From fertilised oocyte to cultivated meat – harnessing bovine embryonic stem cells in the cultivated meat industry
Eldar Zehorai A * , Ayelet Maor-Shoshani A , Natali Molotski A , Anastasya Dorojkin A , Nitzan Marelly A , Tami Dvash A and Neta Lavon A *A
Abstract
Global demand for animal protein is on the rise, but many practices common in conventional production are no longer scalable due to environmental impact, public health concerns, and fragility of food systems. For these reasons and more, a pressing need has arisen for sustainable, nutritious, and animal welfare–conscious sources of protein, spurring research dedicated to the production of cultivated meat. Meat mainly consists of muscle, fat, and connective tissue, all of which can be sourced and differentiated from pluripotent stem cells to resemble their nutritional values in muscle tissue. In this paper, we outline the approach that we took to derive bovine embryonic stem cell lines (bESCs) and to characterise them using FACS (fluorescence-activated cell sorting), real-time PCR and immunofluorescence staining. We show their cell growth profile and genetic stability and demonstrate their induced differentiation to mesoderm committed cells. In addition, we discuss our strategy for preparation of master and working cell banks, by which we can expand and grow cells in suspension in quantities suitable for mass production. Consequently, we demonstrate the potential benefits of harnessing bESCs in the production of cultivated meat.
Keywords: bovine, cellular agriculture, cultivated meat, cultured meat, differentiation, embryonic stem cells, lab grown meat, pluripotency.
Introduction
With global demand for protein on the rise, efficient and scalable solutions are needed to access safe and nutritious animal products without overstepping planetary boundaries, defined as the environmental limits within which humanity can safely operate without destabilising earth’s eco-system (Goodwin and Shoulders 2013; Mattick 2018). One such solution is cellular agriculture, which harnesses cells and tissue engineering techniques to grow animal products in controlled environments (Reddi 1994; Mol et al. 2005; McLeod 2011; Goodwin and Shoulders 2013; Mattick 2018; Ben-Arye and Levenberg 2019; Hubalek et al. 2022; Ianovici et al. 2022). Conventional meat primarily contains skeletal muscle (90%), fat and connective tissues (10%) and blood vessels (<1%) (Listrat et al. 2015, 2016). In recent years, skeletal muscle, fat, and connective tissues have been engineered for various medical purposes such as treating muscle dystrophy, transplantations, and repairing soft tissue defects (Gomillion and Burg 2006; Vandenburgh et al. 2008; Yao et al. 2013; Nam et al. 2015). Much of this science is transferred to cellular agriculture, opening the door for cultivated meat – an application of animal cells – to address many of humanity’s most formidable food-related challenges. Several factors are currently challenging and need to be overcome to produce cultivated meat in scale. These include developing a scalable and low-cost cultivation process, sourcing non-animal-derived growth media, facilitating consumer acceptance and sourcing cells.
Pluripotent stem cells as cell source for cultivated meat
Pluripotent stem cells (PSCs) are natural candidates as a cell source for the cultivated meat industry. These cells are able to self-renew and have indefinite proliferative capabilities with a high growth rate, both essential qualities for the development of an efficient, cost-effective cultivated meat production process (Edelman et al. 2005; Lavon et al. 2018; Mattick 2018; Ben-Arye and Levenberg 2019; Lavon 2022). Bovine PSCs can give rise to the mesoderm germ layer and further differentiate to myocytes, adipocytes, and fibroblasts, which together define the structure and organoleptic properties of cultivated meat products. The clear advantages of using PSCs in the cultivated meat industry stands out when considering the establishment of cell banks as a cellular reservoir for process production, where one vial of cells can be used in several production batches and one cell bank can yield indefinite amounts of meat. In addition, due to their size and morphology, PSCs can reach high cell density (cells/cm2) when grown in 2D culture vessels, and can be cultured in suspension, form aggregates and even grow on microcarriers (Singh et al. 2010; Kwok et al. 2017; Lavon et al. 2018; Lipsitz et al. 2018; Manstein et al. 2019; Bodiou et al. 2020), attributes that are needed for 3D culture which simplify upscaled procedures.
PSCs can be sourced as either embryonic stem cells (ESCs) from the inner cell mass, or as induced pluripotent stem cells (iPSCs) from somatic cells. In various papers, stem cells were shown to be produced from ovine, porcine, chicken, fish and bovine (Collodi et al. 1992; Wakamatsu et al. 1994; Pain et al. 1996; Fuet and Pain 2017; Kinoshita et al. 2021). However, unlike human or mouse species, limited studies were conducted to study the derivation of chicken, bovine, ovine and fish PSCs. Bovine ESCs (bESCs), which are used as a source of cells for the meat product developed by Aleph Farms (Lavon 2022), are derived from the inner cell mass (ICM) of a blastocyst, formed 7–8 days post fertilisation, and collected in a non-surgical procedure from the donor cow (Evans and Kaufman 1981; Martin 1981; Bogliotti et al. 2018). Once the blastocyst is transferred to the lab, the ICM is isolated, either surgically or enzymatically, and cultured to produce bESCs. Specific medium and growth factors are required to maintain the propagation and the pluripotency of the derived cells, and to inhibit their spontaneous differentiation (Chan et al. 2013; Gafni et al. 2013; Valamehr et al. 2014; Ware et al. 2014). iPSCs are produced by reprogramming somatic cells to express OCT4, KLF4, C-MYC and SOX2 transcription factors, also known as the Yamanaka factors. These cells were shown to be produced from porcine, chicken, fish and bovine sources (Ezashi et al. 2009; Wu et al. 2009; Fuet and Pain 2017; Peng et al. 2019; Su et al. 2021). The Yamanaka factors can be expressed using either viral vectors, episomes, mRNA transfection or any other method of expression (Takahashi and Yamanaka 2006; Seki and Fukuda 2015; Karagiannis et al. 2019). As for ESCs, culture conditions and medium supplements must be maintained to prevent spontaneous differentiation of iPSCs, and using specific inducers they can be differentiated to any cell type. While ESCs and iPSCs have similar propagation and differentiation abilities, epigenetic traits of iPSCs may affect these capabilities. Moreover, reprogramming of somatic cells to iPSCs may also introduce genetic modification to the produced cell line and as a result they may be labelled as genetically modified products (Rosselló et al. 2013; Pillai et al. 2019). For the above reasons, we chose bESCs as our source of cells.
Cell sourcing for cultivated meat industry
The cellular composition of cultivated meat is of mainly skeletal myocytes with integration of adipocytes and fibroblasts. In the production process of cultivated meat, PSCs (either ESCs or iPSCs) are differentiated to mesenchymal stem cells (MSCs), which can be found for instance in bone marrow, adipose, and skeletal tissues. In turn, MSCs are further differentiated to adipocytes, fibroblasts and to some skeletal muscle cells (Prockop 1997; Okamura et al. 2018). Upon selection of cell source for the cultivated meat industry, several parameters, such as genetic background, species breed and gender, need to be considered. The genetic background of cells utilised to produce ESCs or iPSCs can in turn affect their differentiation potential. For instance, differentiation potential of iPSCs sourced from adipose- derived MSCs differs from bone marrow–derived iPSCs (Dai et al. 2021; Zagury et al. 2022). However, in regards of ESCs, it is yet unclear if the origin of the breed can affect the pluripotency or differentiation potential of the ESCs.
In this article review we describe the approaches taken to derive Aleph Farms’s bESCs and the means we took to characterise them. We show our ability to differentiate our bESCs to MSCs and discuss their potential differentiation to the cell types required for cultivated meat production. We finally outline our strategy for preparation of Master and Working Cell Banks that allow us to indefinitely expand and grow our bESCs and show our ability to grow our cells in suspension in amounts suitable for mass production. All the above highlights the potential use of harnessing bESCs to promote the production process of a high quality, nutritional, and economically feasible cultivated meat.
Materials and methods
Collection of flushed embryos
Bovine heifers were superovulated 8–12 days post oestrus by injection of FSH and prostaglandins (PG), then inseminated 5 days post initiation of superovulation. Seven days post insemination, the embryos were collected by non-surgical uterine flush using complete flush medium (Agtech, ME, USA). At the farm, under binocular microscope, blastocysts were collected to a holding cap and transferred to 5-well plates (minitube culture dish, WI, USA) containing holding medium (Agtech, ME, USA). Embryos were then counted and examined for their stage and grade, then transferred to Aleph’s labs.
Derivation of bovine embryonic stem cell line
At the laboratory, embryos were placed in a biological safety cabinet and carefully washed in CSC-NXc medium (Irvine Scientific, CA, USA) to remove residual holding medium. The stage and grade of the embryos were re-examined under a binocular microscope. Then, they were carefully transferred to CSC-NXc drops, covered in oil for embryos (Irvine Scientific, CA, USA) and incubated overnight at 38.5°C, 5% CO2, to allow their maturation. The following day, in the biological safety cabinet under binocular microscope, embryos at the blastocyst stage were surgically cut to isolate their ICM. Embryos were then carefully transferred to 12-well plates pre-seeded with feeder layer in mTeSR1 medium (StemCell technologies, BC, CA) supplemented with growth factors and incubated at 38.5°C, 5% CO2 for 48 h. Medium of isolated ICMs was replaced daily and the wells were examined daily until detection of initial colonies.
Cell culture maintenance
The cells of the initial colonies were incubated at 38.5°C, 5% CO2 and grown in mTeSR1 medium (StemCell technologies, BC, CA) supplemented with growth factors and replaced daily. Cells were allowed to grow and propagate and were transferred to a new culture growth plate when they reached full confluency. Each transfer is considered as one passage.
Cell bank preparation
Following several propagation cycles, a seed stock of 30 vials was cryopreserved, each containing 1 × 106 cells. Vials were frozen in designated containers allowing cooling of 1°C/min at (−80°C) and transferred to liquid nitrogen for storage 1–3 days following freezing. To create a Master Cell Bank (MCB), one vial from the seed stock was thawed and propagated for several passages, in an increasing vessel size and volume. Once reaching sufficient cell numbers, cells were detached and 200 vials, each containing 5 × 106 cells, were frozen and stored as described. To create a Working Cell Bank (WCB), one vial from the MCB was thawed and propagated for several passages, in an increasing vessel size and volume. Once reaching sufficient cell numbers, cells were detached and >200 vials, each containing 5 × 106 cells, were frozen and stored as described.
Immunofluorescence staining
Cells were carefully washed in phosphate-buffered saline (PBS), then fixed with 4% paraformaldehyde (PFA) soultion (Biolab, IL). PFA was then discarded, and the cells were washed in PBS. Further blocking was done in 5% bovine serum albumin (BSA), and 0.2% Triton X-100 in PBS followed by incubation with primary antibodies in PBS, containing 1% BSA: PE-OCT4 (Abcam, MA, USA), Brachyury (R&D Systems MN, USA).
Next, cells were washed in PBS. In case the primary antibody was not conjugated, cells were incubated with a secondary antibody, goat-anti-mouse Alexa fluoro-594 (Abcam, MA, USA). Finally, cells were washed in PBS followed by nuclei stained using DAPI (4′,6-diamidino-2-phenylindole) (Sigma Aldrich, MO, USA). Imaging was performed using EVOS fluorescence microscopy.
Flow cytometry analysis
Cells were washed in PBS and incubated with anti-SSEA-4-FITC (R&D Systems MN, USA) in FACS (fluorescence-activated cell sorting) staining buffer (R&D Systems) and then washed with PBS. Data acquisition was done using Attune NxT flow cytometer (Thermo Scientific, MA, USA). Data analysis was performed using Kaluza software.
Real-time PCR
Total RNA was extracted from cells using NucleoSpin RNA purification kit (MACHEREY-NAGEL, DE). One microgram of total RNA was reverse transcribed by RevertAid First Strand cDNA Synthesis Kit (ThermoScientific, MA, USA) using the Thermal Cycler SimpliAmp device (ThermoScientific, MA, USA). Real-time PCR was performed using specific primers with TaqMan Fast Advanced Master Mix (ThermoScientific, MA, USA) using a QuantStudio 5 Real-time PCR device (ThermoScientific, MA, USA). Readouts were normalised to a housekeeping level. Data is presented as mean fold change using the 2(−ΔΔCT) method.
Results
Derivation of bovine embryonic stem cell lines
bESCs were derived from the ICM of blastocysts. At the farm, superovulated female bovines heifers were artificially inseminated. Then, 7 days post insemination, embryos were non-surgically flushed, collected and visualised using binocular microscope to determine their stage and grade. Embryos were then transferred to Aleph Farms’s laboratory and incubated in a medium that allows their recovery and maturation (Fig. 1a). The following day, embryos were examined under the microscope and their maturation into the blastocyst stage was determined. Mature embryos were then individually transferred to a culture plate pre-seeded with feeder-layer, and each embryo was surgically dissected to isolate its ICM. Isolated ICMs attached and adhered 24 h post seeding (Fig. 1b) and initial colonies showing ES-like morphology were visualised 5 days post seeding (Fig. 1c).
Derivation of bovine embryonic stem cell line. (a) Embryos flushed 7 days post in vivo insemination, imaged following recovery, and maturation. (b) Isolated inner cell mass (ICM) attached to feeder layer (images acquired 8-days post seeding using bright field EVOS-Fl microscope (×10 and 20 magnification).
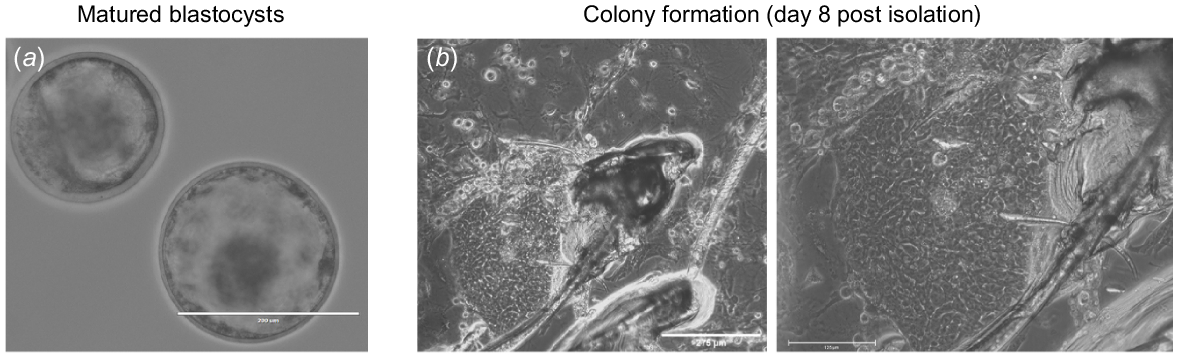
Bovine embryonic stem cell line characterisation
The initial colony was allowed to grow and proliferate on feeder layer for five passages. Then, the pluripotency features of the newly derived bESCs were characterised. Immunofluorescence staining against the pluripotency marker OCT4, showed its presence in over 90% of the imaged colony cells (Fig. 2a). This finding was confirmed by flow cytometry analysis using the pluripotency marker SSEA4, showing positive staining of 97% of the gated population (Fig. 2b). We next employed real-time PCR analysis on two derived cell lines that showed the expression of the pluripotent markers NANOG, SALL4, DNMT3B and OCT4 (Fig. 2c).
Bovine embryonic stem cell line characterisation. (a) Morphological features of bESC line, imaged in brightfield (right) or using immunofluorescence staining using anti-OCT4 antibody and DAPI (left and centre) for nuclei visualisation (unstained cells presented in lower panel, n = 3). (b) Flow cytometry analysis of cells positive to stage-specific embryonic antigen-4 (SSEA4). (c) Real-time PCR analysis of two derived cell lines shows the expression of pluripotent markers: NANOG, SALL4, DNMT3B and OCT4 (expression level relative to non-pluripotent cell line – bovine embryonic fibroblasts (BEFs)).
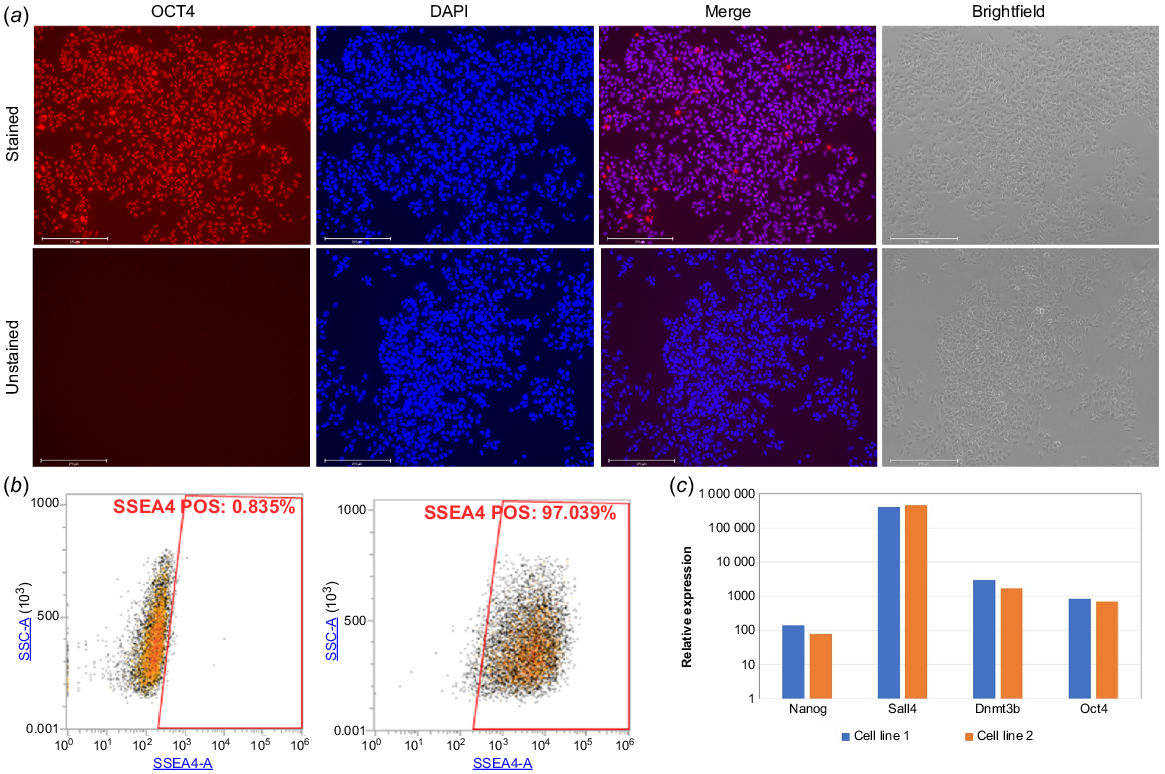
Differentiation of bESCs to mesoderm committed cells
PSCs can be differentiated to the three germ layers: endoderm, ectoderm, and mesoderm. We evaluated the potential of our cells to differentiate to mesoderm committed cells (MCCs). To test the potential use of our derived bESCs, we cultured them in media either maintaining their pluripotency or i inducing their differentiation to MCCs. Real-time PCR analysis showed that while OCT4 and NANOG expression was high in medium maintaining pluripotency, it was significantly reduced following incubation in differentiation medium. In addition, accompanied with OCT4 and NANOG downregulation, we noticed an increase in the expression of the mesenchymal markers Brachyury (TBXT) and TBX6 following induction of differentiation (Fig. 3a). Increase in the expression of Brachyury was also observed at the protein level using immunofluorescence staining (Fig. 3b).
Differentiation of bESCs to mesoderm committed cells. (a) Following incubation with differentiation medium, real-time PCR analysis shows the downregulation of pluripotent markers OCT4 and NANOG and the upregulation of mesoderm markers Brachyury (TBXT) and TBX6 (expression level relative to bESCs) (b) Immunofluorescence staining against mesenchymal marker Brachyury (TBXT), shows its expression in differentiated cells (upper panel) and not in pluripotent cells (lower panel) (n = 3).
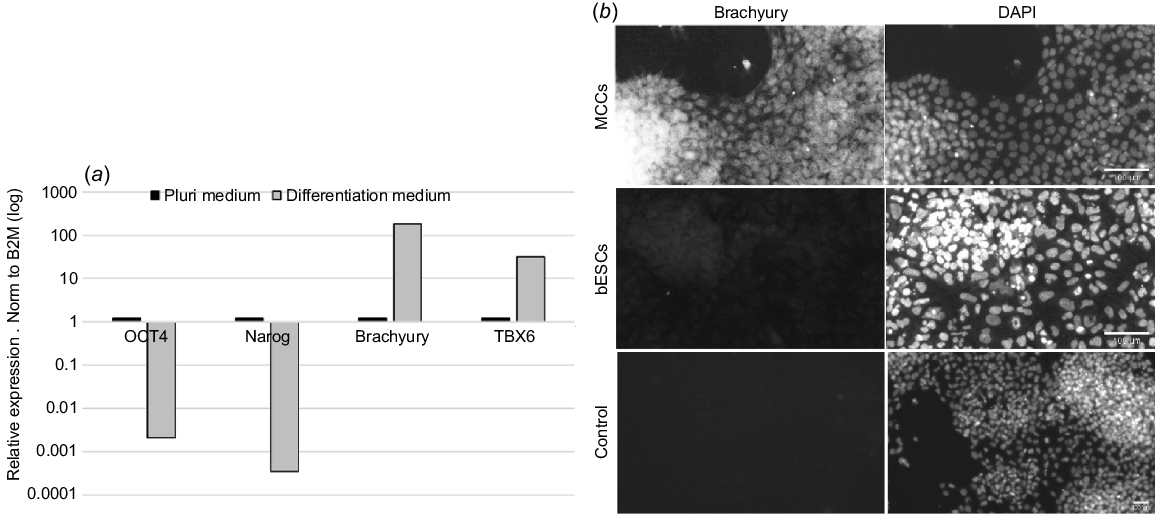
Cell bank preparation and characterisation
The main advantage of bESCs is their indefinite proliferative capability and high growth rate, both essential for the high population doubling levels required throughout the production process of cultivated meat. In addition, by harnessing bESCs for the establishment of cell banks, one can avoid repeated biopsy collection. Cell bank samples are extensively characterised for their growth profiles, characteristics, and assurance that they are free of adventitious agents, ensuring a safe, reproducible, and consistent production process. Furthermore, bESCs are cost-effective since one vial of thawed cells from an MCB is sufficient for the establishment of an entire WCB, each composed of at least 200 vials. In turn, each vial of WCB can be used for an entire production process (Fig. 4a). Thus, from a single derivation procedure using one blastocyst, more than 40 000 batches of production processes can be performed, giving rise to thousands of tonnes of cultivated meat products. After establishing our cell banks, we tested our bESCs for their growth and pluripotency during extended passages, correlating to entire production process. Growth rate, represented as the population doubling time (PDT) of cells was found to be ~24 h on average, demonstrating the high proliferative growth rate of our bESCs. Flow cytometry analysis showed that >80% of cells were expressing SSEA4, representing the high pluripotency of our bESCs. Both growth rate and pluripotency were found to be stable along 65 population doubling levels (PDLs), demonstrating the potential use of bESCs in the cultivated meat industry (Fig. 4b, c). In addition, we employed RNA sequence-based digital karyotyping across different generations to test genomic stability along 15 passages. RNA expression per chromosome was calculated based on the expression level of genes located on each chromosome. The average fold change between the early and late passages was calculated and found to be of similar expression (Fig. 4d). Thus, all employed analyses confirmed the stability of our derived cell lines.
Cell bank preparation and characterisation. (a) Schematic representation of Master Cell Bank (MCB) and Working Cell Bank (WCB) preparation, each containing >200 vials proved and tested to be free of adventitious agents. (b, c) Cells were tested for their growth and pluripotency during extended passages correlating to the entire cultivated meat production process. Growth rate, presented by population doubling time (PDT; b) and pluripotency presented by population percentage positive for SSEA4 marker (c), were found to be stable along 65 population doubling levels (PDL). Average PDT was found to be ~24 h. The blue line represents the acceptance criteria of ≥80% cells positive for SSEA4. (d) Digital karyotyping shows genomic stability between the early and late passages.
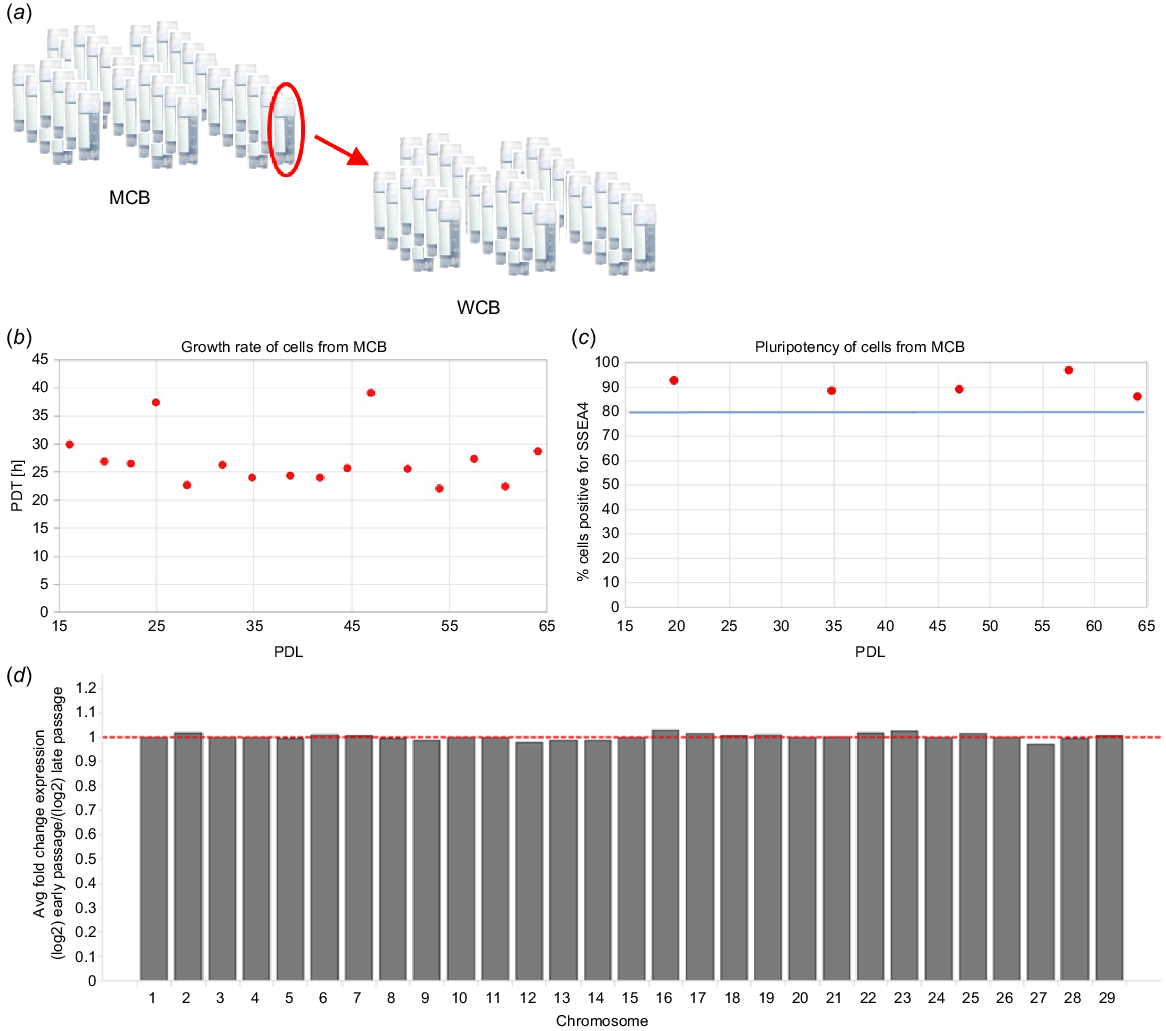
Cell growth rate and stability
The use of bESCs is cost-effective, since one thawed vial of cells is sufficient for several production batches. In addition, compared to satellite and mesenchymal cells, due to their small size and tendency to crowd, bESCs can reach a high cell density in 2D culture (cells per cm2), simplifying scale-up procedures prior to cell seeding in bioreactors. In addition, bESCs can also grow in suspension as aggregates, or attached to edible microcarriers which enables 3D culture in bioreactors. We therefore tested and demonstrated the ability of our derived bESC lines to grow as aggregates in small-scale vessels and showed the growth of aggregate size over 4 days of culture, indicative of cell growth (Fig. 5a). We next tested the growth of our bESC lines in stirred tank bioreactors (STBs) and showed that viable cell concentration increased by an average of 50-fold in 7 days in STBs, demonstrating that extensive growth can be obtained (Fig. 5b).
Cell growth and stability. (a) Representative brightfield images showing bESCs cultured as aggregates grown in growth medium in suspension. Aggregate size increased during 4 days in culture, indicative of cell growth. (b) Viable cell number increased by an average of 50-fold in 7 days in an STB, tested in four different STB runs.
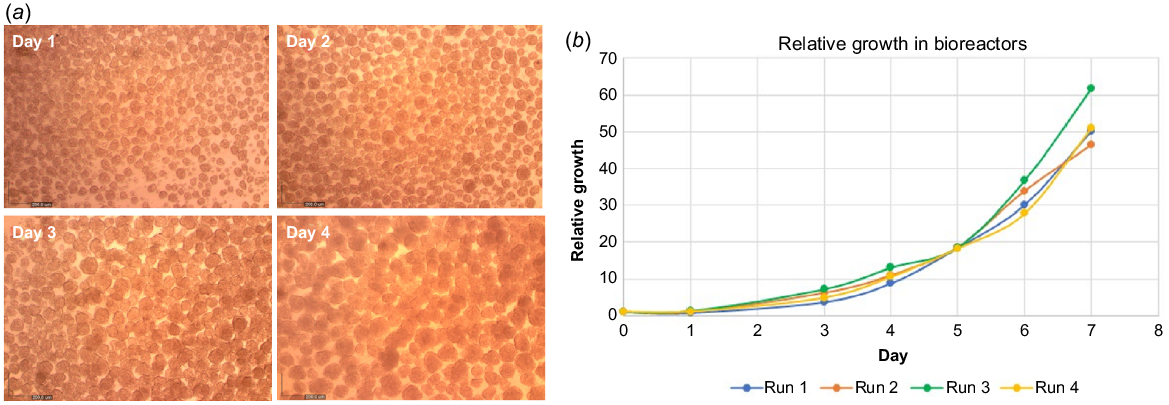
Discussion
Cellular agriculture – including one of animal cell’s most promising applications, cultivated meat – can help meet demand for accessible and high-quality nutrition. Producing cultivated meat requires a process of several steps, including sourcing cells, derivation of pluripotent stem cells, their propagation and expansion, and finally their differentiation into the desired specialised cells that form the final cultivated meat product. As an initial cell source for the production process, iPSCs and ESCs are both excellent candidates with similar propagation and differentiation characteristics. However, each type harbours different benefits and traits that should be considered when sourcing cells. While ESCs are derived in a procedure that does not require any genetic modification due to their early stage of development, their use may raise consumer acceptance issues. In the case of iPSCs, while their somatic origin frees us from any ethical issues and may prove to be beneficial due to epigenetic traits (or genetic memory of somatic cell of origin) they require genetic modification of somatic cells and in turn may also present regulatory concerns. That being said, PSCs stand out as a source of cells for cultivated meat products when one considers their capacity for indefinite propagation and expansion.
In this paper, we show the strategy we chose, following previously published papers, to produce our PSC lines by isolating the ICM from fertilised oocytes to form initial bESC colonies. Validation of our bESCs was performed by sequential analysis to ensure the combined expression of pluripotency markers. Initially, bESCs were analysed using FACS for the pluripotency marker SSEA4, showing positive staining of over 97% of the gated population. Next, using real-time PCR analysis, the gated cell population showed the expression of the pluripotency markers NANOG, SALL4, DNMT3B and OCT4, the latter further validated using immunofluorescence staining. Finally, following induced differentiation, we showed the downregulation of these markers accompanied by upregulation of mesenchymal markers Brachyury (TBXT) and TBX6. Next, we describe the procedure that provides an initial cell bank of PSCs which usually yields several vials. Out of each vial, an MCB of at least 200 vials can be prepared, and in turn a WCB of the same number of vials can be produced, thus translating to at least 40 000 production batches of thousands of tonnes of cultivated meat. Additionally, our PSC lines were shown to maintain pluripotency and genetic stability, ensuring the recurrence of product production. By avoiding the need for repeated biopsies from animals, we decrease the costs of cell isolation and analytics and increase food safety and security of our meat production process and the final products. Another advantage of this process is that PSC vials are easily transported, reducing their carbon footprint, and advancing the process’s overall sustainability.
The global rise in demand for protein has made it essential that humanity develops food production processes that support and maintain the ecosystem, strengthen global and national food security, and reduce land and soil stress. In this paper, we described the strategies we implemented to harness the indefinite propagation and expansion properties of bESCs to develop an efficient and robust production process of high-quality cultivated meat products. At Aleph Farms, we are committed to furthering technology that supports a just and inclusive transition to sustainable and secure food systems. As we launch our products globally, we will expand our impact in terms of improving sustainability, food security and animal welfare in these systems – and it all starts with one fertilised oocyte.
Data availability
The data that support this study will be shared upon reasonable request to the corresponding author.
Acknowledgements
The authors of this paper wish to thank Dr Rachel Eiges for her guidance and support, Dr Ronit Bakimer for her valuable insights and Dr Ori Koren for his contribution to this study.
References
Ben-Arye T, Levenberg S (2019) Tissue engineering for clean meat production. Frontiers in Sustainable Food Systems 3, 46.
| Crossref | Google Scholar |
Bodiou V, Moutsatsou P, Post MJ (2020) Microcarriers for upscaling cultured meat production. Frontiers in Nutrition 7, 10.
| Crossref | Google Scholar |
Bogliotti YS, Wu J, Vilarino M, Okamura D, Soto DA, Zhong C, Sakurai M, Sampaio RV, Suzuki K, Izpisua Belmonte JC, Ross PJ (2018) Efficient derivation of stable primed pluripotent embryonic stem cells from bovine blastocysts. Proceedings of the National Academy of Sciences of the United States of America 115(9), 2090-2095.
| Crossref | Google Scholar |
Chan Y-S, Göke J, Ng J-H, Lu X, Gonzales KAU, Tan C-P, Tng W-Q, Hong Z-Z, Lim Y-S, Ng H-H (2013) Induction of a human pluripotent state with distinct regulatory circuitry that resembles preimplantation epiblast. Cell Stem Cell 13, 663-675.
| Crossref | Google Scholar |
Collodi P, Kame Y, Ernst T, Miranda C, Buhler DR, Barnes DW (1992) Culture of cells from zebrafish (Brachydanio rerio) embryo and adult tissues. Cell Biology and Toxicology 8(1), 43-61.
| Crossref | Google Scholar | PubMed |
Dai X, Guan Y, Zhang Z, Xiong Y, Liu C, Li H, Liu B (2021) Comparison of the differentiation abilities of bone marrow-derived mesenchymal stem cells and adipose-derived mesenchymal stem cells toward nucleus pulposus-like cells in three-dimensional culture. Experimental and Therapeutic Medicine 22(3), 1018.
| Crossref | Google Scholar |
Edelman PD, McFarland DC, Mironov VA, Matheny JG (2005) Commentary: in vitro-cultured meat production. Tissue Engineering 11(5–6), 659-662.
| Crossref | Google Scholar |
Evans MJ, Kaufman MH (1981) Establishment in culture of pluripotential cells from mouse embryos. Nature 292, 154-156.
| Crossref | Google Scholar | PubMed |
Ezashi T, Telugu BPVL, Alexenko AP, Sachdev S, Sinha S, Roberts RM (2009) Derivation of induced pluripotent stem cells from pig somatic cells. Proceedings of the National Academy of Sciences of the United States of America 106(27), 10993-10998.
| Crossref | Google Scholar |
Gafni O, Weinberger L, Mansour AAF, Manor YS, Chomsky E, Ben-Yosef D, Kalma Y, Viukov S, Maza I, Zviran A, Rais Y, Shipony Z, Mukamel Z, Krupalnik V, Zerbib M, Geula S, Caspi I, Schneir D, Shwartz T, Gilad S, Amann-Zalcenstein D, Benjamin S, Amit I, Tanay A, Massarwa R, Novershtern N, Hanna JH (2013) Derivation of novel human ground state naive pluripotent stem cells. Nature 504, 282-286.
| Crossref | Google Scholar |
Gomillion CT, Burg KJL (2006) Stem cells and adipose tissue engineering. Biomaterials 27(36), 6052-6063.
| Crossref | Google Scholar | PubMed |
Goodwin JN, Shoulders CW (2013) The future of meat: a qualitative analysis of cultured meat media coverage. Meat Science 95(3), 445-450.
| Crossref | Google Scholar | PubMed |
Hubalek S, Post MJ, Moutsatsou P (2022) Towards resource-efficient and cost-efficient cultured meat. Current Opinion in Food Science 47, 100885.
| Crossref | Google Scholar |
Ianovici I, Zagury Y, Redenski I, Lavon N, Levenberg S (2022) 3D-printable plant protein-enriched scaffolds for cultivated meat development. Biomaterials 284, 121487.
| Crossref | Google Scholar | PubMed |
Karagiannis P, Takahashi K, Saito M, Yoshida Y, Okita K, Watanabe A, Inoue H, Yamashita JK, Todani M, Nakagawa M, Osawa M, Yashiro Y, Yamanaka S, Osafune K (2019) Induced pluripotent stem cells and their use in human models of disease and development. Physiological Reviews 99, 79-114.
| Crossref | Google Scholar | PubMed |
Kinoshita M, Kobayashi T, Planells B, Klisch D, Spindlow D, Masaki H, Bornelöv S, Stirparo GG, Matsunari H, Uchikura A, Lamas-Toranzo I, Nichols J, Nakauchi H, Nagashima H, Alberio R, Smith A (2021) Pluripotent stem cells related to embryonic disc exhibit common self-renewal requirements in diverse livestock species. Development 148, dev199901.
| Crossref | Google Scholar |
Kwok CK, Ueda Y, Kadari A, Günther K, Ergün S, Heron A, Schnitzler AC, Rook M, Edenhofer F (2017) Scalable stirred suspension culture for the generation of billions of human induced pluripotent stem cells using single-use bioreactors. Journal of Tissue Engineering and Regenerative Medicine 12, e1076-e1087.
| Crossref | Google Scholar |
Lavon N (2022) New technologies for cultivated meat production. Trends in Biotechnology 40, 632-633.
| Crossref | Google Scholar |
Lavon N, Zimerman M, Itskovitz-Eldor J (2018) Scalable expansion of pluripotent stem cells. Advances in Biochemical Engineering/Biotechnology 163, 23-38.
| Crossref | Google Scholar | PubMed |
Lipsitz YY, Woodford C, Yin T, Hanna JH, Zandstra PW (2018) Modulating cell state to enhance suspension expansion of human pluripotent stem cells. Proceedings of the National Academy of Sciences of the United States of America 115(25), 6369-6374.
| Crossref | Google Scholar |
Listrat A, Lebret B, Louveau I, Astruc T, Bonnet M, Lefaucheur L, Bugeon J (2015) How muscle structure and composition determine meat quality. INRA Productions Animales 28(2), 125-136.
| Crossref | Google Scholar |
Listrat A, Lebret B, Louveau I, Astruc T, Bonnet M, Lefaucheur L, Picard B, Bugeon J (2016) How muscle structure and composition influence meat and flesh quality. The Scientific World Journal 2016, 3182746.
| Crossref | Google Scholar |
Manstein F, Halloin C, Zweigerdt R (2019) Human pluripotent stem cell expansion in stirred tank bioreactors. Methods in Molecular Biology 1994, 79-91.
| Crossref | Google Scholar |
Martin GR (1981) Isolation of a pluripotent cell line from early mouse embryos cultured in medium conditioned by teratocarcinoma stem cells. Proceedings of the National Academy of Sciences of the United States of America 78(12), 7634-7638.
| Crossref | Google Scholar |
Mattick CS (2018) Cellular agriculture: the coming revolution in food production. Bulletin of the Atomic Scientists 74(1), 32-35.
| Crossref | Google Scholar |
Mol A, Driessen NJB, Rutten MCM, Hoerstrup SP, Bouten CVC, Baaijens FPT (2005) Tissue engineering of human heart valve leaflets: a novel bioreactor for a strain-based conditioning approach. Annals of Biomedical Engineering 33(12), 1778-1788.
| Crossref | Google Scholar | PubMed |
Nam K-H, Smith AST, Lone S, Kwon S, Kim D-H (2015) Biomimetic 3D tissue models for advanced high-throughput drug screening. SLAS Technology 20(3), 201-215.
| Crossref | Google Scholar | PubMed |
Okamura LH, Cordero P, Palomino J, Parraguez VH, Torres CG, Peralta OA (2018) Myogenic differentiation potential of mesenchymal stem cells derived from fetal bovine bone marrow. Animal Biotechnology 29(1), 1-11.
| Crossref | Google Scholar |
Pain B, Clark ME, Shen M, Nakazawa H, Sakurai M, Samarut J, Etches RJ (1996) Long-term in vitro culture and characterisation of avian embryonic stem cells with multiple morphogenetic potentialities. Development 122(8), 2339-2348.
| Crossref | Google Scholar | PubMed |
Peng L, Zhou Y, Xu W, Jiang M, Li H, Long M, Liu W, Liu J, Zhao X, Xiao Y (2019) Generation of stable induced pluripotent stem-like cells from adult zebra fish fibroblasts. International Journal of Biological Sciences 15(11), 2340-2349.
| Crossref | Google Scholar | PubMed |
Pillai VV, Kei TG, Reddy SE, Das M, Abratte C, Cheong SH, Selvaraj V (2019) Induced pluripotent stem cell generation from bovine somatic cells indicates unmet needs for pluripotency sustenance. Animal Science Journal 90(9), 1149-1160.
| Crossref | Google Scholar | PubMed |
Prockop DJ (1997) Marrow stromal cells as stem cells for nonhematopoietic tissues. Science 276(5309), 71-74.
| Crossref | Google Scholar | PubMed |
Reddi AH (1994) Symbiosis of biotechnology and biomaterials: applications in tissue engineering of bone and cartilage. Journal of Cellular Biochemistry 56, 192-195.
| Crossref | Google Scholar | PubMed |
Rosselló RA, Chen C-C, Dai R, Howard JT, Hochgeschwender U, Jarvis ED (2013) Mammalian genes induce partially reprogrammed pluripotent stem cells in non-mammalian vertebrate and invertebrate species. eLife 2, e00036.
| Crossref | Google Scholar |
Seki T, Fukuda K (2015) Methods of induced pluripotent stem cells for clinical application. World Journal of Stem Cells 7(1), 116.
| Crossref | Google Scholar | PubMed |
Singh H, Mok P, Balakrishnan T, Rahmat SNB, Zweigerdt R (2010) Up-scaling single cell-inoculated suspension culture of human embryonic stem cells. Stem Cell Research 4, 165-179.
| Crossref | Google Scholar | PubMed |
Su Y, Wang L, Fan Z, Liu Y, Zhu J, Kaback D, Oudiz J, Patrick T, Yee SP, Tian X, Polejaeva I, Tang Y (2021) Establishment of bovine-induced pluripotent stem cells. International Journal of Molecular Sciences 22(19), 10489.
| Crossref | Google Scholar | PubMed |
Takahashi K, Yamanaka S (2006) Induction of pluripotent stem cells from mouse embryonic and adult fibroblast cultures by defined factors. Cell 126, 663-676.
| Crossref | Google Scholar |
Valamehr B, Robinson M, Abujarour R, Rezner B, Vranceanu F, Le T, Medcalf A, Lee TT, Fitch M, Robbins D, Flynn P (2014) Platform for induction and maintenance of transgene-free hiPSCs resembling ground state pluripotent stem cells. Stem Cell Reports 2, 366-381.
| Crossref | Google Scholar | PubMed |
Vandenburgh H, Shansky J, Benesch-Lee F, Barbata V, Reid J, Thorrez L, Valentini R, Crawford G (2008) Drug-screening platform based on the contractility of tissue-engineered muscle. Muscle & Nerve 37, 438-447.
| Crossref | Google Scholar | PubMed |
Wakamatsu Y, Ozato K, Sasado T (1994) Establishment of a pluripotent cell line derived from a medaka (Oryzias latipes) blastula embryo. Molecular Marine Biology and Biotechnology 3(4), 185-191 Available at https://europepmc.org/article/med/8000476.
| Google Scholar | PubMed |
Ware CB, Nelson AM, Mecham B, Hesson J, Zhou W, Jonlin EC, Jimenez-Caliani AJ, Deng X, Cavanaugh C, Cook S, Tesar PJ, Okada J, Margaretha L, Sperber H, Choi M, Blau CA, Treuting PM, Hawkins RD, Cirulli V, Ruohola-Baker H (2014) Derivation of naïve human embryonic stem cells. Proceedings of the National Academy of Sciences of the United States of America 111(12), 4484-4489.
| Crossref | Google Scholar |
Wu Z, Chen J, Ren J, Bao L, Liao J, Cui C, Rao L, Li H, Gu Y, Dai H, Zhu H, Teng X, Cheng L, Xiao L (2009) Generation of pig induced pluripotent stem cells with a drug-inducible system. Journal of Molecular Cell Biology 1(1), 46-54.
| Crossref | Google Scholar | PubMed |
Yao R, Zhang R, Lin F, Luan J (2013) Biomimetic injectable HUVEC-adipocytes/collagen/alginate microsphere co-cultures for adipose tissue engineering. Biotechnology and Bioengineering 110(5), 1430-1443.
| Crossref | Google Scholar | PubMed |
Zagury Y, Ianovici I, Landau S, Lavon N, Levenberg S (2022) Engineered marble-like bovine fat tissue for cultured meat. Communications Biology 5, 927.
| Crossref | Google Scholar |