongoing management of the important ecosystem services and conservation values of the Burdekin–Townsville wetlands, the largest wetland complex on the Australian east coast, requires explicit acknowledgement of the importance and diversity of the wetlands (Adame et al. 2019). Although the nestedness analysis might suggest that management objectives could address environmental drivers and issues at a broad scale rather than piecemeal, the unique characteristics of each type of wetland and associated impact may warrant explicit individual conservation, as elsewhere (e.g. Murray et al. 2012). For example, deepwater confined lagoons, extensive multi-depth wetlands and small intermittent wetlands differ substantially with regard to weed and water-quality management (Waltham et al. 2020). Also, the nature of any potential special requirements of rare species is unclear.
Driscoll et al. (2012) found that ~90% of their waterbird data were recorded from outside the Ramsar site and therefore inadequate for protecting the palustrine wetlands, as elsewhere in Australia (Bino et al. 2016). Our results support this conclusion. Wetlands are treated as a continuum by waterbirds and other biota (Connolly et al. 2012; Tibby et al. 2019; Waltham et al. 2020; Pearson et al. 2021) and need to be recognised and managed accordingly. Although a great deal of focus has previously been placed on water quality (NQ Dry Tropics 2016) and the Ramsar site, it is gratifying to note that NQ Dry Tropics, the regional natural resources management body, is undertaking management activities across the wetland complex and catchment in keeping with management goals (NQ Dry Tropics 2016, 2024; Abbott et al. 2020). Likewise, the Great Barrier Reef Marine Park Authority supports coastal ecosystem protection, including areas of remnant floodplain set aside during the development of the BHWSS (GBRMPA 2013). In summary, the following are important conservation goals that will enhance not only bird assemblages but also aquatic biota:
protecting remnant natural wetlands against future development;
managing levels of groundwater and salinity of groundwater-dependent wetlands;
water management to allow for natural seasonal flushing and drying;
rehabilitation of watercourses and removal of barriers to allow natural connectivity between wetlands;
identifying priority wetlands and undertaking rehabilitation as required;
maintenance and restoration of riparian and contiguous terrestrial vegetation, especially to enhance waterbird breeding and roosting opportunities (Brandis et al. 2024);
controlling pest species, especially invasive plants; and
development of a floodplain management plan and monitoring program to integrate the needs of water use and ecosystem function.
Conservation management of the wetlands could benefit significantly from an overarching framework for regulation, on-ground management and dissemination of information to secure long-term health, given the national importance of these wetlands (Connolly et al. 2012; Driscoll et al. 2012), as has been advocated for the whole Burdekin system (Pearson et al. 2022). Inclusion of a large proportion of the freshwater wetland complex in an expansion of the Ramsar site to the south would be a welcome development (Kingsford et al. 2021).
Data availability
The data that support this study will be shared upon reasonable request to the corresponding author.
Declaration of funding
Funding for Survey 1 was provided by the Australian Land and Water Resources Research and Development Council and the Queensland Water Resources Commission.
Acknowledgements
We thank Birdlife Australia, especially A. Silcocks, for making data available and all contributors to the database, especially N. Rains. G. Lukacs assisted with data collection in Survey 1. We acknowledge the extensive constructive comments on the manuscript by the associate editor and three reviewers.
References
Abbott BN, Wallace J, Nicholas DM, Karim F, Waltham NJ (2020) Bund removal to re-establish tidal flow, remove aquatic weeds and restore coastal wetland services—North Queensland, Australia. PLoS ONE 15(1), e0217531.
| Crossref | Google Scholar |
Adame MF, Arthington AH, Waltham N, Hasan S, Selles A, Ronan M (2019) Managing threats and restoring wetlands within catchments of the Great Barrier Reef, Australia. Aquatic Conservation: Marine and Freshwater Ecosystems 29(5), 829-839.
| Crossref | Google Scholar |
Alexander J, Fielding CR, Pocock GD (1999) Flood behaviour of the Burdekin River, tropical north Queensland, Australia. Geological Society, London, Special Publications 163(1), 27-40.
| Crossref | Google Scholar |
Almeida-Neto M, Ulrich W (2011) A straightforward computational approach for measuring nestedness using quantitative matrices. Environmental Modelling & Software 26(2), 173-178.
| Crossref | Google Scholar |
Australian Government (2024) Directory of important Wetlands in Australia – Burdekin-Townsville coastal aggregation – QLD005 information sheet. Available at www.dcceew.gov.au/water/wetlands/australian-wetlands-database/directory-important-wetlands [accessed 5 March 2024]
Bernhardt E (2022) Coastal freshwater wetlands squeezed between migrating salt marshes and working lands. Science Advances 8(26), eadd1628.
| Crossref | Google Scholar |
Bino G, Kingsford RT, Brandis K (2016) Australia’s Wetlands – learning from the past to manage for the future. Pacific Conservation Biology 22(2), 116-129.
| Crossref | Google Scholar |
BirdLife Australia (2022) Welcome to BIRDATA: Collecting data to protect Australia’s birds. Available at birdata.birdlife.org.au/ [accessed 15 April 2022]
Brandis KJ, Francis RJ, Bino G (2024) Vegetation and inundation characteristics of waterbird breeding sites in the Murray–Darling Basin, Australia. Marine and Freshwater Research 75, MF23221.
| Crossref | Google Scholar |
Būhning-Gaese K (1997) Determinants of avian species richness at different spatial scales. Journal of Biogeography 24(1), 49-60.
| Crossref | Google Scholar |
Caley P, Reid JRW, Colloff MJ, Barry SC (2022) On inferring population trends of mobile waterbirds from aerial transect surveys in variable environments. Environmental and Ecological Statistics 29(1), 3-31.
| Crossref | Google Scholar |
Cintra R (2019) Waterbird community composition in relation to lake physical traits and wetland limnological conditions in the Amazon basin. Hydrobiologia 826, 43-65.
| Crossref | Google Scholar |
Colloff MJ, Caley P, Saintilan N, Pollino CA, Crossman ND (2015) Long-term ecological trends of flow-dependent ecosystems in a major regulated river basin. Marine and Freshwater Research 66(11), 957-969.
| Crossref | Google Scholar |
Connolly N, Kahler C, Mackay S, Fry S, Cameron R (2012) Variations in wetland condition across Land Zones in the lower Burdekin. Aquatic weed distributions determined by underlying differences in water and salinity regimes. Department of Environment and Heritage Protection, Queensland Government. Available at www.nqdrytropics.com.au/publications/ [accessed 20 May 2022]
Cooper M, Lewis SE, Smithers SG (2016) Spatial and temporal dynamics of suspended sediment causing persistent turbidity in a large reservoir: Lake Dalrymple, Queensland, Australia. Marine and Freshwater Research 68(7), 1377-1390.
| Crossref | Google Scholar |
Daniel J, Polan H, Rooney RC (2021) Determinants of wetland- bird community composition in agricultural marshes of the Northern Prairie and Parkland Region. Wetlands 41(1), 14.
| Crossref | Google Scholar |
Davidson NC (2014) How much wetland has the world lost? Long-term and recent trends in global wetland area. Marine and Freshwater Research 65(10), 934-941.
| Crossref | Google Scholar |
Davidson NC, Van Dam AA, Finlayson CM, McInnes RJ (2019) Worth of wetlands: revised global monetary values of coastal and inland wetland ecosystem services. Marine and Freshwater Research 70(8), 1189-1194.
| Crossref | Google Scholar |
Davis AM, Lewis SE, O’Brien DS, Bainbridge ZT, Bentley C, Mueller JF, Brodie JE (2014) Water resource development and high value coastal Wetlands on the lower burdekin floodplain, Australia. In ‘Estuaries of Australia in 2050 and beyond’. (Ed. E Wolanski) pp. 223–246. (Springer: Dordrecht) 10.1007/978-94-007-7019-5_13
de Groot D, Brander L, Finlayson CM (2018) Wetland ecosystem services. In ‘The Wetland book: I: structure and function, management, and methods’. (Eds CM Finlayson, M Everard, K Irvine, RJ McInnes, BA Middleton, AA van Dam, NC Davidson) pp. 323–333. (Springer: Netherlands) 10.1007/978-90-481-9659-3_66
Driscoll P, Milton S, Harding S (2012) Waterbird and shorebird surveys of the Bowling Green Bay Ramsar Site. Australian Government Department of Sustainability, Environment, Water, Populations and Communities, Australia. Available at www.environment.gov.au/water/publications/environmental/wetlands/index.html [accessed 20 May 2022]
Fluet-Chouinard E, Stocker BD, Zhang Z, Malhotra A, Melton JR, Poulter B, Kaplan JO, Goldewijk KK, Siebert S, Minayeva T, Hugelius G, Joosten H, Barthelmes A, Prigent C, Aires F, Hoyt AM, Davidson N, Finlayson CM, Lehner B, Jackson RB, McIntyre PB (2023) Extensive global wetland loss over the past three centuries. Nature 614, 281-286.
| Crossref | Google Scholar | PubMed |
Garnett ST, Duursma DE, Ehmke G, Guay P-J, Stewart A, Szabo JK, Weston MA, Bennett S, Crowley GM, Drynan D, Dutson G, Fitzherbert K, Franklin DC (2015) Biological, ecological, conservation and legal information for all species and subspecies of Australian bird. Scientific Data 2, 150061.
| Crossref | Google Scholar |
GBRMPA (2019) Great barrier reef outlook report 2019. Great Barrier Reef Marine Park Authority, Townsville. Available at elibrary.gbrmpa.gov.au/jspui/handle/11017/3474 [accessed 4 March 2024]
Grieger R, Capon SJ, Hadwen WL, Mackey B (2021) Spatial variation and drivers of vegetation structure and composition in coastal freshwater wetlands of subtropical Australia. Marine and Freshwater Research 72(12), 1746-1759.
| Crossref | Google Scholar |
Halse SA, Pearson GB, Hassell C, Collins P, Scanlon MD, Minton CDT (2005) Mandora Marsh, north-western Australia, an arid-zone wetland maintaining continental populations of waterbirds. Emu - Austral Ornithology 105(2), 115-125.
| Crossref | Google Scholar |
Hamilton AJ, Conort C, Bueno A, Murray CG, Grove JR (2017) Waterbird use of farm dams in south-eastern Australia: abundance and influence of biophysical and landscape characteristics. Avian Research 8(1), 2.
| Crossref | Google Scholar |
Hamza F, Selmi S (2018) Diversity of waterbirds wintering in Douz wetlands (south Tunisia): factors affecting wetland occupancy and species richness. Ecological Research 33(5), 917-925.
| Crossref | Google Scholar |
Herring MW, Robinson W, Zander KK, Garnett ST (2019) Rice fields support the global stronghold for an endangered waterbird. Agriculture, Ecosystems & Environment 284, 106599.
| Crossref | Google Scholar |
Joppa LN, Montoya JM, Solé R, Sanderson J, Pimm SL (2010) On nestedness in ecological networks. Evolutionary Ecology Research 12, 35-46.
| Google Scholar |
Khaliq I, Arshad MI, Gill AH, Chaudhry AA, Maan MA, Iqbal MA, Akbar M, Bowler DE (2019) Long-term changes of a waterbird community over 26 years at a Pakistani Ramsar Site. Wetlands Ecology and Management 27, 363-376.
| Crossref | Google Scholar |
Kingsford RT (1999) Aerial survey of waterbirds on wetlands as a measure of river and floodplain health. Freshwater Biology 41(2), 425-438.
| Crossref | Google Scholar |
Kingsford RT (2013) Conservation of waterbirds in Australia. Pacific Conservation Biology 19(4), 366-378.
| Crossref | Google Scholar |
Kingsford RT, Porter JL (2009) Monitoring waterbird populations with aerial surveys – What have we learnt? Wildlife Research 36(1), 29-40.
| Crossref | Google Scholar |
Kingsford RT, Roshier DA, Porter JL (2010) Australian waterbirds – time and space travellers in dynamic desert landscapes. Marine and Freshwater Research 61(8), 875-884.
| Crossref | Google Scholar |
Kingsford RT, Bino G, Porter JL (2017) Continental impacts of water development on waterbirds, contrasting two Australian river basins: global implications for sustainable water use. Global Change Biology 23(11), 4958-4969.
| Crossref | Google Scholar | PubMed |
Kingsford RT, Porter JL, Brandis KJ, Ryall S (2020) Aerial surveys of waterbirds in Australia. Scientific Data 7, 172.
| Crossref | Google Scholar | PubMed |
Kingsford RT, Bino G, Finlayson CM, Falster D, Fitzsimons JA, Gawlik DE, Murray NJ, Grillas P, Gardner RC, Regan TJ, Roux DJ, Thomas RF (2021) Ramsar Wetlands of international importance–improving conservation outcomes. Frontiers in Environmental Science 9, 643367.
| Crossref | Google Scholar |
Laurance WF, Dell B, Turton SM, Lawes MJ, Hutley LB, McCallum H, Dale P, Bird M, Hardy G, Prideaux G, Gawne B, McMahon CR, Yu R, Hero J-M, Schwarzkopf L, Krockenberger A, Douglas M, Silvester E, Mahony M, Vella K, Saikia U, Wahren C-H, Xu Z, Smith B, Cocklin C (2011) The 10 Australian ecosystems most vulnerable to tipping points. Biological Conservation 144(5), 1472-1480.
| Crossref | Google Scholar |
Lavery HJ, Seton D (1974) Mammals and birds of the lower Burdekin River district, north Queensland 2. Birds. Queensland Journal of Agricultural and Animal Sciences 31, 371-382.
| Google Scholar |
Lemly AD, Kingsford RT, Thompson JR (2000) Irrigated agriculture and wildlife conservation: conflict on a global scale. Environmental Management 25, 485-512.
| Crossref | Google Scholar | PubMed |
Li C, Zhang Y, Zha D, Yang S, Huang ZYX, de Boer WF (2019) Assembly processes of waterbird communities across subsidence wetlands in China: a functional and phylogenetic approach. Diversity and Distributions 25(7), 1118-1129.
| Crossref | Google Scholar |
Ma Z, Cai Y, Li B, Chen J (2010) Managing Wetland habitats for waterbirds: an international perspective. Wetlands 30, 15-27.
| Crossref | Google Scholar |
Morton SR, Brennan KG, Armstrong MD (1990a) Distribution and abundance of ducks in the Alligator Rivers Region, Northern Territory. Australian Wildlife Research 17(6), 573-590.
| Crossref | Google Scholar |
Morton SR, Brennan KG, Armstrong MD (1990b) Distribution and abundance of magpie geese, Anseranas semipalmata, in the Alligator Rivers Region, Northern Territory. Australian Journal of Ecology 15(3), 307-320.
| Crossref | Google Scholar |
Morton SR, Brennan KG, Armstrong MD (1993a) Distribution and abundance of grebes, pelicans, darters, cormorants, rails and terns in the Alligator Rivers Region, Northern Territory. Wildlife Research 20(2), 203-217.
| Crossref | Google Scholar |
Morton SR, Brennan KG, Armstrong MD (1993b) Distribution and abundance of herons, egrets, ibises and spoonbills in the Alligator Rivers Region, Northern Territory. Wildlife Research 20(1), 23-43.
| Crossref | Google Scholar |
Murray CG, Loyn RH, Kasel S, Hepworth G, Stamation K, Hamilton AJ (2012) What can a database compiled over 22 years tell us about the use of different types of wetlands by waterfowl in south-eastern Australian summers? Emu - Austral Ornithology 112(3), 209-217.
| Crossref | Google Scholar |
NQ Dry Tropics (2016) Burdekin Dry Tropics Natural Resource Management Plan 2016–2026. Available at nrm.nqdrytropics.com.au/downloadpdf/ [accessed 5 March 2024]
NQ Dry Tropics (2024) Restoration of the Ramsar Wetlands of Bowling Green Bay Catchment (2019-2023). Available at www.nqdrytropics.com.au/projects/waterways-wetlands-and-coasts-program/restoration-of-the-ramsar-wetlands-of-bowling-green-bay-catchment-2019-2023/ [accessed 4 March 2024]
Osland MJ, Chivoiu B, Enwright NM, Thorne KM, Guntenspergen GR, Grace JB, Dale LL, Brooks W, Herold N, Day JW, Sklar FH, Swarzenzki CM (2022) Migration and transformation of coastal wetlands in response to rising seas. Science Advances 8(26), eabo5174.
| Crossref | Google Scholar |
Patterson BD, Atmar W (1986) Nested subsets and the structure of insular mammalian faunas and archipelagos. Biological Journal of the Linnean Society 28(1–2), 65-82.
| Crossref | Google Scholar |
Pearson RG, Connolly N, Benson LJ, Cairns A, Clayton P, Crossland M, Hortle KG, Leonard K, Nolen J (2019) Invertebrate responses to land use in tropical streams: discrimination of impacts enhanced by analysis of discrete areas. Marine and Freshwater Research 70(4), 563-575.
| Crossref | Google Scholar |
Pearson RG, Connolly NM, Davis AM, Brodie JE (2021) Fresh waters and estuaries of the Great Barrier Reef catchment: Effects and management of anthropogenic disturbance on biodiversity, ecology and connectivity. Marine Pollution Bulletin 166, 112194.
| Crossref | Google Scholar | PubMed |
Pearson RG, Davis AM, Birtles RA (2022) Enhancing whole-of-river conservation. Marine and Freshwater Research 73(6), 729-741.
| Crossref | Google Scholar |
Perna CN, Cappo M, Pusey BJ, Burrows DW, Pearson RG (2012) Removal of aquatic weeds greatly enhances fish community richness and diversity: an example from the Burdekin River floodplain, tropical Australia. River Research and Applications 28(8), 1093-1104.
| Crossref | Google Scholar |
Queensland Government (2018) Wetlandinfo: Walking the Landscape – Lower Burdekin Catchment Story. Available at https://wetlandinfo.des.qld.gov.au/wetlands/ecology/processes-systems/water/catchment-stories/ [accessed 20 August 2024]
Queensland Government (2024) Wetlandinfo: Burdekin-Townsville Coastal Aggregation. Available at wetlandinfo.des.qld.gov.au/wetlands/facts-maps/diwa-wetland-burdekin-townsville-coastal-aggregation/ [accessed 4 March 2024]
Sica YV, Quintana RD, Bernardos JN, Calamari NC, Gavier-Pizarro GI (2020) Wetland bird response to habitat composition and configuration at multiple spatial scales. Wetlands 40, 2513-2525.
| Crossref | Google Scholar |
Stevens BS, Conway CJ (2020) Predictive multi-scale occupancy models at range-wide extents: Effects of habitat and human disturbance on distributions of wetland birds. Diversity and Distributions 26(1), 34-48.
| Crossref | Google Scholar |
Sunwater (2024) Burdekin Haughton Water Supply Scheme. Available at www.sunwater.com.au/schemes/burdekin-haughton/ [accessed 15 August 2024]
Tibby J, Barr C, Marshall JC, Richards J, Perna C, Fluin J, Cadd HR (2019) Assessing the relative impacts of land-use change and river regulation on Burdekin River (Australia) floodplain wetlands. Aquatic Conservation: Marine and Freshwater Ecosystems 29(10), 1712-1725.
| Crossref | Google Scholar |
Tickner D, Opperman JJ, Abell R, Acreman M, Arthington AH, Bunn SE, Cooke SJ, Dalton J, Darwall W, Edwards G, Harrison I, Hughes K, Jones T, Leclère D, Lynch AJ, Leonard P, McClain ME, Muruven D, Olden JD, Ormerod SJ, Robinson J, Tharme RE, Thieme M, Tockner K, Wright M, Young L (2020) Bending the curve of global freshwater biodiversity loss: an emergency recovery plan. BioScience 70(4), 330-342.
| Crossref | Google Scholar | PubMed |
Waltham NJ, Coleman L, Buelow C, Fry S, Burrows D (2020) Restoring fish habitat values on a tropical agricultural floodplain: Learning from two decades of aquatic invasive plant maintenance efforts. Ocean & Coastal Management 198, 105355.
| Crossref | Google Scholar |
Whiteoak K, Binney J (2012) Literature review of the economic value of ecosystem services that wetlands provide. Marsden Jacob Associates/Australian Department of Sustainability, Environment, Water, Population and Communities. Available at www.environment.gov.au/water/publications/environmental/wetlands/index.html [accessed 5 March 2024]