Vitamin K: history, metabolism, and nutrition in the horse
Jazmine E. Skinner

A School of Agriculture and Environmental Science, University of Southern Queensland, Toowoomba, Qld 4350, Australia.
B Agricure Pty Ltd., Braemar, NSW 2575, Australia.
C Queensland Alliance for Agriculture and Food Innovation, The University of Queensland, St Lucia, Qld 4072, Australia.
Abstract
Vitamin K (VK) has long been known for its essential role in blood coagulation. However, over the past decade, evidence has mounted for its intrinsic and essential roles in other functions within the body, including bone metabolism, calcification, brain development and glucose metabolism. Thus, VK should no longer be considered a single-function ‘haemostasis vitamin’, but rather as a ‘multi-function vitamin’. While current research has focused on its emerging role in human nutrition, the role that VK plays in other species such as the horse has not been well described, with most of our current understanding having been extrapolated from other species, especially rodents. This review assesses the current state of knowledge of VK as it pertains to human and animal nutrition, and, where data exist, its metabolism and nutrition in the horse is explored. Future research on the roles of VK as they pertain to horses, particularly extra-hepatic functions, is necessary. Such insight will allow a greater understanding of how VK is metabolised, facilitating the development of recommendations to assist in the health, growth, and longevity of horses.
Keywords: animal nutrition, equine nutrition, menadione (MD), menaquinones (MK-n), osteocalcin (OC), phylloquinone (PK), vitamin K (VK), vitamin K-dependent proteins (VKDPs).
Introduction
Vitamin K (VK) consists of a group of structurally related compounds (Fig. 1), all of which have a 2-methyl-1, 4-naphthoquinone ring system, and differ in the structure of their isoprenoid side chain at the 3- position (Shearer and Newman 2014). The two naturally occurring classes of VK are phylloquinone (PK, also referred to as K1), which is synthesised in a single form by plants, and the menaquinones (MK-n, also referred to as K2), which are synthesised in a series of forms (MK-4 to MK-14) by intestinal bacteria. Menadione (MD; sometimes referred to as K3) is the synthetic form of the vitamin and lacks a side chain and is more accurately described as a provitamin. It can have toxic side-effects in humans and horses (Rebhun et al. 1984; Thijssen et al. 2006) but is routinely added to animal diets. The different forms of a vitamin are sometimes referred to as vitamers.
The different forms of vitamin K (VK): phylloquinone (PK), menaquinones (MK-n), menaquinone-4 (MK-4) and menadione (MD).
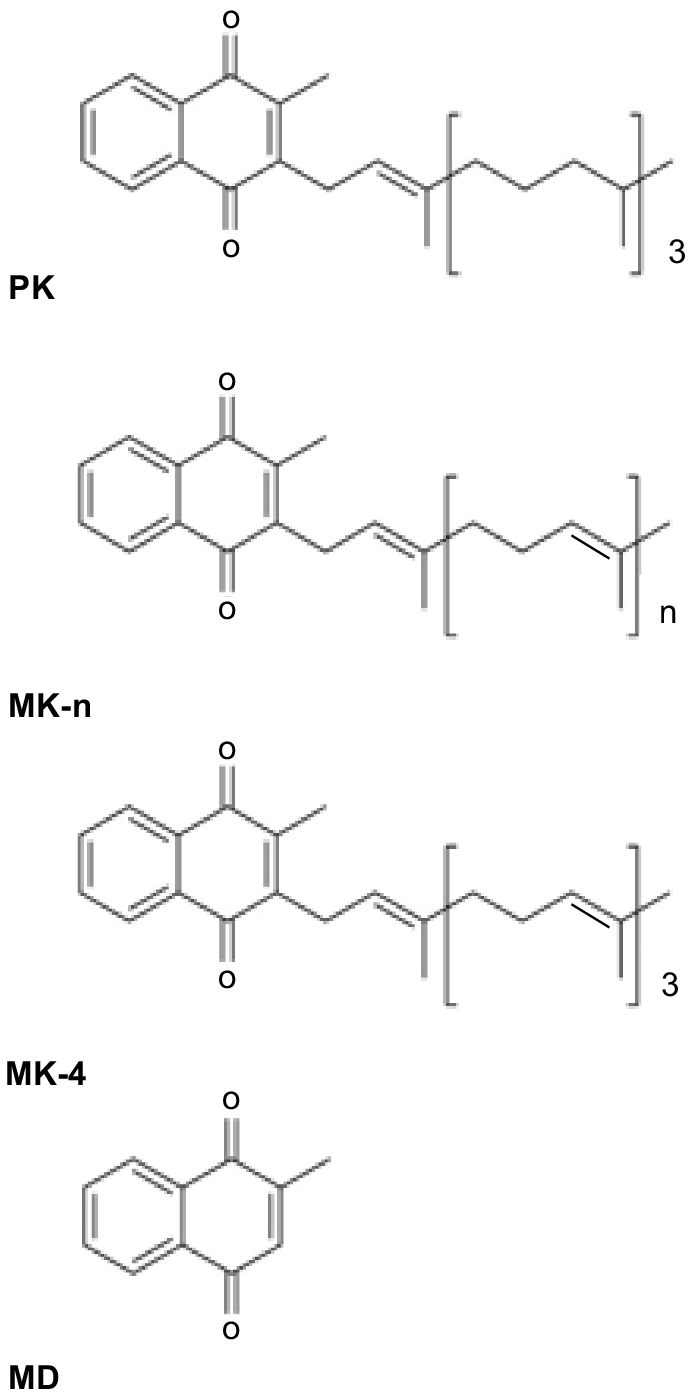
VK has received considerably less attention over the past 50 years than have other fat-soluble vitamins. Intakes of VK beyond that required for normal blood coagulation were believed to confer no additional benefits, and have therefore been rarely investigated (Booth and Rajabi 2008). The requirements and physiological role of VK in humans appeared to be well known (Booth 2009), especially as blood coagulation disorders were rarely reported. This view of VK has changed following the delineation of its cofactor role in protein carboxylation, and the growing awareness of its interactions with other essential metabolic functions in tissues throughout the body, beyond that of blood coagulation, including metabolism of bone, sphingolipids, energy, and inflammation (Shearer et al. 2012). At present, 17 vitamin K-dependent proteins (VKDPs) have been characterised (Table 1), but the functionality of some remains to be elucidated (Shearer and Okano 2018). However, little research has been undertaken into the role that VK may play beyond coagulation in the horse.
VKDP | Location | Function | |
---|---|---|---|
Hepatic proteins | |||
Prothrombin, Factor VII, IX, and X | Liver | Coagulation | |
Proteins C, S and Z | Unknown | Anti-coagulation | |
Extra-hepatic proteins | |||
Matrix Gla-protein (MGP) | Cartilage, vascular tissue | Inhibition of calcification | |
Osteocalcin | Osteoblasts | Bone turnover and glucose metabolism | |
Gla-rich protein (GRP) | CNS, spinal cord, thyroid | Modulation of Ca | |
Gas6 | Smooth muscle, endothelium | Regulation of vascular homeostasis and sphingolipid metabolism | |
Periostin | Periosteum, osteoblasts, remodelling tissues | Fibrilogenesis and wound healing | |
Keratoepithelin | Most extra-hepatic tissues | Microtubule stability and positive regulation of mutations. | |
Transmembrane Gla-proteins (TMG) 1, 2, 3, 4 | Unknown | Unknown |
This review will explore the metabolism of VK as it relates to coagulation, and its role in other metabolic processes beyond that of coagulation. While current research has focused on its emerging role in human nutrition, the role that VK plays in other species such as the horse has not been well described, with most of our current understanding being extrapolated from other species such as rodents; caution should be exercised when extrapolating to other species. Where data exist, the role of VK and VKDPs in the horse will be discussed along with other relevant studies. The possible roles of VK in the modulation of equine metabolic diseases will also be addressed. However, before turning to the horse, an overview of the history, along with our current understanding of VK metabolism and function, is discussed.
Historical perspective
VK was discovered independently in 1935 by two research scientists, namely, Henrich Dam in Denmark (Dam 1935a, 1935b) and Herman Almquist in the USA (Almquist and Stokstad 1935a, 1935b). Two references are cited for both Dam and Almquist here, as both references are cited interchangeably for each author when the discovery of VK is reviewed. This new dietary factor cured a haemorrhagic condition that developed in chicks fed low-lipid diets. Dam (1935a) named the factor VK, as it was the next letter in the alphabet that had not been used to designate a vitamin and coincidently the first letter of the Danish word ‘koagulation’. In 1943, the Nobel Prize in Physiology or Medicine was co-awarded to Henrik Dam ‘for his discovery of vitamin K’ and to Edward Doisy ‘for his discovery of the chemical nature of vitamin K’. Dam and Almquist have both given accounts of the discovery of VK (Almquist 1941, 1975; Dam 1942) and it is interesting to compare these accounts, with those prepared by Jukes (1980), Bentley and Meganathan (1982), and van Oostende et al. (2011).
At about the time of the discovery of VK, there was interest in North America in a haemorrhagic condition that occurred predominantly in cattle consuming mouldy sweet clover (Melilotus alba and Melilotus officinalis) hay (Cheeke 1998). Horses also suffered from sweet clover poisoning/toxicity, but the only recorded case is of a Percheron mare in Canada (McDonald 1980). Link (1959) provided a fascinating description of the discovery of dicumarol, the compound responsible for sweet clover poisoning/toxicity. Sweet clover contains a glycoside that is converted to dicumarol by fungal metabolism. The isolation of dicumarol and its derivatives, which were shown to be potent VK antagonists, was conducted at the University of Wisconsin. The most widely used dicumarol derivative is warfarin. Warfarin is named for the Wisconsin Alumni Research Foundation, and it is used globally in human and veterinary medicine and as a rodenticide.
A breakthrough in our understanding of VK functionality occurred in 1974 when John Suttie (also University of Wisconsin) and colleagues discovered the VK-dependent (VKD) carboxylation of γ-carboxyglutamic acid (Gla) residues in the liver microsomes of rodents, paving the way for research to uncover various VK-dependent proteins (VKDPs) throughout the body (Shah and Suttie 1974). It was soon shown that Gla-proteins originating from liver were essential components of the blood-clotting cascade, including prothrombin, factors VII, XI, X, Protein C and S (Price et al. 1980). The discovery of extra-hepatic VKDPs, Gla-protein mRNA and carboxylase in a wide range of tissues suggested that the functions of Gla-proteins and, consequently of VK, extended beyond their well established role in blood clotting (Marchetti et al. 2000). This discovery has led to VK becoming a multifunctional vitamin, with roles in many tissues rather than a single purpose ‘haemostasis’ vitamin. This has prompted further research, resulting in the discovery of several VKDPs with distinct roles in an array of additional functions, including, calcium homeostasis, growth and energy metabolism, signal transduction and apoptosis (Hallgren et al. 2013). Involvement of these proteins extends beyond the liver to metabolism in a range of areas, including bone, sphingolipids, glucose and even reproduction (Oury et al. 2013; Manna and Kalita 2016).
In any discussion of VK, it would be remiss not to include the significant contribution of Carl Martius, a Swiss scientist, made in the 1950s (Martius 1961), before the role of VK in protein carboxylation was known. The discoveries by Martius and his colleagues have been fundamental to unravelling the perplexing questions surrounding the interconversion of VK vitamers; (PK, MD and MK-4) as described by Shearer (2022). They were able to show that the side chain of PK was removed after absorption and the resulting metabolite, MD, a precursor of MK-4, is formed by prenylation of MD in the target tissue (Shearer and Okano 2018). Another important advance has been the isolation of the gene UbiA prenyltransferase domain containing 1 (UBIAD1), that encodes for prenyltransferase, by Okano and his group in Japan (Shearer and Okano 2018). Importantly, a recent study by Ellis et al. (2022) demonstrated that conversion to MK-4 was found for a range of VK vitamers (PK, MK-4, MK-7, MK-9) in different tissues, suggesting that this is a common pathway for in vivo VK metabolism, with MK-4 being the ‘active’ metabolite/vitamer. In this pathway, MD is an intermediary, and this appears to provide an explanation of why MD has been successful as an animal feed supplement. Moreover, this study by Ellis et al. (2022) provided a possible explanation of how the different K vitamers can maintain VK status and biological function of an individual or animal.
Impetus for many of the human VK studies is due to the known links between warfarin therapy, haemodialysis, and chronic kidney disease (CKD) to arterial calcification (Westenfeld et al. 2012; Fusaro et al. 2015; Holden et al. 2015; Fusaro et al. 2017a; McCabe et al. 2017). As a result of the widespread use of warfarin, a VK antagonist, as an anticoagulant, studies have evaluated the effects of its long-term use on patients, and therefore much of the recent knowledge on the functions of VK is based on this body of research. Likewise, research on VK in animals has also been conducted on warfarin and anticoagulation therapies, predominantly utilising rodent models (Belij et al. 2012).
Metabolism, function and assessment of VK status
The metabolism (absorption, distribution, excretion) of VK is complex due to the different molecular forms of the vitamin. Some aspects of metabolism are poorly defined and species differences may exist but require elucidation. To complicate matters, MK-4 appears to be produced in the tissues of mammals via conversion from both PK and MD after either injection or oral supplementation (Al Rajabi et al. 2012). In the intestine, MD may be produced as an intermediary from the conversion of PK within the gut endothelium, where it is converted to MK-4 by the enzyme UBIAD1 (Thijssen et al. 2006). However, as noted above, the recent papers by Ellis et al. (2022) and Shearer (2022) provided an explanation for this apparent confusion. A detailed account of the key features of VK metabolism and function has been given by Shearer and Okano (2018). Except for the very early research with chickens, our current understanding of VK metabolism has been acquired from human studies using rodent models, although there has been some research undertaken with fish (Krossoy et al. 2011).
Absorption, transport, and excretion
Each of the different forms of VK varies in absorption efficiency, reflecting different chemical structures and the rate of release from the food or bacterial matrix in which it is encased (Schurgers and Vermeer 2000). As a fat-soluble substance, VK is absorbed in a manner similar to dietary fats, and there is evidence that absorption of the vitamin is enhanced by dietary fat (Goncalves et al. 2015). The absorption of VK was first described by Hollander (1973), who identified maximal uptake of PK in the duodenum and jejunum of rats. In contrast, Goncalves et al. (2015) found greater uptake in the ileum. In contrast, MK-n occurs in various forms with different side-chain lengths (as denoted by ‘n’; Shearer and Newman 2008). There are short- and long-chain menaquinones from MK-4 to MK-14 (Shearer and Newman 2014) and the length of the side chain influences absorption (Thane et al. 2002). MD, unlike the other forms, lacks a side chain and there has been conjecture as to whether this prevents this form from activating extra-hepatic VKDPs, other than those involved in the blood clotting cascade (Al Rajabi et al. 2012); however, its conversion to MK-4 is now well described.
In the intestinal lumen, PK and MK-n are incorporated into mixed micelles and then taken up by intestinal enterocytes (Shearer et al. 2012). Micelles are a combination of bile salts, products of pancreatic lipolysis and other lipids (Shearer et al. 2012). After being absorbed into the enterocytes, PK is thought to be transported by a carrier protein, but specific carrier proteins for intra-cellular transport across the enterocyte have not been identified (Reboul and Borel 2011). In the enterocyte, the vitamin becomes bound to chylomicrons (Schurgers and Vermeer 2002) and in this configuration it is released into circulation via the lymphatic system to be recruited by the liver (Shea and Booth 2007). The release into circulation of these particles is achieved via the thoracic duct (Shearer and Newman 2008). Receptor mediated uptake of the chylomicron remnants (CR) into the liver is then facilitated by hepatic parenchyma cells (Schurgers and Vermeer 2002).
Transport of VK, like that of vitamin E, appears to be facilitated by lipoproteins; however, unlike vitamin E, VK has no specific plasma carrier protein (Shearer 1995). The primary transporters of PK are believed to be triglyceride-rich lipoproteins (TRL) and very low-density lipoproteins (VLDL; Shea and Booth 2007). After intestinal absorption, both PK and MK-n are transported by these lipoproteins to the liver (Shearer et al. 2012). Binding of the chylomicrons to these lipoproteins allows them to enter the hepatocytes via receptor-mediated endocytosis.
Although VK is absorbed in a manner similar to that of other fat-soluble vitamins it is not stored to the same extent and requires regular dietary replenishment. PK, the major source of VK intake, is rapidly absorbed but efficiently excreted, as shown by Shearer et al. (1974), who found it to be rapidly metabolised and excreted in bile (40–50%) via faeces, and in urine as glucuronide conjugates (20%) (Barkhan and Shearer 1977). Similar studies have not been undertaken with MK-n but large concentrations have been found in faeces, presumably reflecting poor absorption in the hindgut (Karl et al. 2017). Interestingly, minimal concentrations of MK-4 are found in plasma and faeces, but MK-4 is rarely produced by bacteria (Collins and Jones 1981; Fernandez and Collins 1987).
Tissue distribution and uptake
VK vitamers are distributed throughout the body, with uptake occurring in the liver, heart, pancreas, lungs, kidneys, brain, and bone (Thijssen and Drittij-Reijnders 1996), with the highest concentrations occurring in adipose tissue (Shea et al. 2010). However, the distribution of the different vitamers is not uniform throughout the body. The liver contains high concentrations of VK and is the primary site for synthesis of VKD coagulation factors (Shearer 1995; Shearer and Newman 2008). It is well documented in humans and rats that PK preferentially accumulates in the liver (McCann and Ames 2009). There is now good evidence that the hepatic turnover of long-chain MK-n is very much slower than that of PK in both rats and humans, resulting in much greater hepatic stores of MK-n (in particular MK-7 to MK-13) than PK (Shearer and Newman 2008). It has been suggested that MK-n may be of greater importance than PK in alleviating risk of age-related diseases such as osteoporosis (Inoue et al. 2009; Pizzorno 2011). This may be attributable to different absorption and transport pathways as MK-n is readily transported to extra-hepatic tissues in humans, whereas most PK is excreted (Falcone et al. 2011).
The uptake of VK by bone (Newman et al. 2002) was discovered with the isolation of VKDPs from the bone matrix (Shearer et al. 2012). However, the exact molecular basis of VK transport and uptake by extra-hepatic tissues has not been as extensively studied. The primary delivery vesicles of VK into bone are believed to be chylomicrons, with low-density lipoproteins (LDL) being the predominate lipoprotein transporters. This was established by Newman et al. (2002) who found that the most efficient uptake of PK into osteoblasts was achieved via LDL. While TRL are believed to be the primary transporters, MK-n has been detected in both LDL and high-density lipoproteins (HDL), suggesting differential transport and uptake routes, perhaps in different tissues (Shea and Booth 2007). This is supported by known differences in human tissue specificity among different isomers, with MK-n being the major form in circulation and bone, and PK being stored at higher concentrations in the liver (Shea and Booth 2007). LDL are the primary transporters of PK to osteoblasts; however, uptake can also occur via TRL and HDLs (Shearer et al. 2012).
There has been much recent interest in the role that VK plays in the brain because of a possible role in cognition (Ferland 2012; Alisi et al. 2019). It has an important role in synthesis of sphingolipids that contribute to both the structural integrity and functionality of brain cell membranes. The VKDP Gas 6 has been located in brain cells and participates in a range of functions, including cell proliferation and differentiation, myelination, mitogenesis and chemotaxis (Ferland 2012). Studies by Thijssen et al. (1996) and Ellis et al. (2022) showed that MK-4 is the only VK vitamer found in the brain, suggesting that only MD, the smallest vitamer, can cross the blood–brain barrier and is then converted to MK-4 (Shearer 2022).
Another tissue that permits limited VK translocation is the placenta. In humans, PK concentrations in umbilical cord blood are extremely low, implying that placental transfer of VK is poor (Shearer et al. 2012). Oral supplementation of VK before delivery in humans significantly increases maternal plasma and umbilical-cord plasma concentrations of PK (Kazzi et al. 1990). In humans, it appears that VK traverses the placenta poorly; as a result, infants who do not receive a prophylactic dose of VK at birth, either orally or by intramuscular injection, are at risk of the haemorrhagic disease, VK-deficiency bleeding (VKDB) (Greer et al. 1997; Shearer 2009). Other contributing factors to the incidence of VKDB are low concentrations of VK in human breast milk at birth (Dror and Allen 2018). Australia has one of the lowest instances of VKDB in the developed world (Zurynski et al. 2020).
The VK cycle and γ-carboxylation
The VK cycle is depicted in Fig. 2. An appreciation of the VK cycle is crucial to understanding the complexity of VK metabolism, and the roles it plays in many functions. VK functions as a cofactor for the enzyme glutamyl carboxylase to convert glutamyl residues to gamma-carboxy-glutamic acid (Gla) residues in proteins. These Gla residues are required for the calcium-mediated action of the proteins and are the location of specific calcium-binding sites. These activated VKDPs are found in many tissues throughout the body and as discussed below are involved in many important physiological processes in addition to blood coagulation.
The VK cycle. During vitamin K-dependent carboxylation, glutamate (Glu) is converted to gamma-carboxyglutamate (Gla) by gamma-glutamyl carboxylase (GGCX), by using a reduced form of VK (KH2), carbon dioxide, and oxygen as cofactors. KH2 is oxidised to VK epoxide (KO). KO is reduced to VK-by-VK epoxide reductase (VKOR). The reduction of VK to KH2 is performed by VKOR and an as-yet-unidentified VKR (adapted from Tie and Stafford 2016).
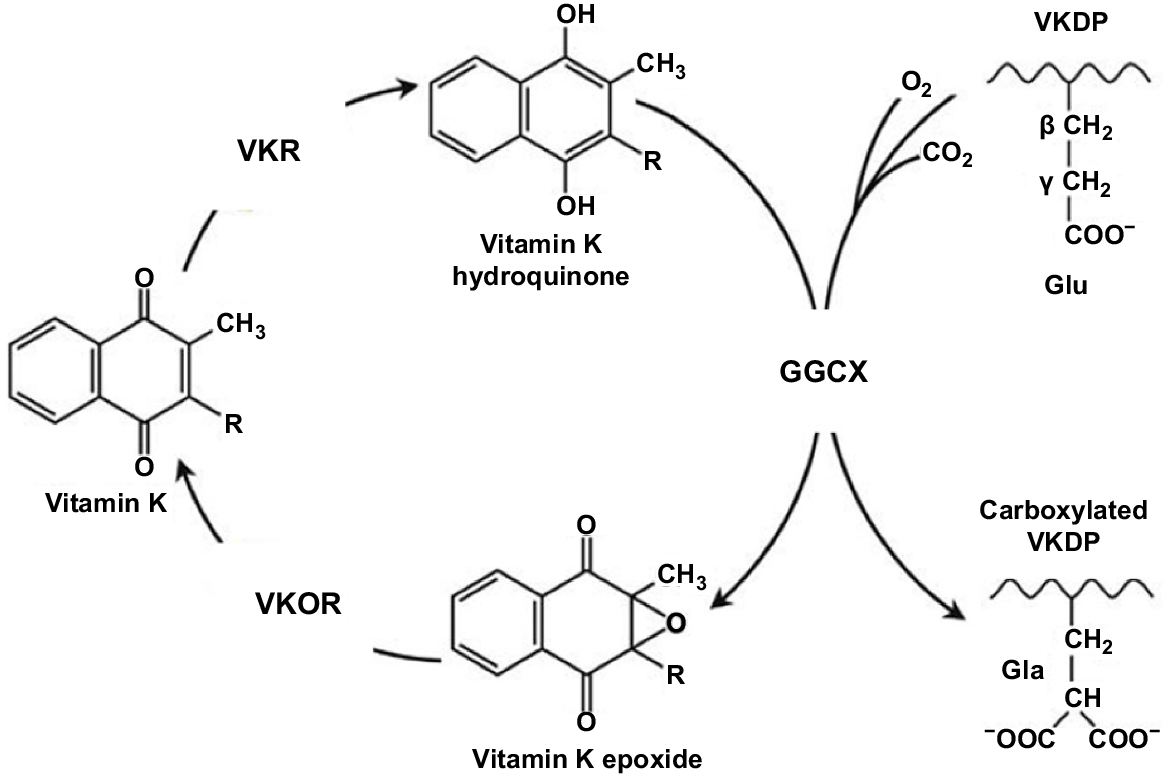
The VK cycle facilitates the post-translational conversion of glutamate (Glu) to gamma-carboxyglutamate (Gla) by gamma-glutamyl carboxylase (GGCX) by using a reduced form of VK (KH2), as was well depicted in Stafford (2005). In so doing, VK undergoes oxidation and reduction in three stages and is regenerated. In the cycle, KH2 is oxidised to VK epoxide (KO), and KO is reduced to VK-by-VK epoxide reductase (VKOR; Li et al. 2004). The reduction of VK to KH2 is performed primarily by this enzyme (Hammed et al. 2013). The oxidation of KH2 to VK 2,3-epoxide (KO) is a two-step reduction process, enabling KO to then be converted back to KH2 via the enzymes VKOR and VK reductase (VKR; Tie et al. 2016). Two primary enzymes involved in the carboxylation process are GGCX and VKOR (Tie et al. 2016). While less is understood about the enzyme VKR, there is no doubt of its importance in the VK cycle, but its exact identity remains unknown (Caspers et al. 2015). In summary, the VK cycle, which is coupled to the carboxylation of proteins, facilitates this process, and allows for the recycling of reduced VK. In the presence of an anticoagulant, the recycling of VK is blocked by the inhibition of VKOR and VKR (Shearer and Newman 2008).
The carboxylation of VKDPs is a vital post-translational modification (PTM) process, and as shown in Fig. 3, coverts specific glutamate residues (Glu) to γ-carboxyglutamate (Gla) residues in VKDPs (Berkner 2005; Lacombe and Ferron 2015). It is essential for the biological function of numerous VKDPs, including the clotting factors (Wallin and Martin 1985). The Gla residues are required for the calcium-mediated action of the proteins and are the location of specific calcium-binding sites. Gla residues adopt a calcium-dependent conformation that promotes clotting factors binding to a membrane surface (Tie and Stafford 2016). The resulting Gla residues show an increased affinity for calcium ions (Malashkevich et al. 2013). Binding then elicits a conformational change, rendering the VKDPs biologically active (Shearer and Newman 2014). The extent to which these proteins become carboxylated influences their activity. This role of the vitamin defines its significance for important physiological processes in addition to blood coagulation, including bone metabolism, energy metabolism, insulin resistance, inflammation, spermatogenesis, apoptosis, and immunity. These latter processes are usually overlooked as the role of many of the VKDPs has not been fully elucidated. Most of the research that has examined these aspects of VK metabolism has been conducted with cell lines (Vermeer et al. 2017).
Assessment of VK status
Traditionally, assessment of VK status has relied on the measurement of blood coagulation, for which VK is an essential cofactor and is therefore indicative of an adequate VK status (Suttie and Booth 2011). In humans, circulating concentrations of VK have been found to have large inter- and intra-individual variation (Shea et al. 2012). Such findings make determining species and gender-specific dietary requirements challenging (Shea and Booth 2016).
With the various challenges presented in determining VK status by using blood clotting time or circulating concentrations of VK (Górska 2019), there is an urgent need to explore other possible measures. The measurement of osteocalcin (OC) has been proposed to be a more suitable measure of subclinical VK deficiency (Gundberg et al. 1998). It is believed that VK is preferentially taken-up by the liver to facilitate optimal blood coagulation (Booth 2009). The remaining VK is then available to facilitate carboxylation of extra-hepatic VKDPs such as OC (McCann and Ames 2009). It is for this reason that inadequate VK intake, or VK intake that meets only the requirement for coagulation, may result in subclinical VK deficiency and induction of underlying diseases such as osteoporosis (Misra et al. 2013). For instance, although OC is not specifically regulated by VK, it influences the extent to which OC becomes carboxylated (Gundberg et al. 2012) and therefore functional. Recent research has suggested the degree of carboxylation of VKDPs, especially of circulating OC, is a more sensitive indicator of VK status than is coagulation (Fusaro et al. 2017b).
To reduce the risk of subclinical VK deficiency and allow determination VK requirements, the analytical challenges presented by VK need to be overcome. It is unlikely that a single biomarker will suffice for VK as different VKDPs are implicated in different diseases and the biomarker will depend on the outcome studied (Shea and Booth 2016). It is beyond the scope of this review to describe the complexity of VK analysis, and the interested reader is referred to Górska (2019) and Card et al. (2020), who have comprehensively reviewed this topic.
VK metabolism and horse nutrition
As depicted in Table 2, there have been only a few studies to investigate VK metabolism in horses. Duello and Matschiner (1970) were the first to identify and extract PK from horse liver. The recovery of PK as the only identified form of VK in horse liver adds support to the view that liver contains mainly those forms of VK provided in the diet of the animal. In a study of different tissues from horses, cattle, pigs, guinea pigs, chickens, rats, and mice, Hirauchi et al. (1989) were able show a range of vitamers in liver, kidneys, heart, spleen, and muscle. The results for all horse tissues and hepatic values for the other species are shown in Table 3. All species had PK and MK-4 in all tissues, but different longer-chain MK-n in hepatic and extra-hepatic tissues. In bovine livers, all MK-n from MK-6 to MK-14 were present as they were in the pig, but at much lower concentrations. Although the guinea pig and horse are hindgut fermenters, like in the pig, their tissues did not contain the very long-chain MK-n. This may reflect differences in either intestinal bacterial MK-n synthesis or absorption. In hindgut fermenters, both activities will occur in the hindgut where the bioavailability of MK-n is likely to be low, as discussed below. In contrast, in foregut fermenters, such as cattle, microbial synthesis of MK-n occurs in the rumen and the synthesised vitamers are subjected to digestion and absorption in the small intestine, the major site of VK absorption (Shearer et al. 2012). These differences in digestive physiology may explain the much higher concentrations of MK-n in bovine tissues.
Study | Cohort/samples | Response | Form of VK and intake | Outcome | |
---|---|---|---|---|---|
Duello and Matschiner (1970) – Tissue analysis of PK | Horse liver. | Tissue PK. | No VK administered. | Identification of PK in horse liver. | |
Rebhun et al. (1984) – Effect of Menadione | n = six horses. | Plasma menadione. | Parenteral administration of MD (intake unknown). | MD induced renal toxicity in five of the six horses. | |
Hirauchi et al. (1989) – Tissue analysis of VK | Horse tissue samples. | PK, MK-4, MK-5-MK-14. | No VK administered. | Identification of PK in horse tissues. | |
Siciliano et al. (2000a) – Effect of exercise on VK status | n = 12 horses (QH, 18–24 months). | Total OC (HAP-binding capacity), serum PK. | Both groups were allowed free access to brome grass hay (273 mg of PK/100 g). Additionally, the exercise group received 40 mg of PK/100 g on the days the animals were exercised. | No significant correlation was present among the serum measures, OC and hydroxyapatite-binding capacity of serum OC, and the bone measures. | |
Siciliano et al. (2000b) – Effect of age at weaning on VK status. | n = 15 horses (QH and TB foals). | Total OC (HAP-binding capacity), serum PK. | No VK administered. | In conclusion, VK status increased, and serum OC decreased with age. In addition, VK status tended to increase at a greater rate in foals weaned early than in those weaned late. | |
Piccione et al. (2005) – Diurnal monitoring of VK | n = five horses (TB mares, 8 years). | Serum PK. | No VK administered. | Serum VK concentration displayed an acrophase, which occurred during the evening. | |
Biffin et al. (2008a) – Effect of VK on bone density and OCD | n = 69 horses radiographed (TB yearlings). (n = 14 monitored from the group with visible lesions). | Total OC %. | In total, 14 horses from the 69 with visible lesions on radiography were monitored for 2 months. A quantity of 3 mg PK in proprietary soluble form administered to three of these horses for 9 days. | In those horses administered PK, % carboxylated OC increased but was not significant. Likewise, in the 14 horses monitored on pasture for 2 months and then stabled for 2 weeks, no significant difference was detected. | |
Inoue et al. (2009) – Circulating VK status and absorption | TB (young horses and mares). (n = unknown). | Plasma vitamin PK, MK-4, and MK-7. | A quantity of 9 mg per day of supplemental menadione. | Plasma MK-7 concentration was extremely low in horses, the nutritional importance of MKn, which is synthesised in the intestinal tract, may not be significant in horses. MD increased MK-4 concentration. | |
Biffin et al. (2010) – Effect of VK supplementation on bone density | n = 26 horses (TB, 2 years). | Growth, bone density, plasma PK. | A quantity of 7 mg QAQ per day, and the control group a blank powder. | Horses may receive suboptimal intakes of VK, and further research is required to determine equine requirements in different management systems and the efficacy of different forms of the vitamin. | |
Terachi et al. (2011) – Circulating VK status and absorption | n = 16 horses (eight mares and eight geldings). | PK, MK-4, menadione. | Each group was given PK, MK-4, or MD at 58 μmol per day, or no VK supplement for 7 days. | The PK concentration increased (P < 0.001) after feeding in the PK group, but no changes in the plasma PK concentration were observed after feeding in the other groups. Plasma MK-4 concentration was greater (P < 0.001) in the MD group. |
Species | Tissue | PK | MK-4 | MK-6 | MK-7 | MK-8 | MK-9 | MK-10 | MK-11 | MK-12 | MK-13 | MK-14 | |
---|---|---|---|---|---|---|---|---|---|---|---|---|---|
Cattle | Liver | 18.4 | 8.2 | 24.5 | 181.8 | 48.4 | 15.3 | 66.3 | 136.4 | 215.6 | 436.9 | 22.6 | |
Pig | Liver | 0.5 | 5.9 | 0.4 | 6.1 | 5.6 | 2.6 | 5.1 | 7.2 | 4.4 | 4.9 | 0.3 | |
Guinea pig | Liver | 39.3 | 17.0 | 7.8 | 5.1 | 1.5 | 3.0 | 13.3 | 2.4 | 0.2 | ND | ND | |
Chicken | Liver | 25.2 | 39.6 | 0.3 | ND | 0.9 | 0.4 | 0.3 | ND | ND | ND | ND | |
Rat | Liver | 4.7 | 7.7 | 26.8 | ND | 1.5 | 1.0 | 4.8 | ND | ND | ND | ND | |
Mice | Liver | 1.2 | 2.6 | 5.4 | ND | ND | ND | 1.2 | 0.5 | ND | ND | ND | |
Horse | Liver | 76.7 | 2.1 | 1.0 | 2.3 | 1.2 | 1.4 | 0.5 | ND | ND | ND | ND | |
Kidneys | 5.8 | 1.5 | ND | ND | ND | ND | 1.6 | ND | ND | ND | ND | ||
Heart | 14.5 | 0.4 | 2.8 | 0.9 | ND | ND | 0.9 | 0.9 | 1.3 | 4.1 | ND | ||
Spleen | 23.7 | 0.7 | 1.1 | 0.9 | 0.8 | 0.4 | ND | ND | ND | ND | – | ||
Muscle | 14.9 | 2.0 | 0.2 | 2.3 | – | – | – | – | – | – | – |
Note: MK-5 was analysed but not detected.
The results of Hirauchi et al. (1989) also indicated that intestinally synthesised MK-n does contribute to the VK economy of the host animal; however, is this significant in horses? Inoue et al. (2009) suggested that MK-n may not be nutritionally significant in horses as they found significantly lower plasma concentrations of bacterial-synthesised MK-7 than those of PK. This supports the findings of Hirauchi et al. (1989) who found that PK concentrations were much higher in almost all horse tissues than were values for MK-n (Table 3). It should be noted that bile is required for VK absorption, as occurs with all lipids (Yeum et al. 2017), but both the horse and rat do not store bile in a gall bladder but appear to secrete it continually, which is different from other species (Cunningham and Klein 2007). Moreover, bile salts and acids are digested in the small intestine and are unlikely to be present to facilitate MK-n absorption in the hindgut (Cunningham and Klein 2007). Further, the horse, unlike rodents, rarely practices coprophagy (Stevens and Hume 1995) and this practice allows rodents access to microbially synthesised MK-n (Harshman et al. 2017). These results and observations suggest that the source, absorption, and distribution of VK in horses may be different from those in humans and rodents, and reflect experimental protocols used in the respective studies, and this requires further research. Rodent studies also suggest that there may be sex, age, and strain differences in the metabolism of VK (Harshman et al. 2016), but these factors have not been examined in horses.
Terachi et al. (2011) evaluated plasma VK concentrations in horses, after dosing (58 μmol/day) with PK, MK-4 or MD for 7 days. Plasma PK concentrations were highest following PK administration and MK-4 concentration was highest in the MD-administered group. The authors concluded that as MK-4 has been suggested to be the most potent form of VK to assist bone metabolism in rodents, their results indicated that MD is a good source of VK for bone health in horses as it was the only vitamer that increased the plasma concentration of MK-4. This finding is in accord with previous research conducted by Inoue et al. (2009), and further highlights the pivotal role of MK-4 in VK and bone metabolism, by inhibiting osteoclasts and promoting osteoblast formation (Shearer 2022).
Equine requirements
The dietary VK requirement of most species is not well defined and depends, to a large extent, on the sensitivity of the clotting-based assay used, the route of vitamin administration, and the form of the vitamin used (Zwakenberg et al. 2017). None of the major reference authorities on the nutrition of the horse in the United States (National Research Council (NRC) 2007), France (Martin-Rosset 2015), or Germany (GEH 2013) lists requirements for VK. This reflects a lack of data on which to predict requirements. In many respects, VK has been neglected in equine nutrition research, due, in part, to very few instances of equine bleeding disorders being reported. There is also a widely held belief that horses receive an adequate intake of VK from grass and forage, along with microbial synthesis in the gut. Nevertheless, McDowell (2000) stated that equine VK status is met by microbial synthesis and yet there is no or very weak evidence to support this conclusion, as detailed in this review. Like the horse, pigs and rabbits are hindgut fermenters and it is recommended that these two species receive a VK dietary supplement of 0.5 mg/kg and 2 mg/kg (McDowell 2000).
Perhaps the horse also requires dietary supplementation with VK. Supplements of VK are added to horse feeds and may indicate the level of VK required. However, current feeding-rate recommendations of commercially available equine VK supplements and feeds that contain a VK premix vary. According to labels, 7 mg/500 kg bodyweight per day is recommended for Bonafide® (Quinaquinone®) for mature horses stabled or without access to pasture. In Kentucky Equine Research’s commercial pre-mixed horse feeds (KER All Phase), 22 mg/kg of MD is added, with a recommended feeding rate of 0.75–1 kg/500 kg bodyweight per day, depending on activity. However, it is difficult to compare these commercial preparations, as Quinaquinone® is based on a soluble form of PK, while the KER product is MD. There is evidence that Quinaquinone® is more rapidly and completely absorbed than is MD (Skinner et al. 2014).
The other question that needs to be addressed when considering VK requirements is whether blood-clotting assays are appropriate for determining VK status; however, as discussed in this review, there is a growing body of evidence to indicate that other biomarkers are required. This becomes very relevant with the identification of several Gla-proteins now known to be reliant on VK for bone mineralisation. Thus, are VK requirements being met to support the optimal equine bone health when only blood clotting is considered? While current evidence in human studies suggests a supportive role (Bonjour et al. 2009), often the doses used are very high pharmacological doses and therefore not reflective of nutritional uptake. Further research is needed to ascertain requirements and the conditions in which supplementation may be warranted.
Equine VK deficiency
VK deficiency is most commonly defined by an impairment of blood coagulation resulting from decreased prothrombin activity (Tie et al. 2016). Moreover, unlike other fat-soluble vitamins, VK reserves are rapidly depleted from the body (Shearer and Newman 2014) but the salvage of VK by the VK cycle reduces the possibility of reduced blood-clotting capacity. While a deficiency of VK in adult horses is believed to be improbable and has not been described, the VK status of young growing horses may be deficient due to decreased pasture intake and limited microbial synthesis (Siciliano et al. 2000a), but little research on the VK status of young, growing horses is available. Likewise, whether there are increased requirements associated with gastrointestinal issues such as fat malabsorption or liver disease is currently not known (Harris et al. 2005). Nevertheless, as discussed below, it is the neonatal foal that is more likely to experience VK deficiency.
The overt clinical sign of a VK deficiency across all species is decreased blood coagulation due to the undercarboxylation of proteins associated with blood coagulation. However, there is increasing recognition of subclinical VK deficiency arising from undercarboxylation of Gla-proteins associated with other functions, such as under-carboxylated osteocalcin (unOC). A subclinical deficiency of this protein has been implicated in the pathogenesis of bone-related diseases such as osteoporosis in poultry and humans (Zhang et al. 2003; Lanham-New 2008). These findings suggest that higher intakes of VK are required for the optimum γ-carboxylation of proteins in extra-hepatic tissues than of hepatic VKDPs (McCann and Ames 2009). Findings by Biffin et al. (2008a) suggest a possible association between inadequate VK intake and development of bone diseases in young horses. This observation warrants further investigation as the consequence of under-carboxylation of extra-hepatic VKDPs is not fully understood (Rishavy and Berkner 2012).
Anticoagulant-induced VK deficiency
There are equine reports of overt VK deficiency induced by anticoagulants, most noticeably warfarin (Vrins et al. 1983). In the presence of warfarin, the pattern of PK excretion changes to a greater proportion in urine (40%) than in bile (20%) (Shearer and Newman 2008). As detailed in Table 4 there have been several reports in which horses have experienced bleeding episodes following accidental ingestion of a rodenticide containing warfarin or related compounds. Deaths of racehorses following exercise (Carvallo et al. 2015) and abortion in an Arabian mare (Zakian et al. 2019) have also been reported after exposure to rodenticides. The effects of warfarin as an anticoagulant have been studied in ponies (Scott et al. 1978, 1979, 1980), and horses (Byars et al. 1986) where it was demonstrated that PK, an antidote to warfarin toxicosis, reversed anticoagulation and controlled haemorrhage. Vermeer and Ulrich (1982) looked at the effects of anticoagulation therapy on carboxylase in the liver, spleen, and kidney of horses and like Scott et al. (1980), they found that in all tissues carboxylase was inhibited following anticoagulation therapy.
Study | Cohort/samples | Response | Form of vitamin K and intake | Outcome | |
---|---|---|---|---|---|
Scott et al. (1978, 1979, 1980) | n = four horses. | Prothrombin time (PT). | Anticoagulant (Warfarin) administered. | Haemorrhage, PK administered intravenously (IV) to reverse anticoagulation and control haemorrhage. | |
Vermeer and Ulrich (1982) – Effect of warfarin | n = eight horses (Four warfarin treated, four controls). | Coagulation time, growth, tissue PK. | No VK administered, 1 g anticoagulant administered. | Synthesis of extrahepatic VKDPs is reduced in parallel with the synthesis of the Coagulation factors II, VII, IX and 9. | |
Byars et al. (1986) – Therapeutic response of PK | n = four horses. | Coagulation time, Serum PK. | PK (100 mg per dose), 300 mg of PK IV, and two horses were given 300 mg of PK subcutaneously. | After discontinuation of warfarin administration, there was a prothrombin time (PT) reversal time of approximately 5 days from the last dose of warfarin. The 100 mg dose of PK shortened the therapeutic response time to 12 h and the PT reversal time to 24 h. | |
Maxie et al. (1992) | n = six SB racehorses. | Coagulation factors. | MD administered prior to racing to control EIPH. | MD toxicity. | |
Edens et al. (1993) | SB colt (n = one). | Coagulation factors. | No VK administered. | Decreased activity of protein C – hyper coagulation and renal disease. | |
Rathgeber et al. (2001) | Foal (n = one). | Coagulation factors. | No VK administered. | Foal diagnosed with Von Willebrand disease. Normal platelet function was compromised. | |
McGorum et al. (2009) | SB colt (n = one). | Coagulation factors. | No VK administered. | Although VK administration resolved colt A’s VKDB, and coagulation indices were normal 5 days after cessation of VK administration, colt A was euthanised at 9 weeks of age because of prolonged failure to thrive. | |
Skinner et al. (2014) – Absorption of vitamin K | n = 12 horses (geldings). | Plasma was analysed for PK, MK-4 and menadione. | There were six treatments (200 mg); control, PK, MK-4, MD and KQ (Quinaquanone™, a soluble form of PK and MKn in the ratio of 10:1). | The soluble form of the vitamin, KQ, was the most efficiently absorbed. There is no specific conversion of PK to MD or MD to MK-4 in plasma. | |
Skinner et al. (2015) – Hindgut absorption of K vitamers | n = four horses (geldings). | Plasma PK, MK-4 and menadione. | 200 mg oral of either (1) KQ (2) KQ coated with 1.5% calcium alginate or (3) PK oil. | There was no further uptake of PK from the spheres in the hind gut, suggesting that the hind gut does not facilitate VK absorption in the horse. | |
Fischer et al. (2017) – Maternal transfer of vitamin K | n = 18 horses (mares and foals). | Plasma and milk sampled for PK and menadione. | Treatment groups (n = six): no VK supplementation (carrier paste only); MD (15 mg); vitamin KQ a soluble form of PK and MKn (15 mg). | PK concentrations in the foal pre-suck plasma and umbilical cord samples were below the detection limit of the analysis (<0.01 ng/mL) across all groups. |
Toxicity
VK is considered to have low toxicity, with the National Research Council (NRC) (1987, 2007) reporting oral toxicity at greater than 1000 times the recommended dietary allowance. MD, which is used widely in vitamin premixes for animal feeds, has been shown to induce kidney toxicity in horses with intravenous or intramuscular use (Rebhun et al. 1984) or in very high oral doses. Rebhun et al. (1984) demonstrated renal tubular nephrosis in horses following administration of MD, at the manufacturer’s dosage guidelines, emphasising the toxicity of this vitamer to horses. Six Standardbred racehorses experienced significant MD toxicity following intravenous injection of the vitamer with 200 mg (50 mg/mL mixed with 7% N-butyl alcohol and aminocaproic acid) prior to racing to counteract exercise-induced pulmonary haemorrhage or epistaxis (Maxie et al. 1992). Within 48 h of administration, all horses exhibited significant signs of toxicity including weakness, depression, muscle stiffness and colic, and were markedly azotemic. Mohamed et al. (2017) observed similar clinical signs within 24 h of draught horses being orally dosed with MD; however, the dose used (10 mg/kg bodyweight) was much higher than the recommended dosage of 0.01–0.02 mg/kg bodyweight. The prognosis for horses with MD toxicity is generally poor due to nephrosis and probable renal failure. In horses, MD should be administered only to treat VK-deficiency coagulopathies as occurs with warfarin, and not to treat other haemorrhagic disorders.
VK status of foals
As noted above, at birth the human neonate can suffer from severe VK deficiency haemorrhagic disease or VKDB (Greer et al. 1997; Shearer 2009; Zurynski et al. 2020). This condition can result from subclinical maternal deficiency, poor placental transfer, and low milk concentrations of VK (Shearer 2009). It has also been demonstrated that supplementation of VK during the last trimester of pregnancy results in improvements in the carboxylation of OC both in mothers and the newborn, on the basis of measurements of umbilical cord blood (Vermeer 2012).
With respect to coagulation, foals appear to have sufficient VK at birth, as there has been only one report of VKDB in foals. Nevertheless, is the VK status of foals adequate for optimum functioning of extrahepatic VKDPs? In the reported incidence of VKDB in a 4-week-old Standardbred colt, there was no history of anticoagulant ingestion (McGorum et al. 2009). The administration of VK successfully stopped the bleeding and prothrombin time returned to normal within 18 h. This case suggests that in horses, as has been shown in humans and rodents, that placental transfer of VK is limited and VK content of milk is low. We have been able to confirm that trans-placental transfer of VK in the mare is low, as negligible concentrations of VK were found in both umbilical cord and foal plasma at birth (Fischer et al. 2017).
An important aspect of improving foal VK status and reducing the risk of VKDB is the provision of VK to the neonate by milk. Most research investigating the composition and quality of mares’ milk has been conducted in Western Europe (Krešimir et al. 2011). Mare’s milk has a composition different from that in most domestic species, but is relatively similar in composition to breast milk, and is frequently utilised as a substitute for infant’s intolerant to cow’s milk in some countries (Doreau and Boulot 1989; Pieszka et al. 2016; Barreto et al. 2019). Colostrum is a more concentrated source of vitamins for foals than is milk produced throughout lactation (Csapó et al. 1995), as shown in Table 5. Recently, Skinner (2020) demonstrated that VK supplementation of the mare increases the concentration of VK in milk, especially in the first few days following parturition. This suggests that colostrum is a critical source of VK for neonatal foals in the period before their gut is sufficiently developed for them to obtain the vitamin from pasture or intestinal microbial synthesis. Furthermore, Siciliano et al. (2000a) identified that VK status tended to increase with age, particularly in foals weaned early as opposed to those weaned late, possibly suggesting increased uptake of VK from pasture.
Postpartum days | ||||
---|---|---|---|---|
Vitamin | 0–0.5 | 8–45 | 5–270 | |
A | 0.8800 | 0.3400 | 0.3520 | |
D3 | 0.0054 | 0.0032 | 0.0029 | |
E | 1.3420 | 1.1280 | 1.1350 | |
PK | 0.0430 | 0.0290 | 0.0320 | |
C | 23.800 | 17.200 | 15.320 |
Sources and bioavailability of VK
Horses receive VK from three sources, namely from forage and other feedstuffs, intestinal bacterial synthesis, and feed additives. The relative importance of these sources for meeting the horse’s requirements will change depending on whether the horse is stabled or on pasture. Most research on VK content of food has been surveys of the human diet (Bolton-Smith et al. 2000; Harshman et al. 2014; Vermeer et al. 2018), which show that VK is provided by leafy green vegetables, vegetable oils, natto, cheese and other animal products. Vegetable oils, especially from soybeans and rapeseed have high concentrations (1500 μg/kg) of PK, which is susceptible to heating and destroyed by light (Ferland and Sadowski 1992). There is no consensus on the importance of MK-n to the vitamin economy of humans or animal hosts (McCann et al. 2019) but it is well documented that PK is the predominate form in herbivores and MK-n in omnivores and carnivores (Thijssen et al. 1996). However, little attention has been given to VK concentrations of animal feedstuffs, because of the lack of bleeding conditions in livestock and the availability of MD as an animal feed additive.
VK in grains and hay
The concentration of the vitamin in cereal grains is very low (0.2–0.4 mg/kg DM) but much higher (3–22 mg/kg DM) in forages (McDowell 2000). The VK status of pastures also varies considerably (Booth 2012) and is largely dependent on environmental factors such as the season, weather, and time of day. Stage of growth and ultraviolet radiation will influence VK status of pasture, with freshly growing green pasture containing the highest concentration of PK. Likewise, the VK content of hay varies and is also sensitive to drying and exposure to ultraviolet radiation (Erkkilä et al. 2004). Biffin et al. (2008b) reported that the VK content of forage was rapidly reduced as it dried during hay production. Therefore, the VK content of hay can be minimal (Biffin et al. 2008b). This has implications for horses with restricted access to pasture, where the VK status of their diet may be insufficient to ensure optimal functioning of extra hepatic VKDPs. Biffin et al. (2008a) concluded that stabled horses fed hay- and a grain-based complete feed should be supplemented with VK.
VK in chloroplasts and bacteria
The commonly held belief is that the requirements for VK can be met from the concentrations of the vitamin found in the diet, and the amount synthesised by gut microbes. However, there is human and rodent evidence that these sources of the VK are not always available (Shearer et al. 2012). VK availability is a function of digestion, absorption, and utilisation. For most nutrients, digestion and absorption are the rate-limiting steps in availability and this appears to be the case for VK, as release from plant or bacterial cells is problematic. PK is tightly bound within the membrane of chloroplasts in plants where it plays a critical role in photosynthesis and in the formation of disulfide bridges in proteins (van Oostende et al. 2011). Likewise, MK-n are tightly bound to cytoplasmic membranes of bacteria where they mediate electron transfer reactions and provide protection from lipid oxidation (Bentley and Meganathan 1982). Studies in humans have shown that PK in leafy vegetables has low availability when compared with PK in vegetable oils. Surprisingly, MK-n in animal products (fermentation products and dairy products, including cheese) were much more available than was the PK in vegetables (Gijsbers et al. 1996; Garber et al. 1999; Schurgers and Vermeer 2000). The same relative VK bioavailability (release from food matrix) was also found in in vitro studies (Jensen et al. 2022). These results suggest that PK in plant cells is resistant to digestion, a situation also likely to occur in the horse, while the MK-n from animal products is released by digestion. Perhaps intestinally synthesised MK-n are not released into the gut to the same extent, given the lack of digestive enzymes in the hindgut. Moreover, the preparation and processing of animal products for human consumption may increase PK digestibility. Animal-based products are unlikely to occur in equine diets.
In a free form or after release from plant cells, PK is readily absorbed in the small intestine (Shearer and Newman 2008), and this has been shown in the horse (Skinner et al. 2014); however, this is less clear in the large intestine, where there is evidence that PK is poorly absorbed (Skinner et al. 2015). For absorption, bile salts are required for micelle formation but are lacking in the hindgut and this may partly explain the lack of PK absorption. Moreover, the mechanisms by which MK-n are absorbed from the colon in humans are unknown (Karl et al. 2017). For these reasons and as it has many forms, MK-n, if it is released from bacterial cells in the caecum and colon of the horse, may not be absorbed; this questions the contribution of MK-n to VK status in horses.
Synthetic VK
To eliminate the possibility of VK deficiency, MD, the synthetic form of VK, is routinely added to animal diets, usually as a sodium bisulphide complex (MSBC), which is soluble and has biological activity at least equal to that of PK (Scott et al. 1976). The European Food Safety Authority (EFSA) reviewed the available evidence and considered MD safe for all animals (EFSA Panel on Additives and Products or Substances used in Animal Feed (FEEDAP) 2014), but did not consider renal toxicity for horses as described above. However, the EFSA Panel on Additives and Products or Substances used in Animal Feed (FEEDAP) et al. (2021) did conclude that synthetic PK is an effective source of VK for horses. The cost of producing the natural forms of VK (PK and MK-n) has precluded their use in feed supplements; it is 100 times more expensive than is MSBC. Phytonadione is PK that is chemically synthesised from MD and has been used in human pharmaceuticals and food supplements since the middle of last century. However, as new roles for VK emerge, there is a consumer-driven push to produce ‘natural’ VK by using biotechnology rather than chemical synthesis (Tarento et al. 2019; Kang et al. 2022). There are many biomanufacturing challenges when scaling the production of a new product to market (Speight et al. 2022) that will need to be overcome in this new era of VK supplementation.
Interactions with other nutrients
Although the availability of a nutrient is usually considered as a function of an individual feedstuff, many factors affect availability, and it has been shown that total diet composition can significantly affect free PK absorption (Jones et al. 2009). This could reflect lipid content or the concentrations of other fat-soluble vitamins in the diet. Moreover, diet can influence the concentrations of different VK vitamers in the gut indirectly through modulation of the gut microflora (Ellis et al. 2021) and this has also been shown when animals are medicated with antibiotics (Quinn et al. 2020). Interactions of the fat-soluble vitamins A, D, E and K have been demonstrated in rodents (Traber 2008) and chickens (Abawi and Sullivan 1989; Guo et al. 2021), especially in relation to intestinal absorption due to competition for similar transport and uptake mechanisms (Goncalves et al. 2014). Large doses of vitamin A have been shown to antagonise VK uptake in humans. Furthermore, human studies have demonstrated that increased dietary vitamin E concentrations will reduce VK absorption (Booth et al. 2004). It has subsequently been shown that high vitamin E concentrations also decrease post-absorptive metabolism of VK that is accompanied by decreased MK-4 tissue concentrations (Tovar et al. 2006). These interactions have not been examined in horses and question the arbitrary micronutrient supplementation practiced in some racehorse stables (Southwood et al. 1993). The interaction between VK and the antioxidant glutathione also warrants further investigation in the horse, with in vitro studies in rats suggesting that VK inhibits glutathione depletion (Elkattawy et al. 2022).
Antagonism between vitamins E and K has been known for many years (Traber 2008). Data from other animals suggest that coagulopathy and impaired bone mineralisation can occur when diets with excessive vitamin E (above the upper safe limit of 1000 mg/kg DM; National Research Council (NRC) 1987) are consumed. However, in horses no signs indicating vitamin E toxicosis have yet been reported even at the high intakes recommended for treatment of equine degenerative myeloencephalopathy (EDM) and other conditions. Interest of late has switched to vitamins D and K, as both have roles in bone health and osteoporosis prevention (Shearer 1997; Fleming 2008; Lanham-New 2008). Both vitamins regulate bone mineralisation, and this is well defined for vitamin D through modulation of calcium and phosphorus homeostasis; a complex area of mineral metabolism (Li et al. 2017). The role for VK is less clear but the vitamin mediates hydroxyapatite formation through carboxylation of proteins required for this process (Shearer and Okano 2018). An important protein in this regard is OC and vitamin D regulates its synthesis (Shearer 1997). Moreover, vitamin D stimulates intestinal calcium absorption, while VK stimulates renal calcium resorption (Iwamoto et al. 2003), both important aspects of calcium homeostasis. Delineation of the interdependence of calcium, phosphorus, vitamin D and VK in equine nutrition is an area worthy of detailed research.
Emerging roles of VK for horses
In this section, implications for equine metabolism and nutrition of some of the roles for VK beyond coagulation are discussed.
VK and bone metabolism
With the isolation of VKDPs located in bone, especially OC, there has been much interest in delineating the role of VK in skeletal health, especially osteoporosis and osteoarthritis (Mott et al. 2019; Su et al. 2019). All the research into the relationship of VK to bone accretion and turnover has centred on these human maladies that usually occur in older adults; similar conditions have not been reported in older animals. However, there are several developmental bone diseases in horses, pigs, chickens, turkeys, and dogs (Ytrehus et al. 2007) that have many similarities with osteoporosis. Research into these animal conditions has not been undertaken with VK, but raises the question of a possible role for VK in the pathogenesis of diseases related to equine bone development, as horses are particularly susceptible to anomalies of bone metabolism.
The VKDP OC is located within bone and plays a vital role in bone metabolism, facilitating the binding of bone minerals with protein (Hauschka et al. 1989). Decreased functionality of this protein has been found to be associated with an increased fracture risk and osteoporosis in humans. The γ-carboxylation of OC, and matrix Gla-proteins (MGP) in humans is indicative of bone health, with higher percentages of ucOC associated with a lower bone mineral density (BMD) and an increased risk of hip fracture (Gundberg et al. 2012). Biffin et al. (2008a) found that Thoroughbred yearlings with radiological lesions had a significantly lower BMD than did those with clean X-rays.
Binkley and Suttie (1995) found that in adults, a maximum VK supplemental intake of 1000 μg was effective in reducing percentage ucOC to its lowest level. Biffin et al. (2010) suggested that to attain 90% carboxylation of OC, 7 mg of vitamin K/day was required by a 500 kg horse using a bioavailable soluble form (Quinaquanone®; KQ); however, it is important to note that this pertains only to horses without access to fresh green pasture (Biffin et al. 2008a). Although a significant difference was not reported, these results emphasised that a higher intake of VK may be necessary for optimal functioning of VKDPs than was previously thought, especially for bone development of the foal. However, when Siciliano et al. (2000b) supplemented young Quarter horses at the initiation of exercise training with PK, there were no differences between treatments in relative bone density distribution in the carpal bones, or cumulative gross lesion score of the carpal joints. No significant correlation was present between OC and hydroxyapatite-binding capacity of serum OC, and other bone measures.
VK and energy metabolism
Studies examining the role of VK in bone health have highlighted the possible role of bone and therefore VK in energy metabolism, leading to the suggestion that bone is an endocrine organ (Lee et al. 2007). Studies in mice and rats found that removal of OC was associated with obesity and reduced glucose and insulin sensitivity, suggesting a link between OC and metabolic-related disorders such as diabetes (Gundberg et al. 2012). It was found that OC had a modulatory effect on adiposity in mice, by improving glucose metabolism, increasing β-cell proliferation and insulin secretion in the pancreas (Booth et al. 2013). Thus, bone was acting as an endocrine organ with OC, produced by osteoblasts, acting as a messenger for bone, targeting the pancreas and adipose tissue (Schwetz et al. 2012; de Paula and Rosen 2013; Moser and van der Eerden 2019). However, it has been proposed that OC may exert its effects through the hormone adiponectin (Booth et al. 2013). An intriguing finding of these mechanistic studies with knockout mice has been that the hormonally active form of OC is undercarboxylated, contrary to involvement in bone metabolism where it is carboxylated (Han et al. 2018; Wang et al. 2021; Alonso et al. 2023). Following carboxylation, OC binds with the minerals in the extracellular bone matrix. However, during bone resorption, the pH drops, creating a more acidic environment that facilitates de-carboxylation of OC. Undercarboxylated osteocalcin is released into the blood as it has lower affinity for the bone matrix and can circulate as a bone hormone (Han et al. 2018; Wang et al. 2021; Alonso et al. 2023).
Although the role of bone in energy metabolism is now appreciated, there is still much to unravel to fully understand the complexity of the intrinsic relationship between bone metabolism and whole-body energy homeostasis (de Paula and Rosen 2013; Dirckx et al. 2019). This is illustrated by studies in humans where a relationship between ucOC and glucose metabolism remains to be demonstrated clinically (Lin et al. 2018). Furthermore, in studies with three different OC knockout mouse models, a hormonal role for the undercarboxylated molecule was not always displayed by the different mouse phenotypes (Wang et al. 2021). Detailed discussions of the inconsistent results of the mouse models and the conflicting views on the endocrine role of OC have been provided by Han et al. (2018), Wang et al. (2021) and Alonso et al. (2023). Moreover, delineating cause and effect has become more complex as compounds with hormonal activity, in addition to OC, have been identified as originating from bone (Oldknow et al. 2015; Han et al. 2018; Dirckx et al. 2019; Wang et al. 2021; Alonso et al. 2023).
There are several equine metabolic disorders that relate to disturbances of energy metabolism, most noticeably obesity, hyperlipidaemia, insulin resistance, and equine metabolic syndrome (EMS). Equine metabolic syndrome was first described at the turn of this century; Johnson (2002) and Harris et al. (2020) have outlined how the concept of EMS has evolved. Insulin resistance is an important component of the disease, but the term insulin dysregulation is now preferred (Frank and Tadros 2014). The disease in horses and the metabolic syndrome in humans differ, but both involve vascular pathology, with the equine syndrome often resulting in laminitis, while cardiovascular disease is the outcome in humans (Ertelt et al. 2014). The pathogenesis of both syndromes is similar but poorly understood. There appears to be a relationship between the syndrome and osteoporosis in humans (Wong et al. 2016), suggesting an interplay with bone and possible hormonal role of OC.
Other pathways mediated by VK
With the recognition of bone as an endocrine organ, interactions with apparently unrelated organs have been found. A role for VK in the function of the brain and nervous system was postulated (Ferland 2012) and it now appears that the vitamin plays a role in brain development and cognition (Moser and van der Eerden 2019). The deposition of calcium in vascular tissues also appears to be in part mediated by VKDPs, with MGP found to act as a calcification-inhibitor (Bjorklund et al. 2020).
Recent research suggests that OC influences the hypothalamic–pituitary–gonadal axis, with a regulatory function on spermatogenesis and male fertility (Oury et al. 2011; Shan et al. 2021; Wang et al. 2021). Furthermore, there is evidence that OC is required for mounting the acute stress response (Berger et al. 2019) or the fight/flight reaction. It is not surprising therefore, that there is increasing evidence of a role for OC in adaption to, and energy utilisation by muscle during exercise (Mera et al. 2016; Dirckx et al. 2019; Alonso et al. 2023).
All these pathways have implications for the nutrition and management of horses. In this regard, an area of research that has received considerable attention in production animals is gut health or gut homeostasis, an area of great importance to horses. Significantly, there is accumulating evidence (reviewed by Lai et al. 2022) that VK plays a role in gut homeostasis through maintenance of intestinal barrier integrity and the gut microbiota, modulation of intestinal immunity, and reduction of inflammation.
Conclusions and perspective
Although VK was discovered some 90 years ago, it is only in the past 20 years, that the extent of the biochemical function of VK has been appreciated. With this increased understanding, VK should no longer be considered a single-function ‘haemostasis vitamin’, but rather as a ‘multi-function vitamin’. It is involved in many physiological processes in addition to blood coagulation, including bone and energy metabolism, spermatogenesis and immunity. These latter processes are usually overlooked as the role of many of the VKDPs has not been elucidated. Most of the research that has examined these aspects of VK metabolism has used either in vitro studies with cell lines or knockout mouse models. This is a complex area as the various vitamers of VK are different, not only with regard to their cofactor activity, but also their absorption, transport, tissue distribution and turnover. Moreover, unlike other fat-soluble vitamins, VK reserves are rapidly depleted from the body.
VK is essential for bone formation and bone mineralisation. The biosynthesis and activation of the key bone proteins (OC, matrix Gla-protein, and protein S) rely on VK. There has been much interest of late in the role of OC as a hormonal messenger for bone and the role that bone plays in whole-body energy homeostasis. Paradoxically, it is the carboxylated form of OC that is active in bone, but the undercarboxylated form of OC that has hormonal activity. The mitigation of osteoporosis in some aging human populations by VK shows much promise, but information on the role of VK in the bone integrity of young children and adults is lacking, as it is for rapidly growing animals. As horses are particularly susceptible to anomalies of bone metabolism, does VK have a role in improving equine bone health?
It is generally accepted that the requirements for VK are met from the diet and from microbial synthesis in the gut. However, there is evidence that these sources of the vitamin are not always bioavailable; PK is synthesised in green plants but destroyed by drying and MK-n is synthesised by bacteria in the hindgut but there is limited evidence that it is absorbed. There are many questions that recent research findings raise for the use of VK in horse nutrition. Which form of VK, when added to animal diets, will provide optimum performance and health, is a very important question. Much of the research on VK metabolism and requirements in animals occurred some two decades ago, but in the intervening period much progress has been made in our understanding of the pivotal role that VKDPs play in different aspects of metabolism and health. There is increasing awareness of the role of VK in bone health, energy metabolism and immunity, but little research has been conducted with animals. How do these findings in humans and rodents relate to VK status and the health and welfare of horses? However, a clear understanding of VK metabolism and function will not be possible till the current analytical limitations of the quantification of the vitamin and its different metabolic forms are overcome. It should then be possible to define the role of VK in equine metabolism and nutrition.
Data availability
Data sharing is not applicable as no new data were generated or analysed during this study.
Conflicts of interest
JRB and HLR are directors of Agricure Pty Ltd that holds a patent (US20150359757A1) on a veterinary VK supplement, sold under the name Quinaquinone®. WLB is a member of the Editorial Board of Animal Production Science but had no role in the peer review and editorial process for this paper. The authors have no further conflicts of interest to declare.
References
Abawi FG, Sullivan TW (1989) Interactions of vitamins A, D3, E, and K in the diet of broiler chicks. Poultry Science 68, 1490-1498.
| Crossref | Google Scholar |
Alisi L, Cao R, De Angelis C, Cafolla A, Caramia F, Cartocci G, Librando A, Fiorelli M (2019) The relationships between vitamin k and cognition: a review of current evidence. Frontiers in Neurology 10, 239.
| Crossref | Google Scholar |
Almquist HJ (1941) Report on vitamin K. Assay by curative biological test. Journal of the Association of Official Agricultural Chemists 24, 405-413.
| Crossref | Google Scholar |
Almquist HJ (1975) The early history of vitamin K. The American Journal of Clinical Nutrition 28, 656-659.
| Crossref | Google Scholar |
Almquist HJ, Stokstad ELR (1935a) Dietary hæmorrhagic disease in chicks. Nature 136, 31.
| Crossref | Google Scholar |
Almquist HJ, Stokstad ELR (1935b) Hemorrhagic chick disease of dietary origin. Journal of Biological Chemistry 111, 105-113.
| Crossref | Google Scholar |
Alonso N, Meinitzer A, Fritz-Petrin E, Enko D, Herrmann M (2023) Role of vitamin K in bone and muscle metabolism. Calcified Tissue International 112, 178-196.
| Crossref | Google Scholar |
Al Rajabi A, Booth SL, Peterson JW, Choi SW, Suttie JW, Shea MK, Miao B, Grusak MA, Fu X (2012) Deuterium-labeled phylloquinone has tissue-specific conversion to menaquinone-4 among Fischer 344 male rats. The Journal of Nutrition 142, 841-845.
| Crossref | Google Scholar |
Barkhan P, Shearer MJ (1977) Metabolism of vitamin K1 (phylloquinone) in man. Proceedings of the Royal Society of Medicine 70, 93-96.
| Crossref | Google Scholar |
Barreto ÍMLG, Rangel AHdN, Urbano SA, Bezerra JdS, Oliveira CAdA (2019) Equine milk and its potential use in the human diet. Food Science and Technology 39, 1-7.
| Crossref | Google Scholar |
Belij S, Miljkovic D, Popov A, Subota V, Timotijevic G, Slavic M, Mirkov I, Kataranovski D, Kataranovski M (2012) Effects of subacute oral warfarin administration on peripheral blood granulocytes in rats. Food and Chemical Toxicology 50, 1499-1507.
| Crossref | Google Scholar |
Bentley R, Meganathan R (1982) Biosynthesis of vitamin K (menaquinone) in bacteria. Microbiological Reviews 46, 241-280.
| Crossref | Google Scholar |
Berger JM, Singh P, Khrimian L, Morgan DA, Chowdhury S, Arteaga-Solis E, Horvath TL, Domingos AI, Marsland AL, Yadav VK, Rahmouni K, Gao X-B, Karsenty G (2019) Mediation of the acute stress response by the skeleton. Cell Metabolism 30, 890-902.e8.
| Crossref | Google Scholar |
Berkner KL (2005) The vitamin K-dependent carboxylase. Annual Review of Nutrition 25, 127-149.
| Crossref | Google Scholar |
Binkley NC, Suttie JW (1995) Vitamin K nutrition and osteoporosis. The Journal of Nutrition 125, 1812-1821.
| Crossref | Google Scholar |
Bjorklund G, Svanberg E, Dadar M, Card DJ, Chirumbolo S, Harrington DJ, Aaseth J (2020) The role of matrix gla protein (MGP) in vascular calcification. Current Medicinal Chemistry 27, 1647-1660.
| Crossref | Google Scholar |
Bolton-Smith C, Price RJG, Fenton ST, Harrington DJ, Shearer MJ (2000) Compilation of a provisional UK database for the phylloquinone (vitamin K1) content of foods. British Journal of Nutrition 83, 389-399.
| Crossref | Google Scholar |
Bonjour J-P, Guéguen L, Palacios C, Shearer MJ, Weaver CM (2009) Minerals and vitamins in bone health: the potential value of dietary enhancement. British Journal of Nutrition 101, 1581-1596.
| Crossref | Google Scholar |
Booth SL (2009) Roles for vitamin K beyond coagulation. Annual Review of Nutrition 29, 89-110.
| Crossref | Google Scholar |
Booth SL (2012) Vitamin K: food composition and dietary intakes. Food & Nutrition Research 56, 5505-5509.
| Crossref | Google Scholar |
Booth SL, Rajabi AA (2008) Determinants of vitamin K status in humans. Vitamins & Hormones 78, 1-22.
| Crossref | Google Scholar |
Booth SL, Golly I, Sacheck JM, Roubenoff R, Dallal GE, Hamada K, Blumberg JB (2004) Effect of vitamin E supplementation on vitamin K status in adults with normal coagulation status. The American Journal of Clinical Nutrition 80, 143-148.
| Crossref | Google Scholar |
Booth SL, Centi A, Smith SR, Gundberg C (2013) The role of osteocalcin in human glucose metabolism: marker or mediator? Nature Reviews Endocrinology 9, 43-55.
| Crossref | Google Scholar |
Byars TD, Greene CE, Kemp DT (1986) Antidotal effect of vitamin K1 against warfarin-induced anticoagulation in horses. American Journal of Veterinary Research 47, 2309-2312.
| Google Scholar |
Cancela ML, Conceicao N, Laize V (2012) Gla-rich protein, a new player in tissue calcification? Advances in Nutrition 3, 174-181.
| Crossref | Google Scholar |
Card DJ, Gorska R, Harrington DJ (2020) Laboratory assessment of vitamin K status. Journal of Clinical Pathology 73, 70-75.
| Crossref | Google Scholar |
Carvallo FR, Poppenga R, Kinde H, Diab SS, Nyaoke AC, Hill AE, Arthur RM, Uzal FA (2015) Cluster of cases of massive hemorrhage associated with anticoagulant detection in race horses. Journal of Veterinary Diagnostic Investigation 27, 112-116.
| Crossref | Google Scholar |
Caspers M, Czogalla KJ, Liphardt K, Muller J, Westhofen P, Watzka M, Oldenburg J (2015) Two enzymes catalyze vitamin K 2,3-epoxide reductase activity in mouse: VKORC1 is highly expressed in exocrine tissues while VKORC1L1 is highly expressed in brain. Thrombosis Research 135, 977-983.
| Crossref | Google Scholar |
Collins MD, Jones D (1981) Distribution of isoprenoid quinone structural types in bacteria and their taxonomic implications. Microbiological Reviews 45, 316-354.
| Crossref | Google Scholar |
Csapó J, Stefler J, Martin TG, Makray S, Csapó-Kiss Z (1995) Composition of mares’ colostrum and milk. Fat content, fatty acid composition and vitamin content. International Dairy Journal 5, 393-402.
| Crossref | Google Scholar |
Dam H (1935a) The antihaemorrhagic vitamin of the chick. Biochemical Journal 29, 1273-1285.
| Crossref | Google Scholar |
Dam H (1935b) The antihæmorrhagic vitamin of the chick: occurrence and chemical nature. Nature 135, 652-653.
| Crossref | Google Scholar |
de Paula FJA, Rosen CJ (2013) Bone remodeling and energy metabolism: new perspectives. Bone Research 1, 72-84.
| Crossref | Google Scholar |
Dirckx N, Moorer MC, Clemens TL, Riddle RC (2019) The role of osteoblasts in energy homeostasis. Nature Reviews Endocrinology 15, 651-665.
| Crossref | Google Scholar |
Doreau M, Boulot S (1989) Recent knowledge on mare milk production: a review. Livestock Production Science 22, 213-235.
| Crossref | Google Scholar |
Dror DK, Allen LH (2018) Overview of nutrients in human milk. Advances in Nutrition 9, 278S-294S.
| Crossref | Google Scholar |
Duello TJ, Matschiner JT (1970) Identification of phylloquinone in horse liver. Archives of Biochemistry and Biophysics 138, 640-645.
| Crossref | Google Scholar |
Edens LM, Morris DD, Prasse KW, Anver MR (1993) Hypercoaguable state associated with a deficiency of protein C in a Thoroughbred colt. Journal of Veterinary Internal Medicine 7, 190-193.
| Crossref | Google Scholar |
EFSA Panel on Additives and Products or Substances used in Animal Feed (FEEDAP) (2014) Scientific opinion on the safety and efficacy of vitamin K3 (menadione sodium bisulphite and menadione nicotinamide bisulphite) as a feed additive for all animal species. EFSA Journal 12, 3532.
| Crossref | Google Scholar |
EFSA Panel on Additives and Products or Substances used in Animal Feed (FEEDAP), Bampidis V, Azimonti G, Bastos ML, Christensen H, Dusemund B, Fašmon Durjava M, Kouba M, López-Alonso M, López Puente S, Marcon F, Mayo B, Pechová A, Petkova M, Ramos F, Sanz Y, Villa RE, Woutersen R, Groop J, Anguita M, Galobart J, Holczknecht O, Manini P, Pettenati E, Pizzo F, Tarrés-Call J (2021) Scientific opinion on the safety and efficacy of an additive consisting of synthetic vitamin K1 (phytomenadione) for horses. EFSA Journal 19, 6538.
| Crossref | Google Scholar |
Elkattawy HA, Ghoneim FM, Eladl MA, Said E, Ebrahim HA, El-Shafey M, Asseri SM, El-Sherbiny M, Alsalamah RH, Elsherbiny NM, Hadhod S (2022) Vitamin K2 (menaquinone-7) reverses age-related structural and cognitive deterioration in naturally aging rats. Antioxidants 11, 514.
| Crossref | Google Scholar |
Ellis JL, Karl JP, Oliverio AM, Fu X, Soares JW, Wolfe BE, Hernandez CJ, Mason JB, Booth SL (2021) Dietary vitamin K is remodeled by gut microbiota and influences community composition. Gut Microbes 13, 1-16.
| Crossref | Google Scholar |
Ellis JL, Fu X, Karl JP, Hernandez CJ, Mason JB, DeBose-Boyd RA, Booth SL (2022) Multiple dietary vitamin K forms are converted to tissue menaquinone-4 in mice. The Journal of Nutrition 152, 981-993.
| Crossref | Google Scholar |
Erkkilä AT, Lichtenstein AH, Dolnikowski GG, Grusak MA, Jalbert SM, Aquino KA, Peterson JW, Booth SL (2004) Plasma transport of vitamin K in men using deuterium-labeled collard greens. Metabolism 53, 215-221.
| Crossref | Google Scholar |
Ertelt A, Barton A-K, Schmitz RR, Gehlen H (2014) Metabolic syndrome: is equine disease comparable to what we know in humans? Endocrine Connections 3, SR81-SR93.
| Crossref | Google Scholar |
Falcone TD, Kim SSW, Cortazzo MH (2011) Vitamin K: fracture prevention and beyond. PM&R 3, 82-87.
| Crossref | Google Scholar |
Ferland G (2012) Vitamin K and the nervous system: an overview of its actions. Advances in Nutrition 3, 204-212.
| Crossref | Google Scholar |
Ferland G, Sadowski JA (1992) Vitamin K1 (phylloquinone) content of edible oils: effects of heating and light exposure. Journal of Agricultural and Food Chemistry 40, 1869-1873.
| Crossref | Google Scholar |
Fernandez F, Collins MD (1987) Vitamin K composition of anaerobic gut bacteria. FEMS Microbiology Letters 41, 175-180.
| Crossref | Google Scholar |
Fischer TJ, Coyle MP, Regtop HL, Talbot AM, Biffin JR, Cawdell-Smith AJ, Bryden WL (2017) Placental transfer of vitamin K in the horse. Journal of Equine Veterinary Science 52, 57.
| Crossref | Google Scholar |
Fleming RH (2008) Nutritional factors affecting poultry bone health. Proceedings of the Nutrition Society 67, 177-183.
| Crossref | Google Scholar |
Frank N, Tadros EM (2014) Insulin dysregulation. Equine Veterinary Journal 46, 103-112.
| Crossref | Google Scholar |
Fusaro M, Dalle Carbonare L, Dusso A, Arcidiacono MV, Valenti MT, Aghi A, Pasho S, Gallieni M (2015) Differential effects of dabigatran and warfarin on bone volume and structure in rats with normal renal function. PLoS ONE 10, e0133847.
| Crossref | Google Scholar |
Fusaro M, Plebani M, Iervasi G, Gallieni M (2017a) Vitamin K deficiency in chronic kidney disease: evidence is building up. American Journal of Nephrology 45, 1-3.
| Crossref | Google Scholar |
Fusaro M, Gallieni M, Rizzo MA, Stucchi A, Delanaye P, Cavalier E, Moyses RMA, Jorgetti V, Iervasi G, Giannini S, Fabris F, Aghi A, Sella S, Galli F, Viola V, Plebani M (2017b) Vitamin K plasma levels determination in human health. Clinical Chemistry and Laboratory Medicine (CCLM) 55, 789-799.
| Crossref | Google Scholar |
Garber AK, Binkley NC, Krueger DC, Suttie JW (1999) Comparison of phylloquinone bioavaliability from food sources or a supplement in human subjects. The Journal of Nutrition 129, 1201-1203.
| Crossref | Google Scholar |
Gijsbers BLMG, Jie K-SG, Vermeer C (1996) Effect of food composition on vitamin K absorption in human volunteers. British Journal of Nutrition 76, 223-229.
| Crossref | Google Scholar |
Goncalves A, Margier M, Roi S, Collet X, Niot I, Goupy P, Caris-Veyrat C, Reboul E (2014) Intestinal scavenger receptors are involved in vitamin K1 absorption. Journal of Biological Chemistry 289, 30743-30752.
| Crossref | Google Scholar |
Goncalves A, Roi S, Nowicki M, Dhaussy A, Huertas A, Amiot M-J, Reboul E (2015) Fat-soluble vitamin intestinal absorption: absorption sites in the intestine and interactions for absorption. Food Chemistry 172, 155-160.
| Crossref | Google Scholar |
Greer FR, Marshall SP, Foley AL, Suttie JW (1997) Improving the vitamin K status of breastfeeding infants with maternal vitamin K supplements. Pediatrics 99, 88-92.
| Crossref | Google Scholar |
Gundberg CM, Nieman SD, Abrams S, Rosen H (1998) Vitamin K status and bone health: an analysis of methods for determination of undercarboxylated osteocalcin. Journal of Clinical Endocrinology & Metabolism 83, 3258-3266.
| Crossref | Google Scholar |
Gundberg CM, Lian JB, Booth SL (2012) Vitamin K-dependent carboxylation of osteocalcin: friend or foe? Advances in Nutrition 3, 149-157.
| Crossref | Google Scholar |
Guo S, Niu J, Xv J, Fang B, Zhang Z, Zhao D, Wang L, Ding B (2021) Interactive effects of vitamins A and K3 on laying performance, egg quality, tibia attributes and antioxidative status of aged Roman Pink laying hens. Animal 15, 100 242.
| Crossref | Google Scholar |
Hallgren KW, Zhang D, Kinter M, Willard B, Berkner KL (2013) Methylation of γ-carboxylated Glu (Gla) allows detection by liquid chromatography–mass spectrometry and the identification of Gla residues in the γ-glutamyl carboxylase. Journal of Proteome Research 12, 2365.
| Crossref | Google Scholar |
Hammed A, Matagrin B, Spohn G, Prouillac C, Benoit E, Lattard V (2013) VKORC1L1, an enzyme rescuing the vitamin K 2,3-epoxide reductase activity in some extrahepatic tissues during anticoagulation therapy. Journal of Biological Chemistry 288, 28733-28742.
| Crossref | Google Scholar |
Han Y, You X, Xing W, Zhang Z, Zou W (2018) Paracrine and endocrine actions of bone – the functions of secretory proteins from osteoblasts, osteocytes, and osteoclasts. Bone Research 6, 1-11.
| Crossref | Google Scholar |
Harris PA, Bamford NJ, Bailey SR (2020) Equine metabolic syndrome: evolution of understanding over two decades: a personal perspective. Animal Production Science 60, 2103-2110.
| Crossref | Google Scholar |
Harshman SG, Saltzman E, Booth SL (2014) Vitamin K: dietary intake and requirements in different clinical conditions. Current Opinion in Clinical Nutrition and Metabolic Care 17, 531-538.
| Crossref | Google Scholar |
Harshman SG, Fu X, Karl JP, Barger K, Lamon-Fava S, Kuliopulos A, Greenberg AS, Smith D, Shen X, Booth SL (2016) Tissue concentrations of vitamin K and expression of key enzymes of vitamin K metabolism are influenced by sex and diet but not housing in C57Bl6 mice. The Journal of Nutrition 146, 1521-1527.
| Crossref | Google Scholar |
Harshman SG, Finnan EG, Barger KJ, Bailey RL, Haytowitz DB, Gilhooly CH, Booth SL (2017) Vegetables and mixed dishes are top contributors to phylloquinone intake in US adults: data from the 2011-2012 NHANES. The Journal of Nutrition 147, 1308-1313.
| Crossref | Google Scholar |
Hauschka PV, Lian JB, Cole DE, Gundberg CM (1989) Osteocalcin and matrix Gla protein: vitamin K-dependent proteins in bone. Physiological Reviews 69, 990-1047.
| Crossref | Google Scholar |
Hirauchi K, Sakano T, Notsumoto S, Nagaoka T, Morimoto A, Fujimoto K, Masuda S, Suzuki Y (1989) Measurement of k vitamins in animal tissues by high-performance liquid chromatography with fluorimetric detection. Journal of Chromatography B: Biomedical Sciences and Applications 497, 131-137.
| Crossref | Google Scholar |
Holden RM, Booth SL, Day AG, Clase CM, Zimmerman D, Moist L, Shea MK, McCabe KM, Jamal SA, Tobe S, Weinstein J, Madhumathi R, Adams MA, Heyland DK (2015) Inhibiting the progression of arterial calcification with vitamin K in hemodialysis patients (iPACK-HD) trial: rationale and study design for a randomized trial of vitamin K in patients with end stage kidney disease. Canadian Journal of Kidney Health and Disease 2, 53.
| Crossref | Google Scholar |
Hollander D (1973) Vitamin K1 absorption by everted intestinal sacs of the rat. American Journal of Physiology 225, 360-364.
| Crossref | Google Scholar |
Inoue Y, Ohba Y, Kobayashi Y, Komori M, Hayakawa S, Matsui T (2009) Determination of plasma concentrations of vitamin K homologues in Thoroughbred horses. Journal of Equine Veterinary Science 29, 395-396.
| Crossref | Google Scholar |
Iwamoto J, Yeh JK, Takeda T, Ichimura S, Sato Y (2003) Comparative effects of vitamin K and vitamin D supplementation on prevention of osteopenia in calcium-deficient young rats. Bone 33, 557-566.
| Crossref | Google Scholar |
Jensen MB, Biltoft-Jensen AP, Jakobsen J (2022) In vitro bioaccessibility of vitamin K (phylloquinone and menaquinones) in food and supplements assessed by INFOGEST 2.0 – vit K. Current Research in Food Science 5, 306-312.
| Crossref | Google Scholar |
Johnson PJ (2002) The equine metabolic syndrome: peripheral Cushing’s syndrome. Veterinary Clinics of North America: Equine Practice 18, 271-293.
| Crossref | Google Scholar |
Jones KS, Bluck LJC, Wang LY, Stephen AM, Prynne CJ, Coward WA (2009) The effect of different meals on the absorption of stable isotope-labelled phylloquinone. British Journal of Nutrition 102, 1195-1202.
| Crossref | Google Scholar |
Jukes TH (1980) Vitamin K – a reminiscence. Trends in Biochemical Sciences 5, 140-141.
| Crossref | Google Scholar |
Kang M-J, Baek K-R, Lee Y-R, Kim G-H, Seo S-O (2022) Production of vitamin K by wild-type and engineered microorganisms. Microorganisms 10, 554.
| Crossref | Google Scholar |
Karl JP, Meydani M, Barnett JB, Vanegas SM, Barger K, Fu X, Goldin B, Kane A, Rasmussen H, Vangay P, Knights D, Jonnalagadda SS, Saltzman E, Roberts SB, Meydani SN, Booth SL (2017) Fecal concentrations of bacterially derived vitamin K forms are associated with gut microbiota composition but not plasma or fecal cytokine concentrations in healthy adults. The American Journal of Clinical Nutrition 106, 1052-1061.
| Crossref | Google Scholar |
Kazzi JN, Ilagan BN, Liang MK-C, Kazzi AG, Grietsell WL, Brans WY (1990) Placental transfer of vitamin K1 in preterm pregnancy. Obstetrics and Gynecology 75, 334-337.
| Google Scholar |
Krešimir K, Vesna G, Klemen P, Angela C (2011) Mare’s milk: composition and protein fraction in comparison with different milk species. Mljekarstvo 61, 107-113.
| Google Scholar |
Krossoy C, Waagbo R, Ornsrud R (2011) Vitamin K in fish nutrition. Aquaculture Nutrition 17, 585-594.
| Crossref | Google Scholar |
Lacombe J, Ferron M (2015) Gamma-carboxylation regulates osteocalcin function. Oncotarget 6, 19924-19925.
| Crossref | Google Scholar |
Lai Y, Masatoshi H, Ma Y, Guo Y, Zhang B (2022) Role of vitamin K in intestinal health. Frontiers in Immunology 12, 791565.
| Crossref | Google Scholar |
Lanham-New SA (2008) Importance of calcium, vitamin D and vitamin K for osteoporosis prevention and treatment. Proceedings of the Nutrition Society 67, 163-167.
| Crossref | Google Scholar |
Lee NK, Sowa H, Hinoi E, Ferron M, Ahn JD, Confavreux C, Dacquin R, Mee PJ, McKee MD, Jung DY, Zhang Z, Kim JK, Mauvais-Jarvis F, Ducy P, Karsenty G (2007) Endocrine regulation of energy metabolism by the skeleton. Cell 130, 456-469.
| Crossref | Google Scholar |
Li T, Chang C-Y, Jin D-Y, Lin P-J, Khvorova A, Stafford DW (2004) Identification of the gene for vitamin K epoxide reductase. Nature 427, 541-544.
| Crossref | Google Scholar |
Li X, Zhang D, Bryden WL (2017) Calcium and phosphorus metabolism and nutrition of poultry: are current diets formulated in excess? Animal Production Science 57, 2304-2310.
| Crossref | Google Scholar |
Lin X, Brennan-Speranza TC, Levinger I, Yeap BB (2018) Undercarboxylated osteocalcin: experimental and human evidence for a role in glucose homeostasis and muscle regulation of insulin sensitivity. Nutrients 10, 847.
| Crossref | Google Scholar |
Link KP (1959) The discovery of dicumarol and its sequels. Circulation 19, 97-107.
| Crossref | Google Scholar |
Malashkevich VN, Almo SC, Dowd TL (2013) X-ray crystal structure of bovine 3 Glu-osteocalcin. Biochemistry 52, 8387-8392.
| Crossref | Google Scholar |
Manna P, Kalita J (2016) Beneficial role of vitamin K supplementation on insulin sensitivity, glucose metabolism, and the reduced risk of type 2 diabetes: a review. Nutrition 32, 732-739.
| Crossref | Google Scholar |
Marchetti M, Tassinari M, Marchetti S (2000) Menadione nicotinamide bisulphite as a source of vitamin K and niacin activities for the growing pig. Animal Science 71, 111-117.
| Crossref | Google Scholar |
Martius C (1961) The metabolic relationships between the different K vitamins and the synthesis of the ubiquinones. The American Journal of Clinical Nutrition 9, 97-103.
| Crossref | Google Scholar |
Maxie G, van Dreumel T, McMaster D, Baird J (1992) Ontario. Menadione (vitamin K(3) toxicity in six horses. Canadian Veterinary Journal 33, 756-757.
| Google Scholar |
McCabe KM, Booth SL, Fu X, Ward E, Adams MA, Holden RM (2017) Vitamin K metabolism in a rat model of chronic kidney disease. American Journal of Nephrology 45, 4-13.
| Crossref | Google Scholar |
McCann JC, Ames BN (2009) Vitamin K, an example of triage theory: is micronutrient inadequacy linked to diseases of aging? The American Journal of Clinical Nutrition 90, 889-907.
| Crossref | Google Scholar |
McCann A, Jeffery IB, Ouliass B, Ferland G, Fu X, Booth SL, Tran TTT, O’Toole PW, O’Connor EM (2019) Exploratory analysis of covariation of microbiota-derived vitamin K and cognition in older adults. The American Journal of Clinical Nutrition 110, 1404-1415.
| Crossref | Google Scholar |
McDonald GK (1980) Moldy sweetclover poisoning in a horse. Canadian Veterinary Journal 21, 250-251.
| Google Scholar |
McGorum BC, Henderson ISF, Stirling D, Wallace R, Haggart C, Thomas AE (2009) Vitamin K deficiency bleeding in a Standardbred colt. Journal of Veterinary Internal Medicine 23, 1307-1310.
| Crossref | Google Scholar |
Mera P, Laue K, Ferron M, Confavreux C, Wei J, Galán-Díez M, Lacampagne A, Mitchell SJ, Mattison JA, Chen Y, Bacchetta J, Szulc P, Kitsis RN, de Cabo R, Friedman RA, Torsitano C, McGraw TE, Puchowicz M, Kurland I, Karsenty G (2016) Osteocalcin signaling in myofibers is necessary and sufficient for optimum adaptation to exercise. Cell Metabolism 23, 1078-1092.
| Crossref | Google Scholar |
Misra D, Booth SL, Tolstykh I, Felson DT, Nevitt MC, Lewis CE, Torner J, Neogi T (2013) Vitamin K deficiency is associated with incident knee osteoarthritis. The American Journal of Medicine 126, 243-248.
| Crossref | Google Scholar |
Mohamed WAM, Bayomi YH, Attia NE, El Raouf MA (2017) Diagnostic approach to nephrotoxicosis with vitamin K3 in draft horses based on iNOS and selective urinary variables. Advances in Animal and Veterinary Sciences 5, 468-476.
| Crossref | Google Scholar |
Moser SC, van der Eerden BCJ (2019) Osteocalcin – a versatile bone-derived hormone. Frontiers in Endocrinology 9, 794.
| Crossref | Google Scholar |
Mott A, Bradley T, Wright K, Cockayne ES, Shearer MJ, Adamson J, Lanham-New SA, Torgerson DJ (2019) Effect of vitamin K on bone mineral density and fractures in adults: an updated systematic review and meta-analysis of randomised controlled trials. Osteoporosis International 30, 1543-1559.
| Crossref | Google Scholar |
Newman P, Bonello F, Wierzbicki AS, Lumb P, Savidge GF, Shearer MJ (2002) The uptake of lipoprotein-borne phylloquinone (vitamin K1) by osteoblasts and osteoblast-like cells: role of heparan sulfate proteoglycans and apolipoprotein E. Journal of Bone and Mineral Research 17, 426-433.
| Crossref | Google Scholar |
Oldknow KJ, MacRae VE, Farquharson C (2015) Endocrine role of bone: recent and emerging perspectives beyond osteocalcin. Journal of Endocrinology 225, R1-R19.
| Crossref | Google Scholar |
Oury F, Sumara G, Sumara O, Ferron M, Chang H, Smith CE, Hermo L, Suarez S, Roth BL, Ducy P (2011) Endocrine regulation of male fertility by the skeleton. Cell 144, 796-809.
| Crossref | Google Scholar |
Oury F, Khrimian L, Denny CA, Gardin A, Chamouni A, Goeden N, Huang Y-Y, Lee H, Srinivas P, Gao X-B, Suyama S, Langer T, Mann JJ, Horvath TL, Bonnin A, Karsenty G (2013) Maternal and offspring pools of osteocalcin influence brain development and functions. Cell 155, 228-241.
| Crossref | Google Scholar |
Piccione G, Percipalle M, Fazio F, Ferrantelli V, Caola G (2005) Circadian variation of blood clotting time and circulating vitamin K in the athletic horse. Comparative Clinical Pathology 14, 86-89.
| Crossref | Google Scholar |
Pieszka M, Luszczynski J, Zamachowska M, Augustyn R, Dlugosz B, Hedrzak M (2016) Is mare milk an appropriate food for people? A review. Annals of Animal Science 16, 33-51.
| Crossref | Google Scholar |
Pizzorno J (2011) Is vitamin K the next vitamin D? Integrative Medicine 10, 10-12.
| Google Scholar |
Price PA, Parthemore JG, Deftos LJ (1980) New biochemical marker for bone metabolism. Measurement by radioimmunoassay of bone GLA protein in the plasma of normal subjects and patients with bone disease. Journal of Clinical Investigation 66, 878-883.
| Crossref | Google Scholar |
Quinn L, Sheh A, Ellis JL, Smith DE, Booth SL, Fu X, Muthupalani S, Ge Z, Puglisi DA, Wang TC, Gonda TA, Holcombe H, Fox JG (2020) Helicobacter pylori antibiotic eradication coupled with a chemically defined diet in INS-GAS mice triggers dysbiosis and vitamin K deficiency resulting in gastric hemorrhage. Gut Microbes 11, 820-841.
| Crossref | Google Scholar |
Rathgeber RA, Brooks MB, Bain FT, Byars TD (2001) Clinical vignette. Von Willebrand disease in a Thoroughbred mare and foal. Journal of Veterinary Internal Medicine 15, 63-66.
| Crossref | Google Scholar |
Rebhun WC, Tennant BC, Dill SG, King JM (1984) Vitamin K3-induced renal toxicosis in the horse. Journal of American Veterinary Medicine Association 184, 1237-1239.
| Google Scholar |
Reboul E, Borel P (2011) Proteins involved in uptake, intracellular transport and basolateral secretion of fat-soluble vitamins and carotenoids by mammalian enterocytes. Progress in Lipid Research 50, 388-402.
| Crossref | Google Scholar |
Rishavy MA, Berkner KL (2012) Vitamin K oxygenation, glutamate carboxylation, and processivity: defining the three critical facets of catalysis by the vitamin K-dependent carboxylase. Advances in Nutrition 3, 135-148.
| Crossref | Google Scholar |
Schurgers LJ, Vermeer C (2000) Determination of phylloquinone and menaquinones in food: effect of food matrix on circulating vitamin K concentrations. Haemostasis 30, 298-307.
| Crossref | Google Scholar |
Schurgers LJ, Vermeer C (2002) Differential lipoprotein transport pathways of K-vitamins in healthy subjects. Biochimica et Biophysica Acta (BBA) – General Subjects 1570, 27-32.
| Crossref | Google Scholar |
Schwetz V, Pieber T, Obermayer-Pietsch B (2012) Mechanisms in endocrinology: the endocrine role of the skeleton: background and clinical evidence. European Journal of Endocrinology 166, 959-967.
| Crossref | Google Scholar |
Scott EA, Sandler GA, Byars TD (1978) Warfarin: effects of intravenous loading doses and vitamin K on warfarin anticoagulation in the pony. American Journal of Veterinary Research 39, 1888-1891.
| Google Scholar |
Scott EA, Sandler GA, Byars TD (1979) Warfarin: effects on anticoagulant, hematologic, and blood enzyme values in normal ponies. American Journal of Veterinary Research 40, 142-146.
| Google Scholar |
Scott EA, Byars TD, Lamar AM (1980) Warfarin anticoagulation in the horse. Journal of American Veterinary Medicine Association 177, 1146-1151.
| Google Scholar |
Shah DV, Suttie JW (1974) The vitamin K dependent, in vitro production of prothrombin. Biochemical and Biophysical Research Communications 60, 1397-1402.
| Crossref | Google Scholar |
Shan C, Yue J, Liu W (2021) Broadening the role of osteocalcin in the hypothalamic–pituitary–gonadal axis. Journal of Endocrinology 249, R43-R51.
| Crossref | Google Scholar |
Shea MK, Booth SL (2007) Role of vitamin K in the regulation of calcification. International Congress Series 1297, 165-178.
| Crossref | Google Scholar |
Shea MK, Booth SL (2016) Concepts and controversies in evaluating vitamin K status in population-based studies. Nutrients 8, 8.
| Crossref | Google Scholar |
Shea MK, Booth SL, Gundberg CM, Peterson JW, Waddell C, Dawson-Hughes B, Saltzman E (2010) Adulthood obesity is positively associated with adipose tissue concentrations of vitamin K and inversely associated with circulating indicators of vitamin K status in men and women. The Journal of Nutrition 140, 1029-1034.
| Crossref | Google Scholar |
Shea MK, Booth SL, Nettleton JA, Burke GL, Chen H, Kritchevsky SB (2012) Circulating phylloquinone concentrations of adults in the United States differ according to race and ethnicity. The Journal of Nutrition 142, 1060-1066.
| Crossref | Google Scholar |
Shearer MJ (1995) Vitamin K. The Lancet 345, 229-234.
| Crossref | Google Scholar |
Shearer MJ (1997) The roles of vitamins D and K in bone health and osteoporosis prevention. Proceedings of the Nutrition Society 56, 915-937.
| Crossref | Google Scholar |
Shearer MJ (2009) Vitamin K deficiency bleeding (VKDB) in early infancy. Blood Reviews 23, 49-59.
| Crossref | Google Scholar |
Shearer MJ (2022) The biosynthesis of menaquinone-4: how a historic biochemical pathway is changing our understanding of vitamin K nutrition. The Journal of Nutrition 152, 917-919.
| Crossref | Google Scholar |
Shearer MJ, Newman P (2008) Metabolism and cell biology of vitamin K. Thrombosis and Haemostasis 100, 530-547.
| Crossref | Google Scholar |
Shearer MJ, Newman P (2014) Recent trends in the metabolism and cell biology of vitamin K with special reference to vitamin K cycling and MK-4 biosynthesis. Journal of Lipid Research 55, 345-362.
| Crossref | Google Scholar |
Shearer MJ, Okano T (2018) Key pathways and regulators of vitamin K function and intermediary metabolism. Annual Review of Nutrition 38, 127-151.
| Crossref | Google Scholar |
Shearer MJ, McBurney A, Barkhan P (1974) Studies on the absorption and metabolism of phylloquinone (Vitamin K1) in man. Vitamins and Hormones 32, 513-542.
| Crossref | Google Scholar |
Shearer MJ, Fu X, Booth SL (2012) Vitamin K nutrition, metabolism, and requirements: current concepts and future research. Advances in Nutrition 3, 182-195.
| Crossref | Google Scholar |
Siciliano PD, Warren LK, Lawrence LM (2000a) Changes in vitamin K status of growing horses. Journal of Equine Veterinary Science 20, 726-729.
| Crossref | Google Scholar |
Siciliano PD, Kawcak CE, McIlwraith CW (2000b) The effect of initiation of exercise training in young horses on vitamin K status. Journal of Animal Science 78, 2353-2358.
| Crossref | Google Scholar |
Skinner JE, Cawdell-Smith AJ, Bryden WL (2014) Equine vitamin K absorption. Journal of Nutrition & Intermediary Metabolism 1, 50.
| Crossref | Google Scholar |
Skinner JE, Cawdell-Smith AJ, Regtop HL, Talbot AM, Biffin JR, Bryden WL (2015) 59 Extent of vitamin K absorption from the equine hindgut. Journal of Equine Veterinary Science 35, 409.
| Crossref | Google Scholar |
Southwood LLD, Evans DL, Bryden WL, Rose RJ (1993) Feeding practices in Thoroughbred and Standardbred racehorse stables. Australian Veterinary Journal 70, 184-185.
| Crossref | Google Scholar |
Speight RE, Navone L, Gebbie LK, Blinco J-AL, Bryden WL (2022) Platforms to accelerate biomanufacturing of enzyme and probiotic animal feed supplements: discovery considerations and manufacturing implications. Animal Production Science 62, 1113-1128.
| Crossref | Google Scholar |
Stafford DW (2005) The vitamin K cycle. Journal of Thrombosis and Haemostasis 3, 1873-1878.
| Crossref | Google Scholar |
Su S, He N, Men P, Song C, Zhai S (2019) The efficacy and safety of menatetrenone in the management of osteoporosis: a systematic review and meta-analysis of randomized controlled trials. Osteoporosis International 30, 1175-1186.
| Crossref | Google Scholar |
Suttie JW, Booth SL (2011) Vitamin K. Advances in Nutrition 2, 440-441.
| Crossref | Google Scholar |
Tarento TDC, McClure DD, Talbot AM, Regtop HL, Biffin JR, Valtchev P, Dehghani F, Kavanagh JM (2019) A potential biotechnological process for the sustainable production of vitamin K1. Critical Reviews in Biotechnology 39, 1-19.
| Crossref | Google Scholar |
Terachi T, Inoue Y, Ashihara N, Kobayashi M, Ando K, Matsui T (2011) Plasma vitamin K concentration in horses supplemented with several vitamin K homologs. Journal of Animal Science 89, 1056-1061.
| Crossref | Google Scholar |
Thane CW, Bates CJ, Shearer MJ, Unadkat N, Harrington DJ, Paul AA, Prentice A, Bolton-Smith C (2002) Plasma phylloquinone (vitamin K1) concentration and its relationship to intake in a national sample of British elderly people. British Journal of Nutrition 88, 437.
| Crossref | Google Scholar |
Thijssen HHW, Drittij-Reijnders MJ (1996) Vitamin K status in human tissues: tissue-specific accumulation of phylloquinone and menaquinone-4. British Journal of Nutrition 75, 121-127.
| Crossref | Google Scholar |
Thijssen HHW, Drittij-Reijnders MJ, Fischer MAJG (1996) Phylloquinone and menaquinone-4 distribution in rats: synthesis rather than uptake determines menaquinone-4 organ concentrations. The Journal of Nutrition 126, 537-543.
| Crossref | Google Scholar |
Thijssen HHW, Vervoort LMT, Schurgers LJ, Shearer MJ (2006) Menadione is a metabolite of oral vitamin K. British Journal of Nutrition 95, 260-266.
| Crossref | Google Scholar |
Tie J-K, Stafford DW (2016) Structural and functional insights into enzymes of the vitamin K cycle. Journal of Thrombosis and Haemostasis 14, 236-247.
| Crossref | Google Scholar |
Tie J-K, Carneiro JDA, Jin D-Y, Martinhago CD, Vermeer C, Stafford DW (2016) Characterization of vitamin K-dependent carboxylase mutations that cause bleeding and nonbleeding disorders. Blood 127, 1847-1855.
| Crossref | Google Scholar |
Tovar A, Ameho CK, Blumberg JB, Peterson JW, Smith D, Booth SL (2006) Extrahepatic tissue concentrations of vitamin K are lower in rats fed a high vitamin E diet. Nutrition & Metabolism 3, 29.
| Crossref | Google Scholar |
Traber MG (2008) Vitamin E and K interactions – a 50-year-old problem. Nutrition Reviews 66, 624-629.
| Crossref | Google Scholar |
Vermeer CV (2012) Vitamin K: the effect on health beyond coagulation – an overview. Food & Nutrition Research 56, 5329.
| Crossref | Google Scholar |
Vermeer C, Ulrich M (1982) Vitamin K-dependent carboxylase in horse liver, spleen and kidney. Thrombosis Research 28, 171-177.
| Crossref | Google Scholar |
Vermeer C, van’t Hoofd C, Knapen MHJ, Xanthoulea S (2017) Synthesis of 2-methyl-1,4-naphthoquinones with higher gamma-glutamyl carboxylase activity than MK-4 both in vitro and in vivo. Bioorganic & Medicinal Chemistry Letters 27, 208-211.
| Crossref | Google Scholar |
Vermeer C, Raes J, van’t Hoofd C, Knapen MHJ, Xanthoulea S (2018) Menaquinone content of cheese. Nutrients 10, 446.
| Crossref | Google Scholar |
Vrins A, Carlson G, Feldman B (1983) Warfarin: a review with emphasis on its use in the horse. Canadian Veterinary Journal 24, 211-213.
| Google Scholar |
Wallin R, Martin LF (1985) Vitamin K-dependent carboxylation and vitamin K metabolism in liver. Effects of warfarin. The Journal of Clinical Investigation 76, 1879-1884.
| Crossref | Google Scholar |
Wang JS, Mazur CM, Wein MN (2021) Sclerostin and osteocalcin: candidate bone-produced hormones. Frontiers in Endocrinology 12, 584147.
| Crossref | Google Scholar |
Wen L, Chen J, Duan L, Li S (2018) Vitamin K dependent proteins involved in bone and cardiovascular health (review). Molecular Medicine Reports 18, 3-15.
| Crossref | Google Scholar |
Westenfeld R, Krueger T, Schlieper G, Cranenburg ECM, Magdeleyns EJ, Heidenreich S, Holzmann S, Vermeer C, Jahnen-Dechent W, Ketteler M, Floege J, Schurgers LJ (2012) Effect of vitamin K2 supplementation on functional vitamin K deficiency in hemodialysis patients: a randomized trial. American Journal of Kidney Diseases 59, 186-195.
| Crossref | Google Scholar |
Wong SK, Chin K-Y, Suhaimi FH, Ahmad F, Ima-Nirwana S (2016) The relationship between metabolic syndrome and osteoporosis: a review. Nutrients 8, 347.
| Crossref | Google Scholar |
Yeum KJ, Booth SL, Roubenoff R, Russell RM (2017) Plasma carotenoid concentrations are inversely correlated with fat mass in older women. Journal of Nutrition, Health and Aging 2, 79-83.
| Google Scholar |
Ytrehus B, Carlson CS, Ekman S (2007) Etiology and pathogenesis of osteochondrosis. Veterinary Pathology 44, 429-448.
| Crossref | Google Scholar |
Zakian A, Mami S, Nouri M, Jalali SM, Tehrani-Sharif M (2019) Brodifacoum toxicosis and abortion in an Arabian mare. Veterinary Research Forum 10, 173-176.
| Crossref | Google Scholar |
Zhang C, Li D, Wang F, Dong T (2003) Effects of dietary vitamin K levels on bone quality in broilers. Archives of Animal Nutrition 57, 197-206.
| Crossref | Google Scholar |
Zurynski Y, Grover CJ, Jalaludin B, Elliott EJ (2020) Vitamin K deficiency bleeding in Australian infants 1993-2017: an Australian Paediatric Surveillance Unit study. Archives of Disease in Childhood 105, 433-438.
| Crossref | Google Scholar |
Zwakenberg SR, Engelen AIP, Dalmeijer GW, Booth SL, Vermeer C, Drijvers JJMM, Ocke MC, Feskens EJM, van der Schouw YT, Beulens JWJ (2017) Reproducibility and relative validity of a food frequency questionnaire to estimate intake of dietary phylloquinone and menaquinones. European Journal of Clinical Nutrition 71, 1423-1428.
| Crossref | Google Scholar |