Silver nanoparticles protect tillering in drought-stressed wheat by improving leaf water relations and physiological functioning
Muhammad Sarwar A , Muhammad Farrukh Saleem A , Najeeb Ullah

A Department of Agronomy, University of Agriculture, Faisalabad, Pakistan.
B Agricultural Research Station, Office of VP for Research and Graduate Studies, Qatar University, Doha 2713, Qatar.
C Department of Agronomy, The University of Lahore, Lahore, Pakistan.
D Agronomic Research Station, Bahawalpur, Pakistan.
Functional Plant Biology 50(11) 901-914 https://doi.org/10.1071/FP23036
Submitted: 21 February 2023 Accepted: 6 July 2023 Published: 25 July 2023
Abstract
The tillering phase of wheat (Triticum aestivum) crops is extremely susceptible to drought. We explored the potential of silver nanoparticles (AgNPs) in protecting wheat genotypes from drought injury during this sensitive stage. After treating with AgNPs (60 ppm), the plants were submitted to different water levels; i.e. 100% field capacity (FC), 75% FC (mild drought), 50% FC (moderate drought) and 25% FC (severe drought) from 15 to 41 days after sowing (tillering phase). Leaf physiological data were collected at stress termination, while yield attributes were recorded at crop maturity. We found that increasing drought intensity significantly impaired leaf physiology and grain yield of both studied genotypes. Compared with control, moderately and severely drought-stressed plants produced 25% and 45% lesser grain yield per spike, respectively (averaged across genotypes and years of study). Likewise, moderate and severe drought reduced photosynthesis by 49% and 76%, respectively, compared with control. In contrast, AgNPs significantly restored leaf physiological functioning and grain yield formation at maturity. For example, under moderate and severe drought, AgNPs-treated plants produced 22% and 17% more grains per plant, respectively, than their respective water-treated plants. Our study suggests that exogenous AgNPs can protect wheat crops from drought during early development stages.
Keywords: cellular biochemistry, drought-tolerance, grain yield, osmo-protectants, senescence, silver nanoparticles, tiller formation, water relations.
Introduction
Global warming and erratic changes in weather patterns are among the major factors affecting crop growth and yields (Ahmad et al. 2022a). Most countries that produce wheat (Triticum aestivum) experience frequent and prolonged drought, particularly during sensitive developmental phases (Lesk et al. 2016; El Sabagh et al. 2019). Drought-induced loss in global wheat yields has been estimated at 20.6% over the past three to four decades (Daryanto et al. 2016). Tillering in winter wheat begins 20–25 days after sowing and this phase is highly sensitive to stress (Fleury et al. 2010). As tillering contributes to 87% of wheat grain yield, any stress during this stage reduces the effective tillers and grain numbers (Tilley et al. 2019). Water shortage prevails mostly at wheat tillering in most of the wheat-growing regions of south Asia, and data suggests that drought during this growth stage is the most common cause of grain yield losses than any other growth stage (Ihsan et al. 2016). Similarly, in many wheat-growing regions, water scarcity is already a major constraint to agricultural productivity and climate change is expected to exacerbate this problem. Studies have shown that under current climate conditions, drought events during the tillering phase of wheat are already frequent and intense in many regions, such as the Mediterranean, south Asia and north Africa (Saeidi et al. 2015; Zhang et al. 2018; Figueroa-Bustos et al. 2019). Moreover, climate projections suggest that drought frequency and intensity will increase in many of these regions in the future (Naumann et al. 2021).
Wheat is classified as moderately drought-stressed when field capacity (FC) drops between 45% and 60%, and severe drought occurs between 25% and 30% of FC (Munsif et al. 2022). Moderate to severe drought stress at wheat tillering for 10 days decreases wheat yield by up to 16% (Abid et al. 2018). Drought at wheat tillering affects the gas exchange components (Pn and Gs) and the chlorophyll fluorescence (Fv/Fm) (Aslam et al. 2022; Hickey et al. 2022). Bukhari et al. (2021) documented that severe drought stress during wheat tillering limits the photosynthetic rate and minimises carbohydrate assimilation. Drought in wheat affects the top-leaf water relations by decreasing the leaf water potential and increasing the leaf osmotic potential (Nawaz et al. 2012).
Drought can be mitigated by growing tolerant genotypes and crop management; e.g. supplemental irrigation (Bayoumi et al. 2008; Dhakal 2021), application of nutrients/growth regulators (Maswada et al. 2021; Aurangzaib et al. 2022) and nanoparticles. Recent studies suggested the high efficiency of foliar spray of nanoparticles for managing drought-stressed wheat (Soliman et al. 2022). Silver nanoparticles (AgNPs) have a strong signalling capability in field crops under drought/abiotic stress, maintaining a favourable water gradient within leaves (Taran et al. 2017; Naqvi et al. 2022). Few workers have reported the mitigatory drought role of AgNPs under drought stress in wheat and other crop plants (Hojjat and Ganjali 2016; Ahmed et al. 2021). Exogenous application of AgNPs increases grain yield in wheat and eggplant under drought stress by maintaining a favourable water potential and protecting the membranes from oxidative stress. According to Iqbal et al. (2019) and Alabdallah et al. (2021), there is little information available on the effects of moderate to severe drought stress from pre-tillering to the end of tillering and no work has been done to our knowledge on the role of AgNPs under moderate to severe drought stresses at wheat tillering. The objectives of this study were to: (1) quantify the impact of moderate to severe drought at tillering on tolerant and sensitive wheat genotypes; and (2) investigate the roles of AgNPs in restraining grain yield, physiology, water relations and plant defensive system from moderate to severe drought stresses at tillering.
Materials and methods
Experimental conditions
Pot experiments were carried out in the wirehouse facility of the University of Agriculture in Faisalabad, Pakistan (31°26′N, 73°06′E; altitude, 184.4 m) during the Rabi (wet) seasons of 2018–19 and 2019–20. Weather data were collected from the metrological observatory of the University of Agriculture in Faisalabad. Weather data for the cropping seasons are in Fig. 1. The soil used in the experiments was analysed before crop planting with properties: organic matter 1.20 ± 0.024%; pH 8.1 ± 0.2; electrical conductivity (Ec-dS/m) 0.55 ± 0.014; available potassium 212 ± 6.0 ppm; available phosphorous 23.2 ± 0.6 ppm; available zinc 1.33 ± 0.034 ppm; and available boron 0.7 ± 0.016 ppm. Each pot was filled with 18 kg of soil in a peat:silt mix (3:1 ratio). Each earthen pot (35 × 25 cm, length × width, 14.7 L capacity) received 15 seeds, which were thinned to 10 seedlings per pot 1 week after germination and each pot received 2.24 g of urea (containing 46% nitrogen). Half of the nitrogen was applied at the time of sowing and the other half was applied 20 days later.
Applying the water treatments
Five days after the main stem had two unfolded leaves (Zodak 12) and before the onset of tillering, different field capacities (FC)/drought levels were maintained in separate pots; i.e. 100% FC (optimal water condition), 75% FC (mild drought stress), 50% FC (moderate drought stress) and 25% FC (severe drought stress), and continued until the last tiller was visible (at 26 days). For each FC treatment, four pots were used. The method described by Ihsan et al. (2016) was used to calculate soil field capacity. To determine the FC treatements, 500 g of soil was oven-dried and then reweighed. The soil saturation paste was created by pouring water and stirring with a scapula. The saturation point was defined as the point at which water began accumulating as a thin layer on the soil surface. As 100% FC, half of the saturation point was used. Similarly, to maintain respective field capacities of 75%, 50% and 25%, water was added daily to maintain the respective FC treatments (Ihsan et al. 2016). After applying thedrought stress, the same agronomic conditions were used for all the pots throughout the experiment.
Silver nanoparticles (AgNPs) were sprayed early in the morning, 1 day before the drought levels were maintained. Tween-20 (0.1% solution) was used as a surfactant and carboxymethyl cellulose (5% solution) as a sticking agent for foliar application. AgNPs were chemically synthesised by reacting silver nitrate (AgNO3) with trisodium citrate (Na3C6H5O7·2H2O) and analysed by scanning electron microscope (SEM) for size analysis using the methods of Jhanzab et al. (2019) and Yasmeen et al. (2015). The AgNPs were uniform, highly pure and fine, spherical in shape, with sizes ranging from 15 to 20 nm. In this study, we used two wheat (Triticum aestivum L.) genotypes: (1) FSD-08 (relatively drought tolerant); and (2) Galaxy-13 (relatively drought sensitive). Both genotypes are commercially grown in wheat-growing areas of Pakistan. The seeds were obtained from the Wheat Research Institute, Ayub Agricultural Research Institute, Faisalabad, Pakistan. These genotypes were screened for drought tolerance in a preliminary experiment based on their relative cell injury (RCI), net photosynthetic rate, stomatal conductance, chlorophyll fluorescence and seed yield (data not shown). The experimental treatments were arranged under a completely randomised design with factorial arrangements, with four replications for each treatment.
Leaf biochemical attributes
Three top leaves were collected from each pot at the end of the drought stress treatments for biochemical analysis and a composite sample was prepared from the leaves of each pot. A 0.5-g leaf sample was taken from the composite sample for extraction of enzyme and malondialdehyde (MDA) contents. Superoxide dismutase (SOD) (U mg−1 protein) activity was measured by inhibiting nitro blue tetrazolium. In an ELISA plate, a reaction mixture of 100 μL enzyme extract was used and absorbance was measured at 560 nm using an ELISA plate reader (Giannopolitis and Ries 1977). Guaiacol oxidation was used to quantify leaf peroxidase (POD) activity (U mg−1 protein) and reaction mixtures were placed in an ELISA plate along with 150 μL enzyme extract and enzyme quantity was measured at 470 nm (Liu et al. 2009). Using the protocol described by Liu et al. (2009), hydrogen peroxide reactant was used to quantify catalase (CAT) activity (U mg−1 protein). In an ELISA plate reader, a reaction mixture containing 150 μL enzyme extract was used and the absorbance was measured at 240 nm. Leaf proline content was determined by extracting 0.5 g of leaves in 3% of 5 mL sulfosalicylic acid. The extraction mixture was centrifuged for 15 min. A volume of 1 mL supernatant from the leaf extraction mixture was then added, followed by 1 mL 3% ninhydrin (prepared in equal parts 6 M orthophosphoric acid and glacial acetic acid) and 1 mL glacial acetic acid. The mixture was vortexed for 10 min before being incubated at 100°C for 1 h and cooled in an ice water bath. Toluene (1 mL) was then added, vortexed and the upper aqueous layer was discarded. The lower organic layer of 150 μL was transferred to an ELISA plate and absorbance was measured at 520 nm using toluene as a blank for a standard curve on an ELISA plate reader (Bates et al. 1973). To quantify glycine betaine, 0.5 g of leaves were homogenised with 5 mL of deionised (DI) water and centrifuged for 5 min. A 10 mL solution of potassium triiodide was made by combining 7.5 g iodine and 10 g potassium iodide in 1 M HCl, after which 1 mL leaf extracts, 0.1 mL potassium triiodide solution and1 mL HCl (2M) were mixed and incubated at 4°C for 1 h before adding 5 mL chilled DI water and 10 mL 1,2-di-di-chloroethane and vortexed for 5 min. The upper aqueous layer was removed and absorbance at 365 nm was measured using an ELISA plate reader and an organic layer (Grieve and Grattan 1983). MDA contents of leaves were determined using the procedure described by (Cakmak and Horst 1991). The supernatant was extracted after homogenising a 0.5-g leaf sample with 3 mL of 0.1% (w/v) trichloroacetic acid (TCA). A volume of 20% TCA (3 mL) solution and 0.5% thiobarbituric acid were added to 0.5 mL of supernatant. A mixture, leaf extract and a blank were added to an ELISA plate and the absorbance was measured at 532 nm and 600 nm.
Leaf water relations
After drought termination, three top leaves from each pot were collected at pre-dawn for water relations (water potential and osmotic potential) and chlorophyll contents, as previously described by Arnon (1949) and Silveira et al. (2009). Leaf water potential (−MPa) was determined immediately after sampling using a Scholander-type pressure chamber (ARIMAD 2, Korea) following the methodology of Scholander et al. (1965). For leaf osmotic potential, the leaves were stored at −20°C for 1 week and then thawed. The osmotic potential of the extracted sap was determined using an osmometer (Osmomat 030).
Relative cell injury (RCI)
Three top leaves from each pot were collected and averaged at 12:00 hours after the drought stress period ended. Two leaf discs with a diameter of 10 mm were obtained from both sides of the top leaves. Both leaf discs were washed three to four times with double distilled water before being placed in test tubes containing deionised water. One set of test tubes was treated in a 50°C water bath for 1 h, while the other set was kept at room temperature at 25°C for an hour. An electrical conductivity (EC) meter was used to measure both heat-treated and control test tubes’ initial EC at room temperature (Model, Jenway 4510, Japan). Following the initial EC, both test tubes were autoclaved for 10 min at 0.1 MPa pressure (Model, HAU-85, Hirayam instruments, Kasukabe-shi Saitama, Japan).
The final EC of both test tubes was determined at room temperature. RCI was determined according to Sullivan (1972):
where C1 and C2 are the initial and final EC of control test tubes, respectively; and T1 and T2 are the initial and final EC readings of heat-treated test tubes, respectively.Photosynthetic parameters
Between 10:00 hours and 12:00 hours, top leaf gas exchange components and chlorophyll fluorescence (Fv/Fm) were measured and averaged from three leaves in each pot immediately after drought stress removal (42 days after sowing). An infra-red gas analyser (LCi Analyzer with Broad Head, Part Number LCi-002/B with Serial Number 32455) was used to determine the net photosynthetic rate (Pn, mol m−2 s−1) and stomatal conductance (Gs, mol m−2 s−1). The gas exchange characteristics of top leaves were measured. After 20 min of dark adaptation of leaves, Fv/Fm was measured using a multi-mode chlorophyll fluorometer (Serial Number 0729501, OptiScience, UK). Maximum Fv/Fm was calculated as a plant stress indicator (Prasad et al. 2008).
After stopping the drought stress, three top leaves from each pot were collected before sunset to determine chlorophyll a and b content. A total of 0.5 g sub-sample was taken from each pot leaf and soaked overnight in 80% acetone. In an ELISA plate, a blank with 80% acetone and 1.5 L leaf extracts was placed and absorbance was measured at 645 and 663 nm wavelengths in an ELISA plate reader for chlorophyll a and b contents (Arnon 1949). The same leaves of non-destructive measurements (gas exchange components) were used for destructive measurements (leaf biochemistry attributes, water relations and chlorophyll contents).
Top leaves senescence and yield attributes
Three top leaves from each pot were tagged to measure the leaf senescence at the end of drought treatment while yield per spike and grain weight per spike were recorded after harvesting the crop. The senescence of top leaves on selected plants was measured 1 day before and 7 days after drought stress. The senescence percentage of selected leaves was calculated by averaging the values measured with a measuring scale. The crop was harvested 125 days after sowing and the number of grains per spike (NGPS), grain weight per spike (GWPS-gram) and 1000-grain weight were recorded and averaged from the selected plants.
Statistical analysis
A three-way ANOVA was used to evaluate the effect of drought levels, AgNPs and genotypes and data (P < 0.01) were statistically analysed using Fisher’s analysis of variance technique (Steel and Torrie 1960). Using STATISTIX 10.1 software, Tukey’s honestly significant difference (HSD) test was used to compare the means at 1% (Gomez and Gomez 2010). A separate ANOVA was run for each factor before running the combined ANOVA. The graphs were formed in MS Excel 2016 and the correlation matrix and Principal Component Analysis (PCA) were carried out in XL-STAT software.
Results
Grain yield components under drought and application of AgNPs
Individual and interactive effects of water levels, genotypes and AgNPs on wheat yield attributes were significant during both years of study (P < 0.01) (Fig. 2). Among the water levels, 50% (moderate drought) and 25% (severe drought) of FC significantly reduced wheat yield attributes compared to 100% FC (optimal water conditions); however, non-significant effects of 75% FC (mild drought) were observed on grain yield attributes. Averaged across 2 years, moderate and severe drought reduced the number of tillers per plant by 33% and 57%, respectively, over the optimal water conditions. Both tolerant and sensitive genotypes were significantly affected by moderate and severe drought stresses, although the degree of damage significantly varied across the genotypes. For example, the sensitive genotype produced 14% and 24% fewer tillers in response to moderate and severe drought, respectively, compared with the tolerant genotype (averaged across both years of study) (Fig. 2a). Among the yield attributes, 1000-grain weight was reduced by 29% and 44% (Fig. 2b), respectively, and grain numbers per plant were reduced by 42% and 62% (Fig. 2c) in moderate to severe drought stresses, respectively (averaged across both years of study), compared with control. Similarly, under moderate and severe drought conditions, total grain weight per plant was significantly reduced (Fig. 2d). Drought (moderate to severe) negatively affected the drought-sensitive genotype (Galaxy-13) more than the drought-tolerant genotype (FSD-08) during both years of study. For example, the sensitive genotype experienced 42% and 51% more reduction in 1000-grain weight and grain numbers per plant, respectively under severe drought than the tolerant genotype (averaged across both years of study) (Fig. 2b, d). Similarly, grain weight per plant was reduced in drought-sensitive over drought tolerant genotype (Fig. 2c). Overall, severe drought reduced wheat tillers by 69% and grain numbers by 52% when compared with optimal conditions.
Effect of moisture regimes at tillering of wheat genotypes and foliar spray of AgNPs (moisture regimes × AgNPs × genotypes, P < 0.01; years × moisture regimes × AgNPs × genotypes, P > 0.05) on (a) number of tillers per plant, (b) 1000-grain weight, (c) grain weight per spike, and (d) number of grains per spike. Values are the means of four replications (n = 4) ± s.e. and treatments with the same letters are not statistically significant at P > 0.01. Levels of significance: drought levels = **; genotypes = **; foliar spray of AgNPs = **; years = n.s.; drought × genotypes = **; drought × AgNPs = **; genotypes × AgNPs = **; drought × genotypes × AgNPs = **; years × drought = n.s.; years × genotypes = n.s.; years × AgNPs = n.s.; years × drought × genotypes = n.s.; years × drought × AgNPs = n.s.; years × genotypes × AgNPs = n.s., years × drought × genotypes × AgNPs = n.s. NGPS, number of grains per spike; FC, field capacity. **P < 0.01; n.s., not significant.
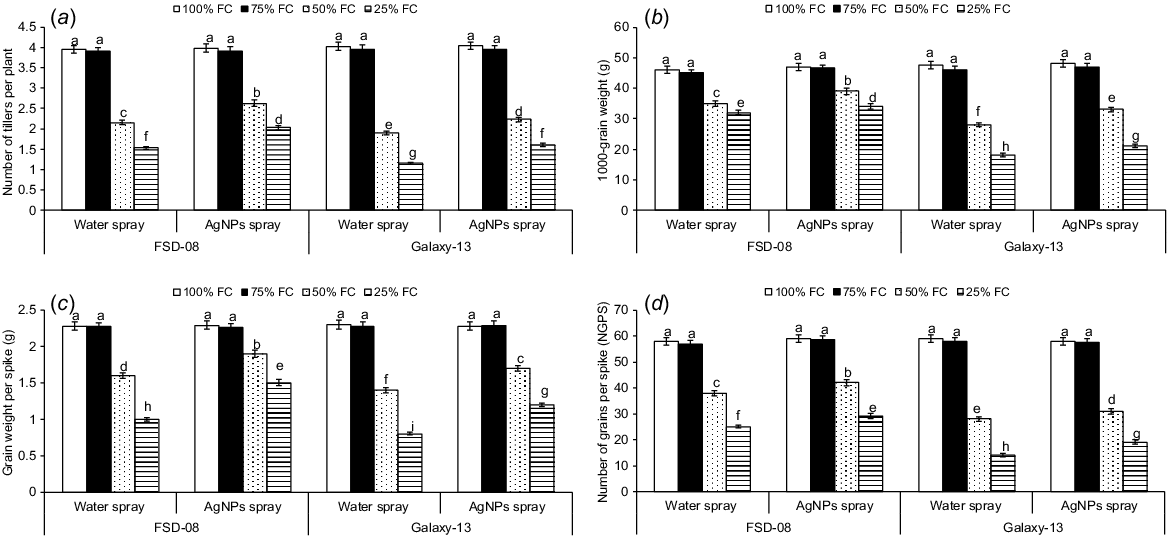
AgNPs increased the number of tillers and yield attributes under moderate and severe drought in both wheat genotypes (Fig. 2). For example, AgNPs treated plants produced 19% and 29% more tillers under moderate and severe drought, respectively, compared to the water-treated plants. However, AgNPs did not affect tillers per plant under mild drought and optimal conditions. When comparing the genotypes, AgNPs increased tillers per plant by 35% and 20% in the drought-tolerant genotype under moderate and severe drought, respectively, compared to the sensitive genotype (Fig. 2a). Similarly, AgNPs increased 1000-grain weight by 14% and 12%, respectively, and grain numbers per plant by 13% and 11%, from moderate to severe drought, respectively, compared to the water-treated plants (Fig. 2b, d). Across the genotypes, AgNPs increased grain numbers per plant by 38% and 37%, respectively, and grain weight per plant by 12% and 18% in the tolerant genotype under moderate and severe drought, compared to the drought-sensitive genotype (Fig. 2c, d). However, AgNPs significantly increased grain numbers and grain weight per plant in both tolerant and sensitive genotypes under moderate and severe drought. Overall, AgNPs increased grain numbers and grain weight per plant by 10% and 30%, respectively, in moderate and severe drought stresses (averaged across) when compared to the corresponding water-treated plants.
Leaf physiological changes in response to drought application of AgNPs
Drought levels, genotypes and AgNPs treatment all significantly affected top leaves senescence, gas exchange, chlorophyll fluorescence and water relations at tillering stage (drought × genotypes × AgNPs; P < 0.01) (Fig. 3, Tables 1 and 2). Compared with optimal conditions, top leaf senescence was amplified by 30% and 48% as the drought level increased from moderate to severe drought stresses. Across the genotypes, the sensitive genotype showed 15% and 26% higher top leaf senescence than the tolerant genotype under moderate and severe drought stresses, respectively (Fig. 3).
Effect of moisture regimes at tillering of wheat genotypes, growth seasons and foliar spray of AgNPs (moisture regimes × AgNPs × genotypes, P < 0.01; years × moisture regimes × AgNPs × genotypes, P > 0.05) on flag leaf senescence of wheat. Values are the means of four replications (n = 4) ± s.e. and treatments with the same letters are not statistically significant at P > 0.01. Levels of significance: drought levels = **; henotypes = **; foliar spray of AgNPs = **; years = n.s.; drought × genotypes = **; drought × AgNPs = **; henotypes × AgNPs = **; drought × genotypes × AgNPs = **; years × drought = n.s.; years × genotypes = n.s.; years × AgNPs = n.s.; years × drought × genotypes = n.s.; years × drought × AgNPs = n.s.; years × genotypes × AgNPs = n.s.; years × drought × genotypes × AgNPs = n.s. FC, field capacity. **P < 0.01; n.s., not significant.
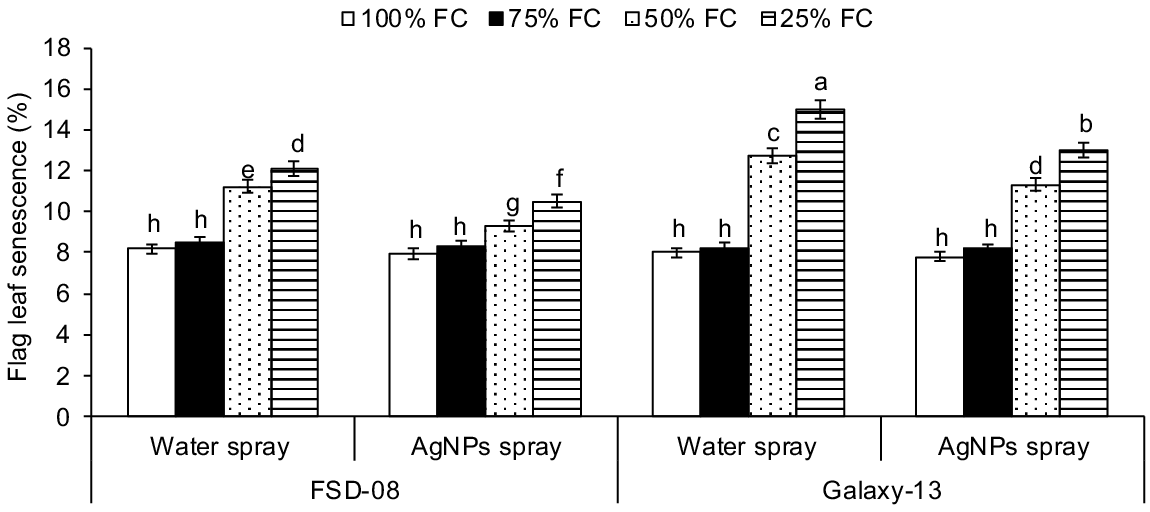
Drought levels | Foliar spray of AgNPs (60 ppm) | Genotypes | SOD (U mg−1 protein) | POD (U mg−1 protein) | CAT (U mg−1 protein) | Proline contents (μmol g−1) | GB (μmol g−1) | MDA (nmol g−1 FW) | Ψw (−MPa) | Ψs (−MPa) | |
---|---|---|---|---|---|---|---|---|---|---|---|
100% field capacity | Water spray | FSD-08 | 94 ± 2.3a | 41 ± 1.01a | 83 ± 2.1ab | 1.20 ± 0.03a | 165 ± 4.2a | 0.63 ± 0.014a | 0.67 ± 0.007a | 0.64 ± 0.015a | |
Galaxy-13 | 93 ± 2.2a | 38 ± 0.95ab | 80 ± 2.0b | 1.23 ± 0.04a | 158 ± 3.9a | 0.62 ± 0.018a | 0.69 ± 0.008a | 0.63 ± 0.014a | |||
AgNPs | FSD-08 | 98 ± 2.6a | 43 ± 1.07a | 95 ± 2.3a | 1.22 ± 0.03a | 164 ± 4.1a | 0.60 ± 0.011a | 0.69 ± 0.008a | 0.65 ± 0.016a | ||
Galaxy-13 | 99 ± 2.7a | 44 ± 1.10a | 90 ± 2.2a | 1.24 ± 0.04a | 160 ± 4.0a | 0.61 ± 0.015a | 0.70 ± 0.009a | 0.66 ± 0.016a | |||
75% field capacity | Water spray | FSD-08 | 96 ± 2.5a | 42 ± 1.05ab | 87 ± 2.1b | 1.24 ± 0.04a | 167 ± 4.3a | 0.64 ± 0.014a | 0.70 ± 0.009a | 0.62 ± 0.014a | |
Galaxy-13 | 97 ± 2.6a | 41 ± 1.02b | 85 ± 2.1b | 1.20 ± 0.03a | 161 ± 3.9a | 0.63 ± 0.018a | 0.69 ± 0.008a | 0.65 ± 0.015a | |||
AgNPs | FSD-08 | 100 ± 2.6a | 45 ± 1.13a | 99 ± 2.5a | 1.29 ± 0.04a | 169 ± 4.5a | 0.61 ± 0.011a | 0.72 ± 0.010a | 0.66 ± 0.016a | ||
Galaxy-13 | 102 ± 2.8a | 46 ± 1.16a | 101 ± 2.5a | 1.27 ± 0.03a | 165 ± 4.2a | 0. 62 ± 0.015a | 0.73 ± 0.010a | 0.67 ± 0.016a | |||
50% field capacity | Water spray | FSD-08 | 125 ± 3.0c | 78 ± 1.95c | 130 ± 3.2c | 1.80 ± 0.06c | 225 ± 5.6c | 1.10 ± 0.028b | 0.99 ± 0.027b | 1.30 ± 0.040b | |
Galaxy-13 | 114 ± 2.9d | 61 ± 1.53d | 115 ± 2.7d | 1.60 ± 0.05d | 213 ± 5.3d | 1.35 ± 0.038a | 1.21 ± 0.034a | 1.50 ± 0.046a | |||
AgNPs | FSD-08 | 180 ± 6.8a | 127 ± 3.13a | 285 ± 7.1a | 2.50 ± 0.07a | 275 ± 6.9a | 0.73 ± 0.020d | 0.86 ± 0.013c | 1.07 ± 0.033c | ||
Galaxy-13 | 150 ± 6.0b | 114 ± 2.91b | 260 ± 6.5b | 2.20 ± 0.06b | 245 ± 6.1b | 0.83 ± 0.023c | 1.07 ± 0.028b | 1.25 ± 0.039b | |||
25% field capacity | Water spray | FSD-08 | 141 ± 3.5c | 87 ± 2.18c | 140 ± 3.5c | 1.95 ± 0.05c | 240 ± 6.0c | 1.45 ± 0.037b | 1.18 ± 0.036b | 1.88 ± 0.045c | |
Galaxy-13 | 120 ± 3.0d | 67 ± 1.68d | 123 ± 3.1d | 1.80 ± 0.05d | 220 ± 5.5d | 1.70 ± 0.049a | 1.66 ± 0.041a | 2.11 ± 0.052a | |||
AgNPs | FSD-08 | 263 ± 6.3a | 137 ± 3.3a | 292 ± 7.1a | 2.70 ± 0.07a | 285 ± 7.2a | 0.86 ± 0.025d | 0.96 ± 0.029d | 1.62 ± 0.040d | ||
Galaxy-13 | 248 ± 5.9b | 122 ± 3.01b | 271 ± 6.7b | 2.30 ± 0.06b | 257 ± 6.5b | 1.06 ± 0.029c | 1.36 ± 0.035c | 1.96 ± 0.048b | |||
HSD | 8.6 | 3.90 | 8.92 | 0.082 | 10.1 | 0.048 | 0.056 | 0.071 | |||
Levels of significance | |||||||||||
Drought levels | ** | ** | ** | ** | ** | ** | ** | ** | |||
Genotypes | ** | ** | ** | ** | ** | ** | ** | ** | |||
Foliar spray of AgNPs | ** | ** | ** | ** | ** | ** | ** | ** | |||
Years | n.s. | n.s. | n.s. | n.s. | n.s. | n.s. | n.s. | n.s. | |||
Drought × genotypes | ** | ** | ** | ** | ** | ** | ** | ** | |||
Drought × AgNPs | ** | ** | ** | ** | ** | ** | ** | ** | |||
Genotypes × AgNPs | ** | ** | ** | ** | ** | ** | ** | ** | |||
Drought × genotypes × AgNPs | ** | ** | ** | ** | ** | ** | ** | ** | |||
Years × drought | n.s. | n.s. | n.s. | n.s. | n.s. | n.s. | n.s. | n.s. | |||
Years × genotypes | n.s. | n.s. | n.s. | n.s. | n.s. | n.s. | n.s. | n.s. | |||
Years × AgNPs | n.s. | n.s. | n.s. | n.s. | n.s. | n.s. | n.s. | n.s. | |||
Years × drought × genotypes | n.s. | n.s. | n.s. | n.s. | n.s. | n.s. | n.s. | n.s. | |||
Years × drought × AgNPs | n.s. | n.s. | n.s. | n.s. | n.s. | n.s. | n.s. | n.s. | |||
Years × genotypes × AgNPs | n.s. | n.s. | n.s. | n.s. | n.s. | n.s. | n.s. | n.s. | |||
Years × drought × genotypes × AgNPs | n.s. | n.s. | n.s. | n.s. | n.s. | n.s. | n.s. | n.s. |
Values are the means ± s.e. (n = 4).
Treatments with the same letters are not statistically significant at P > 0.01.
FSD-08, drought tolerant; Galaxy-13, drought susceptible; SOD, superoxide dismutase; POD, peroxidase; CAT, catalase; GB, glycine betaine; MDA, malondialdehyde; Ψw, water potential; ΨS, solute potential; AgNPs, silver nanoparticles; HSD, honestly significant difference.
**P < 0.01; n.s., not significant.
Drought levels | Foliar spray of AgNPs (60 ppm) | Genotypes | RCI (%) | Pn (μmol m−2 s−1) | Gs (m mol m−2 s−1) | Fv/Fm | Chlorophyll a (mg g−1 FW) | Chlorophyll b (mg g−1 FW) | |
---|---|---|---|---|---|---|---|---|---|
100% field capacity | Water spray | FSD-08 | 40 ± 1.2b | 29.5 ± 0.72b | 0.91 ± 0.019a | 0.92 ± 0.023a | 1.73 ± 0.041a | 0.26 ± 0.006a | |
Galaxy-13 | 51 ± 1.3a | 29.4 ± 0.71b | 0.89 ± 0.018a | 0.91 ± 0.021a | 1.74 ± 0.043a | 0.27 ± 0.007a | |||
AgNPs | FSD-08 | 28 ± 0.7c | 30.0 ± 0.75a | 0.93 ± 0.022a | 0.93 ± 0.022a | 1.75 ± 0.044a | 0.23 ± 0.005a | ||
Galaxy-13 | 41 ± 1.1b | 30.5 ± 0.76a | 0.92 ± 0.021a | 0.92 ± 0.021a | 1.76 ± 0.045a | 0.24 ± 0.006a | |||
75% field capacity | Water spray | FSD-08 | 43 ± 1.3b | 29.3 ± 0.72b | 0.90 ± 0.019ab | 0.94 ± 0.024a | 1.75 ± 0.043a | 0.25 ± 0.006a | |
Galaxy-13 | 55 ± 1.4a | 29.5 ± 0.73b | 0.91 ± 0.020a | 0.93 ± 0.023a | 1.74 ± 0.042a | 0.26 ± 0.005a | |||
AgNPs | FSD-08 | 32 ± 0.8c | 30.2 ± 0.76a | 0.94 ± 0.023a | 0.94 ± 0.024a | 1.76 ± 0.044a | 0.24 ± 0.004a | ||
Galaxy-13 | 45 ± 1.2b | 30.7 ± 0.77a | 0.93 ± 0.022a | 0.95 ± 0.025a | 1.77 ± 0.045a | 0.23 ± 0.005a | |||
50% field capacity | Water spray | FSD-08 | 60 ± 1.5b | 16.8 ± 0.53b | 0.58 ± 0.012c | 0.59 ± 0.013b | 1.26 ± 0.023c | 1.02 ± 0.025b | |
Galaxy-13 | 80 ± 2.2a | 13.4 ± 0.43d | 0.49 ± 0.008d | 0.46 ± 0.011c | 0.92 ± 0.020d | 1.14 ± 0.027a | |||
AgNPs | FSD-08 | 47 ± 1.3c | 20.3 ± 0.61a | 0.71 ± 0.017a | 0.72 ± 0.017a | 1.44 ± 0.030a | 0.74 ± 0.014d | ||
Galaxy-13 | 60 ± 1.5b | 17 ± 0.55c | 0.59 ± 0.014b | 0.53 ± 0.013b | 1.11 ± 0.025b | 0.90 ± 0.021c | |||
25% field capacity | Water spray | FSD-08 | 85 ± 2.3b | 12.2 ± 0.33b | 0.44 ± 0.009c | 0.46 ± 0.008c | 0.87 ± 0.017c | 1.30 ± 0.033b | |
Galaxy-13 | 94 ± 2.4a | 6.3 ± 0.31d | 0.22 ± 0.007d | 0.23 ± 0.006d | 0.49 ± 0.015d | 1.40 ± 0.035a | |||
AgNPs | FSD-08 | 60 ± 1.5d | 14.2 ± 0.41a | 0.50 ± 0.012a | 0.53 ± 0.010a | 1.13 ± 0.026a | 0.98 ± 0.024d | ||
Galaxy-13 | 70 ± 1.7c | 7.7 ± 0.32c | 0.27 ± 0.011b | 0.27 ± 0.008b | 0.58 ± 0.019b | 1.20 ± 0.028c | |||
HSD | 2.75 | 0.64 | 0.036 | 0.031 | 0.050 | 0.040 | |||
Levels of significance | |||||||||
Drought levels | ** | ** | ** | ** | ** | ** | |||
Genotypes | ** | ** | ** | ** | ** | ** | |||
Foliar spray of AgNPs | ** | ** | ** | ** | ** | ** | |||
Years | n.s. | n.s. | n.s. | n.s. | n.s. | n.s. | |||
Drought × genotypes | ** | ** | ** | ** | ** | ** | |||
Drought × AgNPs | ** | ** | ** | ** | ** | ** | |||
Genotypes × AgNPs | ** | ** | ** | ** | ** | ** | |||
Drought × genotypes × AgNPs | ** | ** | ** | ** | ** | ** | |||
Years × drought | n.s. | n.s. | n.s. | n.s. | n.s. | n.s. | |||
Years × genotypes | n.s. | n.s. | n.s. | n.s. | n.s. | n.s. | |||
Years × AgNPs | n.s. | n.s. | n.s. | n.s. | n.s. | n.s. | |||
Years × drought × genotypes | n.s. | n.s. | n.s. | n.s. | n.s. | n.s. | |||
Years × drought × AgNPs | n.s. | n.s. | n.s. | n.s. | n.s. | n.s. | |||
Years × genotypes × AgNPs | n.s. | n.s. | n.s. | n.s. | n.s. | n.s. | |||
Years × drought × genotypes × AgNPs | n.s. | n.s. | n.s. | n.s. | n.s. | n.s. |
Values are the means ± s.e. (n = 4).
Treatments with the same letters are not statistically significant at P > 0.01.
FSD-08, drought tolerant; Galaxy-13, drought susceptible; RCI, relative cell injury; Pn, net photosynthetic rate; Gs, stomatal conductance; Fv/Fm, chlorophyll fluorescence; Chl, chlorophyll contents; AgNPs, silver nanoparticles; HSD, honestly significant difference.
**P < 0.01; n.s., not significant.
Leaf physiological traits such as Pn, Gs, Fv/Fm and chlorophyll a were reduced by 44%, 40%, 38% and 32%, respectively, under moderate, and by 67%, 62%, 59% and 57%, respectively, under severe drought compared to optimal conditions (averaged across). Across the genotypes, moderate and severe drought caused significantly more damage to the sensitive than tolerant genotype. For example, under severe drought leaf Pn, Gs, Fv/Fm and chlorophyll a content of sensitive genotype were reduced by 48%, 48%, 51% and 47%, respectively, of drought tolerant genotype (averaged across during both years of study) (Table 2).
Both moderate and severe drought significantly affected leaf water and osmotic potential, with a maximum reduction in water potential by 0.79-fold and an increase in solute potential by 1.83-fold in response to optimal water conditions (averaged across) under severe drought. Under moderate and severe drought stresses, the sensitive genotype had 25% and 44% lower water potential, respectively, compared to the tolerant genotype (averaged across both years of study) (Table 1). When comparing the AgNPs effects under drought levels, foliar spray of AgNPs reduced top leaf senescence (Fig. 3) and increased Pn, Gs, Fv/Fm and chorophyll a and b contents under moderate to severe drought stress with a relatively greater increase observed under moderate drought stress (Table 2). For example, AgNPs reduced top leaf senescence by 12% and 13% under moderate and severe drought stresses, respectively, compared to water-treated plants under the same drought stresses. Similarly, AgNPs reduced top leaf senescence by 13% and 11% under moderate to severe drought stresses of the tolerant compared to the sensitive genotype (averaged across both years of study). Exogenous application of AgNPs showed non-significant effects on top leaf senescence, gas exchange components and water relations under optimal water (Fig. 3).
When leaf physiology was compared under various drought levels, AgNPs improved Pn, Gs, Fv/Fm and chlorophyll a by 23%, 24%, 19% and 18%, respectively, under moderate drought stress, while the increase was 13%, 15%, 15% and 24% under severe drought stress, compared to the water-treated plants of corresponding drought treatments averaged across both years of study. Across the genotypes under moderate and severe drought, AgNPs improved Pn, Gs, Fv/Fm, chlorophyll a and water potential by 42%, 43%, 47%, 63% and 26%, respectively, in the tolerant compared to the sensitive genotype (Tables 1 and 2).
Drought levels, genotypes and AgNPs all had a significant effect on antioxidants (SOD, POD, CAT, proline and GB), MDA and RCI (drought × genotypes × AgNPs; P < 0.01) (Tables 1 and 2). Antioxidants, MDA and RCI levels were increased significantly from moderate to severe drought stress compared to optimal water conditions; however, no significant increase in antioxidants, MDA and RCI was observed under mild drought. When compared the drought levels, SOD, proline and GB increased by 40%, 70% and 47%, respectively, under moderate drought stress while the increase was 95%, 83% and 52%, respectively, under severe drought stress over the optimal water conditions averaged across both years of study. Similarly, under severe drought, leaf MDA and RCI levels were increased by 98% and 78%, respectively, over the plants of optimal water conditions averaged across both years of study. From moderate to severe drought stresses, the MDA and RCI have significantly increased both in sensitive and tolerant genotypes, but the most pronounced effect of drought was observed in the sensitive genotype. AgNPs increased antioxidants and reduced MDA contents and RCI from moderate to severe drought stresses, but had no significant effect under mild drought stress and optimal water conditions (Tables 1 and 2). Foliar spray of AgNPs, for example, increased SOD, proline and GB by 33%, 45% and 18%, respectively, under moderate, and 95%, 33% and 17%, under severe drought, compared to their corresponding water-treated plants. Similarly, AgNPs reduced MDA and RCI by 35% and 21%, respectively, under moderate drought stress, and 40% and 27%, respectively, under severe drought stress (averaged across both years of study) compared to water-treated plants of corresponding drought stresses. The effect AgNPs application was significant on both wheat genotypes. When genotypes were compared, AgNPs increased SOD, proline and GB by 61%, 19% and 42%, respectively, more in the drought-tolerant genotype under moderate drought stress than in the drought-sensitive genotype (average across both years of study). Likewise, AgNPs reduced MDA and RCI by 28% and 38%, respectively, greater in drought-tolerant genotypes under moderate drought stress over the drought-sensitive genotype averaged across both years of study.
Principal component analysis (PCA)
PCA was used to estimate the relative effects of top leaf senescence, MDA, RCI and water potential (Ψw) on 1000-grain weight, grain numbers, Pn and Gs of drought-stressed wheat at tillering. Post-drought treatment PCA loading matrix shows a strong negative correlation of top leaf senescence, MDA, RCI and water potential (Ψw) with 1000-grain weight, NGPS, Pn and Gs. Leaf stress indicators such as MDA, RCI, senescence and water potential all had a strong and positive correlation, whereas 1000-grain weight, NGPS, Pn and Gs showed a strong and positive correlation. Top leaf senescence and water potential fell in the negative quadrant of PCA, indicating that these traits contributed most to the reduction of yield and gas exchange components, while MDA and RCI also had a negative correlation with grain yield and gas exchange components (Fig. 4a). The first component (PC 1) covered the greatest amount of variation (96.77%), followed by the second component (PC 2), which covered 1.95% of the total variation. The A, B, C and D dots represent 100% FC (ideal water conditions), the L, M, N and O dots represent 75% FC (mild drought stress), the W, X, Y and Z dots represent 50% FC (moderate drought stress), and the 1, 2, 3 and 4 dots represent 25% FC (severe drought stress) (Fig. 4b, c). The A, B, C and D dots, as well as the L, M, N and O dots, are scattered closely together and away from the vertical line in the positive quadrant of PCA, indicating the close and positive performance of yield and gas exchange components with a weak correlation with MDA, RCI, top leaf senescence, and Ψw at 100% and 75% field capacity. The dots 1, 2, 3 and 4 dots are scattered away from the vertical line and also are away from each other, indicating that the grain yield and gas exchange components perform poorly with MDA, RCI, top leaf senescence and Ψw under severe drought stress and have a strong negative association with these parameters. Similarly, the W, X, Y and Z dots indicate that lower grain yield and less value of gas exchange component under moderate drought stress (Fig. 4b, c). The maximum variation of PC 1 shows that MDA, RCI, top leaf senescence and Ψw have a strong and negative correlation with 1000-grain weight, NGPS, Pn and Gs (Fig. 5).
Principle component analysis. (a) Monoplot, (b) biplot correlation, (c) degree of relation, and (d) principle components contribution of malondialdehyde (MDA), relative cell injury (RCI), flag leaf senescence (FLS), leaf water potential (Ψw) with the net photosynthetic rate (Pn), stomatal conductance (Gs), 1000-grain weight (1000 GW), number of grains per spike (NGPS) and glycine betaine (GB) under water regimes of wheat (averaged across both years of study). A, B, C and D, 100 FC moisture regime; L, M, N and O, 75% FC moisture regime; W, X, Y and Z, 50% FC moisture regime; 1, 2, 3 and 4, 25% FC moisture regime.
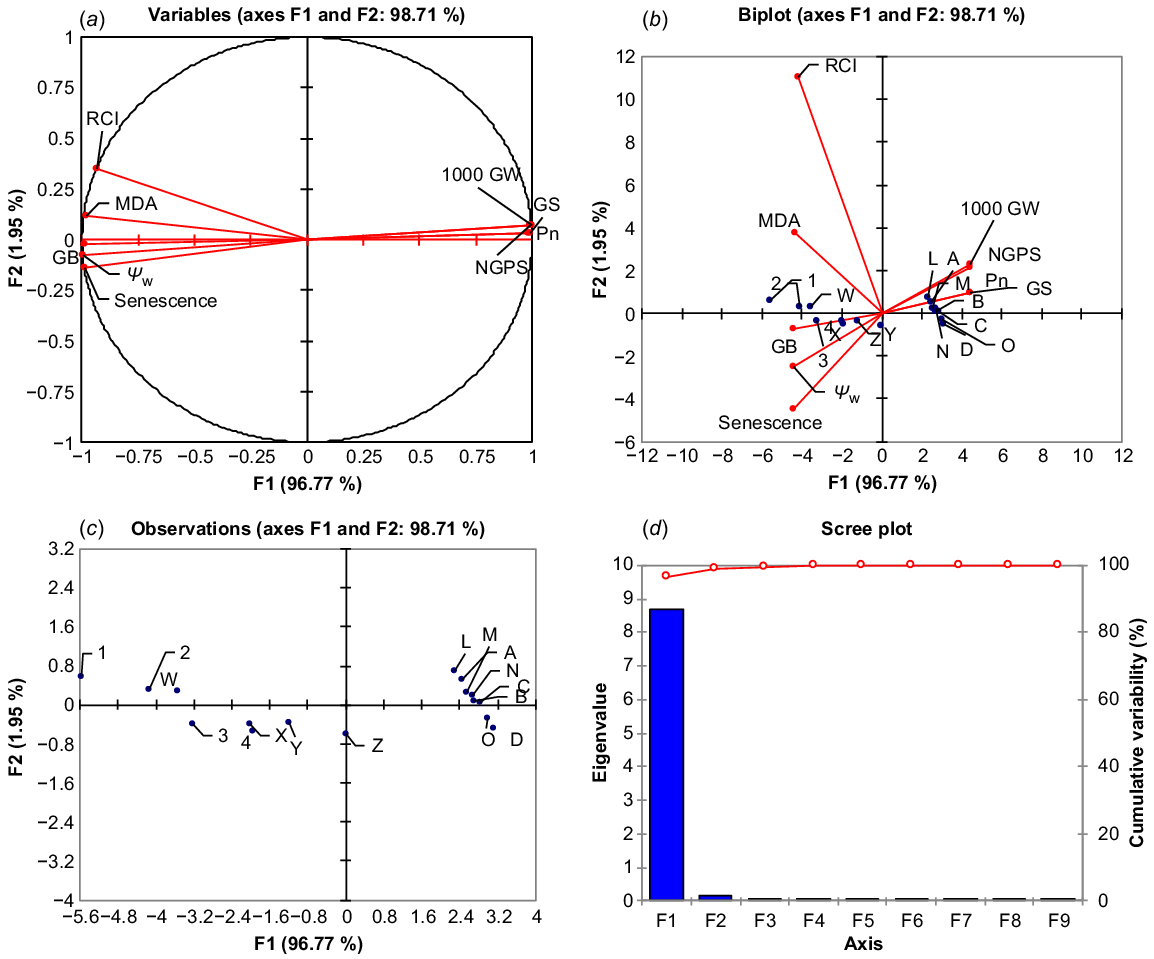
Heat map correlation matrix of malondialdehyde (MDA), relative cell injury (RCI), flag leaf senescence (FLS), leaf water potential (Ψw), glycine betaine (GB), net photosynthetic rate (Pn), stomatal conductance (Gs), 1000-grain weight (1000 GW), number of grains per spike (NGPS) and under water and application of AgNPs.
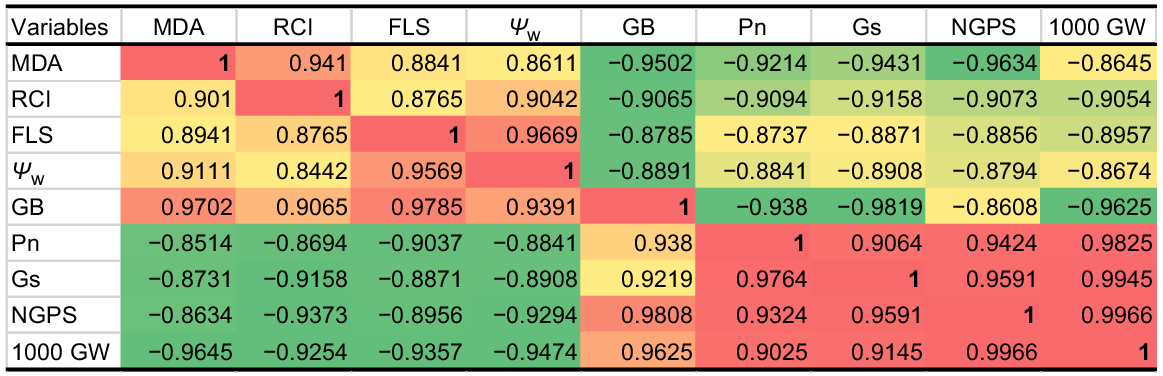
Discussion
Drought stress during tillering reduces growth and yield of wheat crops
In this study, moderate and severe drought significantly reduced tillers per plant and thus grain yield formation, whereas mild drought stress did not affect wheat crops. Water scarcity during tillering may have limited the initiation of new tillers via hormonal regulation, assimilate synthesis/translocation and water supplies (Abid et al. 2018; Yang et al. 2022). Drought during tillering is among the leading causes of yield loss in wheat crops (Nakhforoosh et al. 2015). For each degree increase in drought intensity; i.e. from 75% FC to 50% FC, the number of tillers per plant, grain number and grain weight per spike were reduced by 3.0%, 2.6% and 1.5%, respectively. Tillering contributes 87% of grain yield in wheat (Tilley et al. 2019), and moderate drought during this stage could reduce up to 21.5% of fertile tillers (Zulfiqar et al. 2022) and 47% of final grain yield (Ramezanpoor and Dastfal 2004). Drought at tillering might disturb the hormones regulating tillering pathway, roots functioning and leaf physiological process (Bacher et al. 2022; Yang et al. 2022). Abid et al. (2016) and Yang et al. (2021) investigated the effects of drought stress on wheat tillering and found that moderate drought stress results in a reduction of the number of tillers per plant, while severe drought stress causes a significant reduction in both tiller number and size. The unaffected wheat tillers under mild drought imply that wheat plants tend to conserve moisture rather than using maximum field capacity. Drought-induced injury to photosynthetic machinery during tillering as observed in this study may slow down the overall efficiency of the assimilation process. Further, the negative effects of drought could be stored in plant memory, limiting leaf physiology performance and disrupting water relations during reproductive stages (Ali et al. 2022; Aurangzaib et al. 2022; Vijayaraghavareddy et al. 2022). Poor grain yield of drought-stressed plants could result from a drop in leaf water content, increase in solute accumulation, damage to photosystem health/gas exchange components, increase in top leaf senescence and oxidative burst (Kalal et al. 2022; Yang et al. 2022). Moderate drought stress (50% field capacity) significantly reduces the grain yield and grain weight per spike in wheat. Similarly, severe drought stress (25% field capacity) had an even more pronounced effect, with a significant reduction in grain yield and grain weight per spike (Hussain et al. 2016; Mickky et al. 2019).
The genotypes used in this study performed differently under various drought levels. For example, the sensitive genotype produced 1.0%, 2.0% and 0.8% fewer tillers, grains and grain weight per spike, respectively, compared to the tolerant genotype for each degree rises in mild drought to moderate drought. This capacity of drought-tolerant genotype to sustain tillering could be associated with increased accumulation of osmolytes/compatible solutes, improved leaf physiology, membrane integrity and water relations as suggested by (Ahmad et al. 2022b; Jamshidi Goharrizi et al. 2022; Shah et al. 2022). The findings suggest that screening wheat genotypes for drought tolerance using the same parameters will aid in the development of better genotypes for future climates. The yield stability of wheat genotypes has been linked with superior leaf physiological functioning and sustaining membrane integrity under heat (Ullah et al. 2022).
AgNPs modulate leaf water status to reduce drought injury
We found that AgNPs increased tiller formation, grain numbers and grain weight per spike by 1.4%, 0.52% and 0.80%, respectively, than water spray, for each degree rises in drought from mild to severe drought stress, supporting our hypothesis that AgNPs can increase yield attributes of drought-stressed wheat. Chanu and Upadhyaya (2019) documented that nanoparticles can restrain the leaf physiology and water relations of drought-stressed field crops. In our study, AgNPs induced 0.30%, 0.8% and 0.60% more tillers, gains numbers and grain weight per spike, respectively, in the tolerant than sensitive genotype, for each degree rise in drought from mild to moderate drought stress. The response of the tolerant genotype to nanoparticles could be associated with its capacity to restrict water loss and sustain leaf physiological functioning under drought, accelerating the growth recovery process (El-Bassiouny et al. 2022). Due to improvements in gas exchange components, membrane stability and water relations, AgNPs increase grain yield in wheat and other cereal crops under drought stress (Jaskulska and Jaskulski 2020; Ahmed et al. 2021). Photosynthesis is the primary source of dry biomass production and grain yield in plants, and as a result of chlorophyll loss and photosynthetic apparatus disassembly under various stresses, the leaf assimilation area decreases and senescence increases (Zhang et al. 2022).
Top leaf senescence increased while leaf physiological components were decreased from moderate to severe drought stress in both genotypes of the current study, with relatively more damage to the sensitive genotype under severe drought. Previous findings suggest that reduced leaf turgidity due to hydraulic failure decreased photosynthetic energy conversion capacity and decreased photosynthetic efficiency due to excessive electron production due to accelerated leaf senescence during wheat crop tillering (Abid et al. 2016, 2018). Akhkha et al. (2011) and El-Afry et al. (2012) examined that moderate drought stress (50% field capacity) limits the photosynthesis rate, stomatal conductance and maximum quantum yield of PSII (Fv/Fm), while severe drought stress (25% field capacity) further decrease these parameters. These studies also found that both moderate and severe drought stresses led to a significant reduction in chlorophyll contents in wheat leaves. In the current study, AgNPs protected plants from drought injury with a greater impact on the tolerant genotype under moderate drought. AgNPs may help to stabilise chloroplast and cell membranes, improving photosynthetic efficiency and gas exchange components (Razzaq et al. 2016; Jhanzab et al. 2019; Kannaujia et al. 2022). AgNPs increase photosynthetic pigments, stomatal opening, photosynthetic rate and yield of photosynthetic machinery under drought stress by improving nutrient uptake and mobility in plants (Ahmed et al. 2021). Leaf water potential (LWP) is a useful index of plant soil water stress that provides insights into plant–water relations (Wankmüller and Carminati 2022). When water availability decreases, plants develop mechanisms to cope with water stress by decreasing LWP (Nishida et al. 2009). In the current study, moderate to severe drought reduced water potential in top leaves while increasing the osmotic potential. The results indicate that drought stress could reduce leaf turgidity in wheat, reducing water movement from the xylem to leaf tissues (Verbeke et al. 2023). Higher leaf solute potential in wheat under drought holds the leaf water, making it unavailable for photosynthesis (Rashid et al. 2022). Moderate drought stress reduces leaf water potential in wheat while severe drought stress further limits the water potential (Arzanesh et al. 2011; Alghory and Yazar 2019). Under moderate to severe drought, exogenously applied AgNPs increased water potential while decreasing osmotic potential by creating a favourable gradient for water movement from the xylem to the leaves. The positive results of AgNPs could be used to improve drought tolerance in the plants cultivated in water-stressed regions.
Drought disrupts the balance between oxidative stress and the plant defensive system, causes lipid bilayer hydrolysis and reduces membrane water and solute potential (Hou et al. 2016; Zada et al. 2020). In this study, medium to severe drought stress weakened the plant defensive system with a higher oxidative burst, indicating higher membrane leakage with lower water potentials. The results appear to indicate that drought stress at wheat tillering causes hydrolysis of membrane lipids, membrane fusion and transient pores in membranes (Sheoran et al. 2015). Drought at wheat tillering causes more damage to membranes than other vegetative and reproductive stages because plant assimilates shift from primary tillers to secondary tillers (Marcińska et al. 2013). Under moderate to severe drought stress in wheat, the overflow of electrons and lipid peroxidation in membranes have caused water and solute leakage from membranes (Sheoran et al. 2015; Rashid et al. 2022). Drought-stressed wheat produces more reactive oxygen species such as MDA, which disrupts the balance of water relations and antioxidant enzymes (Hameed et al. 2011). Under drought, AgNPs can stimulate the plant’s defensive system and protect cellular membranes from oxidative injury (Alabdallah et al. 2021). Our findings suggest that under drought, exogenous application of AgNPs stimulates the production of osmoprotectants and enzymatic antioxidants maintaining the water potential by increasing membrane stability. This study suggests that using AgNPs during drought stress during wheat tillering could mitigate the negative effects of water scarcity.
Conclusion
Moderate to severe drought stress severely affected the leaf physiology, plant defensive system and grain yield of both wheat genotypes, with significantly more damage to drought susceptible genotype. Further, severe drought caused more damage to leaf physiology and grain yield. The foliar application of AgNPs in this study induced drought tolerance and strengthened the plant physiology and defensive system of both genotypes. The drought-tolerant genotype had the most pronounced effect of AgNPs on plant physiology and grain yield. Our findings suggest that foliar application of AgNPs before the onset of drought stress can protect wheat crops from drought stress during tillering. However, additional field studies are required to confirm the efficacy of AgNPs under different drought levels.
Acknowledgements
The authors thank the Analytical Lab, Department of Agronomy University of Agriculture Faisalabad Pakistan and the Medicinal Plant Biochemistry Lab, Department of Biochemistry University of Agriculture Faisalabad Pakistan for providing technical support.
References
Abid M, Tian Z, Ata-Ul-Karim ST, Cui Y, Liu Y, Zahoor R, Jiang D, Dai T (2016) Nitrogen nutrition improves the potential of wheat (Triticum aestivum L.) to alleviate the effects of drought stress during vegetative growth periods. Frontiers in Plant Science 7, 981.
| Crossref | Google Scholar |
Abid M, Ali S, Qi LK, Zahoor R, Tian Z, Jiang D, Snider JL, Dai T (2018) Physiological and biochemical changes during drought and recovery periods at tillering and jointing stages in wheat (Triticum aestivum L.). Scientific Reports 8, 4615.
| Crossref | Google Scholar |
Ahmad W, Ullah N, Xu L, El Sabagh A (2022a) Editorial: global food and nutrition security under changing climates. Frontiers in Agronomy 3, 799878.
| Crossref | Google Scholar |
Ahmad A, Aslam Z, Javed T, Hussain S, Raza A, Shabbir R, Mora-Poblete F, Saeed T, Zulfiqar F, Ali MM, Nawaz M, Rafiq M, Osman HS, Albaqami M, Ahmed MAA, Tauseef M (2022b) Screening of wheat (Triticum aestivum L.) genotypes for drought tolerance through agronomic and physiological response. Agronomy 12, 287-303.
| Crossref | Google Scholar |
Ahmed F, Javed B, Razzaq A, Mashwani Z-u-R (2021) Applications of copper and silver nanoparticles on wheat plants to induce drought tolerance and increase yield. IET Nanobiotechnology 15, 68-78.
| Crossref | Google Scholar |
Akhkha A, Boutraa T, Alhejely A (2011) The rates of photosynthesis, chlorophyll content, dark respiration, proline and abscicic acid (ABA) in wheat (Triticum durum) under water deficit conditions. International Journal of Agriculture and Biology 13, 215-221.
| Google Scholar |
Alabdallah NM, Hasan MM, Salih AM, Roushdy SS, Al-Shammari AS, Alsanie SI, El-Zaidy M (2021) Silver nanoparticles improve growth and protect against oxidative damage in eggplant seedlings under drought stress. Plant, Soil and Environment 67, 617-624.
| Crossref | Google Scholar |
Alghory A, Yazar A (2019) Evaluation of crop water stress index and leaf water potential for deficit irrigation management of sprinkler-irrigated wheat. Irrigation Science 37, 61-77.
| Crossref | Google Scholar |
Ali N, Anjum MM, Khan GR, Ali R (2022) Unraveling wheat grain quality, physiological indices, dry matter accumulation, and attenuating water stress adverse effect via foliar potassium application at different growth stages. Gesunde Pflanzen 74, 41-52.
| Crossref | Google Scholar |
Arnon DI (1949) Copper enzymes in isolated chloroplasts. Polyphenoloxidase in Beta vulgaris. Plant Physiology 24, 1-15.
| Crossref | Google Scholar |
Arzanesh MH, Alikhani HA, Khavazi K, Rahimian HA, Miransari M (2011) Wheat (Triticum aestivum L.) growth enhancement by Azospirillum sp. under drought stress. World Journal of Microbiology and Biotechnology 27, 197-205.
| Crossref | Google Scholar |
Aslam H, Ahmad MSA, Alvi AK, Rani W, Athar H-u-R, Al-Ashkar I, Almutairi KF, Ullah N, Ayman E-S (2022) He–Ne laser priming enhances drought tolerance in wheat through differential modification of photosynthetic pigments and antioxidative enzymes. Agronomy 12, 2376-2393.
| Crossref | Google Scholar |
Aurangzaib M, Ahmad Z, Jalil MI, Nawaz F, Shaheen MR, Ahmad M, Hussain A, Ejaz MK, Tabassum MA (2022) Foliar spray of silicon confers drought tolerance in wheat (Triticum aestivum L.) by enhancing morpho-physiological and antioxidant potential. Silicon 14, 4793-4807.
| Crossref | Google Scholar |
Bacher H, Sharaby Y, Walia H, Peleg Z (2022) Modifying root-to-shoot ratio improves root water influxes in wheat under drought stress. Journal of Experimental Botany 73, 1643-1654.
| Crossref | Google Scholar |
Bates LS, Waldren RP, Teare ID (1973) Rapid determination of free proline for water-stress studies. Plant and Soil 39, 205-207.
| Crossref | Google Scholar |
Bayoumi TY, Eid MH, Metwali EM (2008) Application of physiological and biochemical indices as a screening technique for drought tolerance in wheat genotypes. African Journal of Biotechnology 7, 2341-2352.
| Google Scholar |
Bukhari MA, Ahmad Z, Ashraf MY, Afzal M, Nawaz F, Nafees M, Jatoi WN, Malghani NA, Shah AN, Manan A (2021) Silicon mitigates drought stress in wheat (Triticum aestivum L.) through improving photosynthetic pigments, biochemical and yield characters. Silicon 13, 4757-4772.
| Crossref | Google Scholar |
Cakmak I, Horst WJ (1991) Effect of aluminium on lipid peroxidation, superoxide dismutase, catalase, and peroxidase activities in root tips of soybean (Glycine max). Physiologia Plantarum 83, 463-468.
| Crossref | Google Scholar |
Chanu TT, Upadhyaya H (2019) Zinc oxide nanoparticle-induced responses on plants: a physiological perspective. In ‘Nanomaterials in plants, algae and microorganisms. Vol. 2’. (Eds DK Tripathi, P Ahmad, DK Chauhan, NK Dubey) pp. 43–64. (Academic Press: London, UK) doi:10.1016/B978-0-12-811488-9.00003-2
Daryanto S, Wang L, Jacinthe P-A (2016) Global synthesis of drought effects on maize and wheat production. PLoS ONE 11, e0156362.
| Crossref | Google Scholar |
Dhakal A (2021) Effect of drought stress and management in wheat – a review. Food & Agribusiness Management 2, 62-66.
| Crossref | Google Scholar |
El Sabagh A, Hossain A, Barutcular C, Islam MS, Awan SI, Galal A, Iqbal MA, Sytar O, Yildirim M, Meena RS, Fahad S, Najeeb U, Konuskan O, Habib RA, Llanes A, Hussain S, Farooq M, Hasanuzzaman M, Abdelaal KH, Hafez Y, Cig F, Saneoka H (2019) Wheat (Triticum aestivum L.) production under drought and heat stress – adverse effects, mechanisms and mitigation: a review. Applied Ecology and Environmental Research 17, 8307-8332.
| Crossref | Google Scholar |
El-Afry MM, El-Nady MF, Abdelmonteleb EB, Metwaly MMS (2012) Anatomical studies on drought-stressed wheat plants (Triticum aestivum L.) treated with some bacterial strains. Acta Biologica Szegediensis 56, 165-174.
| Google Scholar |
El-Bassiouny HMS, Mahfouze HA, Abdallah MMS, Bakry BA, El-Enany MAM (2022) Physiological and molecular response of wheat cultivars to titanium dioxide or zinc oxide nanoparticles under water stress conditions. International Journal of Agronomy 2022, 1-15.
| Google Scholar |
Figueroa-Bustos V, Palta JA, Chen Y, Siddique KHM (2019) Early season drought largely reduces grain yield in wheat cultivars with smaller root systems. Plants 8, 305-319.
| Crossref | Google Scholar |
Fleury D, Jefferies S, Kuchel H, Langridge P (2010) Genetic and genomic tools to improve drought tolerance in wheat. Journal of Experimental Botany 61, 3211-3222.
| Crossref | Google Scholar |
Giannopolitis CN, Ries SK (1977) Superoxide dismutases: I. Occurrence in higher plants. Plant Physiology 59, 309-314.
| Crossref | Google Scholar |
Grieve CM, Grattan SR (1983) Rapid assay for determination of water soluble quaternary ammonium compounds. Plant and Soil 70, 303-307.
| Crossref | Google Scholar |
Hameed A, Bibi N, Akhter J, Iqbal N (2011) Differential changes in antioxidants, proteases, and lipid peroxidation in flag leaves of wheat genotypes under different levels of water deficit conditions. Plant Physiology and Biochemistry 49, 178-185.
| Crossref | Google Scholar |
Hickey K, Wood M, Sexton T, Sahin Y, Nazarov T, Fisher J, Sanguinet KA, Cousins A, Kirchhoff H, Smertenko A (2022) Drought tolerance strategies and autophagy in resilient wheat genotypes. Cells 11, 1765-1790.
| Crossref | Google Scholar |
Hojjat SS, Ganjali A (2016) The effect of silver nanoparticle on lentil seed germination under drought stress. International Journal of Farming and Allied Sciences 5, 208-212.
| Google Scholar |
Hou Q, Ufer G, Bartels D (2016) Lipid signalling in plant responses to abiotic stress. Plant, Cell & Environment 39, 1029-1048.
| Crossref | Google Scholar |
Hussain M, Waqas-ul-Haq M, Farooq S, Jabran K, Farroq M (2016) The impact of seed priming and row spacing on the productivity of different cultivars of irrigated wheat under early season drought. Experimental Agriculture 52, 477-490.
| Crossref | Google Scholar |
Ihsan MZ, El-Nakhlawy FS, Ismail SM, Fahad S, daur I (2016) Wheat phenological development and growth studies as affected by drought and late season high temperature stress under arid environment. Frontiers in Plant Science 7, 795.
| Crossref | Google Scholar |
Iqbal M, Raja NI, Mashwani Z-U-R, Wattoo FH, Hussain M, Ejaz M, Saira H (2019) Assessment of AgNPs exposure on physiological and biochemical changes and antioxidative defence system in wheat (Triticum aestivum L) under heat stress. IET Nanobiotechnology 13, 230-236.
| Crossref | Google Scholar |
Jamshidi Goharrizi K, Baghizadeh A, Karami S, Nazari M, Afroushteh M (2022) Expression of the W36, P5CS, P5CR, MAPK3, and MAPK6 genes and proline content in bread wheat genotypes under drought stress. Cereal Research Communications 9, 1-12.
| Crossref | Google Scholar |
Jaskulska I, Jaskulski D (2020) Effects of using nanoparticles of silver (AgNPs) and copper (CuNPs) in foliar fertilizers. Przemysl Chemiczny 99, 250-253.
| Google Scholar |
Jhanzab HM, Razzaq A, Bibi Y, Yasmeen F, Yamaguchi H, Hitachi K, Tsuchida K, Komatsu S (2019) Proteomic analysis of the effect of inorganic and organic chemicals on silver nanoparticles in wheat. International Journal of Molecular Sciences 20, 825.
| Crossref | Google Scholar |
Kalal PR, Tomar RS, Jajoo A (2022) SiO2 nanopriming protects PS I and PSII complexes in wheat under drought stress. Plant Nano Biology 2, 100019.
| Crossref | Google Scholar |
Kannaujia R, Singh P, Prasad V, Pandey V (2022) Evaluating impacts of biogenic silver nanoparticles and ethylenediurea on wheat (Triticum aestivum L.) against ozone-induced damages. Environmental Research 203, 111857.
| Crossref | Google Scholar |
Lesk C, Rowhani P, Ramankutty N (2016) Influence of extreme weather disasters on global crop production. Nature 529, 84-87.
| Crossref | Google Scholar |
Liu D, Zou J, Meng Q, Zou J, Jiang W (2009) Uptake and accumulation and oxidative stress in garlic (Allium sativum L.) under lead phytotoxicity. Ecotoxicology 18, 134-143.
| Crossref | Google Scholar |
Marcińska I, Czyczyło-Mysza I, Skrzypek E, Filek M, Grzesiak S, Grzesiak MT, Janowiak F, Hura T, Dziurka M, Dziurka K, Nowakowska A, Quarrie SA (2013) Impact of osmotic stress on physiological and biochemical characteristics in drought-susceptible and drought-resistant wheat genotypes. Acta Physiologiae Plantarum 35, 451-461.
| Crossref | Google Scholar |
Maswada HF, Sunoj VSJ, Prasad PVV (2021) A comparative study on the effect of seed pre-sowing treatments with microwave radiation and salicylic acid in alleviating the drought-induced damage in wheat. Journal of Plant Growth Regulation 40, 48-66.
| Crossref | Google Scholar |
Mickky B, Aldesuquy H, Elnajar M (2019) Drought-induced change in yield capacity of ten wheat cultivars in relation to their vegetative characteristics at heading stage. Physiology and Molecular Biology of Plants 25, 1137-1148.
| Crossref | Google Scholar |
Munsif F, Farooq U, Arif M, Shah T, Jehangir M, Zaheer S, Akhtar K, Khan MS, Ahmad I, Ahmad W, Ali S, Amir R (2022) Potassium and salicylic acid function synergistically to promote the drought resilience through upregulation of antioxidant profile for enhancing potassium use efficiency and wheat yield. Annals of Applied Biology 180, 273-282.
| Crossref | Google Scholar |
Nakhforoosh A, Grausgruber H, Kaul H-P, Bodner G (2015) Dissection of drought response of modern and underutilized wheat varieties according to Passioura’s yield-water framework. Frontiers in Plant Science 6, 570.
| Crossref | Google Scholar |
Naqvi SMZA, Haq SIU, Iderawumi ARM, Anwar A, Mujtaba G, Khan NM, Islam A, Awais M, Mustafa T, Iqbal J (2022) Green synthesis of silver nanoparticles and anti-oxidant activity in plants under semiarid condition–a review. Pakistan Journal of Weed Science Research 28, 321-332.
| Crossref | Google Scholar |
Naumann G, Cammalleri C, Mentaschi L, Feyen L (2021) Increased economic drought impacts in Europe with anthropogenic warming. Nature Climate Change 11, 485-491.
| Crossref | Google Scholar |
Nawaz F, Ahmad R, Waraich EA, Naeem MS, Shabbir RN (2012) Nutrient uptake, physiological responses, and yield attributes of wheat (Triticum aestivum L.) exposed to early and late drought stress. Journal of Plant Nutrition 35, 961-974.
| Crossref | Google Scholar |
Nishida K, Khan NM, Shiozawa S (2009) Effects of salt accumulation on the leaf water potential and transpiration rate of pot-grown wheat with a controlled saline groundwater table. Soil Science and Plant Nutrition 55, 375-384.
| Crossref | Google Scholar |
Prasad P, Staggenborg S, Ristic Z (2008) Impacts of drought and/or heat stress on physiological, developmental, growth, and yield processes of crop plants. In ‘Response of crops to limited water: understanding and modeling water stress effects on plant growth processes. Vol. 1’. (Eds L Ahuja, VR Reddy, SA Saseendran, Q Yu) pp. 301–355. (American Society of Agronomy, Crop Science Society of America, Soil Science Society of America)
Rashid U, Yasmin H, Hassan MN, Naz R, Nosheen A, Sajjad M, Ilyas N, Keyani R, Jabeen Z, Mumtaz S, Alyemeni MN, Ahmad P (2022) Drought-tolerant Bacillus megaterium isolated from semi-arid conditions induces systemic tolerance of wheat under drought conditions. Plant Cell Reports 41, 549-569.
| Crossref | Google Scholar |
Razzaq A, Ammara R, Jhanzab HM, Mahmood T, Hafeez A, Hussain S (2016) A novel nanomaterial to enhance growth and yield of wheat. Journal of Nanoscience and Technology 2, 55-58.
| Google Scholar |
Saeidi M, Ardalani S, Jalali-Honarmand S, Ghobadi M-E, Abdoli M (2015) Evaluation of drought stress at vegetative growth stage on the grain yield formation and some physiological traits as well as fluorescence parameters of different bread wheat cultivars. Acta Biologica Szegediensis 59, 35-44.
| Google Scholar |
Scholander PF, Bradstreet ED, Hemmingsen EA, Hammel HT (1965) Sap pressure in vascular plants: negative hydrostatic pressure can be measured in plants. Science 148, 339-346.
| Crossref | Google Scholar |
Shah SMDM, Shabbir G, Malik SI, Raja NI, Shah ZH, Rauf M, Zahrani YA, Alghabari F, Alsamadany H, Shahzad K, Yang SH (2022) Delineation of physiological, agronomic and genetic responses of different wheat genotypes under drought condition. Agronomy 12, 1056.
| Crossref | Google Scholar |
Sheoran S, Thakur V, Narwal S, Turan R, Mamrutha HM, Singh V, Tiwari V, Sharma I (2015) Differential activity and expression profile of antioxidant enzymes and physiological changes in wheat (Triticum aestivum L.) under drought. Applied Biochemistry and Biotechnology 177, 1282-1298.
| Crossref | Google Scholar |
Silveira JAG, Araújo SAM, Lima JPMS, Viégas RA (2009) Roots and leaves display contrasting osmotic adjustment mechanisms in response to NaCl-salinity in Atriplex nummularia. Environmental and Experimental Botany 66, 1-8.
| Crossref | Google Scholar |
Soliman MH, Alnusairi GSH, Khan AA, Alnusaire TS, Fakhr MA, Abdulmajeed AM, Aldesuquy HS, Yahya M, Najeeb U (2022) Biochar and selenium nanoparticles induce water transporter genes for sustaining carbon assimilation and grain production in salt-stressed wheat. Journal of Plant Growth Regulation 42, 1-22.
| Crossref | Google Scholar |
Taran N, Storozhenko V, Svietlova N, Batsmanova L, Shvartau V, Kovalenko M (2017) Effect of zinc and copper nanoparticles on drought resistance of wheat seedlings. Nanoscale Research Letters 12, 60.
| Crossref | Google Scholar |
Tilley MS, Heiniger RW, Crozier CR (2019) Tiller initiation and its effects on yield and yield components in winter wheat. Agronomy Journal 111, 1323-1332.
| Crossref | Google Scholar |
Ullah A, Al-Busaidi WM, Al-Sadi AM, Farooq M (2022) Bread wheat genotypes accumulating free proline and phenolics can better tolerate drought stress through sustained rate of photosynthesis. Journal of Soil Science and Plant Nutrition 22, 165-176.
| Crossref | Google Scholar |
Verbeke S, Padilla-Díaz CM, Martínez-Arias C, Goossens W, Haesaert G, Steppe K (2023) Mechanistic modeling reveals the importance of turgor-driven apoplastic water transport in wheat stem parenchyma during carbohydrate mobilization. New Phytologist 237, 423-440.
| Crossref | Google Scholar |
Vijayaraghavareddy P, Lekshmy SV, Struik PC, Makarla U, Yin X, Sreeman S (2022) Production and scavenging of reactive oxygen species confer to differential sensitivity of rice and wheat to drought stress. Crop and Environment 1, 15-23.
| Crossref | Google Scholar |
Wankmüller FJP, Carminati A (2022) Stomatal regulation prevents plants from critical water potentials during drought: result of a model linking soil–plant hydraulics to abscisic acid dynamics. Ecohydrology 15, e2386.
| Crossref | Google Scholar |
Yang H, Hu W, Zhao J, Huang X, Zheng T, Fan G (2021) Genetic improvement combined with seed ethephon priming improved grain yield and drought resistance of wheat exposed to soil water deficit at tillering stage. Plant Growth Regulation 95, 399-419.
| Crossref | Google Scholar |
Yang H, Xiao Y, He P, Ai D, Zou Q, Hu J, Liu Q, Huang X, Zheng T, Fan G (2022) Straw mulch-based no-tillage improves tillering capability of dryland wheat by reducing asymmetric competition between main stem and tillers. The Crop Journal 10, 864-878.
| Crossref | Google Scholar |
Yasmeen F, Razzaq A, Iqbal MN, Jhanzab HM (2015) Effect of silver, copper and iron nanoparticles on wheat germination. International Journal of Biosciences (IJB) 6, 112-117.
| Google Scholar |
Zada A, Ali A, Shah A, Gill S, Hussain I, Ullah Z, Sher H (2020) Physiological and molecular characterization of bread wheat (Triticum aestivum L.) for drought resistance [Preprint]. Authorea 1-12.
| Crossref | Google Scholar |
Zhang J, Zhang S, Cheng M, Jiang H, Zhang X, Peng C, Lu X, Zhang M, Jin J (2018) Effect of drought on agronomic traits of rice and wheat: a meta-analysis. International Journal of Environmental Research and Public Health 15, 839-852.
| Crossref | Google Scholar |
Zhang Q, Tang W, Peng S, Li Y (2022) Limiting factors for panicle photosynthesis at the anthesis and grain filling stages in rice (Oryza sativa L.). The Plant Journal 109, 77-91.
| Crossref | Google Scholar |
Zulfiqar B, Raza MAS, Saleem MF, Aslam MU, Iqbal R, Muhammad F, Amin J, Ibrahim MA, Khan IH (2022) Biochar enhances wheat crop productivity by mitigating the effects of drought: insights into physiological and antioxidant defense mechanisms. PLoS ONE 17, e0267819.
| Crossref | Google Scholar |