The impact of irrigation duration on crop N uptake, lint yield and internal NUE in cotton using standard urea
John Smith
A
B
Abstract
This is the first research to investigate the impact of irrigation duration on crop productivity and nitrogen (N) performance indicators in southern New South Wales (SNSW), Australia, as the industry strives for improved N productivity.
To benchmark the impact of different irrigation durations on waterlogging and related impacts on available soil and fertiliser N, cotton productivity and nitrogen use efficiency (NUE).
Two field experiments were conducted in SNSW where cotton was grown with varying rates of fertiliser N application interacting with different durations of furrow irrigation applications during the period from first flower to crop maturity.
Waterlogged conditions occurred within the top 20 cm of the soil profile during irrigations. These conditions coincided with fertiliser placement and high concentrations of available mineral N, which created conditions conducive for N loss. However, the internal NUE (kg lint kg crop N uptake−1) and N balance were not impacted by irrigation duration in either experiment, despite differences in duration of waterlogged conditions that averaged 15 h irrigation−1. Partial N budgets suggested that 48% of the available N to the crop could not be found in the plant or soil mineral N pools after harvest.
Modification of the duration of irrigation applications was not an effective management option to improve NUE in irrigated cotton; however, it improved water productivity (bales per megalitre).
Further research is required to consider the implications of other irrigation systems such as overhead sprinkler and drip systems for improvement of NUE.
Keywords: cotton, crop N balance, internal NUE, irrigation, nitrogen use efficiency, nitrogen fertiliser, partial N budget, waterlogging.
Introduction
Approximately 82% of the area sown to cotton (Gossypium hirsutum L.) in Australia each year is irrigated, with variation between seasons primarily due to availability of water for irrigation (Cotton Australia 2016). Surface irrigation predominates in the Australian cotton industry, with flood-furrow irrigation used across 90% of the irrigated cotton area and the remainder utilising overhead sprinkler irrigation (ABARES 2019). Furrow irrigation offers advantages over other systems due to comparatively lower initial capital requirements and low energy and maintenance costs (Koech et al. 2014). However, irrigated cotton growers are looking for ways to increase the economic and agronomic efficiency of surface irrigation systems due to the high labour requirements, rising cost of energy and low water use efficiency of these systems (Grabham 2012). A challenge for surface-irrigation of cotton in Australia is the concentration of production on soils which have inherent physical and chemical characteristics that result in low internal drainage rates. These characteristics increase the frequency of waterlogging events following irrigation (Milroy et al. 2009), with cotton reported to be particularly sensitive to waterlogged conditions. Waterlogging events have been reported to reduce lint yield by between 10% (Bange et al. 2004) and 40% (Hodgson and Chan 1982). The duration of waterlogging events can be exacerbated by poor soil structure, combined with excessive field length, inadequate slope, uneven levelling and bed formation and substantial rainfall after an irrigation (Bange et al. 2004).
Nitrogen (N) fertiliser is a key driver for productivity of irrigated cotton systems in Australia and N application rates have increased to support higher lint yields in an attempt to maximise gross margins (Macdonald et al. 2017). However, irrigation has been shown to influence the amount of fertiliser N losses through gaseous loss, surface runoff and deep drainage pathways, with peak N losses typically occurring after irrigation events (Macdonald et al. 2015). The proportion of fertiliser N lost as nitrous oxide (N2O) has been shown to increase exponentially as fertiliser N application rates increase (Grace et al. 2016). High gaseous N losses are mainly the result of denitrification, which occurs under conditions of low soil oxygen availability that typically correspond with high soil water contents, with denitrifying bacteria using nitrate (NO3−)-N as an alternate electron acceptor [Firestone and Davidson (1989) cited in Jamali et al. (2016) and Redding et al. (2016)]. Given that soil redox potential and oxygen levels are regulated by soil water content (Jamali et al. 2016), manipulation of the timing and quantity of irrigation water may provide an opportunity to mitigate gaseous losses (Scheer et al. 2013). However, it is important that any changes to the design of surface-irrigation systems maintain a balance between the rapid completion of irrigations that apply the target depth of water and refill the soil water profile, and rapid drainage of excess water from the field to expedite recovery from waterlogging (North et al. 2010).
There has been little work comparing the impact of irrigation management on crop N recovery and fertiliser N loss in single field-based studies in irrigated cotton (Macdonald et al. 2018), and particularly in terms of the N performance metrics that would indicate whether yield limitations caused by waterlogged conditions were due to prevention of N uptake by the plant, or to poor conversion of accumulated N into lint yield, i.e. internal nitrogen use efficiency (NUE; kg lint kg biomass N−1). Ballester et al. (2021) assessed the impact of irrigation frequency, with total water quantity applied varying by an estimated maximum of <10% within each season, on the partial factor productivity of N (kg lint kg applied N−1) and internal NUE (iNUE, kg lint kg biomass N−1) in southern NSW (SNSW), showing that there were considerable seasonal and irrigation management interactions in both parameters. However, the extent of waterlogging in each situation and the role that it played in the various N performance metrics was not assessed. Research conducted in northern NSW by Hodgson and Chan (1982), Constable et al. (1990) and Bange et al. (2004) considered the physiological impacts of waterlogging on growth and N uptake but not the impact on N performance metrics. There is also a growing amount of work that discusses N emissions, particularly N2O, that are generated in response to different irrigation management, such as that by Scheer et al. (2013) and Macdonald et al. (2016) in cotton and by Jamali et al. (2015) in sorghum and wheat (Jamali et al. 2016). However, none directly discuss the impacts on agronomic and physiological N performance metrics. Our study used different irrigation durations (the time in which the siphons applied water to the field) to vary the potential periods of waterlogging following irrigation and to assess the impact of this management strategy on crop biomass N accumulation and lint yield at two flood-furrow surface-irrigated sites in SNSW. In addition to agronomic performance, irrigation treatments were assessed for their impact on the conversion of accumulated crop N into lint yield (iNUE) and the proportion of soil and fertiliser N at sowing that could be accounted for in the plant and soil mineral N pools at the end of the season.
Materials and methods
Field experiment sites
The data were collected from two field experiments in SNSW in the 2016/17 and 2017/18 growing seasons, where irrigated cotton was grown under flood-furrow irrigation. The flood-furrow system applied water from a supply channel via siphons, with water traversing the length of the field in defined furrows and infiltrating soil between the furrows.
The crop at each site was grown on 1.8 m wide raised beds with two rows of cotton per bed with a 1 m spacing between the plant rows. Experiment 1 was planted at the Yanco Agricultural Research station on a Red Chromosol, following two and a half years of ryegrass-dominant pasture, with the pasture sprayed out in August before paddock preparation for the cotton crop commenced. Experiment 2 was on a commercial farm near Darlington Point on a Grey Vertosol following cotton in the previous summer cropping season (i.e. back-to-back cotton) with an approximate 6-month fallow period between crops during which paddock preparation was completed. The location and soil characteristics, including soil classification (Isbell 2016), are provided in Table 1.
Site | Soil type A | Previous crop | Slope (%) | Pre-season mineral N | pHw | pHCa | P(Colwell) (mg/kg) | CEC (cmol(+) kg−1) | Al(KCl) (cmol(+) kg−1) | EC1:5 (ds/m) | Chloride1:5 (mg Cl/kg) | OC (%) | |
---|---|---|---|---|---|---|---|---|---|---|---|---|---|
Experiment 1 | |||||||||||||
Yanco (34°60′S 146°41′E) | Red Chromosol | Pasture (grass) | 0.07 | 78 | 6.9 | 6.0 | 39 | 16.4 | <0.1 | 0.06 | <10.0 | 0.9 | |
Experiment 2 | |||||||||||||
Darlington Point (34°57′S 146°00′E) | Grey Vertosol | Cotton | 0.06 | 176 | 7.3 | 6.7 | 67 | 28.8 | – | 0.23 | 49 | 0.9 |
CEC, cation exchange capacity; EC, electrical conductivity.
Pre-season soil samples to quantify background soil N status were taken to a depth of 90 cm and analysed in 30 cm increments down the profile: 0–30, 30–60, and 60–90 cm. Soil samples were analysed using standard methods described in detail in Rayment and Lyons (2011). Specifically, the 0–30 cm sample was analysed for Colwell P (Method 9B2) and Phosphorus Buffering Index (PBI; Method 912b); pH in water (Method 4A1) and CaCl2 (Method 4B1); exchangeable cations – NH4OAc (Method 15D1) and exchangeable Al3+ (KCl) – Method 15G1; electrical conductivity and chloride (1:5 soil/water) – Method 3A1; and Walkely and Black organic carbon (Method 6A1). Soil mineral N (ammonium- and NO3−-N) concentrations were quantified for all profile layers using KCl extraction (Method 7C2b). The pre-season samples were taken from two locations within the experimental area and were bulked together for analysis. Post-season soil samples were collected from three replicates of selected N rates within each irrigation duration in Experiment 1 before the samples from each N rate within each irrigation treatment were combined and a subsample analysed. A similar post-season sampling strategy was employed in Experiment 2, with only two fertiliser rates sampled but each replicate sample for N rate and irrigation duration was analysed separately. The pre-season and post-season mineral N concentrations were converted to a mass (kg mineral N ha−1) for each profile layer using estimated site bulk densities and then summed to provide profile totals in the top 90 cm.
Field experiment site management
The variety 746B3F, a full season variety with Bollgard® 3 and Roundup Ready Flex® traits incorporated (Cotton Seed Distributors 2024), was grown at both sites. Experiment 1 was planted on 26 October 2016 and Experiment 2 on 10 October 2017. Pest and weed control at each site was managed with consideration of the insect thresholds within the industry’s Insecticide Resistance Management Strategy and herbicide application limitations within the Bollgard Resistance Management Plans (CottonInfo 2020). Application of plant growth regulators and timing of defoliation was determined by monitoring vegetative growth rate, estimating cut-out (when the crop stops producing new fruiting sites) and determining nodes above white flower (the number of nodes above the last white flower on the main stem) (CottonInfo 2020). Chemical defoliation commenced when 60–65% of the bolls were open (Bange 2013), with up to four chemical defoliant applications required to enable harvest (picking) of the seed-cotton (a combination of cotton seed and lint).
Field experiment design
A split-plot design was utilised in both studies, with the duration of irrigation application as the main plots and fertiliser treatments as the subplots. Each main plot consisted of either 10 (Experiment 1) or four (Experiment 2) fertiliser N application rates ranging from very low to supra-optimal, to establish the linear and curvilinear components of the N-response surface at each location. Experiment 2 had additional fertiliser treatment subplots that consisted of different fertiliser application timing and fertiliser products, although only the responses to standard granular urea applied prior to sowing (upfront application) are reported in this paper. The field experiments consisted of four (Experiment 1) and five (Experiment 2) replicate blocks.
In Experiment 1, the N fertiliser treatments were applied on 20 October 2016 before the crop was planted on 26 October 2016 (i.e fertiliser N was applied ‘upfront’). In Experiment 2, the upfront application of fertiliser was on 13 September 2017, and the crop was planted on 10 October 2017.
The different irrigation duration treatments, 9 vs 18 h in Experiment 1 and 12 vs 24 h in Experiment 2, commenced following the early season sampling at first flower and were maintained for each irrigation event for the remainder of the season (i.e. eight irrigation events in Experiment 1 and five in Experiment 2). Experiment 1 was established in a small field with a total field length of 300 m, with the experiment located near the irrigation water supply channel and traversing 220 m down the slope of the field. The 9 h (standard) duration in Experiment 1 was established to work in with other farming operations at the research facility. In Experiment 2, the standard duration (12 h) was based on a typical commercial farming operation for a field with a total length of 450 m. The experiment was located near the irrigation water supply channel and traversed 220 m down the slope of the field. Both experiments were positioned so that irrigation water flowed through the experimental sites and was not affected by water accumulation in the lower slope areas near the water drainage system for each field.
Soil matric potential, measured using Irrometer Watermark sensors, was used to schedule irrigations in both experiments (Yadvinder et al. 2014), with sensors deployed at profile depths of 20, 40 and 60 cm. In Experiment 1 there were two sensors deployed at each depth, while an additional sensor was deployed at the 40 cm depth in Experiment 2. In both experiments, the Watermark sensors were installed in one replication of each irrigation treatment, with data logged every 30 min by a MK Hansen Company am400 soil moisture logger. In Experiment 1, irrigation events for both irrigation durations were initially scheduled when matric potential values in the 9 h irrigation duration treatment reached between −60 and −80 kPa at 20 cm depth, but the 9 h irrigations did not completely refill the soil water profile as the crop advanced through boll development irrigations. Adopting more frequent irrigations in Experiment 1 was not possible due to field access requirements for ground rig operations, as the experiment had been established with access lanes between replications to allow a ground rig to traverse the field without interfering with the experimental plots (Fig. 1). The access lanes were not planted with cotton and so remained wetter than the rest of the field, so the later season matric potential irrigation triggers were extended to values between −120 and −140 kPa. These problems were not experienced in Experiment 2, where a consistent irrigation trigger was able to maintain soil matric potential below −75 kPa at 20 cm soil depth in the 12 h duration treatment for most of the season.
Layout of Experiment 1, conducted in southern NSW in the 2016/17 cotton growing season to investigate the impact of irrigation duration on nitrogen use efficiency in irrigated cotton. Each replication included the 9 and 18 h irrigation durations as the main plots with each main plot labelled. The cut-out sections represent the end of the two central rows that were used for sampling in the determination of crop N uptake and harvested for the determination of lint yield. Photo: John Smith.
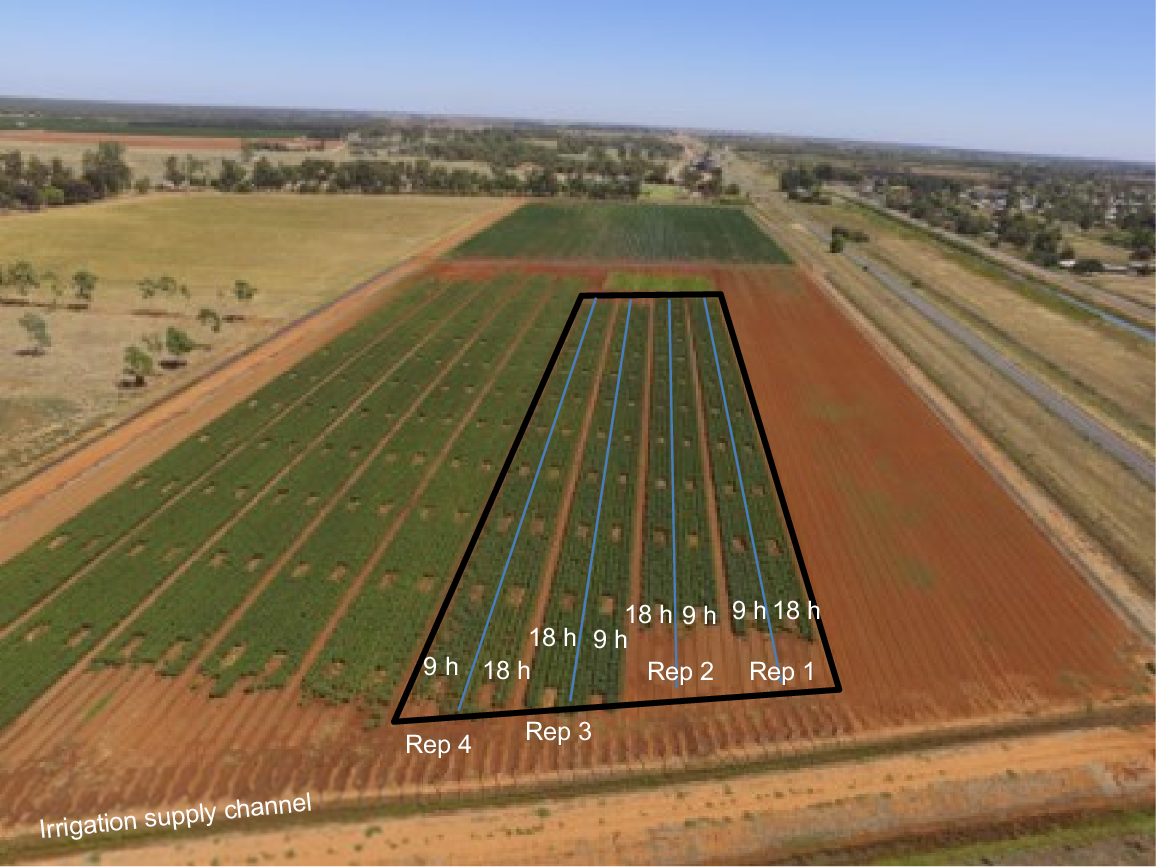
Measurements of the intensity of waterlogging relate to the chemical changes associated with the oxidation–reduction status of the soil environment, and soil redox potential (Eh) has been identified as a suitable measure of these conditions within the soil matrix (Blackwell 1983 cited in Setter and Waters 2003). These studies defined the duration of waterlogging by the period during which Eh fell below 350 mV (disappearance of molecular O2), with a return to aerobic conditions occurring when Eh returned to ≥400 mV after the irrigation (Setter and Waters 2003). Probes to measure Eh were custom-made by Paleo Terra™ and were installed in a subset of irrigation events after first flower of the crop when the different irrigation strategies were implemented. Each Eh monitoring site was established adjacent to the location of the Watermark sensors and consisted of two probes, spaced approximately 3 m apart within the same plant row, with sensors at 10, 20 and 40 cm below the surface of the raised bed. The sensor located at 20 cm was at an equivalent depth to the bottom of the irrigation furrow between the raised beds, and the 10 cm sensors were at a depth equivalent to the mid-point between the bottom of the irrigation furrow and soil surface in the beds. An Ionode IJ-64 platinum wire reference electrode was used in conjunction with the Paleo Terra™ redox probe to complete the required circuit in the soil for the Eh measurement. Redox potential was logged at hourly intervals using a prototype version of the WiField logger (Goanna Telemetry, Goondiwindi, Qld, Australia) which periodically sent data to a cloud-based data storage and internet interface in real time (Brinkhoff et al. 2019).
Plant N analysis
Sampling to determine crop biomass and N uptake was conducted at first flower (prior to commencement of irrigation treatments) and in the week prior to defoliation (maximum N uptake) at both sites. In Experiment 1 the first flower sampling was conducted from a subset of four of the 10 fertiliser application rates (0, 50, 100 and 200 N), while five of the 10 fertiliser application rates (i.e. 0, 100, 150, 200 and 400 N) were sampled just prior to defoliation. In Experiment 2 all plots were sampled at both sampling times.
The biomass samples used to quantify crop N uptake consisted of 1 m of plant row of the selected fertiliser rates on each occasion. Whole plants were cut at the cotyledon node, dried and weighed to determine total dry biomass (kg ha−1). In the late-season sampling, lint was removed from bolls to allow effective grinding, with representative subsamples selected and analysed for total N using Dumas combustion and a LECO analyser (Rayment and Lyons 2011). The lint removed to facilitate sample grinding has been shown to contain a negligible amount of N (Macdonald et al. 2017) and so was assumed to not contribute significantly to total above-ground crop N content.
Once defoliated, the two central rows of each plot were harvested (picked) using a two-row mechanical picker (Rochester and Bange 2016). Seed-cotton weights were recorded for each plot and a subsample (approximately 500 g) taken for determination of the proportions of lint and seed in the sample (turnout) using a 20-saw gin (Constable and Hodgson 1990). The seed from this sample was analysed for total N using Dumas combustion and a LECO analyser (Rayment and Lyons 2011) and used to calculate the total N exported from each treatment at harvest.
Whole-crop N performance metrics
The N performance indicators were based on those reported by Smith et al. (2022). The whole-crop indicators used follow: internal N use efficiency to determine the efficiency at which the crop was able to convert biomass N into lint; (iNUE – kg lint kg crop N uptake−1); a crop N balance describing the net effect of N inputs and N removal from the cotton crop (N balance – N applied in fertiliser minus N removed in cotton seed); and a partial N budget calculated using mass balance: Partial N budget – (pre-season soil mineral N + apparent net N mineralised + applied N rate) − (crop N uptake + post-season soil mineral N).
Irrigation water use index (IWUI)
Water application was measured using galvanised steel flumes installed into the base of the four irrigation furrows that were not compacted by machinery traffic, and represented two replications for each of the two irrigation durations in Experiment 2. Odyssey® water level loggers were mounted on the side of the flume to measure water height during irrigation and converted to water volume (megalitres, ML) using calibration equations based on the dimensions of the flumes. Water application measurements were recorded for the three irrigations prior to first flower when the irrigation duration treatments started. During the irrigation treatment period (i.e. crop stages from first flower to crop maturity) the first two irrigations provided meaningful data. As a result, these irrigations were used to estimate the water application for the other three irrigations during the irrigation treatment period.
The IWUI was used as an index to measure the impact of the different irrigation durations on water productivity. Roth et al. (2013) identified IWUI as a suitable index for the comparison of irrigation management practices within the same season given that it only accounts for water applied. It was calculated as follows:
Statistical analysis
Statistical analysis of the field experiments was conducted using Genstat 19th edition (VSN International 2015). To test for differences in crop N uptake and lint yield and the subsequent N performance indicators resulting from different irrigation durations and the application of fertiliser N, a general analysis of variance was used to assess each experiment separately, except in Experiment 1 where Residual Maximum Likelihood analysis was used for lint yield to better account for spatial variability at the site.
Regression analyses were conducted to fit response surfaces to the crop N uptake and lint yield data using Genstat. An optimum lint yield for each experiment, defined as 95% of the maximum lint yield (Y95) determined from the response to increasing rates of fertiliser N at each site, was derived for each irrigation duration, with the fertiliser N required to produce Y95 for that site–treatment combination determined from the N response function. The relationship between fertiliser N rate and lint yield has been described by quadratic functions in other studies, e.g. Rochester and Bange (2016), but in these experiments the relationships were best described by asymptotic relationships.
Results
Soil matric potential
Soil matric potential data indicated that the 9 h irrigation duration in Experiment 1 was only able to completely replenish the water content of the soil profile until the irrigation of 11 February 2017 (approximately the mid-point of boll development), after which there was a progressive decline in soil matric potential (indicating drier soil) at 20 cm soil depth (Fig. 2). This clearly showed that replenishment of the soil water profile at this depth was not achieved during this shorter irrigation duration. There was greater depletion and replenishment of soil water at 40 and 60 cm soil depths in this treatment, with soil matric potential returning to values below −20 kPa on all except two occasions at the 40 cm profile depth. The greater and more rapid decline in soil matric potential in the intermediate profile layers, particularly 40 cm depth (Fig. 2), indicated that this was where crop water extraction was greatest after each irrigation event. Crop water extraction from the 60 cm soil depth was suppressed for approximately 3 days following each irrigation but became more rapid once the soil matric potential at 40 cm approached −100 kPa.
Soil matric potential in Experiment 1 for the 9- and 18-h irrigation durations (a) and the 12- and 24-h irrigation durations in Experiment 2 (b). Experiments 1 and 2 were conducted in the 2016/17 and 2017/18 cotton cropping seasons, respectively.
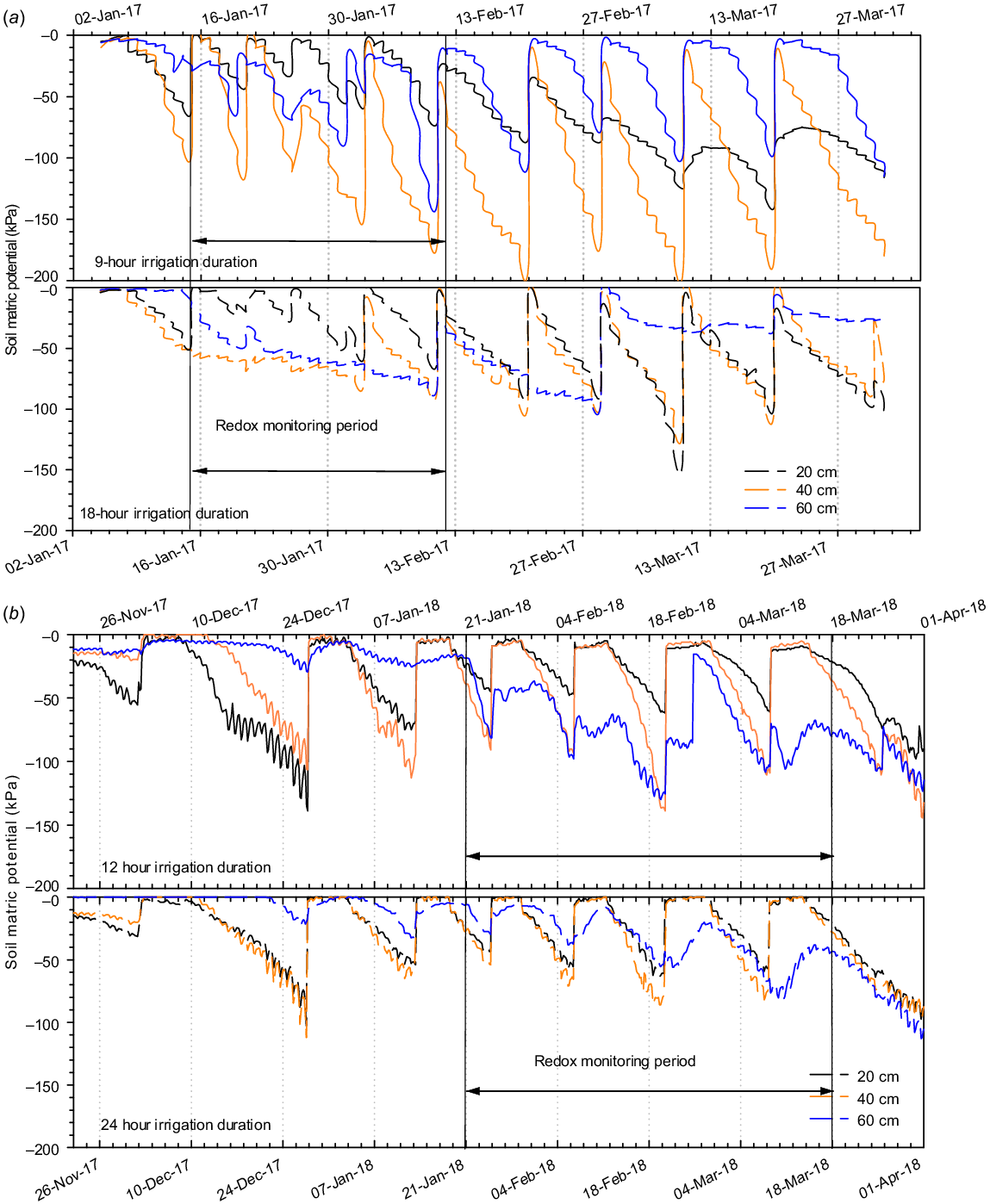
In contrast to 9 h irrigation duration, the 18 h duration was able to refill soil water at 20 cm across all monitored irrigations, with matric potential values reaching between 0 and −20 kPa during each irrigation (Fig. 2), consistent with more water being visible at the surface of the bed in the longer irrigation duration. However, this irrigation strategy appeared less effective at replenishing soil water at the 40 and 60 cm soil depths, with crop water extraction from these depths being minimal until the irrigation in early February, after which crop water extraction at 40 cm became evident. For the remainder of the season crop water extraction from the 20 and 40 cm layers was very similar, with each irrigation able to achieve consistent replenishment of soil water (i.e. soil matric value below −10 kPa). Crop water extraction was minimal at 60 cm in this longer irrigation duration and evidence of penetration of water to this depth during irrigation was intermittent.
The irrigation durations in Experiment 2 (i.e. 12 and 24 h) replenished soil water to near saturation (below −10 kPa) at both the 20 and 40 cm soil depths (Fig. 2). Following each irrigation, the soil matric potential remained near saturation at these soil depths for a period of ~4 days. There was a more rapid decline in the matric potential at 40 cm depth, particularly in the 12 h irrigation duration, indicating greater soil water extraction at this depth that resulted in drier soil conditions between irrigation events (values approaching −100 kPa). Crop water extraction from the 60 cm soil depth did not become significant until late January, with the 12 h irrigation duration progressively less able to effectively re-wet this deeper layer as the season progressed. A similar pattern of increased water extraction at 60 cm was observed in the 24 h treatment, although the longer irrigation duration allowed this deeper soil layer to be more completely replenished by irrigation throughout the season, enabling a greater contribution to crop water extraction.
Redox potential
In Experiment 1, the sensors at 10 and 20 cm depths in the 9 h irrigation treatment showed an average of 12.4 ± 3.4 h (10 cm depth) and 33.9 ± 10.9 h (20 cm depth) of waterlogging (Table 2). Interestingly, while the duration of waterlogging increased by a factor of 2 in the 10 cm soil depth when irrigation duration was increased to 18 h, there was actually less waterlogging indicated (7.7 ± 5.2 versus 33.9 ± 10.9 h) in the 20 cm layer. The increased waterlogging at 10 cm with 18 h irrigation was consistent with the longer period of water infiltration across the bed.
Irrigation duration (h) | Redox sensor depth (cm) | |||||||||
---|---|---|---|---|---|---|---|---|---|---|
Duration (h) of waterlogging (Eh ≤350 mV and returning to ≥400 mV) | Time (h) from 350 mV to lowest mV reading | Time (h) to increase from lowest reading to ≥400 mV | ||||||||
10 | 20 | 40 | 10 | 20 | 40 | 10 | 20 | 40 | ||
Experiment 1 | ||||||||||
9 | 12.4 (±3.7) | 33.9 (±10.9) | 0.0 | 8.3 (±2.5) | 17.9 (±6.4) | 0.0 | 4.1 (±1.7) | 16.0 (±7.7) | 0.0 | |
18 | 26.4 (±6.4) | 7.7 (±5.2) | 0.0 | 16.3 (±4.1) | 6.4 (±4.3) | 0.0 | 10.1 (±2.5) | 1.3 (±1.3) | 0.0 | |
Experiment 2 | ||||||||||
12 | 5.6 (±3.1) | 30.5 (±6.2) | 1.1 (±1.1) | 5.0 (±3.0) | 22.9 (±4.9) | 1.0 (±1.0) | 0.6 (±0.4) | 7.6 (±1.8) | 0.1 (±0.1) | |
24 | 6.9 (±2.6) | 48.1 (±5.9) | 0.0 | 6.5 (±2.5) | 44.3 (±4.9) | 0.0 | 0.4 (±0.2) | 3.8 (±2.0) | 0.0 |
The standard error of the duration for each sensor at each depth is shown in parentheses. Experiments 1 and 2 were conducted in the 2016/17 and 2017/18 cotton cropping seasons, respectively.
Waterlogged conditions in Experiment 2 were experienced for an average of 5.6 ± 3.1 h (10 cm depth) and 30.5 ± 6.2 h (20 cm depth) for each irrigation during the subset of four irrigations that were monitored with the 12 h irrigation duration (Table 2), and 6.9 ± 2.6 and 48.1 ± 5.9 h at the same depths, respectively, for irrigation duration of 24 h. The impact of irrigation duration on waterlogging at this site was quite different to Experiment 1, with short periods of waterlogging at 10 cm that represented only 30–45% of the irrigation duration, and were very similar in both irrigation treatments. The period of waterlogging at 20 cm increased 5–7 times over that recorded at 10 cm and was equivalent to more than twice the irrigation durations (30.5 ± 6.2 h for 12 h irrigation and 48.1 ± 5.9 h for 24 h irrigation). Soil conditions at 40 cm were relatively stable and remained aerobic during irrigation, with only one irrigation event in the 12 h irrigation treatment experiencing waterlogging (Table 2).
The recovery from waterlogging in Experiment 1 was longer compared to Experiment 2 [16.0 ± 7.7 h (47%) versus 7.6 ± 1.8 h (25%)], which was not consistent with the steeper field slope in the field used for Experiment 1 (Tables 1, 2). This suggests possible soil structural characteristics that extended the waterlogging periods at the Experiment 1 site.
Lint yield production
The application of fertiliser N in Experiment 1 provided significant increases in lint yield, with responses maximised at application rates >200 kg N ha−1 (maximum yields of ~3400 kg ha−1 – Fig. 3), after which no further increases were recorded. Irrigation duration did not exert a strong influence on lint yield, although there was a significant interaction with N rate apparently driven by a reduction in lint yield, resulting from the longer irrigation duration where no fertiliser N was applied. Crop yields equivalent to 95% maximum lint yield (Y95) were very similar for the 9 and 18 h durations (3110 and 3010 kg ha−1, respectively), although the fertiliser N rates required to achieve that target were higher for the 9 than the 18 h duration (186 vs 140 kg N ha−1).
The response of cotton lint yield to applied fertiliser nitrogen (N), across two experiments and two different irrigation durations at each site. The blue vertical arrows indicate the fertiliser N application rate required for 95% maximum lint yield, with solid blue arrows representing the standard irrigation duration and dashed blue arrows representing the longer irrigation duration at each site. Vertical bars represent the standard error for each fertiliser N application rate in each irrigation duration. Experiments 1 and 2 were conducted in the 2016/17 and 2017/18 cotton cropping seasons, respectively.
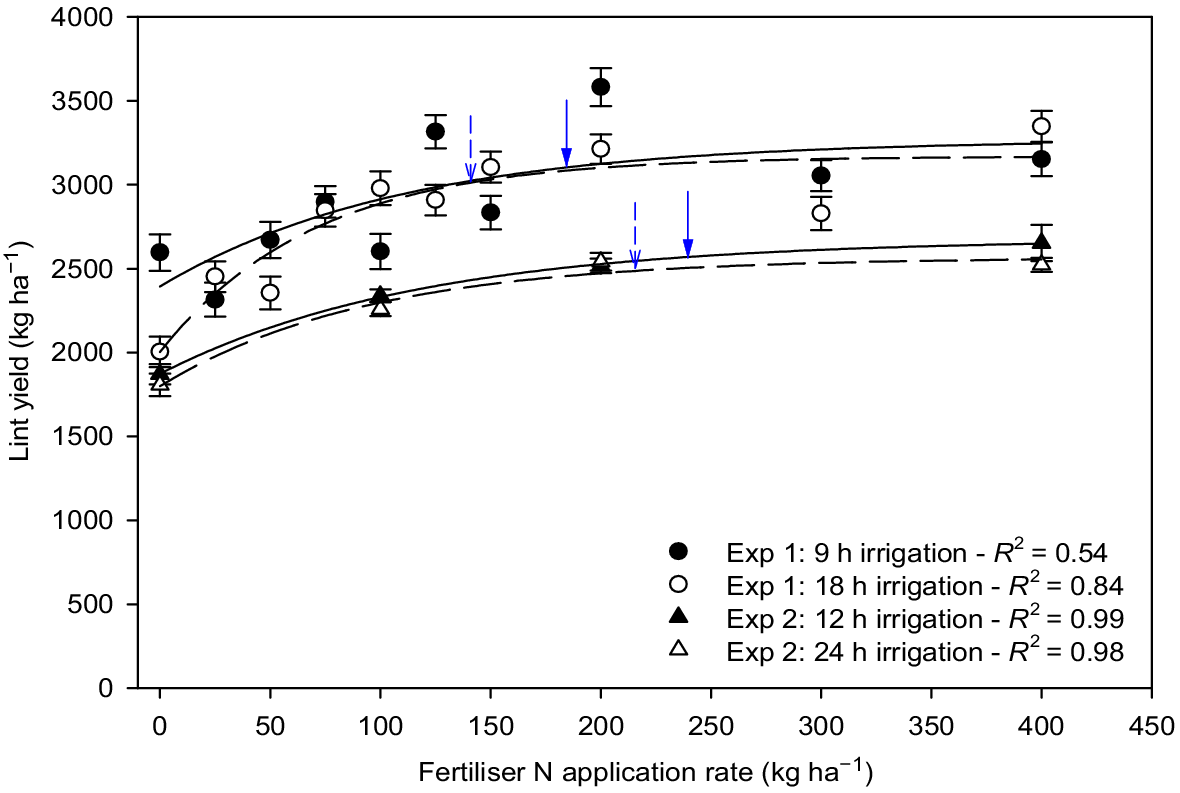
All fertiliser N rates produced significant increases in lint yield relative to the unfertilised control in Experiment 2, albeit with diminishing incremental lint responses as fertiliser rates increased (Fig. 3). There was no impact of irrigation duration on lint yield in this experiment, and the lint yield–fertiliser N response surfaces were very similar for the two irrigation durations. The similarity in relationships between fertiliser N rate and irrigation duration also meant that there was no difference in lint yield where it was optimised for Y95 (2520 and 2430 kg ha−1 for 12 and 24 h irrigation durations, respectively), and the fertiliser N rates required to achieve Y95 were likewise similar (240 and 216 kg N ha−1 for 12 and 24 h durations, respectively).
Crop growth and iNUE
Dry matter production at first flower was not responsive to fertiliser application across the range of fertiliser rates applied in either experiment. However, there was a clear difference between the sites in the early growth of cotton crops, with an average biomass of 2870 kg ha−1 at first flower in Experiment 1 compared to 1740 kg ha−1 in Experiment 2 (Table 3). In Experiment 1, crop extraction of soil N in the unfertilised treatment was 52 kg N ha−1, with the application of fertiliser N resulting in increased crop N accumulation up to application rates of 100 kg fertiliser N ha−1 (i.e. maximum crop N contents of 82 kg biomass N ha−1), after which no further increases in biomass N were recorded. The crop N uptake at first flower in Experiment 2 was not responsive to fertiliser N rate and the average N uptake across all N rates was 51 kg N ha−1, similar to the uptake from soil N alone in Experiment 1 (Table 3).
First flower | Defoliation | iNUE (kg lint kg crop N uptake−1) | Post-season mineral N (kg ha−1) | |||||
---|---|---|---|---|---|---|---|---|
Dry matter | Crop N uptake | Dry matter | Crop N uptake | |||||
(kg ha−1) | ||||||||
Experiment 1 | ||||||||
Fertiliser rate (kg N ha−1) | 9 h | 18 h | ||||||
0 | 2544 | 52a | 10,383a | 138a | 17.4 | 68 | 50 | |
100 | 2991 | 82b | 12,334ab | 183b | 16.6 | 86 | 82 | |
150 | 13,298bc | 204b | 15.5 | |||||
200 | 2892 | 89b | 14,480c | 216bc | 14.2 | 108 | 71 | |
400 | 14,977c | 247c | 13.1 | 180 | 148 | |||
Fertiliser rate (l.s.d. P = 0.05) | Ns | 13 | 1995 | 40 | ns | – | ||
9 h irrigation | – | – | 12,704 | 199 | 14.9 | |||
18 h irrigation | – | – | 13,485 | 196 | 15.7 | |||
Irrigation duration (l.s.d. P = 0.05) | ns | ns | ns | – | ||||
Irrigation.fert rate (l.s.d. P = 0.05) | ns | ns | ns | – | ||||
Experiment 2 | ||||||||
Fertiliser rate (kg N ha−1) | 12 h | 24 h | ||||||
0 | 1759 | 47 | 10,098a | 167a | 11.2c | 77 | 64 | |
100 | 1741 | 49 | 11,597b | 200b | 11.5c | |||
200 | 1722 | 54 | 13,269c | 254c | 10.1b | 122 | 145 | |
400 | 1737 | 54 | 14,583d | 290d | 9.1a | |||
Fertiliser rate (l.s.d. P = 0.05) | Ns | ns | 820 | 17 | 0.8 | 49 | ||
12 h irrigation | – | – | 13,032 | 243 | 10.6 | – | – | |
24 h irrigation | – | – | 13,267 | 253 | 9.9 | – | – | |
Irrigation duration (l.s.d. P = 0.05) | – | ns | ns | ns | ns | ns | ||
Irrigation × fertiliser rate (l.s.d. P = 0.05) | – | ns | ns | ns | ns | ns |
Significant differences in dry matter, crop N uptake and internal N use efficiency (iNUE) resulting from fertiliser and irrigation treatments are represented by superscript letters within each column.
During the period from the first flower to the pre-defoliation sampling, ~80% of total dry matter production in the unfertilised treatments was accumulated, with dry matter production prior to defoliation showing a positive response to application of fertiliser in both experiments (Table 3). In Experiment 1, increases in dry matter were not significant above 150 kg fertiliser N ha−1; however, in Experiment 2 there were significant increases in dry matter production across the range of fertiliser rates used.
Crop N uptake at defoliation in Experiment 1 showed a similar response to that for dry matter production, with a non-significant trend for increasing biomass N in the 200 and 400 kg N ha−1 treatments (Table 3). Crop N contents determined for N rates delivering Y95 were similar for both irrigation durations (215 and 200 kg N ha−1 for the 9 and 18 h durations, respectively), with the additional N accumulated in crop biomass representing fertiliser N recovery efficiencies of 39% and 46% for the 9 and 18 h durations, respectively. In Experiment 2, crop biomass N increased significantly with each additional fertiliser increment to the maximum rate applied (400 kg N ha−1, Table 3), although there was clear evidence of reduced efficiencies of fertiliser N recovery by the crop at rates above 200 kg N ha−1 (Table 3). Crop N contents at N rates delivering Y95 were effectively identical (250 and 247 kg N ha−1 for 12 and 24 h durations, respectively), with the additional N accumulated in crop biomass representing fertiliser recovery efficiencies of 35% and 38% for the 12 and 24 h durations, respectively. Neither study therefore showed any evidence of either a greater fertiliser N requirement to achieve Y95 or a reduced ability to recover the applied fertiliser N in crop biomass in response to longer irrigation duration (Table 3).
The efficiency with which additional biomass N accumulation delivered increases in lint yield declined as the fertiliser application rate increased in Experiment 1, particularly in the 9 h duration, although the decline was non-significant (Table 3). The similarities in lint yield and crop N uptake where the fertiliser input was optimised for Y95 resulted in no effect of irrigation duration and an average iNUE of 14.8 ± 0.4 kg lint kg crop N−1.
In Experiment 2, iNUE was consistently lower than in Experiment 1 and there was a significant decline in iNUE from 11.5 to 9.6 kg lint kg biomass N−1 as fertiliser N application increased above 100 kg N ha−1 (Table 3). However, irrigation duration did not affect iNUE, with 10.5 and 9.9 kg lint kg biomass N−1 for the 12 and 24 h durations, respectively. Once again, the similarities in lint yield and crop N uptake between the irrigation durations where fertiliser input was optimised for Y95 lint yield resulted in no difference in iNUE, with an average 9.8 ± 0.1 kg lint kg crop N−1.
N removal, balance and partial budget
The application of fertiliser N increased the amount of N removed in seed above that from soil N alone in both experiments (Table 4). In Experiment 1, there was a significant interaction between irrigation duration and fertiliser N rate due to greater increases in seed N removal with increasing N rate in the 18 than the 9 h duration (Table 4). This interaction was due primarily to the greater cotton seed yield and resulting N removal in the 9 h duration without applied N (135 kg N ha−1) than in the 18 h equivalent (93 kg N ha−1 – Table 4), with subsequent increases in seed N removal across the range of applied N rates representing only a 23% increase in the 9 h duration but a 94% increase in the 18 h duration. When fertiliser N was applied at rates that delivered Y95 in each duration, the apparent additional removal of fertiliser N in cotton seed represented only 12% of the fertiliser N applied in the 9 h duration, compared to approximately 49% of the applied fertiliser N in the 18 h duration (Table 4).
Experiment 1 | N removal (kg ha−1) | N balance (kg ha−1) | Partial N budget (kg ha−1) | ||||
---|---|---|---|---|---|---|---|
Experiment 1 | |||||||
Irrigation duration (h) | 9 | 18 | 9 | 18 | 9 | 18 | |
Fertiliser rate (kg N ha−1) | |||||||
0 | 135 | 93 | −135 | −93 | 0 | 0 | |
100 | 163 | 159 | −63 | −59 | 38 | 23 | |
150 | 149 | 152 | 1 | −2 | |||
200 | 152 | 174 | 48 | 26 | 91 | 92 | |
400 | 166 | 180 | 234 | 220 | 176 | 196 | |
Seed N at Y95 | 158 | 161 | |||||
Fertiliser rate (l.s.d. P = 0.05) | 16 | 16 | – | ||||
Irrigation duration (l.s.d. P = 0.05) | Ns | Ns | – | ||||
Irrigation.fert rate (l.s.d. P = 0.05) | 22 | 22 | – | ||||
Experiment 2 | |||||||
Irrigation duration (h) | 12 | 24 | 12 | 24 | 12 | 24 | |
Fertiliser rate (kg N ha−1) | |||||||
0 | 87 | 86 | −87 | −86 | 0 | 0 | |
100 | 111 | 115 | −11 | −15 | |||
200 | 132 | 143 | 68 | 57 | 73 | 83 | |
400 | 133 | 149 | 267 | 251 | |||
Seed N at Y95 | 130 | 139 | |||||
Fertiliser rate (l.s.d. P = 0.05) | 8 | 8 | – | ||||
Irrigation duration (l.s.d. P = 0.05) | Ns | ns | – | ||||
Irrigation.fert rate (l.s.d. P = 0.05) | Ns | ns | – |
Optimised lint yield was defined as 95% of maximum yield (Y95).
In Experiment 2, there was no variability in seed N removal between irrigation durations in the unfertilised treatment, with seed N removal across the N rate range increasing by 52% (12 h) and 73% (24 h), and no response to N rates > 200 kg N ha−1 (Table 4). The efficiency with which fertiliser N was removed in harvested product was low, with the additional N removed in seed at N rates required to achieve Y95 lint yields representing only 18% (12 h) and 25% (24 h) of the applied fertiliser N (Table 4).
The irrigation durations in Experiment 1 did not affect the crop N balance, with fertiliser N application rates of ~150 kg N ha−1 required to balance the N removal in cotton seed for both irrigation treatments (Table 4). This was similar to the fertiliser N rate required for Y95 in the 18 h duration (140 kg N ha−1) but the equivalent rate in the 9 h duration (186 kg N ha−1) generated an apparent surplus of mineral N.
In Experiment 2, the relationship between fertiliser N application and removal of N in cotton seed suggested that fertiliser N applications of about 110 kg N ha−1 (108 and 114 kg N ha−1 for 12 and 24 h duration, respectively) were required to balance N removal in cotton seed (Table 4). The rates applied for Y95 in the experiment (i.e. 239 and 216 kg N ha−1 for 12 and 24 h duration, respectively) were much higher than that required for N balance, indicating a large mineral N surplus. Some of this surplus N remained in the soil after the crop was harvested, with increases in the soil profile N compared to the amount of soil N prior to fertiliser N application at the start of the season (Table 3).
An apparent N budget was calculated from the N input and output data across the rates of fertiliser N to investigate the fate of fertiliser that was not accounted for in the cotton biomass and soil mineral N pools. In Experiment 1, analysis across the fertiliser N rates in both irrigation durations showed a strong positive relationship between the rate of fertiliser N applied and the amount of N that could not be recovered in the plant or readily available soil mineral N pools at the end of the season (Fig. 4). The irrigation durations provided slopes that did not differ from each other, and the combined data indicated that only 52% of the applied fertiliser N could be accounted for in crop biomass or in labile mineral N pools in the soil profile at the end of the growing season. We were unable to identify the fate of the remaining 48% of applied N, with immobilisation into the organic N pool and permanent loss pathways such as denitrification, leaching and runoff all possible candidates. Although beyond the scope of this study, the ultimate fate of residual N in crop residues is also uncertain.
Nitrogen (N) that could not be accounted for in the mineral N or crop biomass pools at the end of the growing season as a function of fertiliser N application rate. The circles represent Experiment 1 and squares present Experiment 2. The regression was conducted for the pooled data in Experiment 1 where four fertiliser N rates were sampled and there was no significant difference between the two irrigation durations. Experiments 1 and 2 were conducted in the 2016/17 and 2017/18 cotton cropping seasons, respectively.
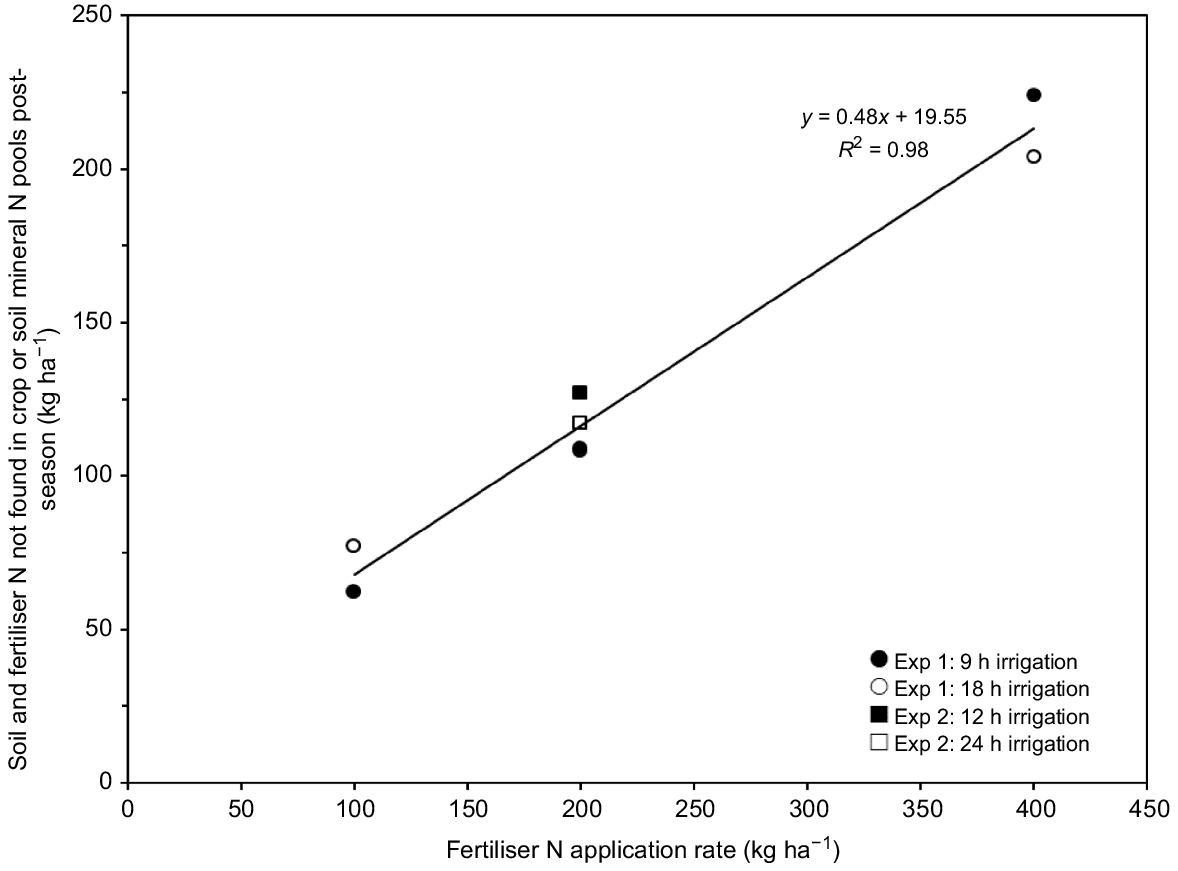
In Experiment 2, a partial N budget was conducted for both irrigation durations with 200 kg N ha−1 applied, and the N that was accounted for in soil mineral N or crop biomass represented 37% and 42% of the fertiliser N application for the 12 and 24 h durations, respectively. These values were similar to those recorded in Experiment 1 at the same fertiliser application rate (~46% – Table 4).
Irrigation water use index
Doubling the irrigation duration from 12 to 24 h from first flower to defoliation in Experiment 2 represented an 86% increase in the volume of water applied, from 3.0 to 5.7 ML across the season (Table 5). At Y95 the shorter irrigation duration (12 h) had a higher lint yield than the 24 h duration (11.10 vs 10.70 bales ha−1), but when this was combined with the greater amount of water applied, there was a 42% decrease in IWUI from 3.65 ± 0.09 to 2.11 ± 0.68 bales ML−1. Although not included in the calculation, 203.5 mm (~2.0 ML) of rainfall occurred during the season, with 174 mm (85%) of this occurring between planting and first flower, i.e. prior to the irrigation duration treatments being implemented.
Irrigation duration (h) | Y95 lint yield (bales) | Estimated seasonal water application A (ML) | IWUI (bales ML−1) | |
---|---|---|---|---|
12 | 11.10 | 3.04 | 3.65 (±0.09) | |
24 | 10.70 | 5.65 | 2.11 (±0.68) |
Discussion
Recent benchmarking of NUE in surface flood irrigation systems in the Australian cotton industry has shown that only 25–30% of the potentially available (soil + fertiliser) N is found in the plant and soil mineral N pools at crop harvest, with the apparent crop recovery of applied fertiliser N much lower than that of soil-derived N (Smith et al. 2022). While the fate of the unaccounted N has not been clearly determined, there is concern that combinations of flooded soils and high soil NO3−-N concentrations, that more typically occur in the early stages of the growing season while crop N demand is low, represent significant denitrification loss risks (Grace et al. 2016; Macdonald et al. 2017; Schwenke et al. 2021). The studies reported here were designed to determine whether contrasting irrigation durations within a flood-furrow irrigation system would impact the efficiency of soil and fertiliser NUE during the period that represented peak N demand by the crop. The irrigation durations were based on the 10–18 h opportunity times recommended for wheat in other surface irrigation systems within the southern Murray Darling Basin (North et al. 2010), with the durations tested either slightly less than or within the optimal range (9 and 12 h) or longer than the recommended range (18 and 24 h). These irrigation durations were much shorter than the 64 and 72 h irrigation durations utilised by Hodgson (1982) and Bange et al. (2004), respectively, to induce differences in plant physiological characteristics and lint yield in northern NSW, but were considered more closely linked to conditions experienced in commercial fields.
It was hypothesised that this range in irrigation durations would change the incidence or severity of waterlogging, and negatively impact NUE, due in part to a reduced yield accumulation. While the objective of reduced waterlogging in the shorter duration treatments was achieved (Table 2), the shorter irrigation duration (9 h) in the Red Chromosol soil in Experiment 1 resulted in incomplete replenishment of the soil water content in soil layers important for crop root activity, and the profile became increasingly dry as the season progressed. This was most pronounced at the 20 cm depth (Fig. 2a) which is the depth typically used to schedule irrigation events in these and other irrigation studies, e.g. Ballester et al. (2021). Water stress can reduce lint yield by 19 kg lint ha−1 day−1 (Constable and Bange 2015) in high yielding cotton crops, but given that lint yield was not significantly different from the longer irrigation duration treatment (Fig. 3) and was above the average lint yield of irrigated cotton in the region (i.e. 2161 kg ha −1 – ABS 2021), it seems that these drier soil conditions did not result in enough water stress or restrict root access to nutrient-rich topsoil layers for long enough to impact productivity. Lint yield in Experiment 2 (average of the irrigation durations at Y95 – 2477 kg lint ha−1) was above average for the region and very similar to the maximum lint yield 2448 kg lint ha−1 achieved by Ballester et al. (2021) under the same farm management.
Fluctuations in soil matric potential highlighted the interactions between root activity and irrigation management on plant-available water within the soil profile. There was greater reliance and extraction of soil water at 40 and 60 cm in the 9 h irrigation duration in Experiment 1, suggesting that despite the ineffective soil water recharge at 20 cm depth, water was able to infiltrate deeper into the soil profile during irrigations. Where longer 18 h irrigation durations were able to maintain relatively higher matric potential in Experiment 1, there was greater soil water extraction by the roots at the shallower soil depths and only minor water extraction and inconsistency in the replenishment of soil water at 60 cm. The reason for this was not clear but suggests that slow lateral wetting in the shorter irrigation duration, particularly later in the season when the cultivated beds were consolidated after several irrigations, restricted the rewetting of the shallow profile layers but still allowed more complete rewetting of deeper profile layer in this Red Chromosol. The more favourable moisture environment in the deeper layers resulted in greater root activity, and hence water extraction, with this pattern further enhanced by the progressive drying of the topsoil over time (Wells and Stewart 2010).
The use of continuously logged soil redox potential to quantify the impacts of different irrigation durations in furrow irrigation cotton was unique in the Australian cotton industry. Other studies have variously used regular soil sampling around irrigation treatment events to allow calculation of the soil air-filled porosity (Hodgson 1982), or measurement of soil water content with a neutron moisture meter or gravimetric sampling (Bange et al. 2004). The data generated from this redox potential monitoring (Table 2) showed that the duration of waterlogging in these raised beds was very different to waterlogging experienced with similar soil types but in basin irrigation layouts with no raised beds in SNSW (North et al. 2010). In those basin irrigation layouts, the development of waterlogged conditions (redox potential < 350 mV) was much slower, taking up to 96 h in some cases, but then persisting for 100–150 h (2.5–3.0 times the irrigation opportunity time) after each irrigation event. This waterlogging duration was effectively twice that observed in the longer treatments at each site in this study (Table 2). The waterlogged conditions created by Hodgson (1982) on a Vertosol in northern NSW persisted for periods up to 12 times longer than the irrigation duration and although direct comparison cannot be made with our studies, it is apparent that irrigation management and field layouts that expedite drainage of water from the field are important factors in management of waterlogging (North et al. 2010). The limited impact of the different waterlogging durations on crop N uptake and lint yield (Table 3), as well as similarities in the N balance and partial N budget (Table 4), suggest that even the shortest waterlogging duration produced conditions that were still sufficient to induce significant loss of N, presumably through denitrification.
Impact of irrigation duration on crop and N productivity
The imposition of the irrigation duration treatments commenced with the first irrigation following first flower appearance, ensuring that crop establishment was unaffected by differences in irrigation management. Neither experiment showed any dry matter responses to N fertiliser application at this time, although crop N uptake significantly increased with fertiliser application rate in Experiment 1 (Table 3). This evidence of early season impacts of fertiliser N applications in Experiment 1 was consistent with the ability of cotton to take up mineral N from banded N fertiliser within the first 70 days after emergence (Boquet and Breitenbeck 2000; Antille et al. 2018), but was not evident in the Vertosol site used in Experiment 2 (Table 3). This was despite similar apparent fertiliser N uptake from the 200 and 400 kg N ha−1 rates prior to defoliation in both experiments (i.e. 78 and 109 kg N ha−1 in Experiment 1 and 78 and 118 kg N ha−1 in Experiment 2, respectively), and suggests delays to fertiliser N access by the cotton crop in the Vertosol – despite similar application methods and timing between the experiments. While these delays, and the reduced biomass production and lint yields at this site, may indicate the involvement of another unidentified factor constraining crop growth at this site, it is also possible that factors related to concentrated urea bands in Vertosols with low rates of diffusion may also contribute to these effects. Janke et al. (2020) and Janke et al. (2021) reported that the slow rates of diffusion out from urea bands in Vertosols results in prolonged conditions of elevated pH, electrical conductivity and aqueous NH3 concentrations in and around the concentrated urea fertiliser bands. Such conditions would have restricted root access and slowed nitrification, and therefore fertiliser N acquisition, in Experiment 2 more so than in Experiment 1 which was conducted on a lighter soil type that allowed greater leaching of N from the fertiliser N application zone.
Maximum crop N uptake, lint yield and iNUE were not influenced by doubling irrigation duration (to 18 h in Experiment 1 and 24 h in Experiment 2) during the flowering and boll development periods in either experiment (Fig. 3 and Table 3). There are no consistent reports about the critical irrigation durations beyond which lint yield starts to be affected. When Hodgson (1982) extended irrigation duration from 4 to 64 h there was a significant reduction in lint yield up to 32 h for each irrigation event, after which lint yields did not decline any further. Bange et al. (2004) also reported variable impacts of irrigation duration and associated waterlogged conditions on lint yield in a series of experiments with irrigation durations of up to 72 h, with an important variable being the height of the planting hill which impacted the stratification of oxygen within the soil profile. Our results showed that within the SNSW production region, increasing irrigation durations out to 18 and 24 h (Table 2) caused waterlogged conditions that persisted for 33.9 ± 10.9 h irrigation event−1 (Experiment 1) and 48.1 ± 5.9 h irrigation event−1 (Experiment 2), but these were not sufficient to induce significant reductions in lint yield. However, irrigation duration is not a simple indicator, with differences in the stratification of waterlogging events occurring due to soil type (Table 2) and presumably other factors related to field micro-relief.
Although extending the irrigation duration did not affect fertiliser N recovery or lint yield, and hence iNUE, our study showed that site-specific or seasonal factors can have a large impact on iNUE with ~50% more lint produced for each kg of crop N uptake in Experiment 1 compared to Experiment 2. Seasonal differences in iNUE were recorded in another study from the same farm as Experiment 2, where variations in irrigation frequency based on different trigger soil matric potentials did not impact iNUE (Ballester et al. 2021). However, there was a ~20% difference in iNUE (i.e. 7.43 ± 1.05 compared to 9.51 ± 0.7 kg lint kg crop N−1) across the two seasons in which Ballester et al. (2021) conducted their studies.
N balance and partial N budget
The quantity of fertiliser required to balance the N removal in cotton seed was not influenced by irrigation duration in either experiment, with the fertiliser input at Y95 generating surpluses of mineral N in three of the four irrigation duration treatments. We were unable to account for 48% of the fertiliser N that was applied at the start of the season in the crop and soil mineral N pools at the end of the season in Experiment 1 (Fig. 4) and 58–63% in Experiment 2 when 200 kg N ha−1 was applied (Table 4). These proportions of fertiliser N loss were comparable to the 47% determined by Scheer et al. (2023) using 15N fertiliser in southern Queensland but on a similar soil type as that used in Experiment 2. While we were unable to determine the fate of the unaccounted fertiliser N (e.g. immobilised in the soil organic N pool, or lost in runoff or denitrification), we identified that waterlogged conditions consistently occurred within the top 20 cm of the soil profile in all systems. This zone contained the highest concentration of mineral N and soil organic matter and was the soil layer into which the fertiliser N was placed. The combination of elevated mineral N, waterlogging and labile organic matter represent a significant risk of gaseous N loss from denitrification, with the loss of soil oxygen through increases in soil water content identified as a key driver of denitrification in N fertilised soils (Barton et al. 1999). This loss pathway was identified as the largest gaseous loss pathway in irrigated cotton production systems in Australia by Rochester and Constable (2000), with recent studies by Macdonald et al. (2017) reporting losses of fertiliser N by this pathway of 38% of the applied N in furrow irrigated cotton in northern NSW. This proportional loss is quite similar to the size of the unaccounted N pool in Experiment 2 in this study, which was conducted on a similar soil type.
Irrigation water use index
Australia is a leader in cotton water productivity on a global scale with levels over twice that of the global average, and the industry remains committed to continual improvement due to increasing competition for access to, and responsible use of, freshwater resources (McLeod 2023). The levels of water productivity achieved in this study (2.11–3.65 bales ML−1) are consistent with those estimated using the linear relationship established by Roth et al. (2013) for IWUI research conducted on commercial farms between 1988 and 2011 and extending to 2017/18. Improvements in IWUI were a function of improved lint yield and reduced water application, with Roth et al. (2013) determining an improvement of 0.09 bales ML−1 year−1. McLeod (2023) also considered that increased irrigation efficiency due to improved irrigation infrastructure and management contributed to the improvements in IWUI. Extending the irrigation duration to 24 h provided better replenishment of soil water (Fig. 2) while extending the period of waterlogging from 30.5 ± 6.2 to 48.1 ± 5.9 h (Table 2) at a soil depth of 20 cm. However, the extended duration of waterlogging did not affect crop N uptake (Table 3) or the amount of N removed in seed and the N balance (Table 4) and there was only a 4% decline in lint yield at Y95, suggesting that the waterlogged conditions did not greatly impact on the key lint yield determining factors of boll number and harvestable bolls (Bange et al. 2004).
Extending the irrigation duration from 12 to 24 h simply resulted in the application of 86% more water, with a concomitant 42% decrease in the amount of lint for each ML of water applied. Reducing or optimising the amount of water pumped is key to reducing energy costs that are second only to crop nutrition as a variable cost in an irrigated cotton gross margin (Welsh et al. 2024).
Conclusion
This study investigated the impact of irrigation management during the flowering and boll development period over two cotton growing seasons on crop productivity, and soil and fertiliser N recovery and use efficiency of urea fertiliser in flood-furrow irrigated cotton in southern NSW. Waterlogged conditions were maintained for time periods that represented approximately twice the duration of the irrigation events. However, differences in duration of waterlogging did not affect crop N uptake, lint yield or the amount of soil and fertiliser N that was unaccounted for at the end of the growing season.
There were clear differences in crop N uptake and lint yield between experiments that suggested that environmental or farm management differences had a greater impact than the differences in irrigation management within each site on the ability of the crop to recover applied N fertiliser and convert crop biomass N into lint yield. Identifying the environmental and site-specific constraints responsible for these large variations seems to represent a more important opportunity to improve NUE in flood-furrow irrigated cotton cropping systems than attempting to refine irrigation management strategies.
An important impact of shortening irrigation durations was the amount of water applied during each irrigation. The resulting increase in the irrigation water use efficiency highlighted the importance of optimising water management as the industry strives to continually improve water productivity to ensure cotton remains a viable use of the most limiting resource within the southern Murray Darling Basin.
Data availability
The data that support this study will be shared upon reasonable request to the corresponding author.
Declaration of funding
This research received direct financial support from the Cotton Research and Development Corporation.
Acknowledgements
The in-kind support from Cotton Seed Distributors, NSW DPI and AgriFutures Australia is gratefully acknowledged. The cooperation from Matt Stott, James Hill and Matt Watson in imposing the irrigation treatments within a large commercial cropping program was essential for this work and is also gratefully acknowledged. Gabby Napier, Craig Hodges and Chris Dawe provided technical field support. Rob Hoogers, James Brinkhoff, Sam North and Don Griffin provided resources for the setup, monitoring and troubleshooting of soil water and redox monitoring equipment.
References
ABARES (2019) Cotton farms in the Murray–Darling Basin. Available at https://www.agriculture.gov.au/abares/research-topics/surveys/irrigation/cotton [Verified 21 October]
ABS (2021) Agricultural commodities, Australia. Available at https://www.abs.gov.au/statistics/industry/agriculture/agricultural-commodities-australia [Verified 4 January]
Antille DL, Pittaway P, Melland AR, Marchuk S (2018) Optimising nitrogen and water interactions in cotton. Cotton Research and Development Corporation, Narrabri, NSW Australia. Available at https://www.crdc.com.au/more-profit-nitrogen
Ballester C, Hornbuckle J, Brinkhoff J, Quayle WC (2021) Effects of three frequencies of irrigation and nitrogen rates on lint yield, nitrogen use efficiency and fibre quality of cotton under furrow irrigation. Agricultural Water Management 248, 106783.
| Crossref | Google Scholar |
Bange MP, Milroy SP, Thongbai P (2004) Growth and yield of cotton in response to waterlogging. Field Crops Research 88(2–3), 129-142.
| Crossref | Google Scholar |
Barton L, McLay CDA, Schipper LA, Smith CT (1999) Annual denitrification rate in agricultural and forest soils: a review. Australian Journal of Soil Research 37(6), 1073-1093.
| Crossref | Google Scholar |
Boquet DJ, Breitenbeck GA (2000) Nitrogen rate effect on partitioning of nitrogen and dry matter by cotton. Crop Science 40(6), 1685-1693.
| Crossref | Google Scholar |
Brinkhoff J, Hornbuckle J, Ballester Lurbe C (2019) Soil moisture forecasting for irrigation recommendation. IFAC PapersOnLine 52(30), 385-390.
| Crossref | Google Scholar |
Constable GA, Bange MP (2015) The yield potential of cotton (Gossypium hirsutum L.). Field Crops Research 182, 98-106.
| Crossref | Google Scholar |
Constable GA, Hodgson AS (1990) A comparison of drip and furrow irrigated cotton on a cracking clay soil 3. Yield and quality of four cultivars. Irrigation Science 11, 149-153.
| Crossref | Google Scholar |
Constable GA, Rochester IJ, Hodgson AS (1990) A comparison of drip and furrow irrigated cotton on a cracking clay soil 1. Growth and nitrogen uptake. Irrigation Science 11, 137-142.
| Crossref | Google Scholar |
Cotton Australia (2016) Cotton production statistics 2016. Available at https://cottonaustralia.com.au [Verified 24th June 2022]
Cotton Seed Distributors (2024) Variety Guide. Available at https://csd.net.au [Verified 20 July]
CottonInfo (2020) 2020 Australian Cotton Production Manual. Available at https://cottoninfo.com.au [Verified 4 January 2021]
Grace P, Shcherbak I, Macdonald B, Scheer C, Rowlings D (2016) Emission factors for estimating fertiliser-induced nitrous oxide emissions from clay soils in Australia’s irrigated cotton industry. Soil Research 54(5), 598 603.
| Crossref | Google Scholar |
Hodgson AS (1982) The effects of duration, timing and chemical amelioration of short-term waterlogging during furrow irrigation of cotton in a cracking grey clay. Australian Journal of Agricultural Research 33(6), 1019-1028.
| Crossref | Google Scholar |
Hodgson AS, Chan KY (1982) The effect of short-term waterlogging during furrow irrigation of cotton in a cracking grey clay. Australian Journal of Agricultural Research 33, 109-116.
| Crossref | Google Scholar |
Jamali H, Quayle WC, Baldock J (2015) Reducing nitrous oxide emissions and nitrogen leaching losses from irrigated arable cropping in Australia through optimized irrigation scheduling. Agricultural and Forest Meteorology 208, 32-39.
| Crossref | Google Scholar |
Jamali H, Quayle W, Scheer C, Baldock J (2016) Mitigation of N2O emissions from surface-irrigated cropping systems using water management and the nitrification inhibitor DMPP. Soil Research 54(5), 481-493.
| Crossref | Google Scholar |
Janke CK, Moody P, Bell MJ (2020) Three-dimensional dynamics of nitrogen from banded enhanced efficiency fertilizers. Nutrient Cycling in Agroecosystems 118(3), 227-247.
| Crossref | Google Scholar |
Janke C, Fujinuma R, Moody P, Bell M (2021) Biochemical changes and distribution of nitrogen from bands of stabilised N-fertilizers in contrasting soils. Geoderma 382, 114770.
| Crossref | Google Scholar |
Koech RK, Smith RJ, Gillies MH (2014) A real-time optimisation system for automation of furrow irrigation. Irrigation Science 32(4), 319-327.
| Crossref | Google Scholar |
Macdonald BCT, Rochester IJ, Nadelko A (2015) High yielding cotton produced without excessive nitrous oxide emissions. Agronomy Journal 107(5), 1673-1681.
| Crossref | Google Scholar |
Macdonald BCT, Chang YF, Warneke S (2016) Potential contributions of surface and ground water to nitrous oxide emissions from irrigated cotton production systems. Agricultural Water Management 168, 78-84.
| Crossref | Google Scholar |
Macdonald BCT, Chang YF, Nadelko A, Tuomi S, Glover M (2017) Tracking fertiliser and soil nitrogen in irrigated cotton: uptake, losses and the soil N stock. Soil Research 55(3), 264-272.
| Crossref | Google Scholar |
Macdonald BCT, Latimer JO, Schwenke GD, Nachimuthu G, Baird JC (2018) The current status of nitrogen fertiliser use efficiency and future research directions for the Australian cotton industry. Journal of Cotton Research 1(1), 15.
| Crossref | Google Scholar |
McLeod M (2023) Identifying the trends and drivers of water productivity in Australian cotton through benchmarking. Cotton Research and Development Corporation. Available at www.insidecotton.com
Milroy SP, Bange MP, Thongbai P (2009) Cotton leaf nutrient concentrations in response to waterlogging under field conditions. Field Crops Research 113(3), 246-255.
| Crossref | Google Scholar |
North S, Griffin D, Grabham M, Gillies MH (2010) Improving the performance of basin irrigation layouts in the southern Murray–Darling Basin. Cooperative Research Centre for Irrigation Futures. Available at www.researchgate.net
Redding MR, Shorten PR, Lewis R, Pratt C, Paungfoo-Lonhienne C, Hill J (2016) Soil N availability, rather than N deposition, controls indirect N2O emissions. Soil Biology and Biochemistry 95, 288-298.
| Crossref | Google Scholar |
Rochester IJ, Bange M (2016) Nitrogen fertiliser requirements of high-yielding irrigated transgenic cotton. Crop & Pasture Science 67(6), 641-648.
| Crossref | Google Scholar |
Rochester IJ, Constable GA (2000) Denitrification and immobilisation in flood-irrigated alkaline grey clays as affected by nitrification inhibitors, wheat straw, and soil texture. Australian Journal of Soil Research 38(3), 633-642.
| Crossref | Google Scholar |
Roth G, Harris G, Gillies MH, Montgomery J, Wigginton D (2013) Water-use efficiency and productivity trends in Australian irrigated cotton: a review. Crop & Pasture Science 64(12), 1033 1048.
| Crossref | Google Scholar |
Scheer C, Grace PR, Rowlings DW, Payero J (2013) Soil N2O and CO2 emissions from cotton in Australia under varying irrigation management. Nutrient Cycling in Agroecosystems 95(1), 43-56.
| Crossref | Google Scholar |
Scheer C, Rowlings DW, Antille DL, De Antoni Migliorati M, Fuchs K, Grace PR (2023) Improving nitrogen use efficiency in irrigated cotton production. Nutrient Cycling in Agroecosystems 125, 95-106.
| Crossref | Google Scholar |
Schwenke G, Nachimuthu G, Baird J, Mercer C, MacDonald B, Bell M, Bischof C, Bai M, Suter H (2021) More Profit from Nitrogen: Enhancing nutrient use in cotton. Cotton Research and Development Corporation, Narrabri, NSW, Australia. Available at https://www.crdc.com.au/more-profit-nitrogen
Setter TL, Waters I (2003) Review of prospects for germplasm improvement for waterlogging tolerance in wheat, barley and oats. Plant and Soil 253, 1-34.
| Crossref | Google Scholar |
Smith J, Fukai S, Bell M (2022) Soil and fertiliser nitrogen performance indicators for irrigated cotton in Australia. Soil Research 61(4), 329-344.
| Crossref | Google Scholar |