Spatial mosaics of small animal communities across habitat and vegetation cover in semiarid Australia
Shirley S. Chia A , Keith Leggett B and Lisa E. Schwanz
A
B
Abstract
The fluctuating environment of arid lands drives spatial and temporal variation in resource availability and habitat suitability for animals. Patches of vegetative growth may create refuges that allow populations to persist when resources are scarce. Yet the links between fine-scale spatial variation and animal abundance are not well known. Here, we examine whether spatial variation in habitat and microhabitat create spatial mosaics in animal abundance. We surveyed ground-dwelling vertebrates and invertebrates in two adjacent habitats in semiarid Australia (cracking clay floodplains and rocky gibber), and across variation in microhabitat (vegetation cover). There was considerable variation in vegetation cover, creating fine-scale spatial mosaics of microhabitats. However, animal community composition did not vary significantly across the two main habitats. At the microhabitat level, numbers of spiders and reptiles were positively associated with woody vegetation cover. Vegetation cover did not significantly impact other taxonomic groups or overall community composition. The results show that response to microhabitats by animal populations is taxon-specific. Woody shrubs may provide a more reliable or more detectable microhabitat refuge compared to grasses for particular taxa. Informed wildlife conservation in arid lands requires investigating taxon-specific habitat and microhabitat associations, as well as the scale and persistence of these associations.
Keywords: arid, arthropods, grassland, insects, mammals, reptiles, shrubland, spiders.
Introduction
Arid lands are characterized by ecosystem processes that create substantial large-scale spatial and temporal variation in the landscape and biota. Variation is driven largely by rainfall events that are often unpredictable across space, time, and intensity in arid lands (Chesson et al. 2004; Loik et al. 2004; Van Etten 2009; Dunkerley 2012). When previously sparse areas of vegetation recover in response to precipitation, small animal populations subsequently increase, presumed to be spreading from population refuges in a spatial mosaic (Meserve et al. 1995; Ernest et al. 2000; Dickman et al. 2001; Berryman and Hawkins 2006; Haythornthwaite and Dickman 2006; Letnic and Dickman 2010; Thibault et al. 2010; Deguines et al. 2017; Pavey et al. 2017; Fischer et al. 2022). Refuges may be fixed geological or hydrological features (e.g. riparian corridors, rocky slopes, hill tops) or shifting portions of the landscape that reflect recent conditions (Milstead et al. 2007; Letnic and Dickman 2010; Pavey et al. 2017). However, we know little about the spatial scale and detectability of apparent refuges and, more broadly, the links between fine-scale spatial variation in the environment and animal communities (Pavey et al. 2017).
Spatial variation in pulses and persistence of plant growth occur at the landscape scale and at microhabitat scales (Lundholm 1976; Hamerlynck et al. 2002; Chesson et al. 2004; Li et al. 2009; Nano and Pavey 2013; Manning et al. 2020). Rainfall triggers a pulse of plant growth and release of nutrient reserves (the pulse–reserve model: Noy-Meir 1973; Reynolds et al. 2004). At the landscape scale, spatially variable rainfall interacts with soil formations (e.g. gullies and channels: Loik et al. 2004; Manning et al. 2020) that are created by aeolian or hydrological processes. Additionally, soils themselves vary in their water holding ability, further driving spatial variation in the resultant primary productivity (Chesson et al. 2004; Loik et al. 2004; Nano and Pavey 2013). Domestic grazing and fire in arid and semiarid landscapes also contribute to landscape-level variation in standing plant biomass (Letnic et al. 2005; Liu et al. 2011). At the microhabitat scale, there is local variation in soil composition, vegetative growth, seed banks and carbon stores that create mosaics of vegetation responding in a pulse of growth (Chesson et al. 2004; Nano and Pavey 2013). In addition, arid land plants often have species-specific responses to rainfall events (speed and nature of response), driving spatial variation at the microhabitat level (Lundholm 1976; Hamerlynck et al. 2002; Chesson et al. 2004; Li et al. 2009; Nano and Pavey 2013). Thus, the pulse–reserve process across a landscape with variation in abiotic and biotic features creates microhabitats of plant cover and composition as well as landscape variation in vegetative community.
Landscape-scale variation in vegetation in arid ecosystems gives rise to a spatial mosaic of animals (Ernest et al. 2000; Andrade and Monjeau 2014; Dalerum et al. 2017; Martínez et al. 2018; Marquart et al. 2020). Animal populations often rely on vegetation communities for success as vegetation provides resources or shelter (from the environment, predators or competitors: Haythornthwaite 2005; Letnic and Dickman 2010; Marquart et al. 2020). It is therefore unsurprising that many animals in arid landscapes increase in abundance following pulses in vegetation growth (Ernest et al. 2000; Stafford Smith and McAllister 2008; Thibault et al. 2010; Letnic et al. 2011; Kelly et al. 2013; Deguines et al. 2017; Burt et al. 2021; Fischer et al. 2022). Resulting spatial variation in vegetation, whether fixed or shifting, could create large-scale and small-scale refuges that allow animal populations to endure extreme and prolonged fluctuations in their environment such as drought conditions (Morton and Baynes 1985; Letnic and Dickman 2010; Letnic et al. 2011).
What remains unclear is whether ecological refuges are associated with vegetative resources. Does spatial variation in vegetation at the microhabitat level lead to detectable variation in the abundance of animals? On the one hand, many animals have very rapid life histories or high vagility, such that abundance can closely match local resource availability (Letnic and Dickman 2010), which would produce strong correlations between microhabitat patches and animal abundance. On the other hand, the responses of many animal populations occur only after a delay following changes in vegetation (Meserve et al. 1995; Ernest et al. 2000; Dickman et al. 2001; Thibault et al. 2010) or, alternatively, are ephemeral and difficult to detect without rapid repeated sampling (Maute et al. 2019; Fischer et al. 2022). In addition, some species may prefer open habitats, respond to microhabitat features other than vegetation (e.g. soil or rock crevices: Parmenter et al. 1989; Pierce et al. 1992; Berryman and Hawkins 2006; Letnic and Dickman 2010; Pulsford et al. 2017), or have adaptations that reduce their dependence on local variation in resources (e.g. retreating underground: Cloudsley-Thompson 1975; Lundholm 1976; Letnic and Dickman 2010). Moreover, trophic interactions may overwhelm the role of resource-driven patterns in determining animal abundance (Letnic and Dickman 2010; Deguines et al. 2017).
The aim of this study was to understand whether variation in habitat (rocky gibber plains versus alluvial clay plains) and microhabitat (local vegetation cover) creates spatial mosaics in animal diversity and abundance. Whereas many previous studies linking animal abundance to microhabitats have been specific in their taxonomic focus (Read 1987; Parmenter et al. 1989; Haythornthwaite 2005; Haythornthwaite and Dickman 2006; Thibault et al. 2010; but see Deguines et al. 2017) or have focused on temporal patterns (Read 1987; but see Ernest et al. 2000; Dickman et al. 2001; Haythornthwaite and Dickman 2006; Thibault et al. 2010), here we examine three major taxonomic groups of animals (invertebrates, small mammals and small reptiles) across multiple sites to examine spatial mosaics. We predicted that animal abundance and diversity would differ across the two habitats and increase with microhabitat vegetation cover.
Materials and methods
The study was conducted at the University of New South Wales Fowlers Gap Arid Zone Research Station in semiarid Australia. Fowlers Gap (31.09°S, 141.70°E, elevation ~181 m) covers 39,000 ha in semiarid NSW (Dunkerley 2013) and is characterized by chenopod shrubland and grassland plant communities. Fowlers Gap land has diverse soil formations and a complex usage history, including pastoral grazing since the 1880s. The property is split in half according to two dominant soil types (stony gibber plains and ranges, ‘Rocky’; flat alluvial clay plains, ‘Clay’), which creates large-scale habitat variation. It also offers fine-scale spatial variation in vegetation cover arising from standard arid ecosystem processes as well as the varied history of pastoral grazing. Thus, it is ideal for examining whether animal communities are linked to vegetation cover at small scales.
To address our aim, invertebrate and small vertebrate diversity and abundance were sampled in the two main geologic habitats of Fowlers Gap (Clay and Rocky) and across microhabitats that varied in vegetation cover and composition. Sampling occurred across six weeks in late austral summer (February–March 2022; one sampling season). Sixteen sampling sites were spread across the property, covering the two main habitats on Fowlers Gap equally (Fig. 1). At all sites, invertebrate sampling arrays were established. Six of the sites (three in each habitat) additionally had trapping arrays for small vertebrates. The six vertebrate sites were chosen based on availability of established traps. The 10 additional sites were selected to capture the range of apparent total vegetation cover on the property at the time of sampling, based on prior knowledge of the landscape. Thus, the sampling sites are not a random sample of total vegetation cover on Fowlers Gap or in each habitat. Three of the sites (vertebrate and invertebrate sampling arrays) were located inside grazing exclosures to include heavily vegetated microhabitats.
Map of UNSW’s Fowlers Gap Arid Zone Research Station showing trapping sites. Circles represent arrays (N = 16) of small pitfall traps that only captured invertebrates. Triangles represent arrays (N = 6) of large pitfall traps that captured vertebrates and invertebrates. Closed symbols are those in the alluvial plain clay soil; open symbols are those in the gibber rocky soils. Green boxes show three long-term grazing exclosures that were sampled.
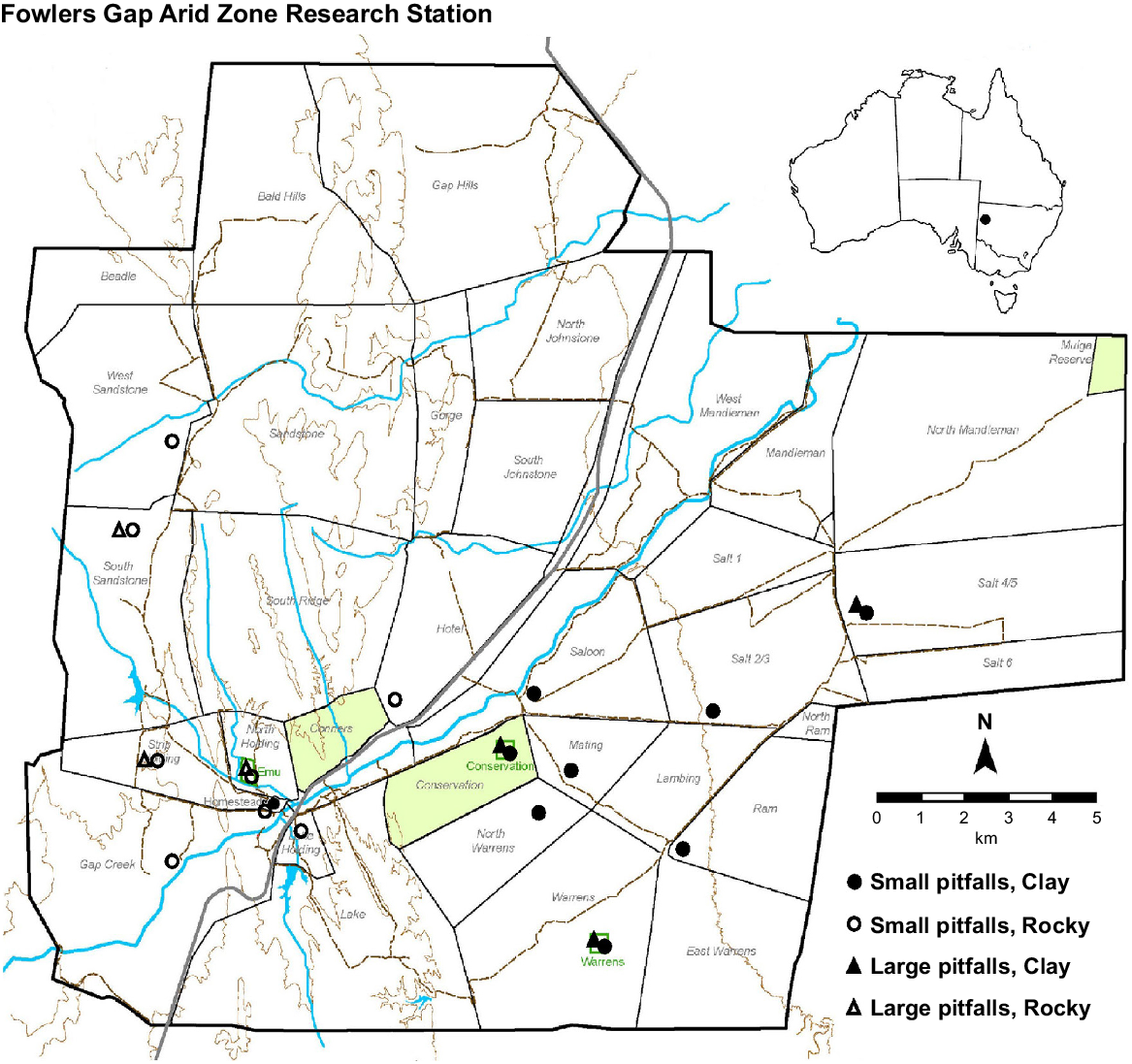
Vegetation surveys
At each site, vegetation surveys were completed by estimating vegetation ground cover along a 50 m transect. Each transect was placed parallel to and at least 50 m away from a road or fence and 50 m away from the pitfall trapping arrays to avoid disturbing animal movement within the arrays. At each 10 m mark a 1 m2 quadrat was outlined and the percentage covers of woody shrubs, grasses and forbs, and bare ground were estimated and recorded (Bonham 2013). The total vegetation cover for each quadrat was calculated by summing the woody cover and grass cover. Average vegetation cover was calculated from the five quadrats.
Animal surveys
Animals were surveyed by means of pitfall traps. Two different sizes of pitfalls were used: (1) ‘Large’ traps, which were used to catch small mammals and reptiles (also capturing invertebrates); and (2) ‘Small’ traps, which caught small invertebrates only (see trap details below). Pitfalls were sampled for four consecutive nights and days. Captured animals were recorded to species (vertebrates) or order (invertebrates). Vertebrates were marked with an organic based nail polish in a discreet spot to identify any recaptures.
Many different pitfall array designs have been used, with design possibly influencing capture success (Mendes et al. 2015). In our study, the ‘large’ pitfalls were part of 90 × 90 m arrays, made up of 12 paired pitfall traps in a 4-by-3 grid (24 traps total: Leggett et al. 2017). Each trap was a white polythene pipe (15 cm diameter; 60 cm deep: Read and Moseby 2001). At the bottom was a layer of fibreglass flyscreen to prevent animals from digging down and escaping. The paired pipes were 11 m apart, connected by a 30 cm tall aluminium drift fence. The traps were checked in the morning for nocturnal animals and in the afternoon for diurnal animals.
For our ‘small’ pitfall traps, we established 16 transect-design arrays (Uetz and Unzicker 1975). At each array, 10 small pitfalls were placed 1 m apart along a 10 m transect. Each pitfall consisted of a 70 mL sample jar that was placed flush to the ground. The small pitfalls were placed at least 50 m away from a road, fence or other pitfall array. The small sample jars were dry and had a soap rim (lemon-scented dish soap) on the inside to prevent captured invertebrates crawling out. The traps were checked every morning to record small invertebrates that were captured.
Statistical analyses
We first analysed whether vegetation cover (total vegetation, grass, and woody vegetation types) varied among sites using separate linear models for each cover class with ‘site’ as a fixed effect. We then examined whether there were significant linear relationships between total cover and woody cover, as well as total cover and grass cover across our sites, looking at both the quadrat level using raw data (linear mixed-effects models with ‘site’ as a random effect) and the site level using vegetation averages across quadrats of each site (simple linear models). We then explored whether there was a difference in each of the vegetation cover types between the Clay and Rocky habitats. Total cover was not expected to differ between habitats as sites were chosen non-randomly to capture the full range of apparent total cover. Linear mixed-effects models were fit using raw quadrat level data and including habitat as a fixed effect and site as a random effect.
To test whether (invertebrate) animal captures in small pitfalls differed according to habitat, a linear model was carried out on total captures and number of Orders per site, with ‘habitat’ as a fixed effect. This comparison could not be replicated for the large pitfalls as there was not a large enough sample size of large pitfall arrays. To determine the relationship between animal captures and vegetation cover, we focused on total invertebrate/vertebrate captures, and number of invertebrate Orders and vertebrate Families. We additionally examined captures of the most common invertebrate Orders (Coleoptera, Araneae and Hymenoptera from small pitfalls and Coleoptera, Araneae, and Orthoptera from large pitfalls: Oberprieler et al. 2019). For vertebrates, there was no family that was captured across most sites, so we focused on Class-level captures (Mammalia and Reptilia). Captures from each array were combined across trap nights. Numbers trapped in small pitfalls were adjusted to account for trapping effort (trap nights) as some of the jars at certain sites were disturbed by animals (e.g. birds), resulting in some sites having reduced trapping effort. Because there were three measures of site vegetation cover (average grass, average woody and average total cover), we performed AIC model selection for the best predictor variable. Simple linear regressions were used to analyse each response variable as a function of their AIC-preferred predictor variable, except for the number of invertebrate Orders and vertebrate Families, where generalised linear models were used to account for count data.
To analyse animal community composition, we included total captures of invertebrate Orders and vertebrate Families. However, seven taxonomic groups (four invertebrate Orders, three vertebrate Families) were excluded from community analyses of the large pitfall data because they were captured at only one or two sites, creating data that were too sparse to be included. Non-metric multidimensional scaling (NMDS) plots were created to visualise community composition across sites for both small pitfall and large pitfall data separately. ANOSIM for each pitfall array type were used to determine whether community composition differed significantly between habitat types. To examine whether differences in community composition among sites were predicted by differences in vegetation cover, a Mantel test was used for each style of pitfall array. This involved creating a ‘Bray–Curtis’ dissimilarity matrix for the animal abundance data and a ‘Euclidean’ distance matrix of the combined measures of total vegetation cover, woody cover, and grass cover.
Data analyses were performed using R ver. 4.2.0 (R Core Team 2022). All simple linear regressions were run using the lm function. To analyse count data (number of invertebrate Orders and vertebrate Families), we used the glm function with family poisson. Linear mixed-effect models were run using the lmer function within the lmerTest package (Kuznetsova et al. 2017). AIC values for competing models were extracted using the AIC function (stats package: R Core Team 2022). NMDS, ANOSIM and Mantel tests were run using the vegan package (Oksanen et al. 2022).
Results
Vegetation
The most common types of vegetation that were observed in quadrats during our sampling period were woody chenopod shrubs and grasses. The chenopods largely consisted of species from the genera Maireana, Atriplex and Sclerolaena. Plants classified as ‘grass cover’ could not be identified taxonomically due to grazing damage and lack of identifiable flowers or fruits. The 16 sampling sites varied significantly among each other in total vegetation cover (F = 2.24, P = 0.01) and grass cover (F = 2.42, P < 0.01), with a non-significant trend for sites to vary in woody cover (F = 1.68, P = 0.08, N = 80).
Across sites, total vegetation was strongly positively correlated with both woody cover and grass cover, regardless of whether this was examined using raw data from each quadrat or using vegetation averages for each site (Table 1). Most sites had a lower grass cover compared with average woody vegetation cover. None of the three vegetation cover types differed significantly between the Clay and Rocky habitats (Total cover, F = 0.28, P = 0.61; Woody cover, F = 0.38, P = 0.55; Grass cover, F = 2.28, P = 0.15; N = 80).
Response | Predictor | F, P | |
---|---|---|---|
Total cover (at quadrat scale, N = 80) | Woody cover | F = 95.66, P < 0.001 | |
Grass cover | F = 32.53, P < 0.001 | ||
Total cover (at site scale, N = 16) | Woody cover | F = 14.55, P = 0.002 | |
Grass cover | F = 10.42, P = 0.006 |
For analyses at the quadrat scale, site was included in the models as a random effect. Values in bold indicate significance at α = 0.05.
Animal captures
In total, during our summer trapping season, we captured invertebrates from 13 Orders and vertebrates from eight Families. Total number of invertebrates in small pitfalls was unrelated to habitat type when considering the number of Orders captured (F = 0.84, P = 0.38) or the total captures (F = 0.16, P = 0.69).
When looking at invertebrate captures in relation to vegetation cover, AIC model selection favored different vegetation cover variables depending on the response variable and type of pitfall array (Table 2). There were several positive relationships between invertebrate captures and vegetation cover; however, for most measures these relationships were not significant (Table 2, Figs 2 and 3). The one exception was that the number of spiders (Araneae) captured had a significant positive relationship with woody cover across the large pitfall arrays (Table 2, Fig. 3).
Pitfall | Response | Best predictor | ΔAIC | Test statistic | |
---|---|---|---|---|---|
Small (N = 16) | Invertebrate Orders | Avg grass cover | 0.339 | z = −0.83, P = 0.41 | |
Total invertebrates A | Avg total cover | 0.778 | F = 1.90, P = 0.19 | ||
Coleoptera A | Avg grass cover | 0.574 | F = 0.90, P = 0.36 | ||
Aranae A | Avg woody cover | 0.453 | F = 0.50, P = 0.49 | ||
Hymenoptera A | Avg total cover | 0.722 | F = 2.02, P = 0.18 | ||
Large (N = 6) | Invertebrate Orders | Avg woody cover | 0.018 | z = −0.22, P = 0.83 | |
Total invertebrates | Avg total cover | 0.068 | F = 0.61, P = 0.48 | ||
Coleoptera | Avg woody cover | 0.131 | F = 0.27, P = 0.63 | ||
Araneae | Avg woody cover | 5.366 | F = 10.74, P = 0.03 | ||
Orthoptera | Avg total cover | 1.649 | F = 4.82, P = 0.09 | ||
Vertebrate Families | Avg total cover | 0.353 | z = 1.19, P = 0.24 | ||
Total vertebrate captures | Avg total cover | 0.560 | F = 4.30, P = 0.11 | ||
Reptilia | Avg woody cover | 7.230 | F = 20.11, P = 0.01 | ||
Mammalia | Avg grass cover | 1.766 | F = 2.84, P = 0.17 |
Best vegetation predictors for invertebrate captures in the small pitfall traps, including total captures (a and b) and the three most common Orders (c, d and e). Counts of invertebrates are an index adjusted for uneven trapping effort (number of captures/trap nights).
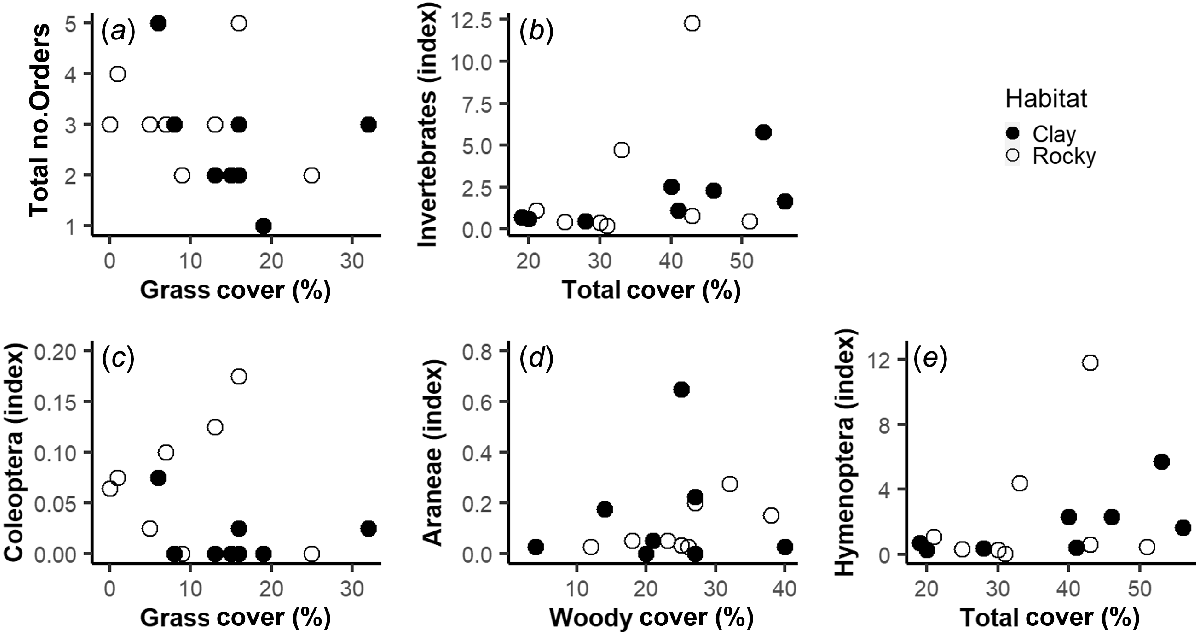
Best vegetation predictors for captures of invertebrates (a, c, e, g and i) and vertebrates (b, d, f and h) in the large pitfall traps. Included are the three most common Orders of invertebrates and two of the three vertebrate Classes captured.
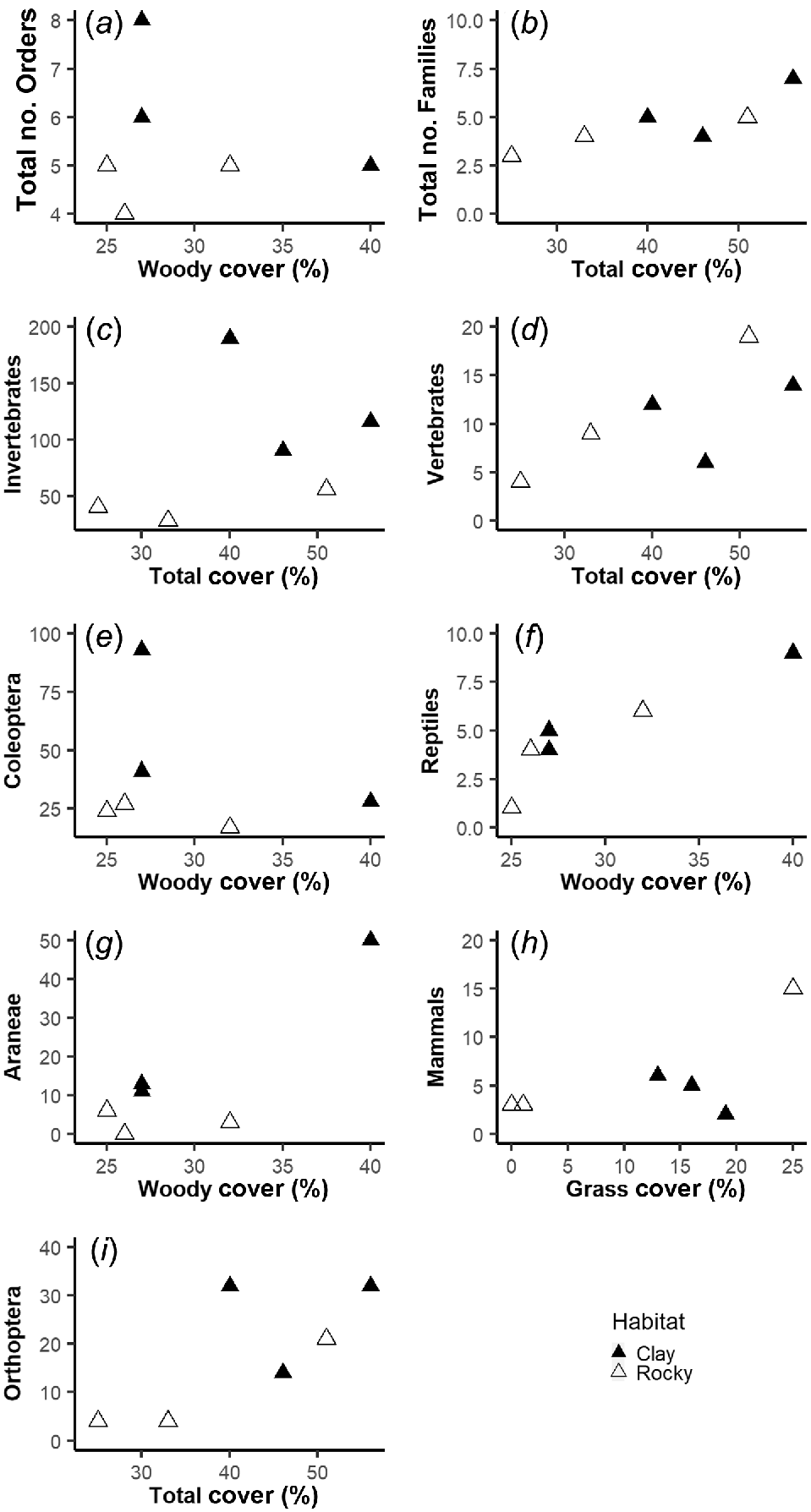
Among vertebrate captures in large pitfalls, captures of reptiles had a significant, positive relationship with woody vegetation (Table 2, Fig. 3). The number of vertebrate Families, and total captures of vertebrates and mammals showed a non-significant positive relationship with vegetation cover (Table 2).
Animal community composition
When looking at the taxonomic groups captured across trap types, there were many more Hymenoptera (ant) captures recorded in the small pitfalls than the large pitfalls (Fig. 4a, c). In addition, there appeared to be more Araneae, Isopoda, Muridae and Scincidae captured in the Clay habitat and more Dasyuridae captured in the Rocky habitat (Fig. 4b, c, d). NMDS plots of community composition showed substantial overlap in site-based community composition from small pitfalls (Fig. 4e), but little apparent overlap from large pitfalls (Fig. 4g). However, for both datasets, community composition did not differ significantly between Clay and Rocky habitats, partly owing to the small sample size for large pitfall survey sites (ANOSIM, small pitfalls: R = −0.02, P = 0.47; ANOSIM, large pitfalls: R = 0.70, P = 0.10). Similarly, differences in community composition were not significantly related to differences in the combined vegetation measures across sites (Mantel, small pitfalls: R = −0.07, P = 0.70; Mantel, large pitfalls: 0.17, P = 0.27; Fig. 4f, h).
Community composition comparison between habitats and as a function of combined vegetation measures. Invertebrates from small pitfalls are shown (a) in entirety and (b) with the overwhelming number of Hymenoptera removed. Captures from the large pitfalls are shown separately for (c) invertebrates and (d) vertebrates. Comparison of community composition sampled with the small pitfalls (e) between the two habitats (NMDS plot: filled circles, clay; open circles, rocky) and (f) as a function of dissimilarity in combined vegetation cover. Comparison of community composition sampled with the large pitfalls (g) between the two habitats (NMDS plot: filled triangles, clay; open triangles, rocky) and (h) as a function of dissimilarity in combined vegetation cover.
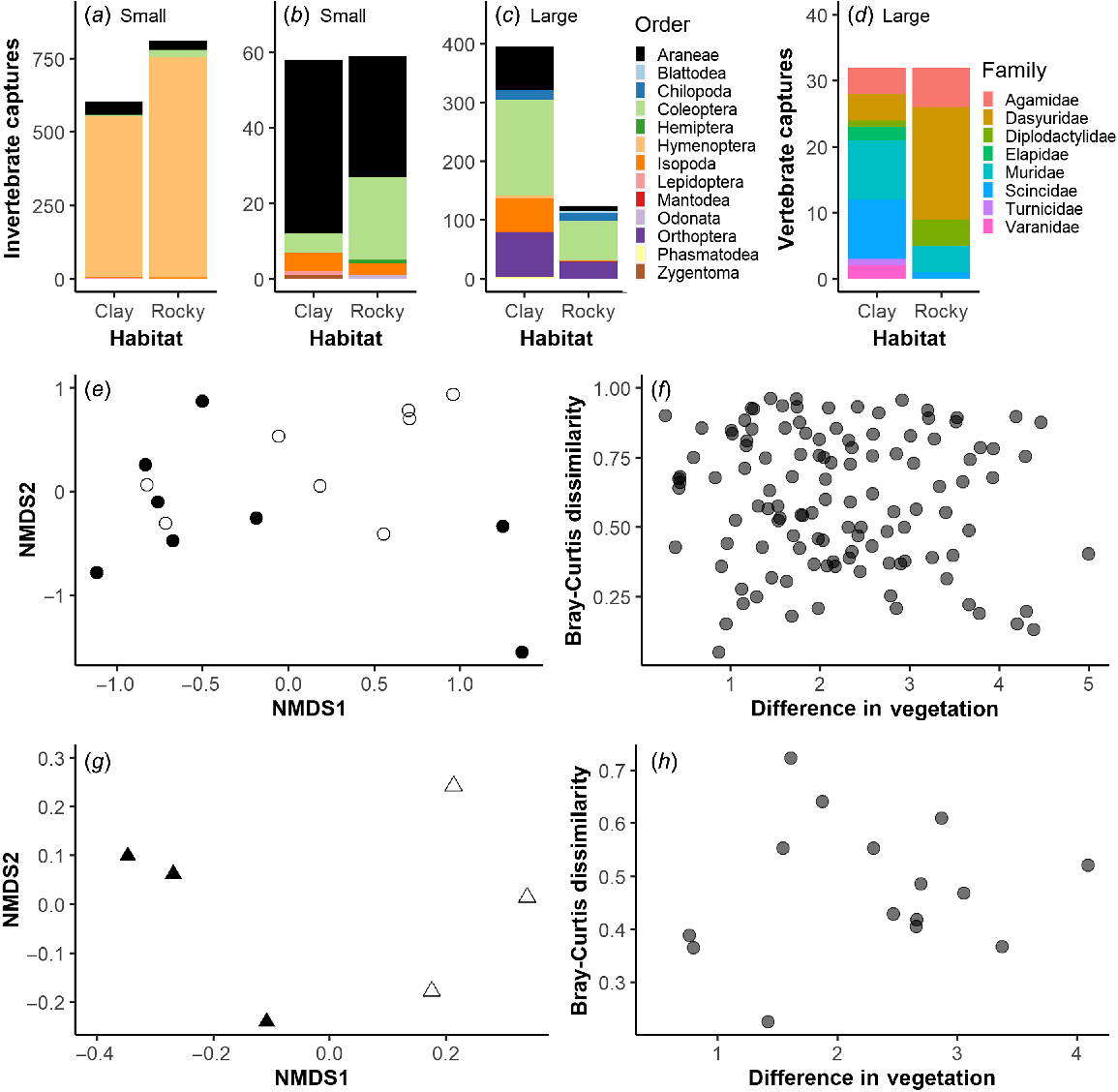
Discussion
Animal abundance and diversity in arid ecosystems is often tied to temporal pulses in resources or large-scale variation in the landscape, thought to be driven in part by spatial refuges where animal populations persist during regional resource limitation. Here, we examined whether animal abundance and composition are linked with fine-scale spatial variation in habitat and microhabitat, such that these spaces might constitute refuges. We observed substantial variation in vegetation cover (i.e. microhabitat) and animal diversity across our sampled sites, yet animal diversity was not generally linked with our measures of habitat or microhabitat.
Our animal captures were suggestive of habitat-level differences in abundance of some taxa (e.g. spiders, beetles, dasyurid marsupials, and skinks), yet we found no significant difference in animal community composition between the two habitats. Previous study of dunnarts (Dasyuridae) at Fowlers Gap found that the species have little preference between habitat types in the area (Read 1987), which is in striking contrast to our notably higher number of dasyurids in the rocky habitat. When considering invertebrates across habitats, the large pitfalls captured many fewer invertebrates in the Rocky habitat compared with the Clay habitat, yet the relative abundance of the major Orders was similar (see also Oberprieler et al. 2019). The difference in overall captures is unlikely to be related to the low detectability of small invertebrates (particularly ants) in large pitfalls, as this trapping bias would impact captures in the two habitats equally. More likely, the increased abundance of marsupial predators in the large pitfall traps in the Rocky habitat led to the associated reduction in invertebrates. Indeed, captures from small pitfall traps had an overall similar number of captures between the two habitats but possibly fewer spiders and more beetles in the Rocky habitat compared with the Clay habitat. In arid lands more broadly, small animal communities have previously been found to vary across major habitats in arid lands (Ernest et al. 2000; Milstead et al. 2007; Dalerum et al. 2017; Martínez et al. 2018; Marquart et al. 2020). Thus, additional investigation is warranted before ruling out habitat-level differences between clay soils and gibber soils in our study area.
At a smaller spatial scale, we sampled across a patchy mosaic of vegetation microhabitats, which predicted the abundance of a subset of our taxa. Greater woody vegetation cover was associated with more spiders (Araneae) and reptiles, though these patterns should be confirmed with a larger sample. Woody vegetation in arid lands provides a stable resource of shelter and food. In particular, spiders in arid and woodland settings typically prefer woody habitat and litter (Whitehouse et al. 2002; Castro and Wise 2009). Similarly, many types of invertebrates (as well as small mammals) are more common near stable vegetation regardless of whether it is grass or woody vegetation (Read 1987; Haythornthwaite 2005; Marquart et al. 2020). Reptiles often benefit from woody debris and woody vegetation, as wood provides thermoregulatory opportunities, retreats from predators and increased termites for food (Morton and James 1988; Pike et al. 2011; Pulsford et al. 2017; Braun et al. 2024). Additionally, the soil around woody plants typically holds moisture for longer periods and acts as a source of water for invertebrates (Hamerlynck et al. 2002; Manning et al. 2020), providing a stable source of food for predators like spiders and reptiles. Thus, our results suggest that woody chenopod shrubs form the foundation of microhabitat mosaics for certain taxa and potentially support population persistence as relatively fixed refuges during droughts.
Despite the suggestive patterns seen in spiders and reptiles, other measures of animal abundance and communities were unrelated to microhabitat vegetation cover. This may reflect true limitations on the extent to which vegetation cover provides population refuges. For example, preferences and sensitivities with respect to habitat and microhabitat are often species-specific and may not be linked to vegetation cover per se (Haythornthwaite and Dickman 2006; Pavey et al. 2017; Lu et al. 2021; Hernandez-Santin and Fisher 2022). In arid Australia, dasyurids show species-specific responses to rainfall and vegetation, with captures sometimes negatively related to microhabitat grass cover (e.g. preferring recently burned sites) or seemingly unrelated to habitat measures from multiple spatial scales (Masters 1993; Haythornthwaite and Dickman 2006; Hernandez-Santin and Fisher 2022). Similarly, previous work at Fowlers Gap performed across a smaller spatial extent than the present study suggested that captures of small dasyurid marsupials are linked to microhabitat features such as vegetation height and density of soil cracks but not to general habitat characteristics (Read 1987). Among invertebrates, percentage grass cover has been linked strongly to populations of herbivores and omnivores but not predators (such as spiders) and detritivores (Marquart et al. 2020; Fischer et al. 2022; Heimbuch et al. 2023). In addition, invertebrate populations in semiarid grasslands have been found to be highly sensitive to plant quality (i.e. leaf nutrient content) and new growth after fire, with less of a role for total biomass (Lu et al. 2021; Heimbuch et al. 2023). Moreover, diversity and abundance of some taxa may also be influenced strongly by predation, competitive exclusion, and their interaction with vegetation (Brown and Munger 1985; Letnic and Dickman 2010; Atwood et al. 2011; Dickman 2014; Terborgh 2015; Deguines et al. 2017).
On the other hand, true links between vegetation cover and animals are challenging to detect in arid lands due to the unpredictable and ephemeral nature of resource availability. In our study, Fowlers Gap received rainfall in the weeks before sampling, and there was already a high abundance of grass and forbs present when vegetation cover was estimated. Although animals in arid lands typically respond to ‘greening’ of vegetation (Hobbs et al. 2008; Letnic and Dickman 2010; Fischer et al. 2022), the timing of the response is variable and we sampled over a short timescale. Vertebrate population numbers exhibit a substantial delay to greening (~1 year) (e.g. Meserve et al. 1995; Dickman et al. 2001; Thibault et al. 2010). Thus, current vegetation cover, particularly fast-growing grasses and forbs, is unlikely to be a good predictor of concurrent vertebrate communities (Dickman et al. 2001). On the other hand, invertebrate populations respond quickly to rainfall (<1 month) and grass growth, yet the effect may be detectable only in specific, limited time windows after rain (Fischer et al. 2022). For example, at Fowlers Gap, invertebrate captures have been found to increase dramatically within two weeks after rainfall, but numbers of only some taxa remained elevated three months later (Maute et al. 2019). These challenges in detecting links between microhabitat characteristics and animal abundance may explain why only woody cover had explanatory power in our study. Persistent vegetation such as woody chenopod shrubs responds to rainfall more slowly (Nano and Pavey 2013; Manning et al. 2020) such that populations of animal species that are sensitive to woody cover may be more likely to maintain numerical associations with woody cover. Importantly, invertebrate taxa that use woody vegetation as an important microhabitat are less likely to be captured with pitfalls and instead require bush beating (e.g. Martinez et al. 2021). Recording invertebrate and vertebrate assemblages at a finer taxonomic scale (e.g. genera or species) and across multiple sampling periods could help tease apart the temporal dynamics and niche variation underlying potential links between population abundance and vegetation.
Refuges are also challenging in a spatial context. Populations of small animals may require a larger spatial extent of favourable habitat than the scale of microhabitats that we sampled. In addition, by definition, we may expect refuges to be rare (Milstead et al. 2007), so they may be difficult to detect in the standard ecological sampling framework. Finally, it is possible that refuges are not places where taxa persist more abundantly during periods of low resource availability but rather places where growth can happen rapidly under the right conditions. Identifying animal refuges in arid settings may often require far greater spatial and temporal scales of sampling than are typically possible in most studies (Ernest et al. 2000; Haythornthwaite and Dickman 2006).
Overall, our results suggest that refuges were not strongly apparent in our study. However, the implications of our findings for arid-zone mosaics and refuges should be viewed in light of the limited temporal scale of the study. Spatial mosaics in animals existed in our study but linking them to driving forces may require understanding vegetation and animal communities over time. Multiple well-timed trapping events are required to gain further knowledge of the relationship between vegetation cover (habitat and microhabitat scales) and animal community composition, abundance, and diversity. This type of monitoring will provide insight in the future as Fowlers Gap has recently removed grazing sheep and will be experiencing altered grazing pressures. Diversity in arid landscapes is often strongly associated with grazing pressure in arid lands (Debano 2006; Liu et al. 2011), so changes to plant and animal communities will be expected. Hopefully, the current study will provide a baseline for future surveys of diversity and abundance following the removal of domestic grazers.
Declaration of funding
The research was funded by the School of Biological, Earth and Environmental Sciences and Fowlers Gap Arid Zone Research Station (UNSW).
Acknowledgements
Fieldwork assistance was provided by Lewis Loncar. The research was approved by the UNSW Animal Ethics Committee (AEC 21/103A). Statistical advice was provided by Claudia Crowther, Rebecca Raynal, Davey Dor, Lewis Loncar and Daphne Willemsen. The manuscript was improved by comments from M. Kasumovic and M. Letnic.
References
Andrade A, Monjeau A (2014) Patterns in community assemblage and species richness of small mammals across an altitudinal gradient in semi-arid Patagonia, Argentina. Journal of Arid Environments 106, 18-26.
| Crossref | Google Scholar |
Atwood TC, Fry TL, Leland BR (2011) Partitioning of anthropogenic watering sites by desert carnivores. The Journal of Wildlife Management 75(7), 1609-1615.
| Crossref | Google Scholar |
Berryman AA, Hawkins BA (2006) The refuge as an integrating concept in ecology and evolution. Oikos 115(1), 192-196.
| Crossref | Google Scholar |
Braun S, Ritchie EG, Doherty TS, Nimmo DG (2024) The red fox (Vulpes vulpes) is the dominant predator of lizard models in a semi-arid landscape, and predation risk is reduced by vegetation cover. Austral Ecology 49, e13530.
| Crossref | Google Scholar |
Brown JH, Munger JC (1985) Experimental manipulation of a desert rodent community: food addition and species removal. Ecology 66(5), 1545-1563.
| Crossref | Google Scholar |
Burt C, Fritz H, Keith M, Guerbois C, Venter JA (2021) Assessing different methods for measuring mammal diversity in two southern African arid ecosystems. Mammal Research 66, 313-326.
| Crossref | Google Scholar |
Castro A, Wise DH (2009) Influence of fine woody debris on spider diversity and community structure in forest leaf litter. Biodiversity and Conservation 18, 3705-3731.
| Crossref | Google Scholar |
Chesson P, Gebauer RLE, Schwinning S, Huntly N, Wiegand K, Ernest MSK, Sher A, Novoplansky A, Weltzin JF (2004) Resource pulses, species interactions, and diversity maintenance in arid and semi-arid environments. Oecologia 141, 236-253.
| Crossref | Google Scholar | PubMed |
Cloudsley-Thompson JL (1975) Adaptations of arthropoda to arid environments. Annual Review of Entomology 20, 261-283.
| Crossref | Google Scholar | PubMed |
Dalerum F, de Vries JL, Pirk CWW, Cameron EZ (2017) Spatial and temporal dimensions to the taxonomic diversity of arthropods in an arid grassland savannah. Journal of Arid Environments 144, 21-30.
| Crossref | Google Scholar |
Debano SJ (2006) Effects of livestock grazing on aboveground insect communities in semi-arid grasslands of southeastern Arizona. Biodiversity & Conservation 15, 2547-2564.
| Crossref | Google Scholar |
Deguines N, Brashares JS, Prugh LR (2017) Precipitation alters interactions in a grassland ecological community. Journal of Animal Ecology 86(2), 262-272.
| Crossref | Google Scholar | PubMed |
Dickman CR, Haythornthwaite AS, McNaught GH, Mahon PS, Tamayo B, Letnic M (2001) Population dynamics of three species of dasyurid marsupials in arid central Australia: a 10-year study. Wildlife Research 28(5), 493-506.
| Crossref | Google Scholar |
Dunkerley D (2012) Effects of rainfall intensity fluctuations on infiltration and runoff: rainfall simulation on dryland soils, Fowlers Gap, Australia. Hydrological Processes 26(15), 2211-2224.
| Crossref | Google Scholar |
Dunkerley D (2013) Sub-daily rainfall events in an arid environment with marked climate variability: variation among wet and dry years at Fowlers Gap, New South Wales, Australia. Journal of Arid Environments 96, 23-30.
| Crossref | Google Scholar |
Ernest SKM, Brown JH, Parmenter RR (2000) Rodents, plants, and precipitation: spatial and temporal dynamics of consumers and resources. Oikos 88(3), 470-482.
| Crossref | Google Scholar |
Fischer C, Gerstmeier R, Wagner TC (2022) Seasonal and temporal patterns of rainfall shape arthropod community composition and multi-trophic interactions in an arid environment. Scientific Reports 12, 3742.
| Crossref | Google Scholar | PubMed |
Hamerlynck EP, McAuliffe JR, McDonald EV, Smith SD (2002) Ecological responses of two Mojave Desert shrubs to soil horizon development and soil water dynamics. Ecology 83(3), 768-779.
| Crossref | Google Scholar |
Haythornthwaite AS (2005) Microhabitat use and foraging behaviour of Sminthopsis youngsoni (Marsupialia: Dasyuridae) in arid central Australia. Wildlife Research 32(7), 609-615.
| Crossref | Google Scholar |
Haythornthwaite AS, Dickman CR (2006) Distribution, abundance, and individual strategies: a multi-scale analysis of dasyurid marsupials in arid central Australia. Ecography 29(3), 285-300.
| Crossref | Google Scholar |
Heimbuch NG, McGranahan DA, Wonkka CL, Vermeire LT, Branson DH (2023) Grasshopper abundance and offtake increase after prescribed fire in semi-arid grassland. International Journal of Wildland Fire 32(12), 1828-1833.
| Crossref | Google Scholar |
Hernandez-Santin L, Fisher DO (2022) Community structure of dasyurid marsupials in the arid Pilbara is consistent with a top-down system, their distribution and abundance depend on that of larger members of the guild. Journal of Arid Environments 198, 104680.
| Crossref | Google Scholar |
Hobbs NT, Reid RS, Galvin KA, Ellis JE (2008) Fragmentation of arid and semi-arid ecosystems: implications for people and animals. In ‘Fragmentation in semi-arid and arid landscapes’. (Eds KA Galvin, RS Reid, RH Behnke Jr, NT Hobbs) pp. 25–44. (Springer: Dordrecht, Netherlands) 10.1007/978-1-4020-4906-4_2
Kelly LT, Dayman R, Nimmo DG, Clarke MF, Bennett AF (2013) Spatial and temporal drivers of small mammal distributions in a semi-arid environment: the role of rainfall, vegetation and life-history. Austral Ecology 38(7), 786-797.
| Crossref | Google Scholar |
Kuznetsova A, Brockhoff PB, Christensen RHB (2017) lmerTest package: tests in linear mixed effects models. Journal of Statistical Software 82(13), 1-26.
| Crossref | Google Scholar |
Leggett KEA, Welaratne T, Letnic M, McLeod S, Dawson T (2017) Rediscovery of the plains mouse (Pseudomys australis) (Rodentia: Muridae) in New South Wales. Australian Mammalogy 40(1), 127-130.
| Crossref | Google Scholar |
Letnic M, Dickman CR (2010) Resource pulses and mammalian dynamics: conceptual models for hummock grasslands and other Australian desert habitats. Biological Reviews 85(3), 501-521.
| Crossref | Google Scholar | PubMed |
Letnic M, Tamayo B, Dickman CR (2005) The responses of mammals to La Niña (El Niño Southern Oscillation)–associated rainfall, predation, and wildfire in central Australia. Journal of Mammalogy 86(4), 689-703.
| Crossref | Google Scholar |
Letnic M, Story P, Story G, Field J, Brown O, Dickman CR (2011) Resource pulses, switching trophic control, and the dynamics of small mammal assemblages in arid Australia. Journal of Mammalogy 92(6), 1210-1222.
| Crossref | Google Scholar |
Li XR, Tan HJ, He MZ, Wang XP, Li XJ (2009) Patterns of shrub species richness and abundance in relation to environmental factors on the Alxa Plateau: prerequisites for conserving shrub diversity in extreme arid desert regions. Science in China Series D: Earth Sciences 52, 669-680.
| Crossref | Google Scholar |
Liu Y, Pan Q, Liu H, Bai Y, Simmons M, Dittert K, Han X (2011) Plant responses following grazing removal at different stocking rates in an Inner Mongolia grassland ecosystem. Plant and Soil 340, 199-213.
| Crossref | Google Scholar |
Loik ME, Breshears DD, Lauenroth WK, Belnap J (2004) A multi-scale perspective of water pulses in dryland ecosystems: climatology and ecohydrology of the western USA. Oecologia 141(2), 269-281.
| Crossref | Google Scholar | PubMed |
Lu X, Zhao X, Tachibana T, Uchida K, Sasaki T, Bai Y (2021) Plant quantity and quality regulate the diversity of arthropod communities in a semi-arid grassland. Functional Ecology 35(3), 601-613.
| Crossref | Google Scholar |
Lundholm B (1976) Adaptations in arid ecosystems. Ecological Bulletins 24, 19-27.
| Google Scholar |
Manning A, Julian JP, Doyle MW (2020) Riparian vegetation as an indicator of stream channel presence and connectivity in arid environments. Journal of Arid Environments 178, 104167.
| Crossref | Google Scholar |
Marquart A, Geissler K, Heblack J, Lobas C, Münch E, Blaum N (2020) Individual shrubs, large scale grass cover and seasonal rainfall explain invertebrate-derived macropore density in a semi-arid Namibian savanna. Journal of Arid Environments 176, 104101.
| Crossref | Google Scholar |
Martínez FJ, Cheli GH, Pazos GE (2018) Structure of ground-dwelling arthropod assemblages in vegetation units of Área Natural Protegida Península Valdés, Patagonia, Argentina. Journal of Insect Conservation 22, 287-301.
| Crossref | Google Scholar |
Martínez FJ, Dellapé PM, Bisigato AJ, Cheli GH (2021) Native shrubs and their importance for arthropod diversity in the southern Monte, Patagonia, Argentina. Journal of Insect Conservation 25, 27-38.
| Crossref | Google Scholar |
Masters P (1993) The effects of fire-driven succession and rainfall on small mammals in spinifex grassland at Uluru National Park, Northern Territory. Wildlife Research 20(6), 803-813.
| Crossref | Google Scholar |
Maute K, Hose GC, Story P, Bull CM, French K (2019) Surviving drought: a framework for understanding animal responses to small rain events in the arid zone. Ecology 100(11), e02884.
| Crossref | Google Scholar | PubMed |
Mendes DM, Leão RdF, Toledo LF (2015) Drift fences in traps: theoretical evidence of effectiveness of the two most common arrays applied to terrestrial tetrapods. Natureza & Conservação 13(1), 60-66.
| Crossref | Google Scholar |
Meserve PL, Yunger JA, Gutiérrez JR, Contreras LC, Milstead WB, Lang BK, Cramer KL, Herrera S, Lagos VO, Silva SI, Tabilo EL, Torrealba M-A, Jaksic FM (1995) Heterogeneous responses of small mammals to an El Niño Southern Oscillation Event in northcentral semiarid Chile and the importance of ecological scale. Journal of Mammalogy 76(2), 580-595.
| Crossref | Google Scholar |
Milstead WB, Meserve PL, Campanella A, Previtali MA, Kelt DA, Gutierrez JR (2007) Spatial ecology of small mammals in north-central Chile: role of precipitation and refuges. Journal of Mammalogy 88(6), 1532-1538.
| Crossref | Google Scholar |
Morton SR, Baynes A (1985) Small mammal assemblages in arid Australia: a reappraisal. Australian Mammalogy 8(3), 159-169.
| Crossref | Google Scholar |
Morton SR, James CD (1988) The diversity and abundance of lizards in arid Australia: a new hypothesis. The American Naturalist 132(2), 237-256.
| Crossref | Google Scholar |
Nano CEM, Pavey CR (2013) Refining the ‘pulse–reserve’ model for arid central Australia: Seasonal rainfall, soil moisture and plant productivity in sand ridge and stony plain habitats of the Simpson Desert. Austral Ecology 38(7), 741-753.
| Crossref | Google Scholar |
Noy-Meir I (1973) Desert ecosystems: environment and producers. Annual Review of Ecology, Evolution, and Systematics 4, 25-51.
| Crossref | Google Scholar |
Oberprieler SK, Andersen AN, Braby MF (2019) Invertebrate by-catch from vertebrate pitfall traps can be useful for documenting patterns of invertebrate diversity. Journal of Insect Conservation 23, 547-554.
| Crossref | Google Scholar |
Oksanen J, Simpson GL, Blanchet FG, Kindt R, Legendre P, Minchin PR, O’Hara RB, Solymos P, Stevens MHM, Szoecs E, Wagner H, Barbour M, Bedward M, Bolker B, Borcard D, Carvalho G, Chirico M, De Caceres M, Durand S, Evangelista HBA, FitzJohn R, Friendly M, Furneaux B, Hannigan G, Hill MO, Lahti L, McGlinn D, Ouellette M-H, Ribeiro Cunha E, Smith T, Stier A, Ter Braak CJF, Weedon J (2022) Vegan: Community Ecology Package. R package version 2.6-4. Available at https://CRAN.R-project.org/package=vegan
Parmenter RR, Parmenter CA, Cheney CD (1989) Factors influencing microhabitat partitioning among coexisting species of arid-land darkling beetles (Tenebrionidae): behavioral responses to vegetation architecture. The Southwestern Naturalist 34(3), 319-329.
| Crossref | Google Scholar |
Pavey CR, Addison J, Brandle R, Dickman CR, McDonald PJ, Moseby KE, Young LI (2017) The role of refuges in the persistence of Australian dryland mammals. Biological Reviews 92(2), 647-664.
| Crossref | Google Scholar | PubMed |
Pierce BM, Longland WS, Jenkins SH (1992) Rattlesnake predation on desert rodents: microhabitat and species-specific effects on risk. Journal of Mammalogy 73(4), 859-865.
| Crossref | Google Scholar |
Pike DA, Webb JK, Shine R (2011) Removing forest canopy cover restores a reptile assemblage. Ecological Applications 21(1), 274-280.
| Crossref | Google Scholar | PubMed |
Pulsford SA, Driscoll DA, Barton PS, Lindenmayer DB (2017) Remnant vegetation, plantings and fences are beneficial for reptiles in agricultural landscapes. Journal of Applied Ecology 54(6), 1710-1719.
| Crossref | Google Scholar |
R Core Team (2022) R: A language and environment for statistical computing. R Foundation for Statistical Computing, Vienna, Austria. Available at https://www.R-project.org/
Read DG (1987) Habitat use by Sminthopsis crassicaudata, Planigale gilesi and Planigale tenuirostris (Marsupialia, Dasyuridae) in semiarid New South Wales. Australian Wildlife Research 14(4), 385-395.
| Crossref | Google Scholar |
Read JL, Moseby KE (2001) Factors affecting pitfall capture rates of small ground vertebrates in arid South Australia. I. The influence of weather and moon phase on capture rates of reptiles. Wildlife Research 28(1), 53-60.
| Crossref | Google Scholar |
Reynolds JF, Kemp PR, Ogle K, Fernández RJ (2004) Modifying the ‘pulse–reserve’ paradigm for deserts of North America: precipitation pulses, soil water, and plant responses. Oecologia 141, 194-210.
| Crossref | Google Scholar | PubMed |
Stafford Smith M, McAllister RRJ (2008) Managing arid zone natural resources in Australia for spatial and temporal variability – An approach from first principles. The Rangeland Journal 30(1), 15-27.
| Crossref | Google Scholar |
Terborgh JW (2015) Toward a trophic theory of species diversity. Proceedings of the National Academy of Sciences 112(37), 11415-11422.
| Crossref | Google Scholar | PubMed |
Thibault KM, Ernest SKM, White EP, Brown JH, Goheen JR (2010) Long-term insights into the influence of precipitation on community dynamics in desert rodents. Journal of Mammalogy 91(4), 787-797.
| Crossref | Google Scholar |
Uetz GW, Unzicker JD (1975) Pitfall trapping in ecological studies of wandering spiders. The Journal of Arachnology 3(2), 101-111.
| Google Scholar |
Van Etten EJB (2009) Inter-annual rainfall variability of arid Australia: greater than elsewhere? Australian Geographer 40(1), 109-120.
| Crossref | Google Scholar |
Whitehouse MEA, Shochat E, Shachak M, Lubin Y (2002) The influence of scale and patchiness on spider diversity in a semi-arid environment. Ecography 25(4), 395-404.
| Crossref | Google Scholar |