Molecular Chemistry for Solar Fuels: From Natural to Artificial Photosynthesis*
Ann Magnuson A B and Stenbjörn Styring AA Department of Chemistry – Ångström Laboratory, Uppsala University, Box 523, SE-751 20 Uppsala, Sweden.
B Corresponding author. Email: ann.magnuson@kemi.uu.se
![]() Ann Magnuson has a PhD in biochemistry from Lund University and is Associate Professor at Uppsala University. Her research includes manganese- and ruthenium-manganese complexes aimed at artificial photosynthesis, as well as the photosynthetic enzymes of nitrogen fixing cyanobacteria. |
![]() Stenbjörn Styring took his PhD in biochemistry at Göteborg University, and is chair Professor in Molecular Biomimetics at Uppsala University. His research concerns the water oxidizing enzyme Photosystem II, as well as development of solar fuels using synthetic manganese-, cobalt- and ruthenium-manganese complexes for artificial photosynthetic oxidation of water. |
Australian Journal of Chemistry 65(6) 564-572 https://doi.org/10.1071/CH12114
Submitted: 24 February 2012 Accepted: 10 April 2012 Published: 21 June 2012
Abstract
The world needs new, environmentally friendly, and renewable fuels to exchange for fossil fuels. The fuel must be made from cheap, abundant, and renewable resources. The research area of solar fuels aims to meet this demand. This paper discusses why we need a solar fuel, and proposes solar energy as the major renewable energy source to feed from. The scientific field concerning artificial photosynthesis is expanding rapidly and most of the different scientific visions for solar fuels are briefly reviewed. Research strategies for the development of artificial photosynthesis to produce solar fuels are overviewed, with some critical concepts discussed in closer detail.
Introduction
The global energy supply is dominated by fossil fuels, accounting for approximately 80 % of the total energy used (Fig. 1, panel A).[1,2] It is beyond all doubt that the reserves of both oil and natural gas will run out within a foreseeable future, making a society based on fossil energy unviable in a longer time perspective. Today’s dominating energy carriers are also connected to environmental and social factors that affect the quality of life. In particular this concerns fossil fuels, from which anthropogenic CO2 emissions have been identified as a main cause behind global warming.[3] It is thus increasingly apparent that future energy carriers should be neutral with respect to CO2 emissions. In addition, the availability of fossil fuels across the planet is unequal, leading to political tension and problems with energy. Nuclear energy is an important energy source that does not lead to production-related CO2 release. However, many people are opposing large nuclear power programs. The resistance comes from fear of major accidents and spreading nuclear technology for military purposes.
![]() |
There is no lack of renewable energy on earth, not even with respect to the large and growing global energy consumption. The most abundant renewable resource is solar energy, which is available in vast quantities. The installed capacity for solar energy is small today,[1,2] and heavy investments in research and development are needed to increase the impact of solar energy. After 30–40 years of research photovoltaics have now reached a mature stage. A diverse range of solar cell technologies exist at all levels, from early research stages to industrial scale.
However, apart from renewable electricity generation, the development of CO2-free non-polluting renewable fuels is of vital importance. Solar fuels as future energy carriers and a way to store solar energy, is a theme that quickly moves up on the global research agenda (Fig. 2).
![]() |
Solar Fuels – Artificial Photosynthesis
The term ‘solar fuel’ is fairly new and is becoming established since the beginning of the new millennium. The recent increase in the field is evident from the rapid growth in research networks and in the number of publications per year. A survey of the larger networks in Europe and the US revealed a burst in the efforts made after 2006 (Fig. 2).† In the US this was a result from decisions made by the Obama administration, and the installation of new large research centres led to a major change on the global arena. In Europe the increase in the number of networks has evolved slower over time and has been overtaken by the US in the last years. The survey was made in spring 2009 and Europe is likely to catch up with the US numbers.
The introduction of solar fuels on a large scale is motivated by concerns about global warming, energy security, equity, and decreased availability of oil and gas. The development is also driven by recent advances in a range of scientific fields that have scientists convinced that solar fuels can be produced in an efficient and cheap way in a not too distant future. Solar energy is a vastly abundant but intermittent energy form, and it is vital that it can be stored for an indefinite time. On one hand, household energy consumption is often high in the morning and early evening, while on the other hand the solar influx is highest at midday. Thus, storage of solar energy is critical to balance the societal energy equation. In the arctic region there is enough solar energy to contribute substantially to the energy system on an annual basis. However, solar energy is essentially lacking in the winter when energy demand is highest. Whether short-term or long-term, energy storage is a key to making solar energy available to a larger population and for wider use. The central idea of a solar fuel is to harvest the energy when the sun shines, store it as a fuel, and then use the fuel whenever the energy is needed.‡
Although storage is a major energy concern, another important aspect is that of ownership. Development of solar fuels may be the break-through in energy availability which could enable the non-legacy world to catch up with the living standards of the legacy world. With limited distribution of fossil fuel reserves, a majority of people are presently unable to increase their standard of living in a fossil fuel based society. The exploitation of the full potential of solar energy as a natural resource requests not only increasing the use of solar electricity, but also demands that solar fuels are produced for storage and transport.
Renewable Materials, Renewable Energy
From a technological viewpoint, raw materials are critical for the development of solar fuels. The raw material should be inexhaustible, cheap, and widely available, which is why many scientists target water as the raw material. Like sunlight, water is an essentially endless and fairly evenly distributed resource. The aim is thus to make a fuel based on solar energy and water, and processes where solar energy provides the driving force for oxidising water can become important on a global scale during the shift away from fossil fuels.
The target fuel is another important technical issue. Many scientists target hydrogen (H2) as the solar fuel. When water is used as starting material, hydrogen is a logical product and there are several recent examples of photochemical hydrogen production.[5–8] However, the transition to a hydrogen based economy is not straightforward and there are technological hurdles to overcome before hydrogen is used worldwide. An alternative is to use CO2 from the atmosphere as a second raw material (in addition to water) and make a carbon based solar fuel. The application of carbon based fuels from renewable sources is technologically uncomplicated on a shorter term, since carbon based fuels are used already today. However, the atmospheric CO2 is very dilute, which raises questions about how the fuel production process can be made efficient. In addition, the chemistry involved in photo-driven reduction of CO2 is much more difficult to master than for hydrogen production that already has been demonstrated in several systems. Therefore this attractive option has scientific impediments that make the arrival of carbon based solar fuels unlikely in the near future.
Research on solar fuels constitutes a novel field that aims to answer the demands and challenges of the future energy system. The development of technologies for direct solar to fuel conversion requires scientific breakthroughs in several areas of fundamental science.
Global Energy Consumption
In 2009 the world energy consumption rate was approximately 16 TW.[1] Predicting future energy use is not an easy task. A widespread statement is that the global energy need will at least double to the year 2050 (Fig. 1, panel D) and may triple by 2100. The most pertinent reasons behind this projected increase relate to the present and increasing world population, and to potential energy savings. Increased health and prosperity is obviously connected to increased income per capita (GDP/capita, Gross Domestic Product). Analyses have also shown a clear correlation between increased energy consumption and a prosperous economy. The difference in energy consumption between rich and poorer nations can be up to three orders of magnitude.[1,2,9] It is important for the analysis that very large populations in Asia, Africa, and South America are low energy consumers today. A large part of the foreseen increase in energy consumption will be by people that use little energy today, around three billion people, which is nearly half of the population of the world in 2012. A second, equally large increase in the world energy consumption will come from future generations. The world population passed seven billion in October 2011 and is projected to increase to 9–10 billion in 2050. These extra three billion new world citizens will also demand equally large quantities of energy. Thus, there will be in total at least six billion more energy consumers whose needs must be met by 2050.
The direct energy consumption from primary sources may be divided into energy used as electricity and energy used as a fuel. This is shown in Fig. 1c. On a global scale, 17 % of energy supplies are used as electricity. The rest, 83 %, is used in some other way, mainly in the form of fuels.[1] The use of electricity is also reflected in the degree of electrification. Far from all countries in the world are fully electrified – a continuous supply of electricity is a luxury commodity. In the legacy world, electricity is mainly generated in large power plants and distributed in massively developed grid systems. Building up similar grids in the non-legacy countries is clearly not the only way forward, particularly not since the technological development is moving away from large scale power plants furnished by nuclear or fossil fuels. It is likely that the investments for the near six billion new energy users need development of a less centralised, highly distributed energy system.
Global electricity usage is growing. The International Energy Agency has estimated that in 2030 the fraction of energy used as electricity will have increased to 22 % of the total energy production.[1] At the same time the total energy consumption also increases, including the use of fuels. Therefore it is doubtful that electricity will be the world’s main energy carrier in a foreseeable future. In a sustainable and CO2 neutral energy economy the fuel needs must be covered by renewable energy sources.
Renewable Energy, Solar Energy
Solar energy is by far the largest source of renewable energy. In one hour, the average irradiance of solar energy incident on the planet surface adds up to the yearly human energy consumption (Fig. 1a, b). Solar energy is available everywhere but it is most abundant in places where human population is dense. Not all of this vast energy influx can be used for energy conversion, largely due to geographical constraints. On the other hand, the average solar irradiance over the entire planet is approximately 350 W m–2 (but can be more than 2000 W m–2 in the tropical regions). If we envision a solar energy system with a modest 10 % energy conversion efficiency, it need only cover an area corresponding to 0.1 % of earth’s total area to provide the 16 TW used by the world in 2009. Clearly, there is much more solar energy available globally than the need projected for 2050 or 2100.
Solar based technologies provide only a small fraction of the total energy production today. In 2009 the combined solar electricity and solar thermal production was 21 TWh globally, around 0.1 ‰ of the total world energy supply.[1,2] To some extent this low degree of utilisation reflects shortcomings of the available technologies, e.g. difficulties with energy storage and insufficient energy supply in the dark or with overcast skies. But the main challenge for solar energy is the low price of fossil fuels. With increasing demand and decreasing supplies of oil and natural gas, the price tag on fossil fuels is likely to change. In addition, increasing use of penalty systems for CO2 emissions are likely to contribute to the rising prices of fossil fuels. It is hard to predict when the costs of fossil fuels will meet the costs of solar based systems, but commercialised solar energy systems will be in high demand when it does. It is necessary that we develop today the solar technologies of tomorrow.
Options for Solar Energy
Methods to collect and use solar energy can be divided in four main categories (Fig. 3). Combustion of already converted solar energy in the form of biomass, fossil fuels, waste etc. is well known technology (Fig. 3, upper left). With more development we can learn to burn and use existing fuels better and cleaner. However, there are no improvements of existing methods that can take our society out of our fossil fuel dependence.
![]() |
High temperature solar power can be used for electricity and fuel production, and advanced heating concepts such as concentrated solar power (CSP) or thermal cycles for solar fuels using heliostats are promising emerging technologies (Fig. 3, bottom left). These techniques are mainly restricted to very sunny areas and will be less important in coastal climates with hazy or overcast skies, and at northern latitudes. Low temperature solar power is useful for many industrial processes as well as heating and cooling of residential buildings.
Electricity from solar panels (Fig. 3, lower right) clearly has the potential to solve the need for electricity also in less sunny areas. Solar cell technology has reached considerable maturity and the installed photovoltaic capacity is increasing rapidly. New generations of solar cells are very promising with respect to both cost and efficiency.[10]
The fourth alternative is to make a solar fuel directly (Fig. 3, upper right). This is the newest of these branches and the most research demanding. There are no available systems outside a few laboratories (however, see ref. 7) and basic research is needed in every corner of this area.
Development of a global energy system based on solar energy needs to use an interdisciplinary approach to achieve systems that are sustainable on a longer term. Future energy systems need to utilise raw materials that are renewable and abundant not only to preserve natural resources momentarily, but to secure the supply for a continuous and growing energy production. Fossil fuels have been so easy to find and inexpensive to extract, that there was very little driving force for the development of other energy systems before the 1970s. In addition, much of the basic science necessary to develop solar fuels has been lacking. Over the last few decades however, research efforts have resulted in a broad pallet of strategies to develop solar fuels that follow different technological pathways.
Indirect vs Direct Methods to Produce a Solar Fuel
It is important to consider that the emerging technologies for solar fuel production have similar elements but also critical differences. One useful distinction is between direct and indirect processes for solar fuel production, another is between molecular and non-molecular systems. Figs 4 and 5 depict a range of research strategies that are currently in focus, divided by these principles.
![]() |
![]() |
Indirect processes for solar fuels are distinguished by the lack of a direct connection between the energy source and the fuel output. In the indirect processes, the solar energy is first converted and stored in the form of an intermediate energy carrier. The energy carrier can be valuable in itself but is not directly useful as a fuel. Two economically important indirect processes for solar fuel production are shown in the bottom panel of Fig. 4: the first is solar fuel from photovoltaics, today hydrogen is mainly produced by electrolysis of water. The general drawback with this method is the detour via the intermediate energy carrier electricity, which results in energy losses in the different conversion steps such as losses in wiring. In addition the intermittency in power feed lowers the efficiency of many electrolysers.
The second important process is fuel from biomass, where the product, biofuel, is secondary to the organism’s metabolism. Biofuel production essentially means to grow a tree, plant, or photosynthetic microorganism, which is rich in wood, oil or something else that can serve as a fuel. The entire organism is then harvested. For example, trees are cut down to produce wood, wood chips, and charcoal; sugarcane and corn are harvested before the carbohydrates are fermented to produce alcohol. In all systems based on harvesting biomass, the limitation is the detour around the life of the organism. Sustaining life is, from a pure energy efficiency stand point, a very inefficient process. In addition, production of fuels with high energy density in addition demands refining the primary biomass, which leads to more losses.
Thus all processes to solar fuel involving biomass are indirect and inefficient with respect to solar energy conversion into the fuel.
Direct Processes for Solar Fuel Production
A common theme for direct processes of solar fuel production, is that they have the potential to become more efficient than indirect processes. Another advantage is that solar fuels produced in a direct process are likely to be more cost effective, since fewer parts and less materials are involved in manufacturing, which may lower the overall cost. There are several strategies to develop direct processes for solar fuels production that can be divided into three categories (Fig. 5). To develop all of these techniques, generating many alternative solutions, and then drive the best candidates to implementation, is a formidable challenge (for recent comprehensive reviews in many of these areas we refer to refs [11–13]). The input in man-hours will be large, and broad scientific know-how is needed. Comparisons between different existing systems should be made with a generous attitude since neither technology has been proven feasible outside any laboratory yet.
Artificial Photosynthesis
In one category of the direct processes we find what is referred to as artificial photosynthesis. The main principle is to design light-driven catalysts that can oxidise water directly without intermediate electricity generation, by mimicking the structural and/or functional principles of natural photosynthesis.[14–16] A solar fuel should be produced by using the reducing power liberated by water oxidation. Artificial photosynthesis naturally also involves attempts to develop catalysts that can reduce a suitable substrate, which will be the basis for the fuel. Systems of this kind have the highest potential with respect to solar energy conversion of all envisioned systems. The target fuel can be hydrogen, which is the objective in most attempts at solar fuel production since the protons released from the water oxidation can be used as a substrate for reduction. But the fuel could also be carbon based, which might be easier to use but is probably more difficult to make.
Molecular and Non-Molecular Processes
A light driven catalyst can be either molecular or non-molecular (Fig. 5). The physical limitations of both are comparable and the scientific problems encountered are of equal magnitude. The main research problem is light-driven water oxidation and the development of catalysts that can assist this reaction. The initial capture of solar energy, and the formation of hydrogen, are both better known processes and easier to achieve.
A system for artificial photosynthesis which is entirely molecular in nature is difficult to achieve but has several advantages that has led to a growing interest in the molecular approach.[11,15–17] The essential characteristic is that molecules have a well defined and well known structure as opposed to, for instance, many semi-conductor materials. The advantage is that a well defined molecular structure lends itself to deliberate synthetic modifications to improve or fine-tune its properties. Molecules are also amenable to spectroscopic studies including FTIR, optical, EPR spectroscopy etc., that give both structural and kinetic information. The catalytic process can thereby be followed and understood to a very detailed level.
Non-molecular systems are based on light-driven processes where catalysis occurs on metal surfaces, semiconductors, or nano-structured materials. Non-molecular catalysts used for water splitting are often metal oxides.[7,12,18–21] A clear disadvantage is that many are made from scarce and expensive metals. In addition it is more difficult to study the catalytic mechanisms when the catalyst is heterogeneous in nature. An important advantage however, is that while most molecular systems studied to date are unstable under illumination, many non-molecular systems are quite robust and tolerant to deleterious side reactions. However, this situation might change when more functional molecular systems have been studied and become better understood.
An interesting development involves the mixing of molecular and non-molecular systems. In this case the capturing of solar energy can be done by a semiconductor while the catalysis may be carried out by molecular catalysts, or vice versa. The catalysts may contain abundant materials like Co, Fe, Mn, or Ni.[7,10,21–23] It is not unlikely that these mixed systems will be the winning concept since they combine advantages from both types of systems.
Photobiological Solar Fuel Production
Photobiological solar fuel production is a different approach, which is perhaps better defined as semi-direct (Fig. 4, top). Photosynthetic microorganisms harvest solar energy and may use it to produce fuel in a continuous process.[13,24–26] The fuel is expelled by the organism, allowing the fuel to be extracted continuously from a growth container commonly known as a photo-bioreactor. The fuel production does not demand the harvest of the organism, and the growing process can in principle be stopped so that it uses very little of the supplied solar energy. The efficiency can therefore be higher than in traditional biofuel production where the organism has to be grown until its biomass is large enough to be harvested. On the other hand, a significant fraction of the absorbed solar energy is used to maintain life, so the theoretical efficiency is lower than for artificial photosynthesis.
There are different types of fuels that can be anticipated from photosynthetic microorganisms. Some cyanobacteria and green algae are the only organisms that combine photosynthetic water oxidation with the capacity to produce hydrogen (Fig. 4, top). Thus, hydrogen is a natural product of both cyanobacteria and green algae and a commonly targeted product in this area of research. The science involves foreseeable efforts in molecular biology, biochemistry, and metabolic engineering, and might already now be close to critical breakthroughs. Demonstration of sustainable photobiological hydrogen production has been made in both cyanobacteria and algae.[27,28]
For microbial solar fuel production, other fuels have also been introduced such as oils, alcohols, and other hydrocarbons (Fig. 4, top). The organisms used are cyanobacterial or algal strains where the genome is known in detail, so that the tools for molecular biology and genetics are well developed. A metabolic pathway leading to biosynthesis of a carbon based fuel can be inserted into the organism, a technique referred to as synthetic biology.[29–31] Synthetic biology is also equally useful for the development of efficient hydrogen production. This branch of solar fuels research is under rapid development.
These methods for solar fuel formation by photosynthetic microorganisms are clearly not indirect, since the fuel is collected without destroying the living cells. However, it is clearly not an entirely direct process either since the metabolic pathways leading to the solar fuel involve energy rich intermediates. The energy which is trapped by photosynthesis is temporarily stored in energy carriers, such as simple sugars or polysaccharides, which are later broken down to produce the fuel. Probably the best terminology is to describe this as a semi-direct process.
An interesting recent development attempts to divert the energy and reducing power from photosynthesis from unwanted pathways that lead to storage, towards the desired pathways that lead to fuel production. There are few examples of this research as yet, but promising results have been presented in cyanobacteria where enzymes in the nitrate reduction metabolism have been removed, resulting in increased hydrogen formation. The hydrogen production increase suggests that reductants from the nitrate assimilation pathway were redirected towards proton reduction.[32]
Principle Reactions in Artificial Photosynthesis
There are some basic principles that must be incorporated to achieve the desired solar fuel. These are outlined in Fig. 6. The first concerns the substrates. If solar fuels should become important on a global scale, the raw material(s) used to produce the fuel must be abundant and globally available, cheap, and easy to transport. Water fulfils all these requirements, and is the prime target for the first substrate in Fig. 6. Water is used as the sacrificial electron donor in natural photosynthesis, where light-driven oxidation of water in Photosystem II provides the biosphere with an endless source of energy.[33,34]
![]() |
The idea for solar fuels production is the same: to use the energy in sunlight to oxidise water and create enough reducing power to form the fuel. The target reaction is shown on the left side in Fig. 6 and is written as:
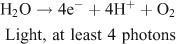
Two options exist to handle the reducing equivalents that are liberated in the reaction: they can be stored in the form of a reduced intermediate, or they can be used directly in reduction of the second substrate, to form the fuel. An interesting option is to use the protons that are released in water oxidation, to produce hydrogen: (Fig. 6, right)

This reaction is chemically feasible and the product is molecular hydrogen, an energy-rich and useful fuel. Present day fuel technologies are not optimised for large-scale hydrogen usage however. It would be advantageous to use atmospheric CO2 as a raw material for a solar fuel. The reducing equivalents that originate from water oxidation could then be used to reduce CO2 into a fuel like methanol or methane. However, it is presently more difficult to drive this multi-electron chemistry with light than to accomplish the reduction of protons to form hydrogen.
Fig. 6 shows the three principal steps that need to be accomplished to make hydrogen from solar energy and water. The first is the absorption of a photon. This is accomplished by a photosensitizer, S (Fig. 6), that is excited by light. The excited state is reducing enough to trigger charge separation, which is the step where the photon energy is converted to chemically useful energy. A highly energetic radical pair is formed transiently, where the electron is separated from the electron hole on the photosensitizer. The radical pair is unstable, and has a high risk of deactivating quickly by electron recombination. To prevent this, the photosensitizer should be coupled to two catalytic systems that can either be molecular and tethered to the photosensitizer via covalently bound linkers, or the catalysts could be integrated non-molecular catalytic materials, such as a metal containing particle on a metal oxide surface. In either case, the electron in the radical pair should be transported to an electron acceptor (A, Fig. 6) while the corresponding electron hole is transferred to an electron donor system (D, Fig. 6). Moving the charges away from the photosensitizer S will stabilise the charge separated state, at the cost of some of the captured energy.
The next set of reactions that need to be achieved are the catalysis of water oxidation and proton reduction. Both demand efficient catalysts (D and A in Fig. 6). A necessary feature for successful solar fuels production from water, is that the oxidised donor system, D+, should be oxidising enough to drive the electron extraction from water. At the same time, the reduced electron acceptor system, A–, must be reducing enough (Fig. 6).
The most difficult reaction to accomplish presently is controlled oxidation of water at ambient temperature and (relatively) neutral pH. Light-induced charge separation on a single-electron level has been demonstrated in numerous synthetic systems.[14,35,36] These include manganese complexes linked to Ru-based photosensitizers aimed to mimic the electron transfer processes of Photosystem II.[37,38] However, the oxidation of two water molecules demands that several light-induced charge separations are coupled to accumulation of redox equivalents on the catalyst. Since photons come one by one the catalyst D must be able to store four electron holes. In nature this accumulative electron transfer is accomplished by the oxygen evolving centre in Photosystem II, which contains a metal cluster with 4 Mn ions and one Ca ion.[33,34] The four manganese ions have high valence that increase during the catalytic cycle, thereby storing four electron holes. The cycle is completed by the formation of the O–O bond and the return of the manganese ions to their original valence states.[39] The exact details of this reaction are not known, making it exceedingly difficult to copy. Several attempts have been made by using man-made manganese complexes.[40–43] The perhaps most promising new development involves using oxides of cobalt instead of manganese.[21,44,45]
The second critical reaction is carried out by the electron acceptor, A. This should be able to store two electrons from two charge separations, and also be able to reduce protons to form hydrogen (Fig. 6). This chemistry is easier to achieve than water oxidation and there are many molecular and non-molecular systems that are able to carry out this chemistry. In biological systems hydrogen production as well as hydrogen oxidation is carried out by hydrogenases. These are enzymes with often bi-metallic active sites composed of Ni-Fe or Fe-Fe systems.[46–48] A molecular complex modelled on the natural hydrogenase active site, can in principle be driven by light to function as a proton reducing catalyst, and the biomimetic chemistry in this field has proven quite successful.[6,17,49]
A problem for both natural and artificial photosynthesis is the kinetic competition from unproductive reactions. Because the excited photosensitizer is often both very oxidising and reducing, it may reduce an already oxidised donor (D+) and oxidise the reduced acceptor (A–) in reverse reactions (Fig. 6). In addition, D+ and A– are often radicals or metal centres with low-lying excited states that can lead to rapid energy transfer or quenching of the sensitizer. To avoid these reactions, the electron transfer steps that lead to fuel production must be more rapid to compete with electron recombination kinetically. In Photosystem II the donor Mn4Ca complex and electron acceptor QuinoneB are located at some distance from the chlorophyll excited states, and the subsequent electron and hole transfer steps stabilise the separated charges against wasteful recombination long enough to permit the catalytic chemistry to occur. A similar separation between the three components of an artificial system needs to be built in by design, to make the process viable.
Conclusion
Much effort is put into development of catalysts that can be driven by the energy in visible light. There are already catalysts that can work with UV light, but this is using a limited part of the solar spectrum. To accomplish the necessary reactions with the relatively moderate energy available in visible light is difficult. This is presently a large obstacle in the development of new systems. Second, many of today’s functioning catalysts are made from expensive and rare elements. Catalysts made from the noble metals Ru and Ir work quite well for water oxidation,[50,51] and hydrogen formation is accomplished by Pt and Pd.[5] Several of these catalysts could in principle be used for solar fuel production, but due to the scarcity of these elements they are not sustainable or suitable for development on larger scale. Abundant elements for active electron acceptor catalysts are Co, Ni, and Fe[17,22,52] that constitute the active parts in several well functioning systems. It is more difficult to accomplish light driven water oxidation, and only a few systems have been shown to work. Oxides of Fe, Mn, and Co are very promising, but much research is still waiting to be done.[40–44]
An interesting problem that will be important for energy conversion efficiency, is whether the entire reaction from water oxidation to hydrogen formation can be achieved by one photon-act only, or if a two-photon reaction is necessary (Fig. 6a, b). With one photon the reaction looks deceptively simple; the high potential state achieved by the charge separation might react directly with the proton to form an energy rich product, while the electron hole is transferred to the water oxidising catalyst, D. However, this reaction will be very difficult to manage. The process is more feasible using two photons, which means that two different light reactions must be developed. In Fig. 6b a two-photon system is drawn as two modules, connected by a dashed line to indicate different solutions. In one module, or half-cell, a strongly oxidising photosensitizer (So in Fig. 6b) is coupled to the water oxidising catalyst, D. In the reducing half-cell, another photon is absorbed by a photosensitizer with a highly reducing excited state (Sr in Fig. 6b) and used to drive hydrogen production by the catalytic acceptor A. The electron hole on Sr should be filled by electrons from the oxidising module.
The problem lies in combining the two modules in a functional device (Fig. 7). The two half cells must work in a concerted manner. Incomplete catalysis will lower the yield considerably, and reactive intermediates that are left uncontrolled will pose a large risk of oxidative damage to the system. On the positive side is also that the use of two photon-acts presents many options for design and material utilisation. For example, the two photons may have different wavelengths. This would allow the utilisation of a wider range of the solar spectrum, and more of the solar energy can be used.
![]() |
In nature there are photosynthetic organisms that use both the single-photon and the two-photon principles. Organisms using the two-photon systems have mastered the catalytic chemistry of water oxidation, and the utilisation of sunlight as their energy source has made them the dominating masters of the biosphere. There is much for us to learn from natural photosynthesis in the development of artificial solar fuel production, for our own sake and for the sake of generations to come.
Acknowledgements
Financial support for the author’s research in the Swedish Consortium for Artificial Photosynthesis is provided by the Knut and Alice Wallenberg Foundation, the Swedish Energy Agency, the Swedish Research Council, and SOLAR-H2 (FP7 EU contract 212508).
References
[1] World Energy Outlook, OECD/IEA, 2010.[2] BP Statistical Review of World Energy June 2011.
[3] IPCC 2007: Climate Change 2007: Synthesis Report. Contribution of Working Groups I, II and III to the Fourth Assessment Report of the Intergovernmental Panel on Climate Change [Core Writing Team, Pachauri, R.K and Reisinger, A. (eds.)]. IPCC, Geneva, Switzerland.
[4] A. Kraytsberg, Y. Ein-Eli, J. Power Sources 2011, 196, 886.
| Crossref | GoogleScholarGoogle Scholar | 1:CAS:528:DC%2BC3cXhtlGnurbK&md5=0eab0066a06bbc873762a5e06ed4a25bCAS |
[5] K. Sakai, H. Ozawa, Coord. Chem. Rev. 2007, 251, 2753.
| Crossref | GoogleScholarGoogle Scholar | 1:CAS:528:DC%2BD2sXhtFKhs7zP&md5=436f7d68437e8d4a5220447da195d3a6CAS |
[6] D. Streich, Y. Astuti, M. Orlandi, L. Schwartz, R. Lomoth, L. Hammarström, S. Ott, Chemistry 2010, 16, 60.
| Crossref | GoogleScholarGoogle Scholar | 1:CAS:528:DC%2BD1MXhs1WksLfE&md5=a09f7bb27baace7f6f71bc824a940a03CAS |
[7] S. Y. Reece, J. A. Hamel, K. Sung, T. D. Jarvi, A. J. Esswein, J. J. Pijpers, D. G. Nocera, Science 2011, 334, 645.
| Crossref | GoogleScholarGoogle Scholar | 1:CAS:528:DC%2BC3MXhtlyqu7vF&md5=e0298161b92e218b9b45c56538dc6eb5CAS |
[8] K. Maeda, K. Domen, J. Phys. Chem. C 2007, 111, 7851.
| Crossref | GoogleScholarGoogle Scholar | 1:CAS:528:DC%2BD2sXlt1Whs78%3D&md5=ae798c23361fda4ba34dab57719f5196CAS |
[9] The World Bank Energy and mining dataset. (URL: http://data.worldbank.org/topic/energy-and-mining).
[10] A. Yella, H.-W. Lee, H.-N. Tsao, C. Yi, A. Kumar, M. K. Nazeeruddin, E. W. Diau, C.-Y. Yeh, S. M. Zakeeruddin, M. Grätzel, Science 2011, 334, 629.
| Crossref | GoogleScholarGoogle Scholar | 1:CAS:528:DC%2BC3MXhtlyqu7nI&md5=e133a1a57aae1b4de8c382af9246514dCAS |
[11] Artificial photosynthesis and solar fuels. Guest editors L. Hammarström, S. Hammes-Schiffer. Special issue of Acc. Chem. Res. 2009, 42, 1859.
[12] Renewable Energy. Guest editors D. G. Nocera, D. Guldi. Chem. Soc. Rev. 2009, 38, 1.
[13] Solar Energy Conversion. Guest editor Villy Sundström. Themed issue of Dalton Trans. 2009, 45, 9937.
[14] M. R. Wasielewski, Chem. Rev. 1992, 92, 435.
| Crossref | GoogleScholarGoogle Scholar | 1:CAS:528:DyaK38XitFWju70%3D&md5=9337a6bd5066293299e7ee33fe8ae289CAS |
[15] A. Magnuson, M. F. Anderlund, O. Johansson, P. Lindblad, R. Lomoth, T. Polivka, S. Ott, K. Stensjö, S. Styring, V. Sundström, L. Hammarström, Acc. Chem. Res. 2009, 42, 1899.
| Crossref | GoogleScholarGoogle Scholar | 1:CAS:528:DC%2BD1MXhtFCjt73P&md5=fa7bfd74288e73c5494e279d8e97f50dCAS |
[16] D. Gust, T. A. Moore, A. L. Moore, Acc. Chem. Res. 2009, 42, 1890.
| Crossref | GoogleScholarGoogle Scholar | 1:CAS:528:DC%2BD1MXhtlyrtrnN&md5=31f8f2b6799f2b3c146caed304bb4212CAS |
[17] S. Ott, S. Styring, L. Hammarström, O. Johansson, Towards Solar Fuels using a biomimetic Approach. Progress in the Swedish Consortium for Artificial Photosynthesis. In Energy production and storage 2010 (Ed. R. Crabtree), Chichester, UK: John Wiley & Sons, Ltd, pp. 199–227.
[18] T. R. Cook, D. K. Dogutan, S. Y. Reece, Y. Surendranath, T. S. Teets, D. G. Nocera, Chem. Rev. 2010, 110, 6474.
| Crossref | GoogleScholarGoogle Scholar | 1:CAS:528:DC%2BC3cXhtl2lsb3I&md5=438de29bdee38432d249bcc4efea7a26CAS |
[19] M. G. Walter, E. L. Warren, J. R. McKone, S. W. Boettcher, Q. Mi, E. A. Santori, N. S. Lewis, Chem. Rev. 2010, 110, 6446.
| Crossref | GoogleScholarGoogle Scholar | 1:CAS:528:DC%2BC3cXhtl2lsbvF&md5=51671dca2cc6d1811c23ea04b8c22579CAS |
[20] X. Chen, S. Shen, L. Guo, S. S. Mao, Chem. Rev. 2010, 110, 6503.
| Crossref | GoogleScholarGoogle Scholar | 1:CAS:528:DC%2BC3cXhtl2lsbvE&md5=2b4147810dfb82f62fbd028c1e75ad5aCAS |
[21] M. W. Kanan, D. G. Nocera, Science 2008, 321, 1072.
| Crossref | GoogleScholarGoogle Scholar | 1:CAS:528:DC%2BD1cXhtVSitrbP&md5=4d554995a8442318e37fd3fd4f64344fCAS |
[22] M. R. Dubois, D. L. Dubois, Chem. Soc. Rev. 2009, 38, 62.
| Crossref | GoogleScholarGoogle Scholar |
[23] M. Risch, V. Khare, I. Zaharieva, L. Gerencser, P. Chernev, H. Dau, J. Am. Chem. Soc. 2009, 131, 6936.
| Crossref | GoogleScholarGoogle Scholar | 1:CAS:528:DC%2BD1MXlsFCnsr8%3D&md5=ea2d468c983f5932657a7baf16a96aefCAS |
[24] Energy Biotechnology. Guest editors P. Lindblad, T. Jeffries. Opin. Biotech. 2009, 20, 318.
[25] O. Kruse, B. Hankamer, Curr. Opin. Biotechnol. 2010, 21, 238.
| Crossref | GoogleScholarGoogle Scholar | 1:CAS:528:DC%2BC3cXmslOhtr0%3D&md5=3baada305c88c3538bfb51c9fad52dbdCAS |
[26] A. Hemschemeier, T. Happe, BBA–Bioenergetics 2011, 1807, 919.
| 1:CAS:528:DC%2BC3MXntVylsbs%3D&md5=f5e984fccb0942987f3501d981c148bdCAS |
[27] A. Melis, L. P. Zhang, M. Forestier, M. L. Girardi, M. Seibert, Plant Physiol. 2000, 122, 127.
| Crossref | GoogleScholarGoogle Scholar | 1:CAS:528:DC%2BD3cXmvFamsw%3D%3D&md5=082fe288bfd2e13248aef04c7efa9708CAS |
[28] A. A. Tsygankov, A. S. Fedorov, S. N. Kosourov, K. K. Rao, Biotechnol. Bioeng. 2002, 80, 777.
| Crossref | GoogleScholarGoogle Scholar | 1:CAS:528:DC%2BD38XptlaltLY%3D&md5=f3066d3054b4adb40054631bbcdf5694CAS |
[29] D. F. Savage, J. Way, P. A. Silver, ACS Chem. Biol. 2008, 3, 13.
| Crossref | GoogleScholarGoogle Scholar | 1:CAS:528:DC%2BD1cXntlKktw%3D%3D&md5=c0e2bdc3c87ecee5315e48a5b4f19ffcCAS |
[30] D. C. Ducat, G. Sachdeva, P. A. Silver, Proc. Natl. Acad. Sci. USA 2011, 108, 3941.
| Crossref | GoogleScholarGoogle Scholar | 1:CAS:528:DC%2BC3MXjs1WqtrY%3D&md5=a226ffa6d30d93861949f3452073d00fCAS |
[31] A. S. Khalil, J. J. Collins, Nat. Rev. Genet. 2010, 11, 367.
| Crossref | GoogleScholarGoogle Scholar | 1:CAS:528:DC%2BC3cXkvVSqs78%3D&md5=f84e970c41f5ca36b52392dca3e56412CAS |
[32] W. Baebprasert, S. Jantaro, W. Khetkorn, P. Lindblad, A. Incharoensakdi, Metab. Eng. 2011, 13, 610.
| Crossref | GoogleScholarGoogle Scholar | 1:CAS:528:DC%2BC3MXhtV2itbzN&md5=6be13140ad371a34961ecec40ffed807CAS |
[33] N. Nelson, C. F. Yocum, Annu. Rev. Plant Biol. 2006, 57, 521.
| Crossref | GoogleScholarGoogle Scholar | 1:CAS:528:DC%2BD28XosVKht7w%3D&md5=5f914926595315fa795e5b2165307b4eCAS |
[34] Y. Umena, K. Kawakami, J. R. Shen, N. Kamiya, Nature 2011, 473, 55.
| Crossref | GoogleScholarGoogle Scholar | 1:CAS:528:DC%2BC3MXkslCmtLg%3D&md5=f7b48594fae4a870a2b3d1c7ed10df43CAS |
[35] D. Gust, T. A. Moore, A. L. Moore, Acc. Chem. Res. 2001, 34, 40.
| Crossref | GoogleScholarGoogle Scholar | 1:CAS:528:DC%2BD3cXnvVWmtrw%3D&md5=3d584d87269cb9174ee404f201709a99CAS |
[36] V. Balzani, A. Credi, M. Venturi, ChemSusChem 2008, 1, 26.
| Crossref | GoogleScholarGoogle Scholar | 1:CAS:528:DC%2BD1cXktFCqur0%3D&md5=2ed3d57962788a29debc1b2ee9348bfeCAS |
[37] L. C. Sun, L. Hammarström, B. Åkermark, S. Styring, Chem. Soc. Rev. 2001, 30, 36.
| Crossref | GoogleScholarGoogle Scholar | 1:CAS:528:DC%2BD3MXkt1Ojug%3D%3D&md5=ece2d0306c3852ac95fa42d837e69581CAS |
[38] M. Borgström, N. Shaikh, O. Johansson, M. F. Anderlund, S. Styring, B. Åkermark, A. Magnuson, L. Hammarström, J. Am. Chem. Soc. 2005, 127, 17504.
| Crossref | GoogleScholarGoogle Scholar |
[39] M. Haumann, P. Liebisch, C. Muller, M. Barra, M. Grabolle, H. Dau, Science 2005, 310, 1019.
| Crossref | GoogleScholarGoogle Scholar | 1:CAS:528:DC%2BD2MXhtF2is7nI&md5=f4bbd9425797009c30e0b94189f8e788CAS |
[40] J. Limburg, J. S. Vrettos, H. Y. Chen, J. C. de Paula, R. H. Crabtree, G. W. Brudvig, J. Am. Chem. Soc. 2001, 123, 423.
| Crossref | GoogleScholarGoogle Scholar | 1:CAS:528:DC%2BD3cXptVyjs7Y%3D&md5=0d642ccbab22d02ffc48b8bb26f5b005CAS |
[41] K. Beckmann, H. Uchtenhagen, G. Berggren, M. F. Anderlund, A. Thapper, J. Messinger, S. Styring, P. Kurz, Energ. Environ. Sci. 2008, 1, 668.
| Crossref | GoogleScholarGoogle Scholar | 1:CAS:528:DC%2BD1MXntFWkt7w%3D&md5=799ebbf1e2de5ff525d34d6975a19a9aCAS |
[42] S. Mukherjee, J. A. Stull, J. Yano, T. C. Stamatatos, K. Pringouri, T. A. Stich, K. A. Abboud, R. D. Britt, V. K. Yachandra, G. Christou, Proc. Natl. Acad. Sci. USA 2012, 109, 2257.
| Crossref | GoogleScholarGoogle Scholar | 1:CAS:528:DC%2BC38XivFSjsr8%3D&md5=4cfa76569ebed1eafcb3ee4c3ea0772cCAS |
[43] M. Wiechen, H.-M. Berends, P. Kurz, Dalton Trans. 2011, 41, 21.
| Crossref | GoogleScholarGoogle Scholar |
[44] Q. Yin, J-M. Tan, C. Besson, Y. V. Geletti, D. G Musaev, A. E. Kuznetsov Luo, K. I. Hardcastle, C. L. Hill, Science 2010, 328, 342.
| Crossref | GoogleScholarGoogle Scholar | 1:CAS:528:DC%2BC3cXks1Sgtbs%3D&md5=31262becc276064b39f67eb7a54d16e6CAS |
[45] D. Shevchenko, M. F. Anderlund, A. Thapper, S. Styring, Energ. Environ. Sci. 2011, 4, 1284.
| Crossref | GoogleScholarGoogle Scholar | 1:CAS:528:DC%2BC3MXms1WitL0%3D&md5=20c888727643d05ce81bdd0b4e17a0c7CAS |
[46] J. C. Fontecilla-Camps, A. Volbeda, C. Cavazza, Y. Nicolet, Chem. Rev. 2007, 107, 4273.
| Crossref | GoogleScholarGoogle Scholar | 1:CAS:528:DC%2BD2sXhtVahs77P&md5=303d63d738e6e35028da0c86d4962c56CAS |
[47] K. A. Vincent, A. Parkin, F. A. Armstrong, Chem. Rev. 2007, 107, 4366.
| Crossref | GoogleScholarGoogle Scholar | 1:CAS:528:DC%2BD2sXhtVWis7bL&md5=da64ae221be8103275698accb9afd3cfCAS |
[48] A. L. Goff, V. Artero, B. Jousselme, P. D. Tran, N. Guillet, R. Métayé, A. Fihri, S. Palacin, M. Fontecave, Science 2009, 326, 1384.
| Crossref | GoogleScholarGoogle Scholar |
[49] B. E. Barton, M. T. Olsen, T. B. Rauchfuss, Curr. Opin. Biotechnol. 2010, 21, 292.
| Crossref | GoogleScholarGoogle Scholar | 1:CAS:528:DC%2BC3cXmslOht78%3D&md5=ccb3c257441125a798ac8a68bc436211CAS |
[50] L. L. Duan, Y. H. Xu, P. Zhang, M. Wang, L. C. Sun, Inorg. Chem. 2010, 49, 209.
| Crossref | GoogleScholarGoogle Scholar | 1:CAS:528:DC%2BD1MXhsFaks7fJ&md5=cd878c696865f4d3a6bcdfb2758d5a51CAS |
[51] J. J. Concepcion, J. W. Jurss, M. K. Brennaman, P. G. Hoertz, A. O. T. Patrocinio, N. Y. M. Iha, J. L. Templeton, T. J. Meyer, Acc. Chem. Res. 2009, 42, 1954.
| Crossref | GoogleScholarGoogle Scholar | 1:CAS:528:DC%2BD1MXht1Cju7%2FE&md5=0ab3a31f38699703109e991f1259ecc2CAS |
[52] T. Hisatomi, J. Brillet, M. Cornuz, F. Le Formal, N. Tétreault, K. Sivula, M. Grätzel, Faraday Discuss. 2012, 155, in press
| Crossref | GoogleScholarGoogle Scholar | 1:CAS:528:DC%2BC38Xhs1emu78%3D&md5=39d2f1c92fbe485ff26b6ebe9709f354CAS |
* This contribution was in part presented at the conference ‘Towards Global Artificial Photosynthesis – Energy, Nanochemistry and Governance’, held on Lord Howe Island, New South Wales, 14–18 August 2011.
† The network and research centre list that is the basis for Fig. 2 was assembled by S. Styring and P. Lindblad, Uppsala University, Sweden in the spring 2009. All networks and research centres from Europe and the US that were active until 2009 were included. Proposals for new networks were not included.
‡ The alternative to conversion and storage of solar energy as a fuel is to produce solar electricity with solar cells and store the electricity in batteries. However, in Li-air batteries which are at the forefront of battery research, the energy content per kilogram is something like wet wood and a factor of around 15 lower than in H2 or 4 lower than in isobutanol, two of the intended solar fuels.[4]