The effect of collar weight and capture frequency on bodyweight in feral cats (Felis catus)
Ned L. Ryan-Schofield




A
B
C
D
E
F
G
H
Abstract
Animal-borne devices can affect animal survival, reproduction, and behaviour through both the addition of weight and bulk and the direct effects of initial and subsequent capture. Researchers commonly employ a general rule of thumb that weight of the device must be less than 5% of bodyweight for terrestrial animals; however, this threshold has little empirical basis.
We evaluated the effects of environmental variables, repeated capture, and weight of animal-borne devices on bodyweight in free-ranging feral cats.
We recaptured feral cats at varying frequencies, wearing GPS and/or VHF collars that ranged from 0.29% to 4.88% of bodyweight, and recorded change in cat weight over time.
Collar weight as a percentage of bodyweight was not a significant predictor of feral cat weight change. Rather, change in bodyweight was best described by a negative relationship with an increasing temperature and number of captures, and a positive relationship with time since collar attachment.
Capture had a significant influence on feral cat weight but collar weights up to 5% of bodyweight did not significantly contribute to weight loss. However, the absence of control cats without collars hindered definitive conclusions on the effect of collar weight on cat weight change.
Researchers should space capture and handling events more than 30 days apart to reduce effects of weight loss from capture and handling. Researchers should also consider increasing collar weight and reducing frequency of capture (where collars are less than 5% of bodyweight), particularly if cat bodyweight is a parameter of interest.
Keywords: biologger, bodyweight, capture, collar impacts, condition, handling, neophobia, recapture, stress, weight change.
Introduction
Of critical importance to wildlife conservation and management is ecological knowledge of animal life histories, such as habitat requirements, foraging and reproductive behaviour, movement, dispersal, and home-range attributes. Such data provide stakeholders with the practical knowledge required to make informed management decisions. However, gathering this information from wild animals in their natural habitat can be challenging. Many species are cryptic, whereas others live in environments where direct observation is challenging or impossible (Cooke et al. 2013; Wilmers et al. 2015).
The development of animal-borne devices or biologgers to overcome these difficulties has dramatically expanded the range of animals that can be studied by researchers (Portugal and White 2018), as well as provided the opportunity to understand aspects of animal physiology and ecology that were not previously possible (Rutz and Hays 2009). These devices have revealed remarkable long-distance movements (McGregor et al. 2016; Pilfold et al. 2017; Pedler et al. 2018), exposed novel hunting behaviours (Sakamoto et al. 2009; Troscianko and Rutz 2015; Korpela et al. 2020), and allowed observation of environmental conditions in inaccessible habitats (Charrassin et al. 2008; Hussey et al. 2015).
However, in some studies, animal-borne devices have been found to change behaviour (Aldridge and Brigham 1988; Bruholt 2018), energetics (Godfrey et al. 2003), survivorship (Osborne et al. 1997; Rasiulis et al. 2014), and reproduction (Demers et al. 2003; Venturato et al. 2009), potentially introducing bias and compromising research validity (Casper 2009). Conversely, other studies have recorded no physiological or behavioural impacts on animals from biologgers (Hines and Zwickel 1985; Laurenson and Caro 1994; Thirgood et al. 1995; Bank et al. 2000; Golabek et al. 2008). Recognition of the potential for animal-borne devices to influence results is critical to making informed inferences and conclusions. Consequently, studies using animal-borne technologies commonly employ a general rule of thumb about the weight of the devices relative to the animal’s body size. For non-flying terrestrial animals, a commonly used rule is that weight of the device must be less than 5% (e.g. Tuyttens et al. 2002) or sometimes 3% (e.g. McGregor et al. 2017) of total bodyweight of the animal to reduce potential behavioural or physiological effects. However, the empirical basis and origin of these thresholds is unclear (Barron et al. 2010), and they have been frequently exceeded (see Portugal and White 2018). Negative effects have also been detected when the weight of the device is less than 5% of bodyweight (Aldridge and Brigham 1988; Coughlin and van Heezik 2015). For instance, several studies have demonstrated negative effects of biologgers on bodyweight. Adult red foxes (Vulpes vulpes) in captivity wearing collars of 1–2% bodyweight significantly decreased in weight relative to uncollared control foxes (Bruholt 2018), while San Joaquin kit foxes (Vulpes macrotis mutica) experienced a decrease in bodyweight shortly after collaring relative to uncollared foxes. Collars ranged from 3% to 7% of bodyweight, but decrease in bodyweight did not differ significantly among collar designs (Cypher 1997).
Fixed limits on device weight are commonly accepted by ethics committees and adopted by researchers because of the lack of more refined knowledge on species and device-specific effects. Ideally, guidelines should be based on empirical examination of the negative effects, or lack thereof, caused by device deployment, as determined for the species, device size and deployment duration required for a particular study (Casper 2009; Barron et al. 2010; White et al. 2013; Bodey et al. 2018; Portugal and White 2018).
Introduced cats (Felis catus) are one of the most harmful and widespread of invasive mammalian predators (Doherty et al. 2016), found on many offshore islands and on all continents bar Antarctica. Feral cats have had substantial impacts on wildlife populations (Loss et al. 2013; Nogales et al. 2013; Bellard et al. 2016; Duenas et al. 2021), and have been especially detrimental on islands (Burbidge and Manly 2002; Medina et al. 2011). Because of their cryptic nature, many studies have used biologgers to gather information on cat hunting behaviour (Moseby and McGregor 2022), home-range size and habitat use (Moseby et al. 2009; Recio et al. 2010, 2014; McGregor et al. 2014, 2017), long-distance movements (Guttilla and Stapp 2010; McGregor et al. 2016; Jansen et al. 2021), and predation rates (Loyd et al. 2013; McGregor et al. 2015), to better inform management of the invasive species. All of these studies used collars of <5% bodyweight and, in some cases, <3% or 4%. However, Coughlin and van Heezik (2015) put GPS collars between ~0.86% and 3.11% of bodyweight on 20 domestic cats, and found collars greater than 2% bodyweight could reduce cat home range by 15% relative to lighter collars. These tracked domestic cats had smaller home ranges (<2 ha) than those reported for feral cats (e.g. 50–12,300 ha Moseby et al. 2009). They were also fed by their owners so did not need to hunt to satisfy their energy requirements and could potentially afford to reduce their ranging and hunting behaviour. The authors suggest that this evidence for reduced mobility in domestic cats indicates that feral cats wearing devices may also be similarly affected, and recommend that collars on feral cats should be no more than 2% of the cat’s bodyweight (Coughlin and van Heezik 2015).
As well as the potential effect of wearing a logging device, study animals must also cope with the stress involved in the capture and fitting or checking of devices. Wild mammals exhibit acute stress responses in reaction to researcher interventions (see also Engelhard et al. 2002; Harcourt et al. 2010; reviewed by Cockrem 2013). Significant human-induced stress responses have been associated with reduced fecundity (Alibhai et al. 2001), lower survival (Breed et al. 2019), and altered behaviour (Rachlow et al. 2014). To cope with the energetic cost incurred by mounting a stress response, an animal must either use stored reserves, or divert resources used for other physiological functions (Moberg 2000). Energy reserves are usually stored as fat, and the biological cost model (Moberg 2000) predicts that stress should cause a loss of bodyweight as fat and other energy stores are expended. Consequently, change in bodyweight has been shown to be a practical and relevant proxy for chronic stress responses (McLaren et al. 2004; Moorhouse et al. 2007; Gelling et al. 2010).
In the present study, we used change in bodyweight to estimate the potential for adverse impacts of biologger collaring on feral cats, and investigated whether lighter collars reduced this impact. We used data from 50 free-living feral cats collared during two time periods in the same study area (McGregor et al. 2020a; Van der Weyde et al. 2023), with collars ranging between 0.29% and 4.88% of total bodyweight. We measured the effect of collar weight, number of recaptures, period of deployment, and environmental variables on the change in bodyweight over time.
Methods
Study site
Our research was conducted at Arid Recovery, a 123 km2 fenced conservation reserve in central South Australia (−30.37144°N, 136.90409°E). The climate is arid with hot dry summers and mild winters, likelihood of rainfall does not vary by month and the long-term average annual rainfall is 144.5 mm (Records from Olympic Dam Aerodrome, www.bom.gov.au/climate/data, accessed March 2022). The habitat is dominated by longitudinal dunes separated by interdunal clay swales, with a small number of minor ephemeral creek lines. Dunes support sparse Acacia and Dodonaea shrubland, whereas swales are covered in low (~50 cm) chenopod (Maireana spp. and Atriplex spp.) species. The reserve is divided into six paddocks, with cats, foxes, and rabbits (Oryctolagus cuniculus) removed from four of these, totalling 60 km2, whereas the remaining two paddocks ‘Red Lake’ (25 km2) and the ‘Experimental Paddock’ (37 km2) contain populations of feral cats and rabbits and are used for landscape-scale manipulation experiments (e.g. Moseby et al. 2012; West et al. 2018; Ross et al. 2019; McGregor et al. 2020a).
Study design
Our study took place in the Experimental Paddock across two periods, the first from August 2016 to February 2017, and the second from April 2021 to December 2021. In the first period, resident cats were captured in the Experimental Paddock using cage traps and soft-jaw leg traps (Victor #1.5), with cat urine as lure. The purpose of this study was to understand how cats responded to rabbit control (McGregor et al. 2020a). For the second period, cats were caught for a study testing the extent of neophobia towards a novel method of feral cat control. These cats were primarily caught outside the reserve using the same methods as in 2016–2017, and released into the Experimental Paddock. Cats were removed from traps and placed into a dark canvas handling bag and were then semi-restrained and fitted with either a VHF, GPS, or video collar of varying weight. No chemical restraint was used, and fitting of collars typically took less than 15 min. Cats were then either released immediately at point of capture, or if temperatures were above 37°C held and housed within a covered cage trap in a cooled shed, and released in the evening at point of capture. Because the cats were collared to monitor their movement and survival, we did not release and recapture a control group of cats without collars.
Cats were typically recaptured after 1 week to 6 months by tracking them, and then netting them or chasing to their daytime burrow and placing a cage-trap at the entrance. Once captured, cats were placed in a dark bag and processed without sedation. We measured weight with a tared 10 kg spring scale (Salter Super Samson 10 kg, 50 g accuracy). In most instances, a new collar of variable weight was placed on the cat, and it was re-released. Cats were recaptured up to six times over the course of the study to check collar fit, change collars and/or download data. Methods were approved by University of Tasmania (A0015720), University of Adelaide (S-2016-112), and University of New South Wales (#20-109A) Animal Ethics Committees.
Analysis
Change in bodyweight was analysed using a candidate set of multivariate linear mixed models using the function glmmTMB (Brooks et al. 2017) in R ver. 4.3.2 (www.r-project.org, accessed April 2024). Explanatory variables included collar weight as a percentage of bodyweight (calculated from weight at previous capture), total number of days since collar was deployed to investigate whether cats habituated to collars over time, the number of times the cat had been recaptured, to test the effect of repeat-handling stress, weight of cat when first captured as a demographic measure, experimental period (2016–2017 or 2021) to explore changes caused by experimental design, rainfall in the past 6 months, and average maximum daily temperature in the month preceding capture, with individual cat ID included as a random effect. Rainfall and temperature data were sourced from the Bureau of Meteorology (https://reg.bom.gov.au/climate/data/) from the closest registered recording location for which data were available over the study period (Olympic Dam Aerodrome, 25 km from the Experimental Paddock, or Andamooka, 30 km from the Experimental Paddock). Because comparable information on prey availability was not available for both study periods, we used rainfall and temperature as an indication of resource availability. Rainfall triggers resource booms in arid Australia, which lead to increases in abundance of a range of desert animals that are preyed on by cats (Letnic et al. 2005), and temperature also alters prey availability because it influences the breeding patterns of rabbits in the study area (Bowen and Read 1998).
We compared models of all combinations of variables without interactions (128 models) by using the Akaike information criterion corrected for small sample sizes (AICc). Parameter estimates were derived for models with delta AICc of 2 or less, and averaged in a model-averaging information-theory framework using the R function MuMIn::model.avg, ver. 1.47.5, available at http://CRAN.R-project.org/package=MuMIn(Barton 2011). Models were visually presented with the R function effects::predictorEffects ver. 4.2-2, available at https://CRAN.R-project.org/package=effects (Fox and Weisberg 2018).
Results
Over the two study periods, we caught and collared 50 feral cats and recorded a total of 98 recaptures. Cats carried collars for a minimum of 1 day and a maximum of 231 days between captures (mean = 54.8, s.d. = 44.3 days), and were recaught on average 1.91 times, with s.d. of 1.18 (min = 1, max = 6 times). Collars ranged between 0.29% and 4.88% of total bodyweight (mean = 2.04, s.d. = 1.22), and cat weight averaged 4.04 kg (s.d. = 0.93 kg), with a minimum of 2 kg and a maximum of 6.4 kg (Fig. 1).
Spread of cat weights (kg) and percentage weights of collar deployed on feral cats at Arid Recovery in 2016–2017 and 2021.
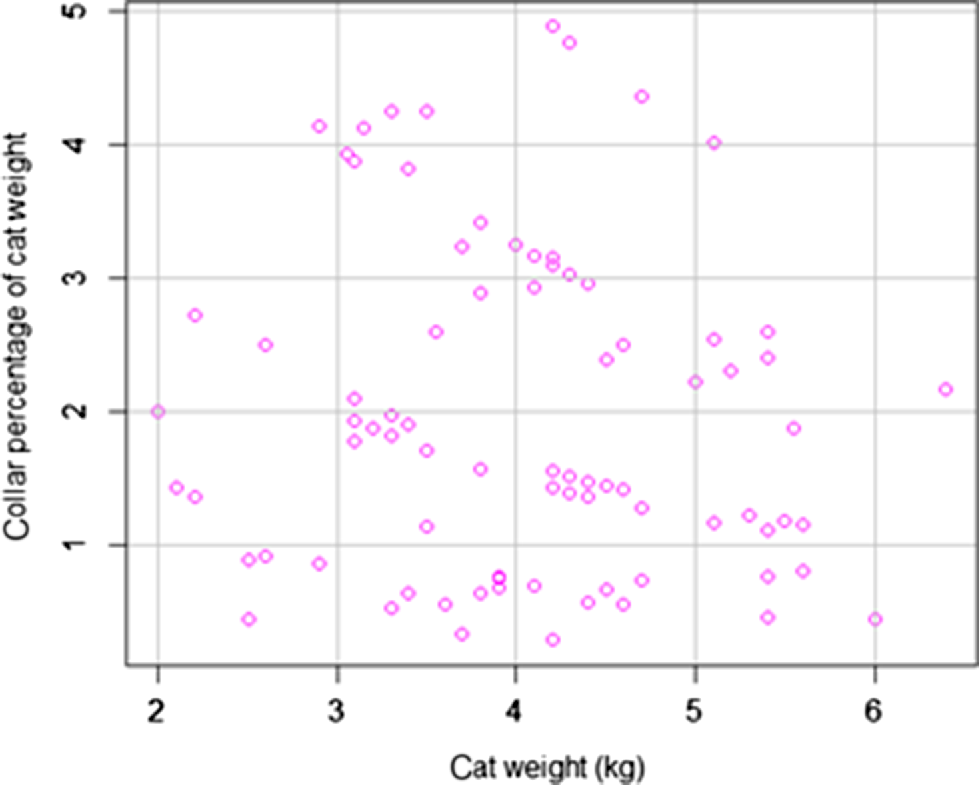
Of the 128 models compared, seven had delta AICc of ≤2 (Table 1), and were subsequently averaged in an information-theory framework.
Model | d.f. | LogLik | AICc | Delta | Weight | |
---|---|---|---|---|---|---|
Max temp. + number of captures + days | 6 | −78.31 | 169.53 | 0.00 | 0.26 | |
Max temp. + number of captures + days + exp. period | 7 | −77.73 | 170.71 | 1.17 | 0.15 | |
Max temp. + number of captures + days + exp. period + rainfall | 8 | −76.62 | 170.85 | 1.31 | 0.14 | |
Max temp. + number of captures + days + rainfall | 7 | −77.87 | 170.99 | 1.45 | 0.13 | |
Max temp. + number of captures + days + initial weight | 7 | −77.87 | 170.99 | 1.45 | 0.13 | |
Max temp. + number of captures + collar percentage + days | 7 | −78.08 | 171.40 | 1.87 | 0.10 | |
Max temp. + number of captures | 5 | −80.41 | 171.48 | 1.94 | 0.10 |
The most important variables for explaining changes in cat weight on recapture were the preceding average maximum monthly temperature, the number of recaptures, and the number of days the collar had been on since last recapture (Table 2). Experimental period, rainfall in the previous 6 months, initial cat weight, and the percentage of bodyweight of the collar were also included in the model averaged output; however, these terms were not significant predictors of cat weight (Table 2).
Explanatory variable | Number of models | Importance | Coefficient | s.e. | Adjusted s.e. | z | P-value | |
---|---|---|---|---|---|---|---|---|
Average maximum temperature | 7 | 1 | −0.024 | 0.010 | 0.010 | 2.44 | 0.015 | |
Number of captures | 7 | 1 | −0.116 | 0.050 | 0.050 | 2.30 | 0.022 | |
Days collar on | 6 | 0.9 | 0.003 | 0.001 | 0.001 | 2.05 | 0.040 | |
Experimental period | 2 | 0.28 | 0.185 | 0.145 | 0.147 | 1.26 | 0.208 | |
Rainfall in the past 6 months | 2 | 0.26 | 0.002 | 0.002 | 0.002 | 1.16 | 0.245 | |
Previous weight | 1 | 0.13 | −0.061 | 0.065 | 0.066 | 0.92 | 0.357 | |
Collar percentage of bodyweight | 1 | 0.10 | −0.031 | 0.046 | 0.047 | 0.67 | 0.506 |
Terms in bold indicate statistically significant variables.
Collar weight as a percentage of bodyweight (Fig. 2a) was not a significant predictor of change in cat weight on recapture (P = 0.506). Rather, our model predicted that cat weight change declined by 24 g (s.e. 10 g, P = 0.015) for each increase in temperature of one degree Celsius (Fig. 2b). After each successive recapture, predicted cat weight change declined by 116 g (s.e. 50 g, P = 0.022) (Fig. 2c). However, we also found a positive relationship between weight change and the amount of time that had passed since the cat was last captured, whereby weight change increased by 3 g per day (s.e. 1 g, P = 0.040) between successive recaptures (Fig. 2d).
Discussion
We found no significant relationship between collar weight and cat weight change in recaptured feral cats. However, our experimental design does not allow us to rule out a potential effect of wearing any collar on effecting weight change, because we did not have a control sample of uncollared feral cats.
Coughlin and van Heezik (2015) described reductions in movement from domestic cats wearing collars of approximately 3% bodyweight. Likewise, it is possible that our cats reduced movement but that this did not lead to weight loss. Coughlin and van Heezik (2015) indicated that the impact of heavier devices on home-range size and distances travelled suggested that devices worn by cats may influence energy expenditure, and to accommodate increased energy expenditure cats could reduce high-energy behaviours, such as ranging and hunting. However, unlike feral cats, domestic cats are fed by their owners and do not need to hunt to satisfy their energy requirements; so, they can potentially afford to reduce their ranging and hunting behaviour, whereas feral cats may not. Unfortunately, as we did not directly measure movement or energy expenditure, we were unable to monitor for any behavioural change that may have offset the increased energy requirements. Alternatively, just as other research has found no influence of animal-borne devices on energy expenditure or foraging behaviour (Berteaux et al. 1996; Golabek et al. 2008), feral cats may simply be more tolerant of heavier collars. Cats are primarily ambush predators, relying on a ‘sit-and-wait’ strategy that relies on stalking and camouflage (e.g. Judge et al. 2012; Moseby and McGregor 2022); so, carrying the extra weight of a collar may not have a material impact.
Monthly maximum temperature was found to have a significant negative effect on feral cat bodyweight. This may occur because juvenile rabbits are less abundant in late summer, because rabbits breed from late winter to early summer in the study area (Bowen and Read 1998). Small juvenile rabbits are likely to be easier for smaller non-breeding female cats and/or younger inexperienced cats to hunt, because smaller cats may be less effective at catching larger prey (Read and Bowen 2001; Fleming et al. 2020). This is supported by Moseby et al. (2021), who found a significant interaction between bodyweight and temperature on rabbit consumption by feral cats, with smaller cats feeding on rabbits only in the cooler months whereas larger cats fed on rabbits regardless of temperature. Approximately 215 g of fresh rabbit is capable of meeting a feral cat’s daily energy requirements (Plantinga et al. 2011), and so a reduction in the availability of a high-energy resource would likely affect feral cats’ ability to maintain weight. In addition, higher temperatures could cause thermal stress in cats. Feral cats experience rapid increases in evaporative water loss and core body temperature when ambient temperatures rise above 30°C, increasing energy requirements to support thermoregulation, while also decreasing potential foraging time as cats seek refuge from extreme heat (Briscoe et al. 2022).
Rainfall in the previous 6 months was included in the model-averaged output; however, there was no support for this variable predicting cat bodyweight. Other studies have shown relationships between rainfall and small rodent abundance because they respond to the abundance of food provided by heavy rains (Read 1992; Brandle and Moseby 1999; Letnic et al. 2005). When numbers are high, rodents can be a key food resource for cats (Read and Bowen 2001; Plantinga et al. 2011; Doherty et al. 2015), and their availability is likely to influence change in cat bodyweight. Because we were unable to directly measure rabbit and rodent activity across the entire study period, it is possible that a relationship between bodyweight and prey availability was missed, or that prey abundance was always high enough to sustain the weights of feral cats in our study. However, it should be noted that although experimental period (2016–2017 or 2021) was not found to be a significant predictor of change in bodyweight, during the 2016–2017 study, there was a large and abrupt experimental reduction in rabbit numbers in the Experimental Paddock, which did cause a significant decline in survival and condition of feral cats (see McGregor et al. 2020a). In total, 8 of 21 cats survived by hunting native rodents along the southern fence line, where their abundance was likely to be greatest because of the proximity to the rest of the fenced reserve (McGregor et al. 2020b; Moseby et al. 2020), potentially causing a decline in small mammals in the Experimental Paddock also (McGregor et al. 2020a). In contrast, native rodent numbers were reported to be high throughout the 2021 period (Arid Recovery, unpubl. data) following good summer rains.
The number of times a cat was recaptured had a significant negative effect on bodyweight. After each successive recapture, our model predicted that change in feral cat weight was reduced by 116 g (s.e. 50 g). However, of 98 recaptures, only 25 represented cats caught three or more times. Although our model predicted a linear reduction in weight as cats were successively recaptured, confidence intervals around model predictions increased greatly after more than two recaptures, likely owing to small sample sizes. Without more data, we cannot conclusively demonstrate that change in weight was monotonic, and could potentially have been a single-step reduction in weight change that was maintained after multiple recaptures, possibly owing to cats acclimating to capture.
Regardless, reduction in weight owing to capture may be attributable to repeated stress caused by the capture and handling methods used. Wild mammals show an acute stress response when they are captured and handled (Engelhard et al. 2002; Harcourt et al. 2010; Breed et al. 2019), which incurs an energetic cost (Moberg 2000) that could translate into an impact on bodyweight. Repeated handling stress has been shown to cause permanent reduction in bodyweight of adult lab rats (Harris et al. 1998; Zhou et al. 1999). In addition, other felids such as cheetah, Acinonyx jubatus (Braud et al. 2019), European wildcats, Felis silvestris (Monterroso et al. 2022), Bornean leopard cats, Prionailurus bengalensis borneoensis (Nájera et al. 2014), and Sunda clouded leopards, Neofelis diardi (Nájera and Hearn 2022), have also been recorded exhibiting stress responses to capture and handling. However, standard error was considerable, potentially because of differences in duration of recapture attempts, or transport of cats to cooled sheds for collaring on hot days. The stress caused by our most commonly used trapping method burrow traps in warrens may also be different from that caused by other methods such as cage trapping, spotlight netting, or leghold trapping. Changes in capture methods used between recapture attempts could also have contributed to the significant variation described by our model; however, methods were recorded inconsistently between sessions and so we were unable to include this explanatory variable in our models. Additionally, we did not use anaesthesia during restraint and collar fitting so as to minimise handling and recovery times; however, possibly handling aware animals is more likely to cause acute stress.
Another contributing explanation for a reduction in weight as a result of capture could be that neophobia induced by handling resulted in behavioural changes, potentially reducing foraging activity. Neophobia (aversion to new or unfamiliar stimuli) can be a plastic trait within individuals, which can be induced by exposure to high-risk environments (Brown et al. 2013). Neophobia benefits animals in high-risk environments, where novel stimuli are likely to be hazardous and so avoidance is advantageous (Greenberg 2003). However, putting energy into avoidance of novel stimuli can incur other costs such as reduced access to resources (Sol et al. 2011). Reduction in cat weight as a result of capture may also have been related to reduced access to resources. The sudden, stressful experience of capture and handling may have triggered an alteration of the cats perceived environmental risk, thereby creating a sustained reduction in their capacity to forage effectively.
Bodyweight was also positively correlated with the amount of time the collar remained on, or the time between successive recaptures. This could be attributed to younger cats growing and gaining weight steadily over time (Jones and Coman 1982); however, the absence of a reliable ageing mechanism for feral cats makes it difficult to determine whether there was a demographic effect at play. Heavier cats are perhaps likely to be older, but although the previous weight of cats was included in the model-averaged output, the term had low importance and the estimate was not statistically significant. Additionally, longer periods between recaptures could also have allowed more time for cats to recover from associated stress, although an interaction between recapture number and time did not feature in any of the top-ranked candidate models. Regardless, because weight change was predicted to increase by 3 g each day post-capture, researchers seeking to minimise the physiological impact of multiple recaptures on feral cats could consider extending the intervals between captures to a minimum of 30 days. This approach could mitigate the adverse effects on cat weight attributed to the stress response induced by frequent recaptures.
Conclusions
Our results showed that captures affect bodyweight in cats. Research involving cat captures should factor that into their experimental design, especially if feral cat weight or condition over time is a parameter of interest.
Although we cannot conclude that wearing a collar had no effect on feral cat bodyweight, we did not find any evidence to suggest that increasing collar weight as a percentage of bodyweight influenced cat weight when collars were <5% of bodyweight. However, as we did not directly measure movement or energy expenditure, we were unable to detect any behavioural change that may have offset the possible increased energy requirements imposed by heavy collars. Future research should address this knowledge gap.
Given that increasing collar weight up to 4.88% did not affect cat weight, but number of recaptures did, it may be more prudent to increase collar weight to maximise data, and so reduce the need for successive recaptures. Alternatively, increasing the time between recaptures to at least 30 days could mitigate weight loss resulting from capture and handling. Future experiments should compare capture and handling methods (particularly with and without anaesthesia) to determine how they influence weight loss and stress levels in feral cats.
Data availability
Our final raw data can be found at https://github.com/nedschofield/collar_impacts. Rainfall and temperature data are publicly available through sources cited within the text.
Conflicts of interest
Sarah Legge is an Editor of Wildlife Research. To mitigate this potential conflicts of interest they were blinded from the review process.
Declaration of funding
This research received support from the Australian Government’s National Environmental Science Program through the Threatened Species Recovery Hub. Arid Recovery, the Foundation for Australia’s Most Endangered species, Australian Research Council linkage grant LP190100291, the Conservation and Wildlife Research Trust, The Biological Society of South Australia, The Nature Conservation Society of South Australia, the Ecological Society of Australia, Bush Heritage Australia, and the University of Adelaide also provided cash and in-kind support.
Author contributions
All authors conceived the ideas and designed methodology; NRS and HM collected the data; NRS analysed the data; NRS led writing of the paper; and all authors contributed critically to drafts and gave final approval.
Acknowledgements
The rabbit removal effort in 2016–2017 was conducted by Frank Bernhardt (Bernhardt’s Pest and Weed Control). Zac Richardson, Tessa Manning, Daniel Markos, Jamie Simpson, Lucy Wood, Alex Marinelli, Emma Pollard, Morgan Humphrey, Athanasia Hatzis, Carla Bruinsma, and Lisa Jokinen assisted with field data collection. Christopher Johnson assisted with project development. Katherine Tuft, Catherine Lynch, Genevieve Hayes, Leanne van Der Weyde, Nathan Manders, and Milly Breward provided logistical support. We also acknowledge the Kokatha people as the Traditional owners of the lands on which this research was undertaken. We recognise and respect the enduring relationship that they have with their land and waters, and we pay our respects to Elders past and present.
References
Aldridge HDJN, Brigham RM (1988) Load carrying and maneuverability in an insectivorous bat: a test of the 5% ‘rule’ of radio-telemetry. Journal of Mammalogy 69, 379-382.
| Crossref | Google Scholar |
Alibhai SK, Jewell ZC, Towindo SS (2001) Effects of immobilization on fertility in female black rhino (Diceros bicornis). Journal of Zoology 253, 333-345.
| Crossref | Google Scholar |
Bank MS, Franklin WL, Sarno RJ (2000) Assessing the effect of radiocollars on juvenile guanaco survival. Oecologia 124, 232-234.
| Crossref | Google Scholar | PubMed |
Barron DG, Brawn JD, Weatherhead PJ (2010) Meta-analysis of transmitter effects on avian behaviour and ecology. Methods in Ecology and Evolution 1, 180-187.
| Crossref | Google Scholar |
Barton K (2011) MuMIn: multi-model inference. R package version 1.0.0. Available at http://CRAN.R-project.org/package=MuMIn
Bellard C, Cassey P, Blackburn TM (2016) Alien species as a driver of recent extinctions. Biology Letters 12, 20150623.
| Crossref | Google Scholar | PubMed |
Berteaux D, Masseboeuf F, Bonzom J-M, Bergeron J-M, Thomas DW, Lapierre H (1996) Effect of carrying a radiocollar on expenditure of energy by meadow voles. Journal of Mammalogy 77, 359-363.
| Crossref | Google Scholar |
Bodey TW, Cleasby IR, Bell F, Parr N, Schultz A, Votier SC, Bearhop S (2018) A phylogenetically controlled meta-analysis of biologging device effects on birds: deleterious effects and a call for more standardized reporting of study data. Methods in Ecology and Evolution 9, 946-955.
| Crossref | Google Scholar |
Bowen Z, Read J (1998) Population and demographic patterns of rabbits (Oryctolagus cuniculus) at Roxby Downs in arid South Australia and the influence of rabbit haemorrhagic disease. Wildlife Research 25, 655-662.
| Crossref | Google Scholar |
Brandle R, Moseby KE (1999) Comparative ecology of two populations of Pseudomys australis in northern South Australia. Wildlife Research 26, 541-564.
| Crossref | Google Scholar |
Braud C, Mitchell EP, Van der Merwe V, Tordiffe ASW (2019) A veterinary survey of factors associated with capture-related mortalities in cheetahs (Acinonyx jubatus). Journal of the South African Veterinary Association 90, a1723.
| Crossref | Google Scholar |
Breed D, Meyer LCR, Steyl JCA, Goddard A, Burroughs R, Kohn TA (2019) Conserving wildlife in a changing world: understanding capture myopathy—a malignant outcome of stress during capture and translocation. Conservation Physiology 7, coz027.
| Crossref | Google Scholar |
Briscoe NJ, McGregor H, Roshier D, Carter A, Wintle BA, Kearney MR (2022) Too hot to hunt: mechanistic predictions of thermal refuge from cat predation risk. Conservation Letters 15, e12906.
| Crossref | Google Scholar |
Brooks ME, Kristensen K, van Benthem KJ, Magnusson A, Berg CW, Nielsen A, Skaug HJ, Mächler M, Bolker BM (2017) glmmTMB balances speed and flexibility among packages for zero-inflated generalized linear mixed modeling. The R Journal 9, 378-400.
| Crossref | Google Scholar |
Brown GE, Ferrari MCO, Elvidge CK, Ramnarine I, Chivers DP (2013) Phenotypically plastic neophobia: a response to variable predation risk. Proceedings of the Royal Society B: Biological Sciences 280, 20122712.
| Crossref | Google Scholar |
Burbidge AA, Manly BFJ (2002) Mammal extinctions on Australian islands: causes and conservation implications. Journal of Biogeography 29, 465-473.
| Crossref | Google Scholar |
Casper RM (2009) Guidelines for the instrumentation of wild birds and mammals. Animal Behaviour 78, 1477-1483.
| Crossref | Google Scholar |
Charrassin J-B, Hindell M, Rintoul SR, Roquet F, Sokolov S, Biuw M, Costa D, Boehme L, Lovell P, Coleman R, Timmermann R, Meijers A, Meredith M, Park Y-H, Bailleul F, Goebel M, Tremblay Y, Bost C-A, McMahon CR, Field IC, Fedak MA, Guinet C (2008) Southern Ocean frontal structure and sea-ice formation rates revealed by elephant seals. Proceedings of the National Academy of Sciences 105, 11634-11639.
| Crossref | Google Scholar |
Cockrem JF (2013) Individual variation in glucocorticoid stress responses in animals. General and Comparative Endocrinology 181, 45-58.
| Crossref | Google Scholar | PubMed |
Cooke SJ, Midwood JD, Thiem JD, Klimley P, Lucas MC, Thorstad EB, Eiler J, Holbrook C, Ebner BC (2013) Tracking animals in freshwater with electronic tags: past, present and future. Animal Biotelemetry 1, 5.
| Crossref | Google Scholar |
Coughlin CE, van Heezik Y (2015) Weighed down by science: do collar-mounted devices affect domestic cat behaviour and movement? Wildlife Research 41, 606-614.
| Crossref | Google Scholar |
Cypher BL (1997) Effects of radiocollars on San Joaquin kit foxes. The Journal of Wildlife Management 61 1412-1423.
| Crossref | Google Scholar |
Demers F, Giroux J-F, Gauthier G, Bêty J (2003) Effects of collar-attached transmitters on behaviour, pair bond and breeding success of snow geese Anser caerulescens atlanticus. Wildlife Biology 9, 161-170.
| Crossref | Google Scholar |
Doherty TS, Davis RA, van Etten EJB, Algar D, Collier N, Dickman CR, Edwards G, Masters P, Palmer R, Robinson S (2015) A continental-scale analysis of feral cat diet in Australia. Journal of Biogeography 42, 964-975.
| Crossref | Google Scholar |
Doherty TS, Glen AS, Nimmo DG, Ritchie EG, Dickman CR (2016) Invasive predators and global biodiversity loss. Proceedings of the National Academy of Sciences 113, 11261-11265.
| Crossref | Google Scholar |
Duenas M-A, Hemming DJ, Roberts A, Diaz-Soltero H (2021) The threat of invasive species to IUCN-listed critically endangered species: a systematic review. Global Ecology and Conservation 26, e01476.
| Crossref | Google Scholar |
Engelhard G, Brasseur S, Hall A, Burton H, Reijnders P (2002) Adrenocortical responsiveness in southern elephant seal mothers and pups during lactation and the effect of scientific handling. Journal of Comparative Physiology B: Biochemical, Systemic, and Environmental Physiology 172, 315-328.
| Crossref | Google Scholar |
Fleming PA, Crawford HM, Auckland CH, Calver MC (2020) Body size and bite force of stray and feral cats—are bigger or older cats taking the largest or more difficult-to-handle prey? Animals 10, 707.
| Crossref | Google Scholar | PubMed |
Gelling M, Montes I, Moorhouse TP, Macdonald DW (2010) Captive housing during water vole (Arvicola terrestris) reintroduction: does short-term social stress impact on animal welfare? PLoS ONE 5, e9791.
| Crossref | Google Scholar | PubMed |
Godfrey JD, Bryant DM, Williams MJ (2003) Radio-telemetry increases free-living energy costs in the endangered Takahe Porphyrio mantelli. Biological Conservation 114, 35-38.
| Crossref | Google Scholar |
Golabek KA, Jordan NR, Clutton-Brock TH (2008) Radiocollars do not affect the survival or foraging behaviour of wild meerkats. Journal of Zoology 274, 248-253.
| Crossref | Google Scholar |
Guttilla DA, Stapp P (2010) Effects of sterilization on movements of feral cats at a wildland–urban interface. Journal of Mammalogy 91, 482-489.
| Crossref | Google Scholar |
Harcourt RG, Turner E, Hall A, Waas JR, Hindell M (2010) Effects of capture stress on free-ranging, reproductively active male Weddell seals. Journal of Comparative Physiology A 196, 147-154.
| Crossref | Google Scholar |
Harris RBS, Zhou J, Youngblood BD, Rybkin II, Smagin GN, Ryan DH (1998) Effect of repeated stress on body weight and body composition of rats fed low-and high-fat diets. American Journal of Physiology – Regulatory, Integrative and Comparative Physiology 275, R1928-R1938.
| Crossref | Google Scholar |
Hines JE, Zwickel FC (1985) Influence of radio packages on young blue grouse. The Journal of Wildlife Management 49, 1050-1054.
| Crossref | Google Scholar |
Hussey NE, Kessel ST, Aarestrup K, Cooke SJ, Cowley PD, Fisk AT, Harcourt RG, Holland KN, Iverson SJ, Kocik JF, Mills Flemming JE, Whoriskey FG (2015) Aquatic animal telemetry: a panoramic window into the underwater world. Science 348, 1255642.
| Crossref | Google Scholar | PubMed |
Jansen J, McGregor H, Axford G, Dean AT, Comte S, Johnson CN, Moseby KE, Brandle R, Peacock DE, Jones ME (2021) Long-distance movements of feral cats in semi-arid South Australia and implications for conservation management. Animals 11, 3125.
| Crossref | Google Scholar | PubMed |
Jones E, Coman BJ (1982) Ecology of the feral cat, Felis catus (L.), in south-eastern Australia III.* Home ranges and population ecology in semiarid north-west Victoria. Wildlife Research 9, 409-420.
| Crossref | Google Scholar |
Judge S, Lippert JS, Misajon K, Hu D, Hess SC (2012) Videographic evidence of endangered species depredation by feral cat. Pacific Conservation Biology 18, 293-296.
| Crossref | Google Scholar |
Korpela J, Suzuki H, Matsumoto S, Mizutani Y, Samejima M, Maekawa T, Nakai J, Yoda K (2020) Machine learning enables improved runtime and precision for bio-loggers on seabirds. Communications Biology 3, 633.
| Crossref | Google Scholar |
Laurenson MK, Caro TM (1994) Monitoring the effects of non-trivial handling in free-living cheetahs. Animal Behaviour 47, 547-557.
| Crossref | Google Scholar |
Letnic M, Tamayo B, Dickman CR (2005) The responses of mammals to La Nina (El Nino Southern Oscillation)-associated rainfall, predation, and wildfire in central Australia. Journal of Mammalogy 86, 689-703.
| Crossref | Google Scholar |
Loss SR, Will T, Marra PP (2013) The impact of free-ranging domestic cats on wildlife of the United States. Nature Communications 4, 1396.
| Google Scholar |
Loyd KAT, Hernandez SM, Carroll JP, Abernathy KJ, Marshall GJ (2013) Quantifying free-roaming domestic cat predation using animal-borne video cameras. Biological Conservation 160, 183-189.
| Crossref | Google Scholar |
McGregor HW, Legge S, Jones ME, Johnson CN (2014) Landscape management of fire and grazing regimes alters the fine-scale habitat utilisation by feral cats. PLoS ONE 9, e109097.
| Crossref | Google Scholar | PubMed |
McGregor H, Legge S, Jones ME, Johnson CN (2015) Feral cats are better killers in open habitats, revealed by animal-borne video. PLoS ONE 10, e0133915.
| Crossref | Google Scholar | PubMed |
McGregor HW, Legge S, Jones ME, Johnson CN (2016) Extraterritorial hunting expeditions to intense fire scars by feral cats. Scientific Reports 6, 22559.
| Crossref | Google Scholar |
McGregor HW, Cliff HB, Kanowski J (2017) Habitat preference for fire scars by feral cats in Cape York Peninsula, Australia. Wildlife Research 43, 623-633.
| Crossref | Google Scholar |
McGregor H, Moseby K, Johnson CN, Legge S (2020a) The short-term response of feral cats to rabbit population decline: are alternative native prey more at risk? Biological Invasions 22, 799-811.
| Crossref | Google Scholar |
McGregor H, Read J, Johnson CN, Legge S, Hill B, Moseby K (2020b) Edge effects created by fenced conservation reserves benefit an invasive mesopredator. Wildlife Research 47, 677-685.
| Crossref | Google Scholar |
McLaren GW, Mathews F, Fell R, Gelling M, Macdonald DW (2004) Body weight change as a measure of stress: a practical test. Animal Welfare 13, 337-341.
| Crossref | Google Scholar |
Medina FM, Bonnaud E, Vidal E, Tershy BR, Zavaleta ES, Josh Donlan C, Keitt BS, Le Corre M, Horwath SV, Nogales M (2011) A global review of the impacts of invasive cats on island endangered vertebrates. Global Change Biology 17, 3503-3510.
| Crossref | Google Scholar |
Monterroso P, Díaz-Ruiz F, Ferreras P, Santos N (2022) Physiological and behavioural impact of trapping for scientific purposes on European mesocarnivores. In ‘Mammal trapping–wildlife management, animal welfare & international standards’. (Ed. G Proulx) pp. 201–214. (Alpha Wildlife Publications: Alberta)
Moorhouse TP, Gelling M, McLaren GW, Mian R, Macdonald DW (2007) Physiological consequences of captive conditions in water voles (Arvicola terrestris). Journal of Zoology 271, 19-26.
| Crossref | Google Scholar |
Moseby KE, McGregor HM (2022) Feral cats use fine scale prey cues and microhabitat patches of dense vegetation when hunting prey in arid Australia. Global Ecology and Conservation 35, e02093.
| Crossref | Google Scholar |
Moseby KE, Stott J, Crisp H (2009) Movement patterns of feral predators in an arid environment – implications for control through poison baiting. Wildlife Research 36, 422-435.
| Crossref | Google Scholar |
Moseby KE, Neilly H, Read JL, Crisp HA (2012) Interactions between a top order predator and exotic mesopredators in the Australian rangelands. International Journal of Ecology 2012, 250352.
| Crossref | Google Scholar |
Moseby KE, McGregor H, Hill BM, Read JL (2020) Exploring the internal and external wildlife gradients created by conservation fences. Conservation Biology 34, 220-231.
| Crossref | Google Scholar | PubMed |
Moseby KE, McGregor H, Read JL (2021) The lethal 23%: predator demography influences predation risk for threatened prey. Animal Conservation 24, 217-229.
| Crossref | Google Scholar |
Nájera F, Hearn AJ (2022) Assessing welfare while capturing free-ranging Sunda clouded leopards (Neofelis diardi) with cage-traps: effects of physical restraint on serum biochemistry. In ‘Mammal trapping–wildlife management, animal welfare & international standards’. (Ed. G Proulx) pp. 215–223. (Alpha Wildlife Publications: Alberta)
Nájera F, Hearn AJ, Ross J, Nathan S, Revuelta L (2014) Alterations in selected serum biochemistry values of free-ranging Bornean leopard cats (Prionailurus bengalensis borneoensis) captured by box traps. Veterinary Research Communications 38, 265-269.
| Crossref | Google Scholar | PubMed |
Nogales M, Vidal E, Medina FM, Bonnaud E, Tershy BR, Campbell KJ, Zavaleta ES (2013) Feral cats and biodiversity conservation: the urgent prioritization of island management. BioScience 63, 804-810.
| Crossref | Google Scholar |
Osborne DA, Frawley BJ, Weeks HP, Jr (1997) Effects of radio tags on captive northern bobwhite (Colinus virginianus) body composition and survival. American Midland Naturalist 137, 213-224.
| Crossref | Google Scholar |
Pedler RD, Ribot RFH, Bennett ATD (2018) Long-distance flights and high-risk breeding by nomadic waterbirds on desert salt lakes. Conservation Biology 32, 216-228.
| Crossref | Google Scholar | PubMed |
Pilfold NW, McCall A, Derocher AE, Lunn NJ, Richardson E (2017) Migratory response of polar bears to sea ice loss: to swim or not to swim. Ecography 40, 189-199.
| Crossref | Google Scholar |
Plantinga EA, Bosch GX, Hendriks WH (2011) Estimation of the dietary nutrient profile of free-roaming feral cats: possible implications for nutrition of domestic cats. British Journal of Nutrition 106, S35-S48.
| Crossref | Google Scholar | PubMed |
Portugal SJ, White CR (2018) Miniaturization of biologgers is not alleviating the 5% rule. Methods in Ecology and Evolution 9, 1662-1666.
| Crossref | Google Scholar |
Rachlow JL, Peter RM, Shipley LA, Johnson TR (2014) Sub-lethal effects of capture and collaring on wildlife: experimental and field evidence. Wildlife Society Bulletin 38, 458-465.
| Crossref | Google Scholar |
Rasiulis AL, Festa-Bianchet M, Couturier S, Côté SD (2014) The effect of radio-collar weight on survival of migratory caribou. The Journal of Wildlife Management 78, 953-956.
| Crossref | Google Scholar |
Read JL (1992) Influence of habitats, climate, grazing and mining on terrestrial vertebrates at Olympic Dam, South Australia. The Rangeland Journal 14, 143-156.
| Crossref | Google Scholar |
Read J, Bowen Z (2001) Population dynamics, diet and aspects of the biology of feral cats and foxes in arid South Australia. Wildlife Research 28, 195-203.
| Crossref | Google Scholar |
Recio MR, Mathieu RSA, Maloney R, Seddon PJ (2010) First results of feral cats (Felis catus) monitored with GPS collars in New Zealand. New Zealand Journal of Ecology 34, 288-296.
| Google Scholar |
Recio MR, Mathieu R, Virgós E, Seddon PJ (2014) Quantifying fine-scale resource selection by introduced feral cats to complement management decision-making in ecologically sensitive areas. Biological Invasions 16, 1915-1927.
| Crossref | Google Scholar |
Ross AK, Letnic M, Blumstein DT, Moseby KE (2019) Reversing the effects of evolutionary prey naiveté through controlled predator exposure. Journal of Applied Ecology 56, 1761-1769.
| Crossref | Google Scholar |
Rutz C, Hays GC (2009) New frontiers in biologging science. Biology Letters 5, 289-292.
| Crossref | Google Scholar | PubMed |
Sakamoto KQ, Takahashi A, Iwata T, Trathan PN (2009) From the eye of the albatrosses: a bird-borne camera shows an association between albatrosses and a killer whale in the Southern Ocean. PLoS ONE 4, e7322.
| Crossref | Google Scholar | PubMed |
Sol D, Griffin AS, Bartomeus I, Boyce H (2011) Exploring or avoiding novel food resources? The novelty conflict in an invasive bird. PLoS ONE 6, e19535.
| Crossref | Google Scholar | PubMed |
Thirgood SJ, Redpath SM, Hudson PJ, Hurley MM, Aebischer NJ (1995) Effects of necklace radio transmitters on survival and breeding success of red grouse Lagopus lagopus scoticus. Wildlife Biology 1, 121-126.
| Crossref | Google Scholar |
Troscianko J, Rutz C (2015) Activity profiles and hook-tool use of New Caledonian crows recorded by bird-borne video cameras. Biology Letters 11, 20150777.
| Crossref | Google Scholar | PubMed |
Tuyttens FAM, Macdonald DW, Roddam AW (2002) Effects of radio-collars on European badgers (Meles meles). Journal of Zoology 257, 37-42.
| Crossref | Google Scholar |
Van der Weyde LK, Blumstein DT, Letnic M, Tuft K, Ryan-Schofield NL, Moseby KE (2023) Can native predators be used as a stepping stone to reduce prey naivety to novel predators? Behavioral Ecology 34, 63-75.
| Crossref | Google Scholar |
Venturato E, Cavallini P, Banti P, Dessì-Fulgheri F (2009) Do radio collars influence mortality and reproduction? A case with ring-necked pheasants (Phasianus colchicus) in central Italy. European Journal of Wildlife Research 55, 547-551.
| Crossref | Google Scholar |
West R, Letnic M, Blumstein DT, Moseby KE (2018) Predator exposure improves anti-predator responses in a threatened mammal. Journal of Applied Ecology 55, 147-156.
| Crossref | Google Scholar |
White CR, Cassey P, Schimpf NG, Halsey LG, Green JA, Portugal SJ (2013) Implantation reduces the negative effects of bio-logging devices on birds. Journal of Experimental Biology 216, 537-542.
| Crossref | Google Scholar | PubMed |
Wilmers CC, Nickel B, Bryce CM, Smith JA, Wheat RE, Yovovich V (2015) The golden age of bio-logging: how animal-borne sensors are advancing the frontiers of ecology. Ecology 96, 1741-1753.
| Crossref | Google Scholar | PubMed |
Zhou J, Yan X, Ryan DH, Harris RBS (1999) Sustained effects of repeated restraint stress on muscle and adipocyte metabolism in high-fat-fed rats. American Journal of Physiology-Regulatory, Integrative and Comparative Physiology 277, R757-R766.
| Crossref | Google Scholar |