Determining the depth and rate of soil movement down the soil profile using an environmental tracer: a hillslope scale assessment
G. R. Hancock
A
B
Abstract
Soil materials can be delivered to depth from both in situ and ex situ materials. Here, we examine a hillslope in an agricultural environment that has been used for cropping and cattle grazing for over 150 years and a parallel area where cattle have been excluded for approximately 20 years. The exclusion area is a shelterbelt and also provides ecological services.
To quantify the depth and rate of down profile soil movement using the environmental tracer 137Cs at points along a hillslope profile.
137Cs concentration is measured to bedrock at regular intervals both inside and outside a fenced of ecological services area pre-drought (2015) and post-drought (2021). In Australia. 137Cs is the by-product of nuclear weapons use and testing from1945 to 1972. Therefore, this places an age constraint on any labelled soil.
Results show that soil materials can move down the soil profile to reach bedrock at decadal time scales. An important finding is that materials from the surface can reach depths of up to 80 cm near the hillslope crest and up to 2.2 m at the base of the hillslope.
This demonstrates a relatively rapid translocation of surface material.
The method provides the ability to quantify the rapid movement of soil components and demonstrates the potential for deep sequestration of soil organic carbon. The results demonstrate the potential for soil amendments and agrochemicals to be rapidly transported to depth. The findings suggest that cattle exclusion has no impact on the movement of soil materials down the profile over the 20-year exclusion period.
Keywords: 137Cs, carbon sequestration, cattle grazing, ecological services, environmental tracer, exclusion area, pedogenesis, shelterbelts.
Introduction
Pedogenesis is a complex process that is site specific and related to underlying geology, climate and time (Jenny 1941). Soil formation is generally thought of a point-based process, however, formation will vary along a hillslope as a result of erosion and deposition and soil hydrology resulting in variable soil depth (i.e. the soil catena) (Knighton 1998). Soils will therefore evolve by a combination of in situ processes as well as materials being delivered from upslope with some material being lost downslope (Jenny 1941; Willgoose 2018).
The movement of soil materials along the hillslope and down the soil profile operates at a number of scales. At the hillslope scale soil erosion and deposition processes transport material in a semi-continuous process, from top of the hillslope to the bottom via a combination of rainsplash and fluvial mechanisms (here aeolian processes are excluded). In agricultural environments soil is also exhumed, displaced and mixed by tillage (Van Oost et al. 2006). While at the agricultural field scale, tillage erosion destroys the soil profile, mixes soil and translocates soil. This gives rise to changes in surface hydrology and geomorphic processes (De Alba 2001, 2003) and changes in hillslope connectivity. The effects of this new disturbance may only become evident in decades post disturbance (De Alba 2001, 2003; De Alba et al. 2004; Van Oost and Govers 2006; Van Oost et al. 2006).
The movement of soil operates in a spasmodic process in response to rainfall events. The movement of soil down the hillslope has often been described as a ‘sediment slug’ (Knighton 1998; Willgoose 2018) as bodies of sediment move in a slug like progression down the hillslope. This process ultimately results in the concept of the soil catena where soils are generally less deep at the top of a hillslope and progressively increase in depth as distance down the hillslope increases. It is recognised that biogenic agents, such as pigs, disturb soil while seeking food and this surface disturbance changes the hydrological and sedimentological connectivity of the surface. The pits produced by the pigs can act as sediment and organic matter traps (a sediment sink) while the excavated soil can divert upslope water and sediment away from the pits (as pigs usually push excavated soil upslope) and the excavated material diffuses into the surrounding landscape. Therefore, local hillslope connectivity is changed. This process will be ongoing and likely dynamic depending on climate and pig numbers (Hancock and Lowry 2021).
At any point on the hillslope, material can removed from upslope, lost downslope to erosion processes, with the hillslope being the net result of these processes over geological time. There are also subsurface processes where there is a movement of material both up and down the soil profile. In terms of material moving up the soil profile biogenic processes such as burrowing and foraging animals, insects (i.e. ant and termites) and tree throw can bring materials to the surface as well as deliver materials to depth (Butler 1995, 2018; Hancock and Lowry 2021).
Soil materials can move vertically down the soil profile by processes that include diffuse clay illuviation and solutes moving along a wetting front. More immediate or direct advective type processes such as soil cracks can provide direct access to the soil subsurface (Wells et al. 2012, 2013; Wells and Hancock 2014; Hancock 2023a). Diffusive processes are mostly controlled by soil texture with clay content being a major influence. Advection is a more rapid transport material via soil voids or cracks (Wells and Hancock 2014). Other biogenic controls such as decaying tree roots and or animal burrows can provide conduits for a concentrated movement of material (Butler 1995, 2018).
These processes are generally well understood qualitatively with a large body of theory (Butler 1995, 2018; Paton et al. 1995; Wells et al. 2012; Wells and Hancock 2014; Willgoose 2018). However, for the majority of sites and soil types, the processes and rates of material movement are only qualitatively known with a quantitative understanding yet to be fully developed (Willgoose 2018). Improved understandings of the processes and rates of the vertical movement of soil materials would enhance soil management and increase agricultural productivity.
This study examines the migration and rate of surface material movement down the soil profile using the anthropogenic isotope 137Cs as a tracer. Several other authors have employed environmental tracers such as 137Cs to examine the vertical movement of soil components (Wells et al. 2012; Gaspar and Navas 2013; Jagercikova et al. 2014; Wells and Hancock 2014; Dalzell et al. 2022). 137Cs in the local environment is a result of the atmospheric testing and use of nuclear weapons between 1945 and 1972 when atmospheric testing ceased. 137Cs when released high into the atmosphere migrated around the globe where it fell out via rainfall. When falling on soil, 137Cs binds strongly with the clay fraction on the soil surface (Loughran 1994; Loughran et al. 2002). This unique anthropogenic chemical and its affinity with clay has allowed it to be used as an environment tracer to track the movement of soil as well as determine soil erosion and deposition rates (Walling and He 1999, 2001; Zapata 2002; Zapata et al. 2002; Walling et al. 2011; Hancock et al. 2019; Gibson et al. 2022).
Here, the depth distribution of the environmental tracer 137Cs is examined at regular depth increments to bedrock. The depth and pattern of 137Cs distribution allows rates and patterns of down profile soil movement to be determined. The study focusses on a section of hillslope where cattle are commercially grazed and an adjacent area where has been fenced off from cattle for 20 years to provide ecological services. The work builds on that of Hancock (2023a).
The aims of this paper are to: (1) examine soil properties at the hillslope scale and the movement of soil material down the soil profile to bedrock; (2) estimate the rate of down profile movement of material; and (3) advance understandings of the effect of landscape management and other influences such as cattle management and cattle exclusion areas on down profile soil movement.
Materials and methods
Study site
The study site (Stanley), located approximately 22 km west of Merriwa, NSW, Australia is a commercial farm with cattle grazing being the dominant landuse (Fig. 1). The site has been well described elsewhere (Hancock and Vallely 2020). In summary, the property has been used for cropping, but cattle grazing has dominated since the early 2000s (Martinez et al. 2010). The local catchment and property is comprised of gently undulating and rolling hills with an elevation range of 300–450 m (Story et al. 1963). The property and catchment has been a focus of several studies investigating landscape evolution and soil erosion, soil moisture and soil C surveys (Martinez et al. 2009, 2010; Hancock and Coulthard 2012; Hancock et al. 2015).
Location of the Stanley study site with elevation contours (right). Aerial imagery of the shelterbelt in 2019 (red box, bottom panel).
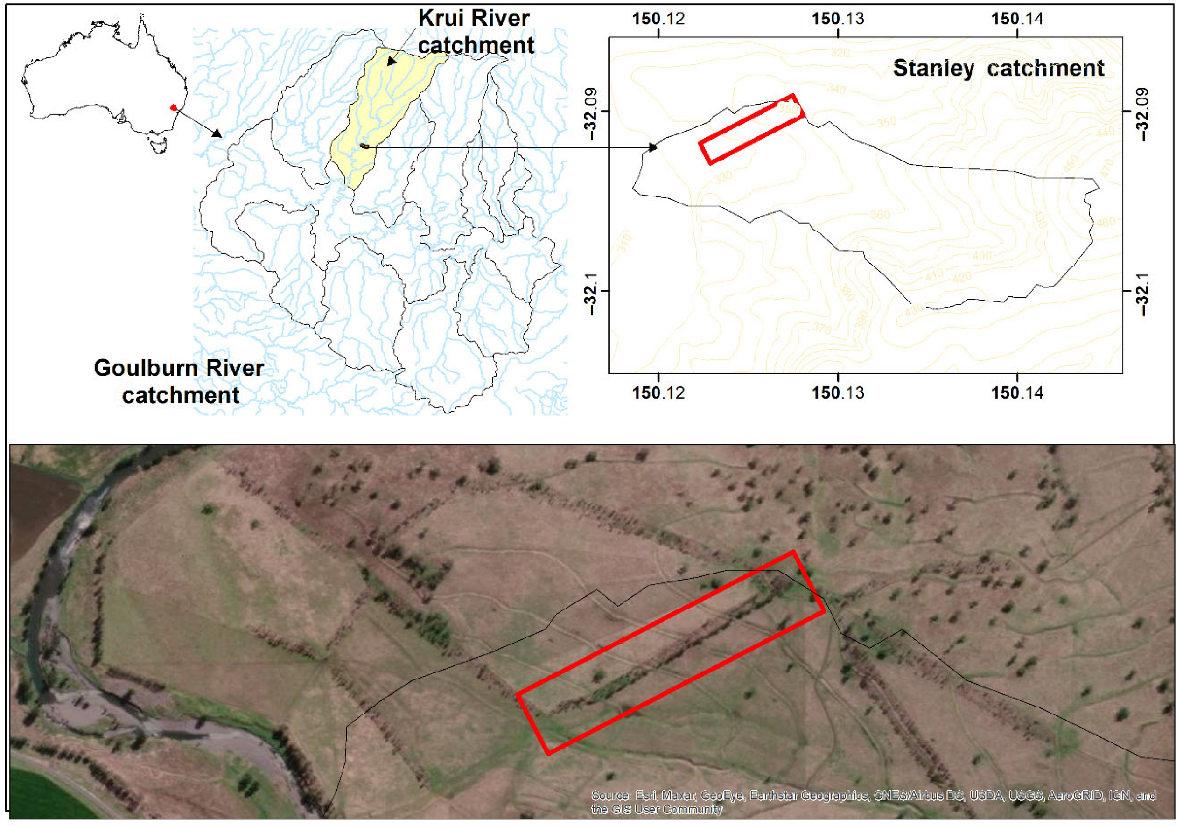
Soils are derived from volcanic geology (Kovac and Lawrie 1991). The major soil types present within the landscape are Chocolate Soils and Euchrozems (Lixic Ferralsol), Black Earths (Chernozem) and Red Clays (Kovac and Lawrie 1991) with high clay content, a large shrink-swell capacity and poor horizon development (Charman and Murphy 2007). The soils are predominantly black and red earths that are derived from the underlying basalt.
The climate is classified as having a subtropical to temperate with mean annual rainfall of 603 mm (1969–2017) (www.bom.gov.au). Mean annual maximum temperature is 23.8°C and the mean annual minimum is 9.1°C. Of particular note is the area was subject to drought from 2016 to 2019, with 2019 having the lowest rainfall on record for the area (Fig. 2). The previous 10 years had average annual rainfall.
Annual rainfall from the nearest Bureau of Meteorology site (Roscommon – (www.bom.gov.au, site number 61287). The dotted line represents annual average rainfall.
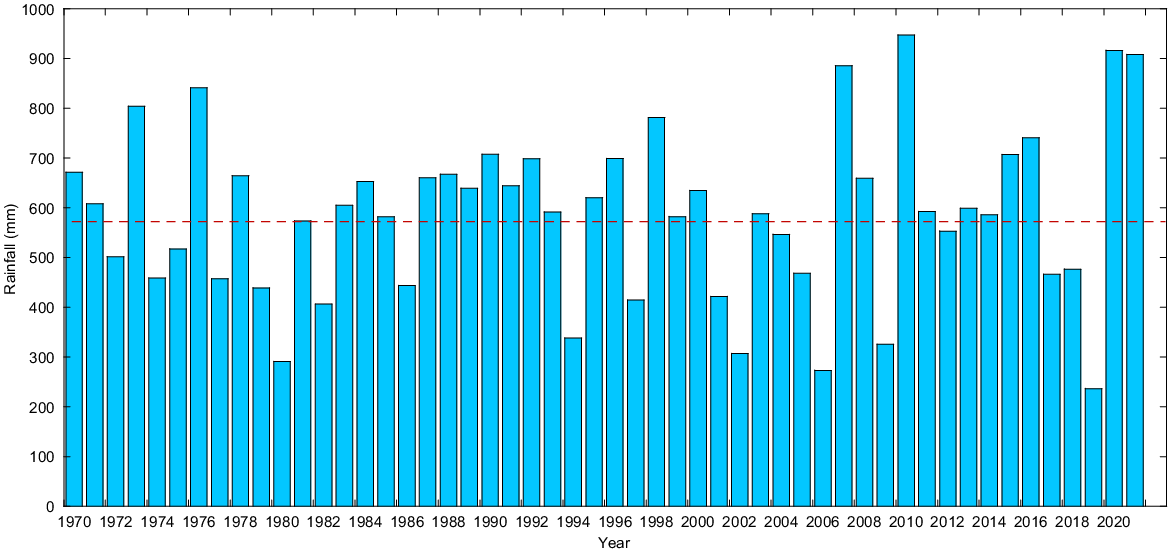
Vegetation is dominated by grasses with native grass species prevalent in the grazing paddocks include plains grass (Austrostipa aristiglumis), wiregrass (Aristida ramose), wallaby grass (Danthonia spp.), red grass (Bothrochloa macra) and blue grass (Dicanthium spp.) (Martinez et al. 2010).
The study here examined two areas: (1) a hillslope where cattle are grazed commercially; and (2) a fenced-off area that excludes cattle and is a ‘shelterbelt’ or cattle ‘exclusion area’ to provide ecological services. The two sites are adjacent to each other and extend for approximately 420 m in a NE to SW orientation from the catchment divide to the bottom of the slope on a creek flat (Figs 1 and 3). Width of the exclusion area is approximately 30 m. Elevation range is 358–381 m with an average slope of 5.5%. The ecological services area was fenced off in 2002 while the external area is commercially grazed. Further detail can be found in Hancock and Vallely (2020).
Field sampling
Sampling at the site was undertaken between May and December 2015 (Hancock and Vallely 2020) with repeat sampling in November and December 2021. The aim was a hillslope scale assessment where samples were collected both Inside and Outside the exclusion area at the Upper, Middle and Lower positions of the hillslope.
The whole soil profile was accessed with trenches excavated by backhoe both ‘Inside’ and ‘Outside’ of the exclusion area to bedrock at ‘Upper’, ‘Middle’ and ‘Lower’ slope positions (0.8–2.2 m soil depth). Samples were then collected in 20-cm intervals from the side of the trench to the maximum soil depth (bedrock) at each sampling site utilising 9.3-cm diameter × 21-cm long cm steel cores.
Laboratory analysis
Each soil sample was weighed and air-dried in an oven at 40°C for 7 days at the University of Newcastle soil laboratory. Samples were then weighed and returned to the oven for a further 24 h (or longer) then weighed to ensure all moisture was removed. Samples were then disaggregated with a mortar and pestle, passed through a 2 mm sieve and the fine earth or soil (<2 mm) and rock fragment (>2 mm) fractions were separated (Loughran 1994; Rayment and Lyons 2010).
137Cs method and measurement
137Cs technique is an environmental tracer that is widely used for a range of applications (Ritchie and McHenry 1975; Zapata 2002; Zapata et al. 2002). 137Cs (half-life, 30.1 years) is an artificial radioisotope that is rapidly and strongly adsorbed to fine soil particles upon reaching the Earth’s surface, thus making it an ideal soil tracer to qualitatively and quantitatively assess soil redistribution rates. The method has been extensively employed in the study region to examine both soil erosion and soil organic carbon (SOC) transport (Martinez et al. 2009, 2010; Wells and Hancock 2014; Hancock et al. 2015, 2019). Due to its uniqueness in the environment and its delivery to the environment between 1945 and 1972, it can be used to provide an age constraint on soil material (Hancock 2023a).
The University of Newcastle houses two p-type high purity germanium (HPGe) gamma ray detectors that were installed in 1992. Both machines are GEM p-type HPGe detectors produced by ORTEC (Model Numbers GEM-35190-P; GEM35P4-70). For 137Cs analysis, the <2 mm soil fraction with a mass of 1000 g were placed in a Marinelli beaker. The beaker containing the soil was then inserted into the gamma detector chamber. Sample count times were approximately 86,400 s (i.e. 24 h), with counting errors in the order of +/- 10% or less achieved (one s.d.) with concentration of 137Cs calculated using the equation and methods outlined by Loughran (1994) and Loughran et al. (2002).
Results
Climate and land management
From establishment of the exclusion area in 2002–2021, the study area has been subject to a variable rainfall. The Millennium Drought (Kiem et al. 2016) occurred between 1997 and 2010, followed by average rainfall conditions until 2016 (Fig. 2). From 2017, a drought occurred with well below average rainfall in 2018 and 2019 with biomass visibly reduced. Above average rainfall occurred in 2020 and 2021. For both droughts, the property was destocked when feed quantity and quality became economically and environmentally unsuitable. Cattle were reintroduced in 2020 when sufficient pasture had regrown (Fig. 3). At no time were cattle allowed into the exclusion area.
Soil depth and physical properties
Excavations to bedrock were conducted in 2015 and 2021 both Inside and Outside the exclusion area. The 2021 sample sites were located approximately 5 m distant from the 2015 sites to ensure that there was no disturbance from the earlier excavation. As there were no trees outside of the shelterbelt, for consistency, all excavations were conducted well away from trees in the exclusion area. The sampling in the shelterbelt was in the centre of the area while the outside sampling was approximately 20 m away from the exclusion area fence.
The excavations demonstrated that soil depth increased moving down the hillslope (Table 1). Soil depth varied from 80 to 120 cm at the top of the hillslope, 100–140 cm at the middle, and 180–220 at the hillslope bottom. Both Inside and Outside the shelterbelt had similar soil depths at the Upper, Middle and Lower locations. Further details on physical properties can be found in Hancock and Vallely (2020).
Environmental tracer distribution
In both 2015 and 2021, cores were collected in a straight line from the surface down the soil profile at each trench. For all sites, 137Cs was present in the surface 0–20 cm layer and generally highest at the surface and decreased in concentration or was absent as soil depth increased (Figs 4–6). 137Cs concentration is displayed at each sampled soil depth. It should be noted that the absence of a bar at a particular depth indicates that 137Cs was not detected at that depth in the soil profile.
137Cs depth distribution pattern in 2015 and 2021 for the Upper location (top of hillslope). (a) Inside (inside the ecological services area), and (b) Outside (cattle grazing) the ecological services area. The absence of a bar at depth indicates that no 137Cs was detected at that soil depth.
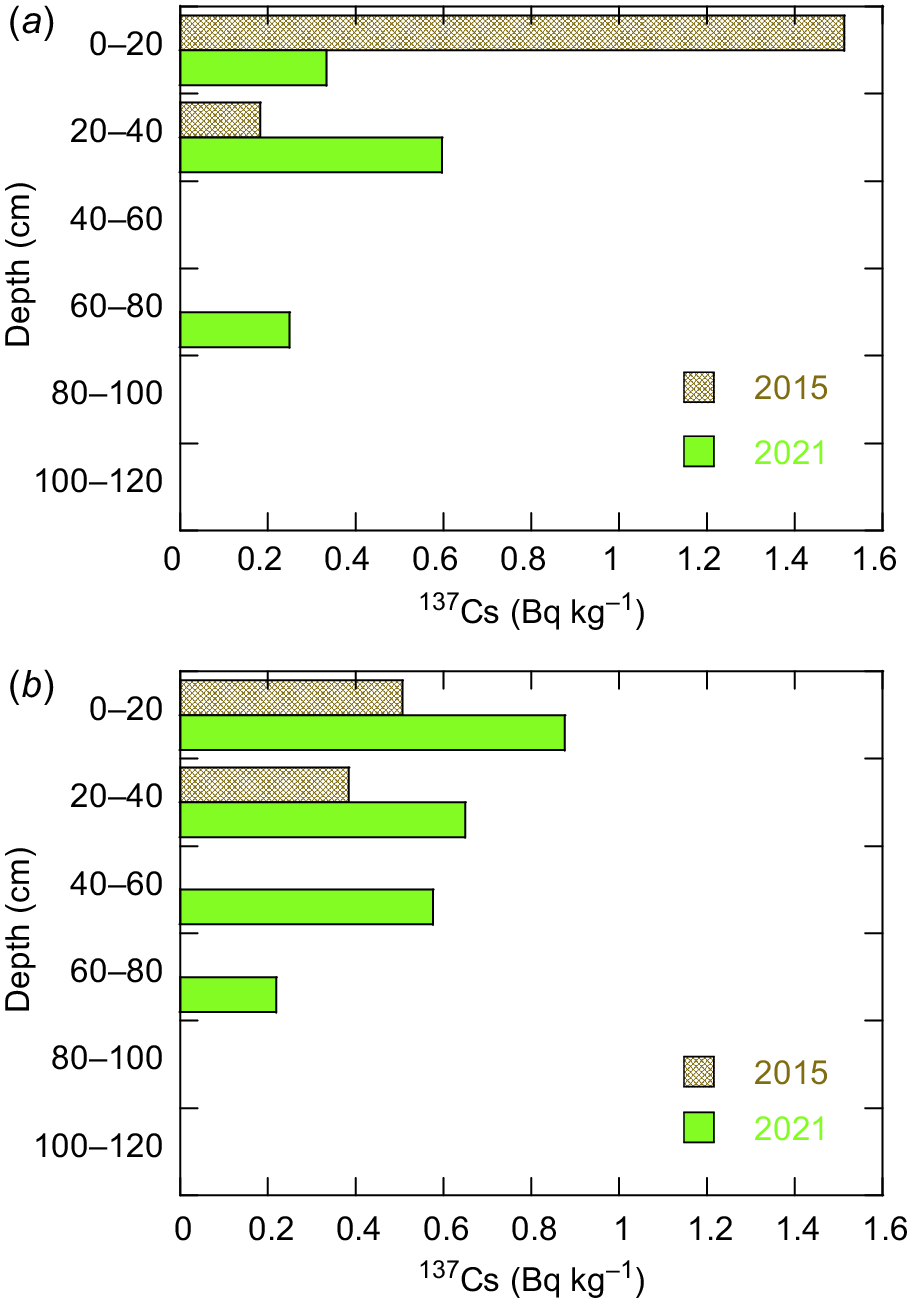
137Cs depth distribution pattern in 2015 and 2021 for the Middle location (mid point of hillslope). (a) Inside (inside the ecological services area), and (b) Outside (cattle grazing) the ecological services area. The absence of a bar at depth indicates that no 137Cs was detected at that soil depth.
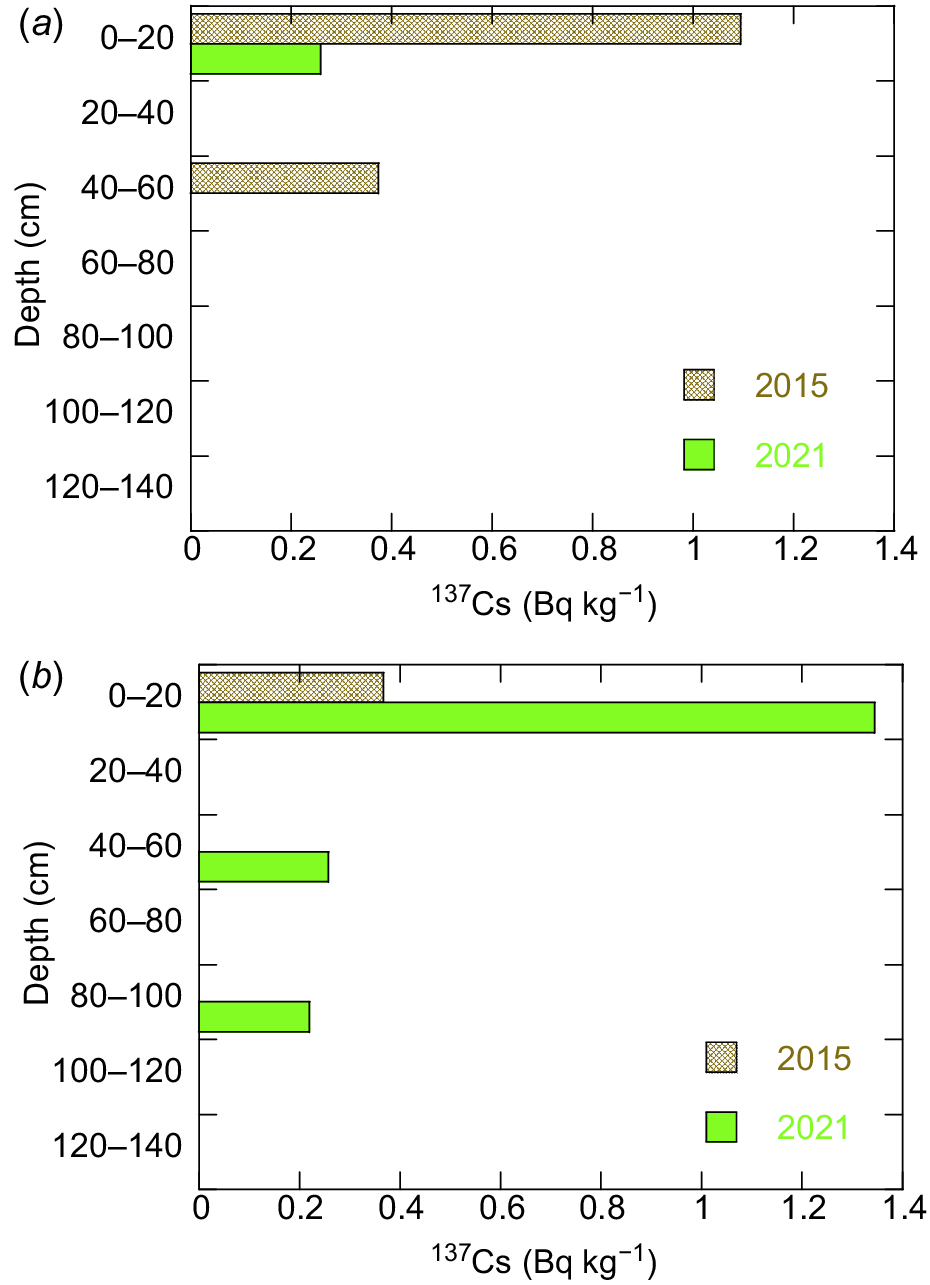
137Cs depth distribution pattern in 2015 and 2021 for the Lower or bottom location (base of hillslope). (a) Inside (inside the ecological services area), (b) Outside (cattle grazing) the ecological services area. The absence of a bar at depth indicates that no 137Cs was detected at that soil depth.
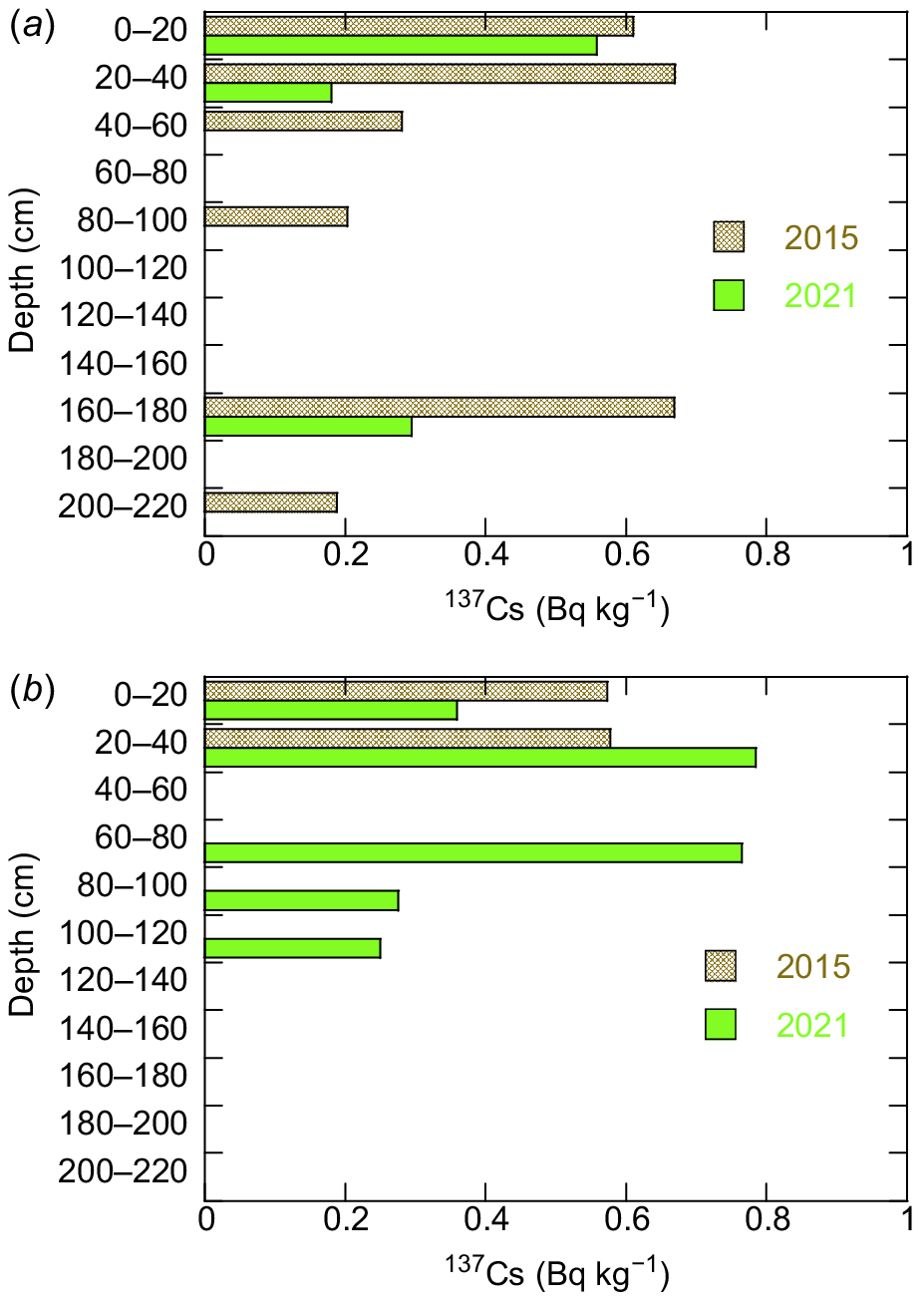
For the Upper or top hillslope location in 2015, 137Cs concentration was highest at the surface with both the Inside and Outside locations having 137Cs present to 20–40 cm (Fig. 4). In 2021, the distribution of 137Cs was discontinuous (absent at 40–60 cm) for the Inside site while it was continuous for the Outside site. However, 137Cs was found to 60–80 cm in the profile at both Inside and Outside locations. This suggests that there has been a delivery of labelled sediment to depth from 2015 to 2021.
For the Middle Inside hillslope position, 137Cs was present to 40–60 cm for the Inside location in 2015 (Fig. 5). For the Outside site, 137Cs was only present in the 0–20 cm layer in 2015. For the 2021 sampling, 137Cs was discontinuous at both locations and only present in the 0–20 cm depth for the Inside location while for the 2021, 137Cs was found to 80–100 cm for the Outside location.
For the Lower or bottom Inside site in 2015 (Fig. 6), 137Cs was highest at 20–40 cm with a very similar concentration to the surface found at 160–180 cm. There was a discontinuous pattern of 137Cs down the profile with labelled material present at 200–220 cm. For the Lower Outside site there was approximately equal concentration of 137Cs in the 0–20 cm and 20–40 cm layers with no 137Cs found below 20–40 cm. For the Inside site in 2021, 137Cs concentration was highest at the surface and present at 20–40 cm with no 137Cs detected until 160–180 cm. In 2021, the highest concentration was found in the 20–40 cm layer, with no 137Cs at 40–60 cm and 137Cs present at 60–80 cm with concentration approximating that of the 20–40 cm layer. 137Cs was present to 120 cm.
Discussion
Soil formation is a combination of eroded and deposited materials from upslope as well as material formed in situ. The profile will also have materials move down the soil profile. The process occurs as a function of wetting and drying and the movement of dissolved materials as well as the illuviation of the fine fraction of soil (Paton et al. 1995; Willgoose 2018). This surface contribution is particularly relevant in regards to pedogenic processes as well as the movement of fertilisers, pesticides, herbicides as well as SOC. The rate and pattern of soil component movement down the profile will be location specific based on site geology, soil mineralogy, climate, vegetation and land use. The findings here suggest that soil materials can move relatively rapidly down the soil profile which suggests the potential for movement of SOC to depth (Hancock 2023a).
Soil properties and soil migration
137Cs has been mostly used to determine soil erosion rates and deposition patterns. The use of 137Cs is well accepted for this purpose with a number of conversion models available to calculate erosion rates for different land use and applications (Zapata et al. 2002). 137Cs has been less well used as an environmental tracer to understand and quantify the movement of soil down the profile. 137Cs has the advantage that it is an anthropogenic tracer with no anthropogenic input to the Australian landscape since 1972. A further advantage is that it well-recognised that it strongly binds to the clay component at the soil surface.
For untilled non-cracking soils, the 137Cs depth distribution profile is that of an exponential decline within the top approximately 20 cm with little 137Cs below this (Loughran 1994; Walling and He 1999, 2001; Walling et al. 2002; Loughran et al. 2002). There is considerable variability in the shape of this exponential decline; however, the general pattern is consistent for all sites globally as well as sites in south-east Australia (Martinez et al. 2010; Hancock et al. 2015; Hancock 2023b). The authors and colleagues have demonstrated this to be the case for several sites within the local study area (Martinez et al. 2009; Wells et al. 2012, 2013; Wells and Hancock 2014; Hancock et al. 2015).
Therefore, any labelled material at depth has translocated via diffusive or advective processes such as:
Near surface 137Cs labelled soil falling to depth down soil cracks in times of dry periods;
Eroded surface material being captured by a crack; or
Animals translocating surface soil to depth.
At this site, it is found that 137Cs migrates to depths far greater than would have occurred by diffusive processes (Wells et al. 2012; Wells and Hancock 2014). Therefore, more direct (advective) delivery is the transfer process.
For the Upper or top of hillslope site, 137Cs is present to a depth of 60–80 cm for both Inside and Outside locations. This suggests that: (1) there is no difference between the movement of surface labelled soil down the soil profile either Inside or Outside the shelterbelt; and (2) migration of soil is not affected by cattle grazing and the shelterbelt has no impact on the translocation of surface labelled material. Further, for the location there appears to be more 137Cs at depth than in 2021 compared to 2015. This suggests a delivery of labelled sediment from 2015 to 2021, likely a result from soil cracking during the 2018–2020 drought.
The 137Cs depth concentration patterns for the Middle and Lower locations were not so consistent with the Middle Inside location having more 137Cs at depth in 2015 than 2021. While the Outside location has considerably more 137Cs at depth in 2021. A similar result was found for the Lower site at the base of the hillslope.
The pattern of 137Cs concentration was inconsistent down the profile for all trenches both Inside and Outside the shelterbelt. Each location had a unique depth distribution. As opposed to untilled sites where soil has been sampled at the near surface (i.e. 0–20 cm) (Martinez et al. 2009), there were no down-profile patterns observed. Including error calculations in each 137Cs concentration did not develop and observable patterns. There was no trench where 137Cs was vertically consistently present down the profile except for the Outside 2021 location. This suggests that 137Cs labelled soil is moving down the profile via cracks which were not visible at the time of sampling.
The lead author has been working at the study site for over 20 years and soil cracks have been observed on many occasions. Cracks are not linear features that open in a regular and consistent manner, with any material delivered down the crack being deliver via a non-linear tortuous pathway (crack references). The increased 137Cs at depth over relatively short time (2015–2021) can only have occurred by advective processes (i.e. soil cracking). Labelled material, originally from the surface falls away from the sides of the cracks and under gravity moves down the profile. While cracking at the site is not monitored by the authors, the most severe drought on record occurred between 2017 and 2020 (Fig. 2) with the lowest recorded rainfall in the areas’ history in 2019. It is very likely that large cracks occurred during this time. It is suggested here that the inconsistent 137Cs pattern down the profile is a result of surface labelled material being delivered along the crack paths with this material being delivered deep down the soil profile.
Rate of soil movement
As an anthropogenic product and a relatively new addition to the environment, 137Cs allows an approximate age of the labelled soil material to be determined (Hancock 2023a, 2023b). For the Upper or top hillslope site, the maximum depth of 137Cs labelled soil is 80 cm and that it can be assumed that 137Cs arrived on the soil surface between 1945 and 1972. This suggests that an age range of between 76 years (2021 subtract 1945) and 48 years (2021 subtract 1972) of material using 2021 as the date of sampling. Therefore, at the earliest, material has migrated to its maximum depth 76 years prior with material assumed to be still migrating. Alternatively, if down profile transport commenced at the end of the fallout period in 1972 then the labelled soil material has a maximum age of approximately 49 years.
The age of 76 years is a maximum age as there was no fallout prior to 1945. It is more likely that the labelled material is much younger than these ages and transported in less time. Soil would be continually migrating down the soil profile. The finding that the migration of 137Cs was only present to 20–40 cm in 2015 and then in 2021 was found to a depth of 60–80 cm suggests that soil can migrate over relatively short time periods.
The data for Upper site suggests the material has translocated to depth from 2015 to 2021 both Inside and Outside the exclusion area in a relatively short time (i.e. 6 years). The findings for the Middle and Lower sites are mixed. However, there appears to be more labelled material at depth for the 2021 Middle and Lower Outside sites, again suggesting a rapid transport between 2015 and 2021.
A general trend is that there is more 137Cs at greater depth for the 2021 Outside location compared to 2015. This suggests a rapid translocation of material from 2015 to 2021. There are no significant differences or patterns in soil properties to explain this difference other than to suggest that soils Outside of the exclusion area developed more cracks than Inside the exclusion area.
The influence of cattle exclusion on soil movement
There have been several studies investigating how differences in land management particularly cropping and vegetation can influence soil properties at depth (Pringle et al. 2011; Murphy 2015; Hobley et al. 2016; Conrad et al. 2017). Dalal et al. (2021) found that the introduction of pasture phase in the cropping cycle may reduce SOC loss while Hulugalle et al. (2012) found similar results for irrigated cotton in Vertosol soils which suggests the migration of soil components down the soil profile.
A question arising from this work is: do fenced-off areas change or alter the migration of soil components particularly when cattle have been excluded for 20 years? Interestingly, there is little difference in the migration of 137Cs down the profile Inside or Outside the shelterbelt at the Upper site. At both Inside and Outside sites, 137Cs was present at 60–80 cm. There also appears to be further migration of 137Cs down the profile in 2021 compared to 2015. This suggests that there may have been enhanced down profile movement of material during the drought as a result of cracking (as described above). For the Middle and Lower sites, the data is inconsistent. For the Middle location 137Cs is present to 40–60 cm in 2015 and only at the surface 0–20 cm layer. While the Middle Outside site has 137Cs present in the 0–20 cm layer in 2015 and to 80–100 cm in 2021. A similar inconsistent pattern was found for the Lower site.
This suggests that either: (1) cattle grazing has no impact on the down profile movement of soil material; or (2) 20 years is insufficient time to observe any differences between grazing area and cattle exclusion. Given the data represents 20 years of cattle exclusion, which is a reasonable time period to be examining potential change in soil processes and properties, it can be argued that well-managed cattle grazing may be considered to have little impact on down profile movement of soil components and pedogenic processes.
Overall, there does not appear to be any strong differences between Inside and Outside the exclusion area.
Study limitations and further work
Soil materials can be delivered to depth from both in situ and ex situ materials (Murphy 2015). The soils here could be considered relatively deep for the Australian agricultural environment and have shrink-swell properties with cracking potential. As soil dries, cracks grow and allow surface soil materials (i.e. soil material labelled with 137Cs) to exfoliate and fall into the cracks (Wilding and Tessier 1988; Wells et al. 2012, 2013; Hancock 2023a). This is then incorporated into the soil profile (Yaalon and Kalmar 1978; Willgoose 2018). The 137Cs activity reported here, particularly at the Lower or bottom site, is at depths greater than that found elsewhere by others. Southard and Graham (1992) reported 137Cs present at depths up to 72 cm while Al-Masri (2006) reported that 137Cs distribution to be related to soil type and movement of material to depth. Curtis (1996) in New South Wales, Australia in an area north of this study suggested that surface cracks enable soil fines labelled with 137Cs to move down the soil profile. Martinez et al. (2010) found 137Cs at depth in the Stanley catchment examined here. Hancock (2023a) also found that 137Cs was present at depths of up to 80 cm at sites in the local study area.
Methods for soil delivery to depth include the burrowing of animals such as wombats and rabbits where soil migrates and or transported along animal burrows (Butler 1995, 2018; Paton et al. 1995; Willgoose 2018). Wombats are present in the area and have burrows in the nearby river banks and hillslopes. They have never been observed in the study area and would have difficulty to coexist with cattle. Rabbits are present in the area but in small numbers and no warrens have been observed. There is the potential for tree roots and their decay may have provide a pathway for soil materials to depth. However, all trees were cleared over 100 years previous predating the arrival of 137Cs. The trees that exist now have been planted in 2002.
Interestingly, the depth to which the 137Cs labelled soil was not consistent and likely reflects the variability in crack depth, the non-vertical nature of the cracks whereas the cores were collected vertically. A further consideration is the orientation of the cracks to capture surface material via erosional processes. The findings reported here could have occurred by chance in that the vertical sampling may have missed collecting labelled material from cracking that was non-vertical or that there are different vertical transport processes at different positions on the hillslope. Further work will examine the size and orientation of cracks.
There is a considerable body of work examining the movement of water, solutes and gases down the soil profile (Jha et al. 2020). There appears to be considerably less research examining the movement of solid soil components. An issue with the physical movement of soil components is that it is often destructive as a sample is required to be collected, processed and analysed in a laboratory. If whole profile assessment is of interest, then a trench needs to be exhumed or a core inserted to the depth required. Both core and trench sampling methods are labour intensive. This will continue to hinder data collection without new and or more rapid measurement methods developed (i.e. proximal sensors, digital soil mapping combined with pedotransfer functions).
Weathering and pedogenesis will also be occurring with new soil being produced at each point on the hillslope as a result of these processes. Soil production is conceptually well-understood but a full understanding of the process is yet to be developed due to the complexity and long time scales of the process. A number of empirical soil production models have been developed (Heimsath et al. 2009). Numerical models have also been developed (Wells et al. 2012, 2013; Welivitiya et al. 2019) but these lack the field and laboratory data to be fully parameterised. Therefore, there is considerable work still be done to understand hillslope scale surface and subsurface evolution. This work adds to the field-based data which can be used in pedogenesis models that include advective and diffusive processes (Wells et al. 2012, 2013; Wells and Hancock 2014).
The presence of 137Cs allows age constraints to be placed on the movement of soil material down the profile as it was absent from the environment prior to 1945. The findings demonstrate a rapid migration of soil material from the surface down the soil profile. While 137Cs was not detected at bedrock for all trenches, this could have been simply by chance that the sampling missed the crack where labelled soil was present. Further sampling is required to better constrain the field data.
It is recognised that the 137Cs concentrations reported here are low. However, the concentrations are similar to that of other local sites with similar soils reported in Hancock (2023a, 2023b). The low concentrations at Stanley suggests that erosion has occurred. A hilltop control site established in 2007 reported considerably higher concentrations than those reported here (Martinez et al. 2010). A loss of 137Cs (and soil) is a logical finding as the shelterbelt area and surrounds were heavily cropped until the present owners in the late 1990s moved to beef cattle grazing and improved soil management (and installation of shelterbelts throughout the property). In addition, a hillslope transect was sampled on the outside of the shelterbelt in 2007 (eastern side) using the same cores and method as described in this paper. Eighteen 20-cm deep cores collected at regular spacings down the hillslope were analysed for 137Cs. The average concentration for these cores was 0.59 Bq kg−1 (s.d. = 0.27 Bq kg−1), which supports the 137Cs concentrations reported here.
The project was conducted on a hillslope with soils having a high clay content (Vertosol soils). Other sites and soils in the study area with less clay (and not derived from basalt soils) have been examined and 137Cs has been at the surface (0–20 cm) only (Hancock 2023a, 2023b). Other tracers with short half-lives such as 7Be (He et al. 2002) would provide insights to the migration of soil components. 7Be could be used from soil samples when cracks are present and then post-crack closure (Zapata et al. 2002; Walling et al. 2011). New methods using 239 + 240Pu (239Pu half-life 24,110 years; 240Pu half-life, 6561 years) are also being developed that have the potential to refine understanding of both spatial and temporal movement of soil (Arata et al. 2016). Further, 137Cs with a half-life of 30.1 years has now undergone radioactive decay of two to three half-lives in the Australian environment therefore its usefulness is reducing rapidly with time and the above new methods need to be explored.
Conclusion
137Cs is a relatively recent and unique component of the soil landscape system. It is well recognised that 137Cs binds to the clay content of soil. The finding that this anthropogenic chemical is present at depth in the soil profile demonstrates that surface soil components can rapidly migrate to depth. At the Lower or bottom of the hillslope examined here, labelled soil is present at bedrock depth of 220 cm.
The work demonstrates that surface material can reach considerable depths in a relatively short time. This suggests that soil components such as organic carbon may be translocated to depths relatively rapidly. This augers well for carbon sequestration and the movement of soil carbon to the deep soil profile for long-term storage. A further implication is that other soil components and chemicals (i.e. agrochemicals and fertilisers) may be migrating along the same pathways. This may be problematic for agrochemicals that have a long half-life.
Declaration of funding
This research was largely supported by Australian Research Council Discovery Grants (DP 0556941: ‘Carbon, nutrient and sediment dynamics in a semi-arid catchment’ and DP110101216: ‘A next generation spatially distributed model for soil profile dynamics and pedogenesis incorporating soil geochemistry and organic matter’).
References
Al-Masri MS (2006) Vertical distribution and inventories of 137Cs in the Syrian soils of the eastern Mediterranean region. Journal of Environmental Radioactivity 86, 187-198.
| Crossref | Google Scholar |
Arata L, Alewell C, Frenkel E, A’Campo-Neuen A, Iurian A-R, Ketterer ME, Mabit L, Meusburger K (2016) Modelling deposition and erosion rates with RadioNuclides (MODERN) – Part 2: a comparison of different models to convert 239+240Pu inventories into soil redistribution rates at unploughed sites. Journal of Environmental Radioactivity 162–163, 97-106.
| Crossref | Google Scholar |
Butler DR (2018) Zoogeomorphology in the Anthropocene. Geomorphology 303, 146-154.
| Crossref | Google Scholar |
Conrad KA, Dalal RC, Dalzell SA, Allen DE, Menzies NW (2017) The sequestration and turnover of soil organic carbon in subtropical leucaena-grass pastures. Agriculture, Ecosystems & Environment 248, 38-47.
| Crossref | Google Scholar |
Dalal RC, Thornton CM, Allen DE, Owens JS, Kopittke PM (2021) Long-term land use change in Australia from native forest decreases all fractions of soil organic carbon, including resistant organic carbon, for cropping but not sown pasture. Agriculture, Ecosystems & Environment 311, 107326.
| Crossref | Google Scholar |
Dalzell BJ, Fissore C, Nater EA (2022) Topography and land use impact erosion and soil organic carbon burial over decadal timescales. Catena 218, 106578.
| Crossref | Google Scholar |
De Alba S (2001) Modeling the effects of complex topography and patterns of tillage on soil translocation by tillage with mouldboard plough. Journal of Soil and Water Conservation 56, 335-345.
| Google Scholar |
De Alba S (2003) Simulating long-term soil redistribution generated by different patterns of mouldboard ploughing in landscapes of complex topography. Soil and Tillage Research 71, 71-86.
| Crossref | Google Scholar |
De Alba S, Lindstrom M, Schumacher TE, Malo DD (2004) Soil landscape evolution due to soil redistribution by tillage: a new conceptual model of soil catena evolution in agricultural landscapes. Catena 58, 77-100.
| Crossref | Google Scholar |
Gaspar L, Navas A (2013) Vertical and lateral distributions of 137Cs in cultivated and uncultivated soils on Mediterranean hillslopes. Geoderma 207–208, 131-143.
| Crossref | Google Scholar |
Gibson AJ, Hancock GR, Healy E, Lindsay L, Moore K, Bretreger D, Cox T, Yeo I (2022) Limitations to the soil impacts of tree regrowth in improved cattle pasture. Agriculture, Ecosystems & Environment 337, 108015.
| Crossref | Google Scholar |
Hancock GR (2023a) Understanding and quantifying whole soil-profile organic carbon transfer using an environmental tracer. Soil Research 61, 775-786.
| Crossref | Google Scholar |
Hancock GR (2023b) Using environmental tracers to understand soil organic carbon and soil erosion on a steep slope hillslope in south-east Australia. Soil Research 61, 616-625.
| Crossref | Google Scholar |
Hancock GR, Coulthard TJ (2012) Channel movement and erosion response to rainfall variability in southeast Australia. Hydrological Processes 26, 663-673.
| Crossref | Google Scholar |
Hancock G, Lowry J (2021) Quantifying the influence of rainfall, vegetation and animals on soil erosion and hillslope connectivity in the monsoonal tropics of northern Australia. Earth Surface Processes and Landforms 46, 2110-2123.
| Crossref | Google Scholar |
Hancock GR, Vallely M (2020) Effects of grazing exclusion on soil organic carbon: hillslope and soil profile results (an Australian example). Science of The Total Environment 705, 135844.
| Crossref | Google Scholar |
Hancock GR, Wells T, Martinez C, Dever C (2015) Soil erosion and tolerable soil loss: insights into erosion rates for a well-managed grassland catchment. Geoderma 237–238, 256-265.
| Crossref | Google Scholar |
Hancock GR, Kunkel V, Wells T, Martinez C (2019) Soil organic carbon and soil erosion – understanding change at the large catchment scale. Geoderma 343, 60-71.
| Crossref | Google Scholar |
He Q, Walling DE, Wallbrink PJ (2002) Chapter 9: Alternative methods and radionuclides for use in soil-erosion and sedimentation investigations. In ‘Handbook for the assessment of soil erosion and sedimentation using environmental radionuclides’. (Ed. F Zapata) pp. 185–215. (Kluwer Academic Publishers: Dordrecht, The Netherlands)
Heimsath AM, Fink D, Hancock GR (2009) The ‘humped’ soil production function: eroding Arnhem Land, Australia. Earth Surface Processes and Landforms 34, 1674-1684.
| Crossref | Google Scholar |
Hobley EU, Baldock J, Wilson B (2016) Environmental and human influences on organic carbon fractions down the soil profile. Agriculture, Ecosystems & Environment 223, 152-166.
| Crossref | Google Scholar |
Hulugalle NR, Weaver TB, Finlay LA (2012) Carbon inputs by wheat and vetch roots to an irrigated Vertosol. Soil Research 50, 177-187.
| Crossref | Google Scholar |
Jagercikova M, Evrard O, Balesdent J, Lefèvre I, Cornu S (2014) Modeling the migration of fallout radionuclides to quantify the contemporary transfer of fine particles in Luvisol profiles under different land uses and farming practices. Soil and Tillage Research 140, 82-97.
| Crossref | Google Scholar |
Jha P, Hati KM, Dalal RC, Dang YP, Kopittke PM, Menzies NW (2020) Soil carbon and nitrogen dynamics in a Vertisol following 50 years of no-tillage, crop stubble retention and nitrogen fertilization. Geoderma 358, 113996.
| Crossref | Google Scholar |
Kiem AS, Johnson F, Westra S, van Dijk A, Evans JP, O’Donnell A, et al. (2016) Natural hazards in Australia: droughts. Climatic Change 139, 37-54.
| Crossref | Google Scholar |
Loughran RJ (1994) The use of the environmental isotope caesium-137 for soil erosion and sedimentation studies. Trends in Hydrology 1, 149-167.
| Google Scholar |
Martinez C, Hancock GR, Kalma JD (2009) Comparison of fallout radionuclide (caesium-137) and modelling approaches for the assessment of soil erosion rates for an uncultivated site in south-eastern Australia. Geoderma 151, 128-140.
| Crossref | Google Scholar |
Martinez C, Hancock GR, Kalma JD (2010) Relationships between 137Cs and soil organic carbon (SOC) in cultivated and never-cultivated soils: an Australian example. Geoderma 158, 137-147.
| Crossref | Google Scholar |
Murphy BW (2015) Impact of soil organic matter on soil properties – a review with emphasis on Australian soils. Soil Research 53, 605-635.
| Crossref | Google Scholar |
Pringle MJ, Allen DE, Dalal RC, Payne JE, Mayer DG, O’Reagain P, Marchant BP (2011) Soil carbon stock in the tropical rangelands of Australia: effects of soil type and grazing pressure, and determination of sampling requirement. Geoderma 167–168, 261-273.
| Crossref | Google Scholar |
Ritchie JC, McHenry JR (1975) Fallout Cs-137: a tool in conservation research. Journal of Soil and Water Conservation 30(341), 283-286.
| Google Scholar |
Southard RJ, Graham RC (1992) Cesium-137 distribution in a California pelloxerert: evidence of pedoturbation. Soil Science Society of America Journal 56, 202-207.
| Crossref | Google Scholar |
Van Oost K, Govers G (2006) Tillage erosion. In ‘Soil erosion in Europe’. (Eds J Boardman, J Poesen) pp. 599–608. (John Wiley & Sons, Ltd) doi:10.1002/0470859202.ch43
Van Oost K, Govers G, de Alba S, Quine TA (2006) Tillage erosion: a review of controlling factors and implications for soil quality. Progress in Physical Geography: Earth and Environment 30, 443-466.
| Crossref | Google Scholar |
Walling DE, He Q (1999) Improved models for estimating soil erosion rates from cesium-137 measurements. Journal of Environmental Quality 28(2), 611-622.
| Crossref | Google Scholar |
Walling DE, He Q (2001) Models for converting 137Cs measurements to estimates of soil redistribution rates on cultivated and uncultivated soils, and estimating bomb derived 137Cs reference inventories (including software for model implementation). A contribution to the IAEA Coordinated Research Programmes on Soil Erosion (D1.50.05) and Sedimentation (F3.10.01). University of Exeter. p. 32.
Walling DE, He Q, Appleby PG (2002) Chapter 7: Conversion models for use in soil-erosion, soil redistribution and sedimentation investigations. In ‘Handbook for the assessment of soil erosion and sedimentation using environmental radionuclides’. (Ed. F Zapata) pp. 111–164. (Kluwer Academic Publishers: Dordrecht, The Netherlands)
Walling DE, Zhang Y, He Q (2011) Models for deriving estimates of erosion and deposition rates from fallout radionuclide (caesium-137, excess lead-201, and beryllium-7) measurements and the development of user friendly software for model implementation. Impact of soil conservation measures on erosion control and soil quality. IAEA-TECDOC-1665. pp. 11–33.
Welivitiya WDDP, Willgoose GR, Hancock GR (2019) A coupled soilscape-landform evolution model: model formulation and initial results. Earth Surface Dynamics 7, 591-607.
| Crossref | Google Scholar |
Wells T, Hancock G (2014) Comparison of vertical transport of 137Cs and organic carbon in agricultural cracking soils. Geoderma 214–215, 228-238.
| Crossref | Google Scholar |
Wells T, Hancock GR, Dever C, Murphy D (2012) Prediction of vertical soil organic carbon profiles using soil properties and environmental tracer data at an untilled site. Geoderma 170, 337-346.
| Crossref | Google Scholar |
Wells T, Hancock GR, Dever C, Martinez C (2013) Application of RothPC-1 to soil carbon profiles in cracking soils under minimal till cultivation. Geoderma 207–208, 144-153.
| Crossref | Google Scholar |
Willgoose G (2018) ‘Principles of soilscape and landscape evolution.’ p. 356. (Cambridge University Press: Cambridge, UK) doi:10.1017/9781139029339
Yaalon DH, Kalmar D (1978) Dynamics of cracking and swelling clay soils: displacement of skeletal grains, optimum depth of slickensides, and rate of intra-pedonic turbation. Earth Surface Processes and Landforms 3, 31-42.
| Crossref | Google Scholar |