Controlling feral ruminants to reduce greenhouse gas emissions: a case study of buffalo in northern Australia
Hugh F. Davies



A Research Institute for the Environment and Livelihoods, Charles Darwin University, Casuarina, NT 0810, Australia.
Wildlife Research 50(11) 899-910 https://doi.org/10.1071/WR22134
Submitted: 5 August 2022 Accepted: 2 December 2022 Published: 5 January 2023
© 2023 The Author(s) (or their employer(s)). Published by CSIRO Publishing. This is an open access article distributed under the Creative Commons Attribution-NonCommercial-NoDerivatives 4.0 International License (CC BY-NC-ND)
Abstract
Context: The bourgeoning carbon economy is creating novel ways to incentivise conservation management activities that have the co-benefits of reducing greenhouse gas (GHG) emissions and social inequality.
Aim: To estimate the monetary value of carbon credits that landowners could generate by reducing ecologically destructive feral populations of the Asian water buffalo (Bubalus bubalis) in northern Australia.
Methods: First, we estimated buffalo enteric emissions based on the population structure of feral buffalo in northern Australia, and discounted the reduction of fire emissions due to the consumption of grassy fuel by feral buffalo. We then predicted the change in buffalo population size across the South Alligator River region of Kakadu National Park under four buffalo management scenarios: (1) no buffalo control; (2) low-intensity buffalo control; (3) moderate-intensity buffalo control; and (4) high-intensity buffalo control. We quantified the reduction of GHG emissions under the three buffalo control scenarios, relative to the scenario of no buffalo control, while discounting the GHG emissions that directly result from buffalo control actions (e.g. helicopter emissions).
Key results: All three buffalo control scenarios substantially reduced the estimated GHG emissions that would otherwise have been produced. The low-intensity buffalo control scenario was predicted to abate 790 513 t CO2-e over the 20-year simulation, worth USD15 076 085 (or USD753 804 year−1). Our high-intensity buffalo control scenario had the greatest reduction in GHG emissions, with a total net abatement of 913 231 t CO2-e, worth USD17 176 437 (or USD858 822 year−1).
Conclusions: The potential value of carbon credits generated by controlling feral buffalo populations in northern Australian savannas far exceeds the management costs.
Implications: The management of feral ruminants could be incentivised by the generation of carbon credits. Such management could simultaneously avoid GHG emissions, generate income for landowners and offer significant ecological benefits.
Keywords: carbon credits, climate change, conservation, feral herbivores, greenhouse gas emissions, northern Australia, ruminants, tropical savanna.
Introduction
In response to the increasingly evident effects of anthropogenic climate change, there is growing impetus to reduce greenhouse gas (GHG) emissions. Stemming from global commitments to reduce GHG emissions made under various treaties (i.e. Kyoto Protocol, Paris Agreement), the pricing of GHG pollution has emerged as a financial mechanism to incentivise GHG emissions reduction (Bradshaw et al. 2013). This has created new ways to incentivise conservation management activities that demonstrably reduce net GHG emissions. For example, the Australian Government’s legislated offset scheme, the Emissions Reduction Fund (ERF), provides a financial basis for fire management across northern Australia’s fire-prone tropical savannas via a carbon-crediting mechanism (Russell-Smith et al. 2013). The approved ‘savanna burning’ methodology allows land managers to earn an Australian Carbon Credit Unit (ACCU) for each (net) tonne of carbon dioxide equivalent (CO2-e) prevented from release into the atmosphere (Corey et al. 2020). ACCUs can then be sold to third parties looking to offset their emissions. Such fire management programs now cover more than 380 000 km2 of northern Australia and are delivering important economic, social and ecological benefits (Russell-Smith et al. 2013; Edwards et al. 2021).
Given the scale of GHG emissions from the Australian agricultural sector, programs to reduce emissions associated with the management of livestock represent a key component of the ERF. In contrast, there has been little attention paid to the potential development of a methodology to incentivise the control of feral herbivores (Bradshaw et al. 2013). This is despite the devastating impacts that numerous species of feral herbivore have had on Australian ecosystems. Importantly, Australia is home to large feral populations of ruminants, including camel (Camelus dromedarius), cattle (Bos taurus, Bos indicus), buffalo (Bubalus bubalis), goat (Capra hircus) and numerous deer species (Rusa unicolor, Dama dama, Cervus elephus, Axis axis, Axis porcinus, Rusa timorensis). Ruminant digestion can produce large amounts of methane (CH4), a potent GHG. For example, an individual camel or buffalo produces >50 kg CH4 year−1, equivalent to >1.25 t CO2 year−1 (Bradshaw et al. 2013).
Quantifying the GHG emissions abatement that can be achieved through the management of populations of feral ruminants could lead to a market-based mechanism to incentivise their ongoing management. Although previous research has investigated the change in GHG emissions as a result of feral camel management in Australia (Drucker et al. 2010; Zeng 2015), to our knowledge no such work has focused on feral buffalo. Here we investigate the potential monetary value of carbon credits that could be generated by reducing feral buffalo populations in northern Australia. We focus on a region where the population dynamics of feral buffalo and the costs of their control have been well documented: the South Alligator River region of Kakadu National Park. We aimed to:
-
Estimate the enteric emissions from feral buffalo;
-
Use population models and published costs of buffalo control to investigate the net value of GHG abatement of feral buffalo control.
Materials and methods
Study system
Our study focused on the South Alligator River region of Kakadu National Park, 250 km east of Darwin in northern Australia (Fig. 1). This region experiences a tropical monsoonal climate with an intense wet season (November–April) followed by a dry season (May–October). Mean annual rainfall is ~1500 mm. The major vegetation types are lowland savanna (characterised by moderately dense trees of Eucalyptus miniata and Eucalyptus tetrodonta, with a grassy understorey), as well as floodplain and Melaleuca forest.
![]() |
Buffalo in northern Australia
The buffalo (B. bubalis) was introduced to northern Australia in the 19th century, and by the 1980s had reached densities as high as 34 km−2 on the South Alligator River floodplains, and 15 km−2 in the region’s lowland savannas (Ridpath et al. 1983). Following the commencement of a major control programme in the late-1980s (the Brucellosis and Tuberculosis Eradication Campaign [BTEC]), buffalo densities decreased dramatically to <0.1 km−2 by the mid-1990s (Skeat et al. 1996). Although there have been numerous small-scale buffalo control operations since the cessation of BTEC in 1995, there has been no large-scale, coordinated control of buffalo. High buffalo densities cause significant ecological impacts, including a reduction in vegetation biomass, changes to species composition, soil compaction and erosion, changes to surface hydrology including the intrusion of saltwater into freshwater swamps, and reduced water quality (Skeat et al. 1996; Werner 2005). It has been suggested that these impacts drive long-term ecological cascades (Petty et al. 2007), and there is growing evidence of the role of large feral herbivores, such as buffalo, in the widespread collapse of northern Australian native mammal populations (Legge et al. 2019; Davies et al. 2020; Stobo-Wilson et al. 2020). However, it is important to note that the well documented collapse of native mammal populations in Kakadu National Park coincided with the BTEC (Woinarski et al. 2001), with mammal decline continuing despite the massive reduction in densities of feral herbivores.
Estimating enteric emissions from feral buffalo
We calculated the net GHG emissions from feral buffalo populations using estimates of buffalo enteric emissions (CH4), adjusted for both buffalo population structure and the reduced emissions from fire in lowland savanna (CH4 and N2O) resulting from the reduction of grassy fuel loads due to buffalo consumption. The emissions of carbon dioxide (CO2) were not accounted for based on the assumption that savanna fires produce no net CO2 flux. All GHG emissions were converted to a CO2 equivalent (i.e. standardising for the global warming potential of each gas), expressed in units of t CO2-e year−1.
Enteric emissions (Ee)
The enteric emissions produced by ruminants are determined by a number of factors, including the rate and volume of feed intake, and the conversion rate of feed energy to CH4 (Calvo Buendia et al. 2019). These factors depend on feed quality (with lower-quality feed generally having higher rates of conversion to CH4) as well as the demographic structure of the ruminant population, which determines the feed biomass consumed (e.g. the proportion of adults and juveniles, reproductive stages, weight etc.). In absence of the detailed information required to estimate CH4 emissions from feral buffalo across northern Australia using higher-tier Intergovernmental Panel on Climate Change (IPCC) methods (i.e. Tiers 2 and 3), we used the Tier 1 method (Calvo Buendia et al. 2019). Tier 1 methods use default emissions factors based on previous studies, across different regions. Our analysis is based on the estimate that enteric emissions from an individual adult buffalo contributes 76 kg CH4 year−1, equivalent to 2.1 t CO2 year−1.
Adjusting Ee for population structure
Because feral buffalo populations in northern Australia include both adults and juveniles, we adjusted the estimate of buffalo enteric emissions based on the structure of these populations. To estimate the relative proportion of juveniles and adults, we used the buffalo age frequency distributions from four northern Australian buffalo populations harvested between 2006 and 2008 by McMahon et al. (2011). Because buffalo in northern Australia become reproductively active between 2 and 3 years of age (Tulloch and Grassia 1981), we classified juvenile buffalo as those <2 years of age, and adult buffalo as those ≥2 years of age. On average across the four populations, the proportion of juveniles (Pj) and adults (Pa) was 43% and 57% respectively. We acknowledge that the accuracy of this population structure is contingent on an unbiased sampling protocol, but note that they are likely reliable because entire family groups were culled at similar times of the year (McMahon et al. 2011). We assumed that a juvenile buffalo produces half the enteric emissions of an adult (38 kg CH4 year−1, equivalent to 1.05 t CO2-e year−1).
We estimated the enteric emissions (Ee) from northern Australian feral buffalo populations as:

where D is the population density of feral buffalo, Pj is the proportion of the buffalo population that are juveniles (0.43), Ej is the annual enteric emissions of juvenile buffalo (1.05 t CO2-e year−1), Pa is the proportion of the buffalo population that are adults (0.57), Ej is the annual enteric emissions of adult buffalo (2.1 t CO2-e year−1).
Accounting for the reduction in fire emissions from lowland savanna due to the consumption of grassy fuel by feral buffalo
Feral buffalo can consume large amounts of herbaceous plant biomass, particularly grasses (Bowman et al. 2010). As a result, they have the potential to reduce fuel loads across savanna landscapes, thereby reducing GHG emissions due to fire. We accounted for this by estimating the net emissions from feral buffalo in lowland savanna (Es) as:

where Ee is estimated enteric emissions (see above), and Ef is estimated reduction in fire emissions due to the consumption of grassy fuels by buffalo in northern Australian lowland savannas. Because adult and juvenile buffalo consume different amounts of vegetation, we again adjusted our estimates based on feral buffalo population structure (outlined above) such that:

where Efba is the estimated emissions from fire in the absence of feral buffalo, Efjb is the estimated emissions from fire at a given density of juvenile buffalo, and Efab is the estimated emissions from fire at a given density of adult buffalo.
Following the methods outlined by Cook et al. (2016), we estimated the emissions from a typical lowland savanna fire regime in the absence of feral buffalo (Efba). First, we estimated the mean proportion of fuel remaining after a fire under a particular fire regime, R, as:
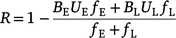
where BE and BL are the mean burning efficiencies in the early and late dry season respectively, UE and UL is the burn uniformity (i.e. the proportion of area within a fire scar that remains unburnt) in the early and late dry season respectively, and FE and FL is the mean fire frequency in the early and late dry season respectively (Cook et al. 2016). These values were chosen to represent a typical lowland savanna fire regime (Table 1).
![]() |
We then calculated the mean fire return interval, r, as:

The maximum grass fuel load, Φmax, was calculated as:

where L is grass fuel load and k is the grass turnover rate.
The mean post-fire residue (i.e. dead organic matter remaining after a fire), Φ(0), was:
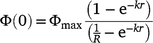
The mean fuel load when a fire occurs, Φr, was:

The mean mass of fuel emitted as gas, Eϕ, was:

The mean annual emissions of methane, , was then calculated as:

where is the emission factor for methane, γ is the fuel carbon content and 1.333 is the molecular to elemental mass ratio for CH4.
The mean annual emissions of N2O, , was then calculated as:

where is the emission factor for N2O, γ is the fuel carbon content, 1.571 is the molecular to elemental mass ratio for N2O, and NC is the fuel nitrogen to carbon ratio.
The total fire emissions in the absence of feral buffalo (Efba) was then calculated as:

where and
are the global warming potentials of CH4 and N2O, respectively, expressed relative to CO2 (28 and 265 respectively).
To estimate the change in emissions due to the consumption of grassy fuel by buffalo, we estimated the emissions from a typical lowland savanna fire regime in the presence of juvenile (Efjb) and adult (Efab) buffalo. To do this, we followed the same approach as outlined above, but reduced the maximum grass fuel load estimate based on the amount of grass consumed by buffalos, such that:
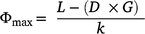
where D is the population density of juvenile or adult buffalo, and G is the amount of grass consumed per individual juvenile or adult buffalo (t ha−1 year−1).
Estimates of the daily consumption of vegetation by adult feral buffalo range from around 4–6 kg day−1 (Williams and Dudzinski 1982; Williams and Ridpath 1982), to as high as 30 kg day−1 (Jesser et al. 2016). To ensure our estimate of buffalo emissions was conservative, we used 30 kg day−1 when estimating the reduction in fire emissions due to the consumption of vegetation by adult buffalo. We assumed that consumption by juvenile buffalo was half of adult consumption (i.e. 15 kg day−1). We note here that our approach assumes buffalo are only eating grass (not browsing on woody plants), that the reduction in fire emissions from buffalo consumption in habitats other than lowland savanna (i.e. floodplains) are negligible, and that fire intensity remains constant. The latter assumption is similar to other GHG accounting approaches for Australian savannas, which assume that fire intensity is not affected by reductions in fire frequency (Russell-Smith et al. 2009; Cook et al. 2016). All inputs are summarised in Table 1.
Combining our estimate of enteric emissions with spatially explicit buffalo population models
To gauge the potential of greenhouse gas abatement of feral buffalo control, we combined our estimates of Ee and Es with spatially explicit population models to contextualise the potential emission reductions within a realistic management framework. Importantly, our management scenarios were costed, thereby permitting us to gauge whether the potential economic benefit of reducing buffalo emissions (by generating and selling carbon credits) outweigh the cost of applying such management. To do this, we explored the net value of GHG abatement from feral buffalo control under different management scenarios across the South Alligator River region of Kakadu National Park.
Buffalo population model inputs
We used the Spatio-Temporal Animal Reduction (STAR) model, developed by McMahon et al. (2010) for several feral species in Kakadu National Park, to predict buffalo population change across Kakadu’s South Alligator River region. We examined four scenarios over a 20-year period: (1) no buffalo control; (2) low-intensity buffalo control; (3) moderate-intensity buffalo control; and (4) high-intensity buffalo control. We based our management scenarios on the pre-specified management scenarios designed by McMahon et al. (2010) to examine how populations will change over time with no management actions (i.e. no buffalo control scenario), and those designed to reduce feral buffalo densities to specified target levels (i.e. our low-, moderate- and high-intensity buffalo control scenarios). Our model inputs are summarised in Table 2. We used the same population demographic parameters (i.e. carrying capacity, maximum intrinsic population growth rate, growth response shape parameter and dispersal probability) as outlined in McMahon et al. (2010). We estimated an initial buffalo density of 0.93 km−2. This estimate was based on the density estimate across Kakadu in 1996 (0.1 km−2, Skeat et al. 1996), adjusted for the habitat types across the South Alligator River region, and an annual population growth rate of 5.25% (Saalfeld 2014). Given the absence of a robust estimate of current buffalo density, this estimate represents our ‘best guess’ but is potentially inaccurate. For our culling scenarios, we adjusted the logistical costs associated with aerial culling based on a recently quoted price for aerial buffalo culling in comparable habitat. The cost of helicopter hire was quoted as USD937 h−1, and other costs (including labour, ammunition, food, accommodation, reporting and administration) as USD356 h−1.
![]() |
Combining population model outputs with estimates of enteric emissions to estimate potential carbon credits
Our population model outputs included the predicted change in buffalo population size across the South Alligator River under our four buffalo management scenarios, as well as the number of animals culled each year, the annual number of helicopter hours required, and an estimated annual cost of management.
Using our estimates of buffalo enteric emissions, we quantified the GHG emissions from buffalo enteric emissions across the South Alligator River region for each of our management scenarios. To do this, we multiplied our estimates of buffalo enteric emissions (t CO2-e km−1 year−1) for the predicted density of buffalo (buffalo km−1) across the entire South Alligator River region. Given the different habitat types, we multiplied Es across the 2300 km2 of lowland savanna, and Ee across the 3000 km2 of floodplain, wetlands, and paperbark forest. The coarse spatial scale of the model (100 km2) may reduce its ability for accurate predictions on which to base management actions; however, it still offers a useful heuristic tool for the purposes of this study (McMahon et al. 2010). We also acknowledge that this approach assumes an even density of buffalo across the lowland savannas and other habitat types of the South Alligator River region. Because buffalo density may be higher in floodplain areas (compared with savanna), this may overestimate the reduction of GHG emissions from fire across the region, thus producing a conservative estimate of the potential GHG abatement from buffalo management.
To gauge the potential GHG abatement from our buffalo management scenarios, we subtracted our estimates of annual buffalo enteric emissions across the South Alligator River region (Fig. 2) – predicted by our low, moderate and high buffalo control scenarios – from our no buffalo control scenario. As such, our annual GHG abatement estimates were quantified against the shifting baseline of buffalo population growth predicted under no buffalo control. This differs from established savanna burning methodology where a baseline estimate of emissions is quantified over a period of no fire management, against which the annual GHG abatement from fire management is calculated.
Once we had quantified the reduction in GHG emissions arising from our buffalo control scenarios, we estimated the net value of abatement. We did this by first calculating the dollar value for the amount of GHG emissions abatement using the current market price of around USD22 for an Australian carbon credit unit (ACCU; equivalent to 1 t CO2-e stored or avoided by a project) (https://www.renewableenergyhub.com.au/market-prices/ accessed on 17/3/2022). To account for the cost of applying each management scenario, we subtracted the estimated cost of applying each management scenario.
To accurately quantify the net value of GHG abatement we also needed to estimate the GHG emitted during the application of management actions. Culling feral animals from aircraft is an effective, commonly used approach in northern Australian landscapes. To account for the GHG emissions released during our buffalo control scenarios, we used the relationship between feral buffalo density and the helicopter flying time required per buffalo killed (McMahon et al. 2010) (Fig. 3). We used this relationship to combine the annual buffalo density, and number of individual buffalo culled each year under our management scenarios, to calculate the total annual helicopter hours. We then combined the number of helicopter hours with information on fuel consumption rate for a suitable helicopter model (Bell 206 Jetranger) of 120 L h−1 (Department of Environment and Conservation 2011) to calculate the total fuel consumed each year. We then combined the total amount of fuel (L) consumed each year with the emission factors per litre consumed of the fuel type (Jet A1 kerosene-type jet fuel: 2.58 kg CO2-e L−1) for this aircraft (Solomon et al. 2007) to estimate the annual amount of GHG emissions from each buffalo control scenario. Our analysis was guided by the Australian Government-approved methods for determining the abatement of greenhouse gas from savanna fire management (Commonwealth of Australia 2015, 2018), and therefore did not include emissions from the extraction, refinement or transportation of fuel.
![]() |
We were then able to calculate the net value of abatement as: the estimated dollar amount of ACCUs generated through the reduction in buffalo enteric GHG emissions (compared to no management) minus the cost of each management scenario, minus the dollar amount of ACCUs lost due to the emissions of GHG during control operations.
Results
Estimated enteric emissions from feral buffalo
By combining IPCC estimates of buffalo enteric emissions, with information of buffalo population structure, we established the relationship between buffalo density and the estimated enteric emissions from buffalo (Ee) (Fig. 4a). To estimate the net emissions from buffalo in lowland savanna, we accounted for the reduction in fire emissions due the consumption of grassy fuels by buffalo (Fig. 4b).
Combining our estimate of enteric emissions with buffalo population models
With no population control, the number of feral buffalo across the South Alligator River region of Kakadu National Park increased from 4942 to 61 541 individuals (Fig. 5). This increase in abundance corresponds to an estimated increase in annual GHG emissions from 7792 t CO2-e in the first year, to 97 282 t CO2-e by the 20th year, totalling 951 703 t CO2-e emitted over the 20-year simulation. This predicted population trajectory (and associated emissions) sets the baseline scenario against which we estimated the potential value of abatement from our three feral buffalo management scenarios.
Under our low-intensity buffalo control scenario, the buffalo population increased from 4942 to 7673 individuals, remaining well below that predicted with no population control (Fig. 5). The population reduction achieved by our low-intensity buffalo control scenario was estimated to reduce the total amount of emissions by 791 158 t CO2-e. Our low-intensity buffalo control scenario cost a total of USD2 337 953. This scenario required a total of 2080 helicopter hours, resulting in the emission of 645 t CO2-e, and an estimated net abatement of 790 513 t CO2-e. This abatement equates to USD17 399 189 worth of carbon credits. Subtracting the estimated total cost of implementing our low-intensity buffalo control scenario resulted in a net potential value of abatement over 20 years of USD15 061 236 (or USD753 062 year−1) (Fig. 6).
![]() |
Under our moderate-intensity buffalo control scenario, buffalo population size decreased from 4942 to 3699 individuals (Fig. 5), abating 870 844 t CO2-e for a total cost of USD2 657 097. This management scenario required 2503 helicopter hours, estimated to emit 776 t CO2-e. For this scenario, we estimated a net abatement of 870 068 t CO2-e, equal to USD19 150 197 worth of carbon credits. Subtracting the estimated total cost of implementing our moderate-intensity buffalo control scenario resulted in a net potential value of abatement of USD16 493 100 (or USD824 655 year−1) (Fig. 6).
Our high-intensity buffalo control scenario achieved the largest reduction in feral buffalo population size (Fig. 5), an estimated abatement of 914 248 t CO2-e, and cost a total of USD2 940 695. This management scenario required a total of 3277 helicopter hours, emitting a total of 1017 t CO2-e, resulting in an estimated net abatement of 913 231 t CO2-e equal to USD20 100 214 worth of carbon credits. The total net potential value of abatement for this scenario was USD17 159 519 (or USD857 976 year−1) (Fig. 6).
For our three buffalo management scenarios, we predicted that the potential value of abatement would outweigh the costs and emissions of conducting feral buffalo management within 4 years (Fig. 6).
Discussion
The bourgeoning carbon economy is creating novel ways to incentivise conservation management activities that demonstrably avoid greenhouse gas (GHG) emissions. We have demonstrated that the potential economic value of carbon credits generated by reducing feral buffalo populations in northern Australia far outweighs the costs of management. Our estimates of GHG abatement represents an important demonstration that the management of feral ruminants could be incentivised by the generation of carbon credits in a similar way to savanna fire management. Such management would not only avoid GHG emissions while generating income for landowners, but it also has the potential to offer important ecological benefits, especially when applied in concert with fire management.
All three of our buffalo management scenarios constrained buffalo population size well below that predicted under no management. In doing so, all three scenarios substantially reduced the estimated buffalo enteric GHG emissions that would otherwise have been produced. Initially, the cost of applying each buffalo management scenario outweighed the potential net value from GHG abatement. However, we estimated that by the fourth year, the economic potential of the GHG abatement achieved under all three management scenarios would outweigh management costs. Even our low-intensity buffalo control scenario was predicted to have large economic returns, abating 790 513 t CO2-e over 20 years, with the estimated net potential value of abatement being USD15 061 236 over 20 years (or USD753 062 year−1). Although our high-intensity buffalo control scenario had the largest initial investment, as well as higher annual management costs and emissions, it had the highest potential economic return due to the greatest reduction in buffalo enteric GHG emissions, totalling a net abatement of 913 231 t CO2-e over the 20-year simulation, potentially worth USD17 159 519 (or USD857 976 year−1).
Our estimates of GHG abatement from feral buffalo control provide a noteworthy comparison to the demonstrated GHG abatement currently being achieved under intensive fire management programs across northern Australian savannas. For example, by increasing the proportion of fires occurring under the benign fire conditions of the early dry season (April–July), the West Arnhem Land Fire Abatement (WALFA) project has delivered a mean annual emissions reduction of 116 968 t CO2-e, while also delivering social, biodiversity and long-term biomass sequestration benefits (Russell-Smith et al. 2013). Comparatively, the maximum estimated annual net GHG abatement in our analysis was 92 594 t CO2-e, recorded in the 20th year of the high-intensity buffalo control scenario. However, the WALFA project area (28 000 km2) is over five times larger than the South Alligator area (5300 km2). When this difference in area is accounted for, the GHG abatement recorded from fire management at WALFA is 4.2 t CO2-e km2, whereas our estimated potential GHG abatement from feral buffalo control across the South Alligator River region could be as high as 17.5 t CO2-e km2. The simultaneous management of fire and feral buffalo could substantially increase the amount of GHG emissions abatement and potential economic gain for landowners.
Our estimates of emissions abatement were quantified against the predicted growth of the buffalo population under no control. Under our ‘no buffalo control’ scenario, the predicted population size remained comparable to previous observations of buffalo population size across the South Alligator River region (Freeland and Boulton 1990; Skeat et al. 1996). Therefore, in this circumstance, we can be confident in the magnitude of our estimated abatement. Regardless, our use of a predicted baseline, rather than an empirically observed baseline, has important implications. It may be risky when applying this method to other areas without robust observations of the maximum size of the buffalo population (i.e. at carrying capacity) in that particular area. Such uncertainty may reduce confidence in the estimated abatement and impact on the ability to sell carbon credits. Another option might be to quantify the emissions abatement from buffalo control against a robust region-specific baseline buffalo density. However, if the buffalo population happened to be well below carrying capacity at the time the baseline estimate was established, this approach would underestimate the true magnitude of emissions abatement. Establishing a defensible, but widely applicable, baseline is one of the difficulties that must be addressed when developing a methodology for generating carbon credits through the management of feral ruminants.
There is evidence that severe disturbance regimes, characterised by frequent high-severity fires, and/or heavy grazing by feral herbivores, have disrupted savanna processes across northern Australia (Skeat et al. 1996; Legge et al. 2019; Stobo-Wilson et al. 2020). Currently, there is now an economic incentive driving improved fire management across more than 380 000 km2 of northern Australia, facilitated by the approved ‘savanna burning’ accounting methodology under the Australian Government’s Emissions Reduction Fund (Corey et al. 2020; Edwards et al. 2021). Unfortunately, such an incentive does not yet exist for the active management of large feral herbivores across northern Australia. This is despite research demonstrating the need for the concurrent management of both fire and feral herbivores for the conservation of native wildlife (Legge et al. 2019). As such, an economic incentive for feral herbivore control across northern Australia (similar to that currently incentivising fire management) could help maximise the economic and ecological benefits of these programs.
Because fire is influenced by a range of factors including weather, rainfall, fuel loads, and ignition points, the amount of GHG emissions abatement achieved through fire management can vary from year to year (Russell-Smith et al. 2013). This potentially poses issues when delivering an emissions abatement to meet contractual obligations (Evans and Russell-Smith 2019). On the other hand, populations of large feral ruminants do not experience large, unpredictable annual fluctuations, resulting in more predictable GHG emissions abatement and reliable income stream for landowners (notwithstanding fluctuations in the price of carbon). However, generating income from GHG abatement via the control of feral herbivores, such as buffalo, requires a range of important considerations. First, there are important animal welfare and ethical considerations that have the potential to influence the economic viability of programs generating carbon credits from the control of feral animals. The culling of feral animals can be highly controversial (Hagis and Gillespie 2021), which could potentially impact on the ability to sell carbon credits generated from the control of feral animals. On the other hand, there can be beneficial animal welfare outcomes from the humane culling of animals suffering from malnutrition and disease (Hampton and Forsyth 2016).
A second critical consideration is the diverse range of stakeholders and values engrained in buffalo exploitation and management across northern Australia, including those of Indigenous people, conservationists, pastoralists and trophy shooters (Albrecht et al. 2009). We note that feral herbivore eradication is not a logistically feasible option for most areas of northern Australia (apart from offshore islands and peninsulas), nor is it likely to be supported across such diverse stakeholders (Albrecht et al. 2009). As such, programs aiming to avoid GHG emissions through the sustained control of feral animals should be developed with broad consultation across stakeholder groups. Such stakeholder consultation could underpin the spatiotemporal application of control measures, thereby reducing conflict and maximising potential benefits.
The GHG emissions reduction from the control of feral ruminants is not included in accounting methodologies approved as part of the Emissions Reduction Fund, Australia’s national carbon offset program. However, because projects aimed at reducing domestic livestock emissions are included, and given the potential economic and ecological benefits, the development of an accounting methodology that incentivises the control of feral ruminants is a conceivable next step (Bradshaw et al. 2013). Before this can happen, targeted research aimed at addressing remaining uncertainties is needed. Our study represents an important proof of concept, demonstrating that the economic potential of carbon credits generated from the control of feral buffalo populations far outweighs the cost of management. Importantly, this is true despite our study investigating a relatively small fraction of the total GHG emissions from large feral ruminants. For example, large feral animals substantially increase GHG emissions via impacts on soil, and disturbance of wetlands and floodplains (Limpert et al. 2021). Much research is still needed to understand the magnitude of these fluxes to accurately quantify the disturbance to these GHG emission pathways caused by feral ruminants. While we estimated the direct enteric emissions from only one species in northern Australia, the same approach could be applied to other species across Australia, including cattle, camels, goats, and deer.
Our results are based on Tier 1 Intergovernmental Panel on Climate Change (IPCC) estimates of buffalo enteric emissions (Calvo Buendia et al. 2019). The uncertainty of these estimates is an important limitation of our study, and future research should aim to refine estimates of GHG emissions from feral ruminant species, including how they vary throughout their lifespan. Our study did not account for the GHG emissions from decomposing carcasses. Although this source of GHG emissions may be negligible compared with those produced by a living animal (Zeng 2015), decomposing carcasses could provide supplementary food for predators (Forsyth et al. 2014). This could have important ecological implications that counteract the expected conservation benefits from feral ruminant management.
The impacts of global climate change are becoming increasingly evident, and motivating new, innovative methods to reduce and offset anthropogenic GHG emissions. The development of the carbon economy has created new ways to incentivise conservation management activities that demonstrably avoid greenhouse gas (GHG) emissions. A monetary incentive for the management of feral ruminants could offer substantial reductions in GHG emissions, while providing important economic and ecological outcomes.
Data availability
This manuscript did not involve the collection of new data. All data used in this manuscript are freely available from the referenced sources.
Conflicts of interest
The authors declare no conflicts of interest.
Declaration of funding
This research was supported by funding from the Australian Government through the Australian Research Council (LP170100305).
Author contributions
Georgina Neave and Hugh Davies conceived the idea. All authors designed the methodology and collated data. Hugh Davies led the analyses and writing. All authors contributed critically to the drafts and gave final approval for publication.
Acknowledgements
We thank Sarah Legge and Jarrad Holmes for constructive comments on an earlier draft.
References
Albrecht, G, Mcmahon, CR, Bowman, DMJS, and Bradshaw, CJA (2009). Convergence of culture, ecology, and ethics: management of feral swamp buffalo in northern Australia. Journal of Agricultural and Environmental Ethics 22, 361–378.| Convergence of culture, ecology, and ethics: management of feral swamp buffalo in northern Australia.Crossref | GoogleScholarGoogle Scholar |
Bowman, DMJS, Murphy, BP, and Mcmahon, CR (2010). Using carbon isotope analysis of the diet of two introduced Australian megaherbivores to understand Pleistocene megafaunal extinctions. Journal of Biogeography 37, 499–505.
| Using carbon isotope analysis of the diet of two introduced Australian megaherbivores to understand Pleistocene megafaunal extinctions.Crossref | GoogleScholarGoogle Scholar |
Bradshaw, CJA, Bowman, DMJS, Bond, NR, Murphy, BP, Moore, AD, Fordham, DA, Thackway, R, Lawes, MJ, McCallum, H, Gregory, SD, Dalal, RC, Boer, MM, Lynch, AJJ, Bradstock, RA, Brook, BW, Henry, BK, Hunt, LP, Fisher, DO, Hunter, D, Johnson, CN, Keith, DA, Lefroy, EC, Penman, TD, Meyer, WS, Thomson, JR, Thornton, CM, VanDerWal, J, Williams, RJ, Keniger, L, and Specht, A (2013). Brave new green world – consequences of a carbon economy for the conservation of Australian biodiversity. Biological Conservation 161, 71–90.
| Brave new green world – consequences of a carbon economy for the conservation of Australian biodiversity.Crossref | GoogleScholarGoogle Scholar |
Calvo Buendia E, Tanabe K, Kranjc A, Baasansuren J, Fukuda M, Ngarize S, Osako A, Pyrozhenko Y, Shermanau P, Federici S (2019) 2019 refinement to the 2006 IPCC guidelines for national greenhouse gas inventories. (IPCC: Gland, Switzerland) Available at https://www.ipcc.ch/report/2019-refinement-to-the-2006-ipcc-guidelines-for-national-greenhouse-gas-inventories/
Commonwealth of Australia (2015) Carbon credits (carbon farming initiative – emissions abatement through savanna fire management) methodology determination 2015. (Australian Government: Canberra, ACT, Australia)
Commonwealth of Australia (2018) Carbon credits (carbon farming initiative – savanna fire management – emissions avoidance) methodology determination 2018. (Australian Government: Canberra. ACT, Australia)
Cook, GD, Meyer, CP (Mick), Muepu, M, and Liedloff, AC (2016). Dead organic matter and the dynamics of carbon and greenhouse gas emissions in frequently burnt savannas. International Journal of Wildland Fire 25, 1252–1263.
| Dead organic matter and the dynamics of carbon and greenhouse gas emissions in frequently burnt savannas.Crossref | GoogleScholarGoogle Scholar |
Corey, B, Andersen, AN, Legge, S, Woinarski, JCZ, Radford, IJ, and Perry, JJ (2020). Better biodiversity accounting is needed to prevent bioperversity and maximize co-benefits from savanna burning. Conservation Letters 13, e12685.
| Better biodiversity accounting is needed to prevent bioperversity and maximize co-benefits from savanna burning.Crossref | GoogleScholarGoogle Scholar |
Davies, HF, Maier, SW, and Murphy, BP (2020). Feral cats are more abundant under severe disturbance regimes in an Australian tropical savanna. Wildlife Research 47, 624–632.
| Feral cats are more abundant under severe disturbance regimes in an Australian tropical savanna.Crossref | GoogleScholarGoogle Scholar |
Department of Environment and Conservation (2011) Fire management services aircraft factsheet. Perth. Available at https://www.dpaw.wa.gov.au/images/conservation-management/fire/aircraft/AircraftFactSheetBell206Jetranger.pdf [Accessed 6 July 2021]
Drucker, AG, Edwards, GP, and Saalfeld, WK (2010). Economics of camel control in central Australia. The Rangeland Journal 32, 117–127.
| Economics of camel control in central Australia.Crossref | GoogleScholarGoogle Scholar |
Edwards, A, Archer, R, De Bruyn, P, Evans, J, Lewis, B, Vigilante, T, Whyte, S, and Russell-Smith, J (2021). Transforming fire management in northern Australia through successful implementation of savanna burning emissions reductions projects. Journal of Environmental Management 290, 112568.
| Transforming fire management in northern Australia through successful implementation of savanna burning emissions reductions projects.Crossref | GoogleScholarGoogle Scholar |
Evans, J, and Russell-Smith, J (2019). Delivering effective savanna fire management for defined biodiversity conservation outcomes: an Arnhem Land case study. International Journal of Wildland Fire 29, 386–400.
| Delivering effective savanna fire management for defined biodiversity conservation outcomes: an Arnhem Land case study.Crossref | GoogleScholarGoogle Scholar |
Forsyth, DM, Woodford, L, Moloney, PD, Hampton, JO, Woolnough, AP, and Tucker, M (2014). How does a carnivore guild utilise a substantial but unpredictable anthropogenic food source? Scavenging on hunter-shot ungulate carcasses by wild dogs/dingoes, red foxes and feral cats in south-eastern Australia revealed by camera traps. PLoS ONE 9, e97937.
| How does a carnivore guild utilise a substantial but unpredictable anthropogenic food source? Scavenging on hunter-shot ungulate carcasses by wild dogs/dingoes, red foxes and feral cats in south-eastern Australia revealed by camera traps.Crossref | GoogleScholarGoogle Scholar |
Freeland, WJ, and Boulton, WJ (1990). Feral water buffalo (Bubalus bubalis) in the major floodplains of the Top End, Northern Territory, Australia – population growth and the brucellosis and tuberculosis eradication campaign. Australian Wildlife Research 17, 411–420.
| Feral water buffalo (Bubalus bubalis) in the major floodplains of the Top End, Northern Territory, Australia – population growth and the brucellosis and tuberculosis eradication campaign.Crossref | GoogleScholarGoogle Scholar |
Hagis, E, and Gillespie, J (2021). Kosciuszko National Park, Brumbies, law and ecological justice. Australian Geographer 52, 225–241.
| Kosciuszko National Park, Brumbies, law and ecological justice.Crossref | GoogleScholarGoogle Scholar |
Hampton, JO, and Forsyth, DM (2016). An assessment of animal welfare for the culling of peri-urban kangaroos. Wildlife Research 43, 261–266.
| An assessment of animal welfare for the culling of peri-urban kangaroos.Crossref | GoogleScholarGoogle Scholar |
Jesser P, Markula A, Csurhes S (2016) Invasive animal risk assessment: water buffalo. Bubalus bubalis. Queensland Department of Agriculture and Fisheries Biosecurity, Brisbane, Qld, Australia. Available at https://www.daf.qld.gov.au/__data/assets/pdf_file/0018/62640/IPA-Water-Buffalo-Risk-Assessment.pdf
Legge, S, Smith, JG, James, A, Tuft, KD, Webb, T, and Woinarski, JCZ (2019). Interactions among threats affect conservation management outcomes: livestock grazing removes the benefits of fire management for small mammals in Australian tropical savannas. Conservation Science and Practice 1, e52.
| Interactions among threats affect conservation management outcomes: livestock grazing removes the benefits of fire management for small mammals in Australian tropical savannas.Crossref | GoogleScholarGoogle Scholar |
Limpert, KE, Carnell, PE, and Macreadie, PI (2021). Managing agricultural grazing to enhance the carbon sequestration capacity of freshwater wetlands. Wetlands Ecology and Management 29, 231–244.
| Managing agricultural grazing to enhance the carbon sequestration capacity of freshwater wetlands.Crossref | GoogleScholarGoogle Scholar |
McMahon, CR, Brook, BW, Collier, N, and Bradshaw, CJA (2010). Spatially explicit spreadsheet modelling for optimising the efficiency of reducing invasive animal density. Methods in Ecology and Evolution 1, 53–68.
| Spatially explicit spreadsheet modelling for optimising the efficiency of reducing invasive animal density.Crossref | GoogleScholarGoogle Scholar |
McMahon, CR, Brook, BW, Bowman, DMJS, Williamson, GJ, and Bradshaw, CJA (2011). Fertility partially drives the relative success of two introduced bovines (Bubalus bubalis and Bos javanicus) in the Australian tropics. Wildlife Research 38, 386–395.
| Fertility partially drives the relative success of two introduced bovines (Bubalus bubalis and Bos javanicus) in the Australian tropics.Crossref | GoogleScholarGoogle Scholar |
Petty, AM, Werner, PA, Lehmann, CER, Riley, JE, Banfai, DS, and Elliott, LP (2007). Savanna responses to feral buffalo in Kakadu National Park, Australia. Ecological Monographs 77, 441–463.
| Savanna responses to feral buffalo in Kakadu National Park, Australia.Crossref | GoogleScholarGoogle Scholar |
Ridpath, MG, Begg, RJ, Dudzinski, ML, Forbes, MA, and Graham, A (1983). Counting the same populations of large tropical mammals from the ground and from the air. Wildlife Research 10, 487–498.
| Counting the same populations of large tropical mammals from the ground and from the air.Crossref | GoogleScholarGoogle Scholar |
Russell-Smith, J, Murphy, BP, Meyer, CP (Mick), Cook, GD, Maier, S, Edwards, AC, Schatz, J, and Brocklehurst, P (2009). Improving estimates of savanna burning emissions for greenhouse accounting in northern Australia: limitations, challenges, applications. International Journal of Wildland Fire 18, 1–18.
| Improving estimates of savanna burning emissions for greenhouse accounting in northern Australia: limitations, challenges, applications.Crossref | GoogleScholarGoogle Scholar |
Russell-Smith, J, Cook, GD, Cooke, PM, Edwards, AC, Lendrum, M, Meyer, CP (Mick), and Whitehead, PJ (2013). Managing fire regimes in north Australian savannas: applying Aboriginal approaches to contemporary global problems. Frontiers in Ecology and the Environment 11, e55–e63.
| Managing fire regimes in north Australian savannas: applying Aboriginal approaches to contemporary global problems.Crossref | GoogleScholarGoogle Scholar |
Saalfeld K (2014) Feral buffalo (Bubalus bubalis): distribution and abundance in Arnhem Land, Northern Territory. NT Department of Land Resource Management, Darwin, NT, Australia.
Skeat AJ, East TJ, Corbett LK (1996) Impact of feral water buffalo. In ‘Landscape and Vegetation Ecology of the Kakadu Region, Northern Australia’. (Eds CM Finlayson, I Von Oertzen) pp. 155–177. (Springer: Dordrecht, The Netherlands)
Solomon S, Qin D, Manning M, Marquis M, Averyt K, Tignor MMB, LeRoy Miller H, Chen Z (2007) Climate change 2007 – the physical science basis. Contribution of Working Group I to the fourth assessment report of the Intergovernmental Panel on Climate Change. (Cambridge University Press: Cambridge, UK) Available at https://www.ipcc.ch/report/ar4/wg1/
Stobo-Wilson, AM, Stokeld, D, Einoder, LD, Davies, HF, Fisher, A, Hill, BM, Mahney, T, Murphy, BP, Scroggie, MP, Stevens, A, Woinarski, JCZ, Bawinanga Rangers Warddeken Rangers Gillespie, GR (2020). Bottom-up and top-down processes influence contemporary patterns of mammal species richness in Australia’s monsoonal tropics. Biological Conservation 247, 108638.
| Bottom-up and top-down processes influence contemporary patterns of mammal species richness in Australia’s monsoonal tropics.Crossref | GoogleScholarGoogle Scholar |
Tulloch, DG, and Grassia, A (1981). A study of reproduction in water buffalo in the Northern Territory of Australia. Wildlife Research 8, 335–348.
| A study of reproduction in water buffalo in the Northern Territory of Australia.Crossref | GoogleScholarGoogle Scholar |
Werner, PA (2005). Impact of feral water buffalo and fire on growth and survival of mature savanna trees: an experimental field study in Kakadu National Park, northern Australia. Austral Ecology 30, 625–647.
| Impact of feral water buffalo and fire on growth and survival of mature savanna trees: an experimental field study in Kakadu National Park, northern Australia.Crossref | GoogleScholarGoogle Scholar |
Williams, CK, and Dudzinski, ML (1982). Ingestion rates, food utilization and turnover of water and sodium in grazing buffaloes, Bubalus bubalis, and cattle, Bos taurus × B. indicus, in monsoonal Northern Territory. Australian Journal of Agricultural Research 33, 743–754.
| Ingestion rates, food utilization and turnover of water and sodium in grazing buffaloes, Bubalus bubalis, and cattle, Bos taurus × B. indicus, in monsoonal Northern Territory.Crossref | GoogleScholarGoogle Scholar |
Williams, CK, and Ridpath, MG (1982). Rates of herbage ingestion and turnover of water and sodium in feral swamp buffalo, Bubalus bubalis, in relation to primary production in a cyperaceous swamp in monsoonal northern Australia. Wildlife Research 9, 397–408.
| Rates of herbage ingestion and turnover of water and sodium in feral swamp buffalo, Bubalus bubalis, in relation to primary production in a cyperaceous swamp in monsoonal northern Australia.Crossref | GoogleScholarGoogle Scholar |
Woinarski, JCZ, Milne, DJ, and Wanganeen, G (2001). Changes in mammal populations in relatively intact landscapes of Kakadu National Park, Northern Territory, Australia. Austral Ecology 26, 360–370.
| Changes in mammal populations in relatively intact landscapes of Kakadu National Park, Northern Territory, Australia.Crossref | GoogleScholarGoogle Scholar |
Zeng, B (2015). Camel culling and carbon emissions in rangelands in central Australia. Journal of Environmental Planning and Management 58, 270–282.
| Camel culling and carbon emissions in rangelands in central Australia.Crossref | GoogleScholarGoogle Scholar |