Australian biogeography, climate-dependent diversification and phylogenomics of the spectacular Chamelaucieae tribe (Myrtaceae)
Francis J. Nge







A
B
C
D
E
F
Handling Editor: Daniel Murphy
Abstract
Chamelaucieae is a diverse tribe in Myrtaceae with ~800 species in 37 genera distributed across Australia. We applied target capture sequencing using the Angiosperms353 probe set for 131 taxa as part of the Genomics for Australian Plants initiative. Sampling all genera (36) from 10 of 11 named subtribes, we present a phylogenomic analysis for the tribe. This phylogenomic approach has allowed us to better resolve subtribal relationships across the tribe, resulting in an updated classification and additional subtribe (total of 12 subtribes including Triplarininae). Despite these advances, the phylogenetic placements of Stenostegiinae, Astarteinae, and Micromyrtinae remain equivocal and resolution of these relationships should be a focus of future research. We constructed a dated phylogeny from this genomic dataset to investigate the tribe’s biogeographic history and diversification dynamics. We estimate that the crown radiation occurred in the Eocene (c. 42 Ma), with the ancestral area of origin in Australia unresolved. Subsequent divergence and origin of subtribes mostly occurred in south-west Western Australia with frequent dispersals from there into the semi-arid and arid interior since the Miocene (20 Ma). Dispersals out of northern and eastern Australia were limited and confined to dispersal events into the arid interior. Using paleoenvironmental diversification models we show that after the initial radiation, diversification in Chamelaucieae declined rapidly until the Eocene–Oligocene boundary extinction pulse event and subsequently more slowly to the present, with a modest increase during the Middle Miocene Climatic Optimum. No significant diversification rate shifts were detected within clades except within the subtribe Chamelauciinae. There was no significant geographic-dependent diversification in the tribe. Our results add to the growing literature revealing that high plant diversity in south-west Western Australia is due to more time for species accumulation attributed to long-term climatic stability rather than elevated diversification rates.
Keywords: Angiosperms353, Australian flora, biogeography, Chamelaucieae, dispersal, diversification rates, Eocene–Oligocene, extinction pulse, Myrtaceae, peripheral vicariance, phylogenomics.
Introduction
Molecular phylogenetic studies have provided important insights into the biogeographic history and connections of the Australian flora, including connections with other Gondwanan landmasses (Ladiges et al. 2003; Barker et al. 2007; Ladiges and Cantrill 2007; Milner et al. 2015), floristic exchanges between Australia and Asia (Nauheimer et al. 2012; Thomas et al. 2012; Crayn et al. 2015), New Zealand (Pole 1994; Lockhart et al. 2001; Winkworth et al. 2005; Meudt et al. 2009) and the Pacific (Gemmill et al. 2002; Birch and Keeley 2013; Keppel et al. 2023). Within Australia, studies showed vicariance between the western and eastern temperate regions of the continent (Crisp and Cook 2007; Ladiges et al. 2010), and recent dispersals between biomes (Ladiges et al. 2006; Cardillo et al. 2017; Nauheimer et al. 2018). Many of these studies, however, have used few (often <10) genetic markers and lack robust support.
Increasingly, phylogenomic methods are being used to address these and other evolutionary questions with unprecedented amounts of genomic data. Target enrichment high-throughput sequencing (HTS) genomic approaches are particularly promising and have been applied to many plant groups including in Australia (e.g. Cardillo et al. 2017; Nge et al. 2021a, 2024a; Schmidt-Lebuhn and Bovill 2021; Crisp et al. 2024). The Genomics for Australian Plants (GAP) initiative has stimulated these efforts to gather and sequence genomic data in a wide range of Australian plant families (Simpson et al. 2024, in press). More comprehensive investigations of the evolutionary history, biogeography and diversification dynamics of the Australian flora is thus now made possible through this initiative.
The tribe Chamelaucieae in Myrtaceae is a diverse component of the Australian flora (Fig. 1), with over 660 formally described species reported by Rye et al. (2020), 36 additional species published since that time (Davis and Rye 2020; Rye 2020, 2021, 2022, 2024; Rye and Barrett 2020; Nge and Thiele 2022; Rye et al. 2022; Thiele et al. 2022; Keighery et al. 2023) and numerous unnamed species awaiting publication. Representatives of the tribe (as the Chamelaucium Desf. alliance sensu Briggs and Johnson 1979) were included in early molecular phylogenetic studies of Myrtaceae (Gadek et al. 1996; Wilson et al. 2001). More focused studies on the alliance by Lam et al. (2002) and Rye et al. (2020) utilised Sanger-sequenced nuclear and plastid markers. These, however, failed to resolve a backbone for the tribe, resulting in uncertain phylogenetic relationships between subtribes sensu Rye et al. (2020). Conversely, Nge et al. (2022) retrieved a well-resolved backbone for Calytrix Labill. (subtribe Calytricinae) by using a HTS approach based on an angiosperm-wide custom bait kit (OzBaits; Waycott et al. 2021) to sequence 100 single-copy nuclear markers. Phylogenomic studies using genomic methods hold promise to resolve the backbone topology for tribe Chamelaucieae as a whole.
Representative floral diversity in Chamelaucieae. (a) Babingtonia grandiflora (Hysterobaeckeinae). (b) Verticordia insignis (Chamelauciinae). (c) Babingtonia pelloeae (Hysterobaeckeinae). (d) Ericomyrtus tenuior (Hysterobaeckeinae). (e) Hypocalymma magnificum (Astarteinae). (f) Verticordia rutilastra (Chamelauciinae). (g) Astartea schaueri (Astarteinae). (h) Cyathostemon blackettii (Astarteinae). (i) Balaustion pulcherrimum (Hysterobaeckeinae). (j) Chamelaucium sp. Koolyanobbing (V. Clarke 644) (Chamelauciinae). (k) Calytrix leschenaultii (Calytricinae). (l) Pileanthus filifolius (Chamelauciinae). Photographs by K. R. Thiele (a–j, l) and F. J. Nge (k).
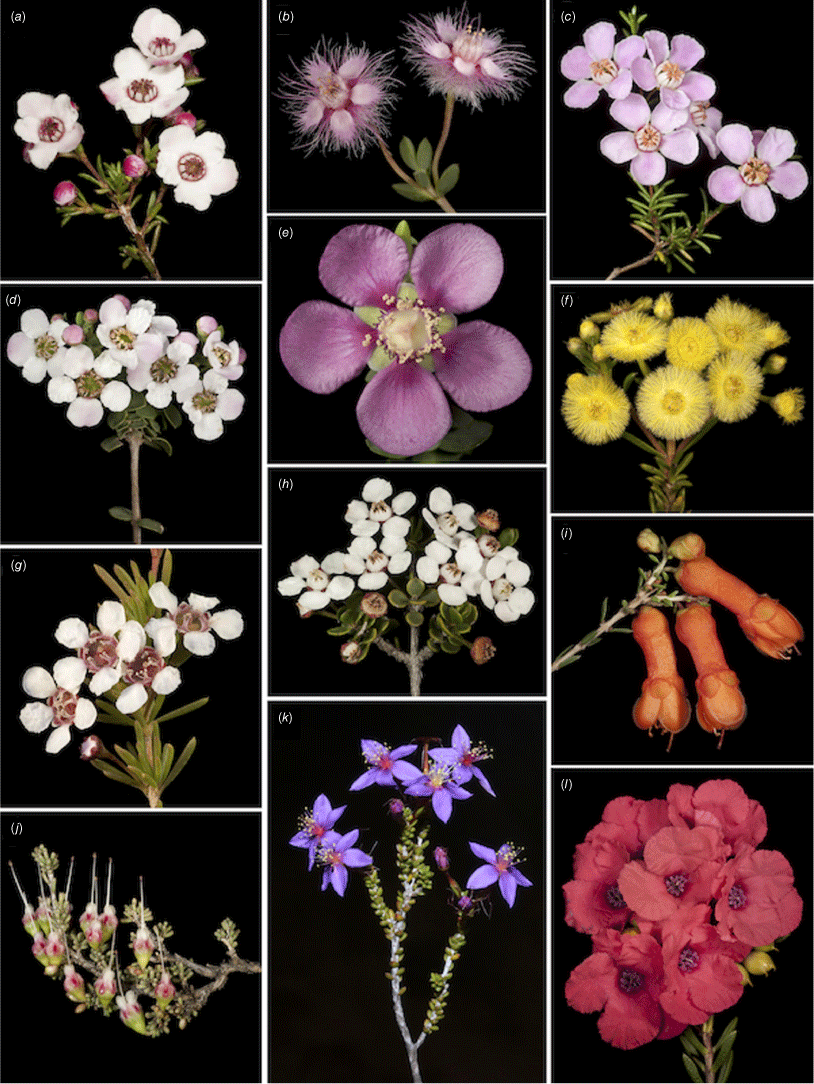
Chamelaucieae is a good candidate clade for improving our understanding of the biogeographic history of the Australian flora. The tribe has a long evolutionary history in the region, with a stem divergence from sister tribe Leptospermeae at c. 51+ Ma and a crown radiation at c. 45 Ma (Thornhill et al. 2015). Most extant species occur in the mesic fringes of the continent and the tribe provides a good test for the peripheral vicariance hypothesis (PVH; Nge et al. 2022) based on an analysis of Calytrix. The PVH posits that increased seasonality and aridification of the Australian continent since the Oligocene have resulted in a peripheral retreat of a once more widespread mesic and aseasonal biota, similar to the biogeography of the African Rand flora (Mairal et al. 2015; Pokorny et al. 2015). The PVH has been supported by biogeographic studies of other Australian plant groups such as Cryptandra Sm. and Pomaderris Labill. (Nge et al. 2021b, 2023), and is suggested from the floristic distributions of other groups (Hammer et al. 2022).
Chamelaucieae also exemplifies another common pattern in the temperate Australian flora: the tribe has very high species richness in south-west Western Australia. Many plant groups in Australia have long been known to exhibit their highest species richness in this area (Hooker 1859; Diels 1906; Burbidge 1960; González-Orozco et al. 2011, 2014; Prentice et al. 2017). Possible explanations for this pattern include lower extinction rates and a steady accumulation of species through time in the south-west (Hopper 2009; Cardillo and Pratt 2013; Cook et al. 2015; Cowling et al. 2015). Nge et al. (2020) inferred that extinction rates were lower in south-west Western Australia than in south-eastern Australia during the Eocene–Oligocene boundary (E-O boundary), whereas Sniderman et al. (2013) found a similar disparity in the Pleistocene. Major global climatic changes occurred during these time periods. The separation of the Drake Passage and Tasmania from Antarctica in the E-O boundary resulted in a sharp global temperature drop of 3°C (Hutchinson et al. 2020; Lauretano et al. 2021), causing regional extinctions and global turnover of biota (Sun et al. 2014; Korasidis et al. 2019). Similarly, Pleistocene glacial cycles (Dröllner et al. 2023; Herbert 2023) resulted in large-scale biotic turnover in many parts of the globe (Rossetto et al. 2007; Byrne 2008; Worth et al. 2009; Rossetto 2015). Interestingly, Nge et al. (2020) found that Chamelaucieae is the only clade across 20 plant families sampled that showed a significant increase in diversification immediately after the E-O boundary. However, the result was based on limited sampling at the generic level (fewer than half of the genera were sampled). More comprehensive sampling within the tribe is required to test this finding. Increases in diversification rates (i.e. rate shifts predominantly from higher speciation rates) have rarely been documented for plants in south-west Western Australia. Instead, most explanations for the high diversity in the region centre on more time being available for a gradual and constant accumulation of species, linked with lower extinction rates than in other regions (Cardillo and Pratt 2013; Cook et al. 2015; Skeels and Cardillo 2019).
A third pattern emerging from previous studies is that south-west Western Australia is a more common source area for dispersal to adjacent arid zone areas than other mesic regions of Australia, across multiple plant groups (Nge et al. 2022, 2023). This bias may be due to the presence of a higher number of lineages and hence more potential colonising lineages in south-west Western Australia than in other regions. Nevertheless, the paucity of dispersals into the arid zone even from species-rich eastern Australian clades such as Pomaderris (Rhamnaceae; Nge et al. 2021b) is puzzling and warrants further investigation.
As part of the GAP initiative, we use a phylogenomic target capture HTS approach to infer evolutionary relationships and resolve the backbone of Chamelaucieae. We use this to investigate the biogeographic history of the tribe and test the following hypotheses:
H1. Biogeographic disjunctions in Chamelaucieae across Australia can be attributed primarily to the Peripheral Vicariance Hypothesis (PVH). A widespread or unresolved ancestral area would provide evidence supporting the PVH.
H2. We expect an asymmetrical dispersal pattern from south-west Western Australia to the arid zone and few dispersal events from other mesic regions to the arid zone, congruent with other plant groups.
H3. Major historical climatic events such as those recorded in the E-O boundary have played a significant role in the evolution and diversification of this tribe.
H4. Few diversification rate shifts (increases) are expected in south-west Western Australian clades in Chamelaucieae, consistent with the expectation that time for species accumulation is the predominant driver of the high plant species richness in the region.
Materials and methods
Taxon sampling
We sampled 131 taxa representing all but one (97%, 36/37) of the genera in Chamelaucieae, and 10 of the 11 subtribes sensu Rye et al. (2020), omitting only Baeckea L. sens. str. in Baeckeinae Schauer (Table 1). The sampling strategy within Chamelaucieae attempted to maximise sampling of generic diversity, utilising available published and unpublished ribosomal and chloroplast phylogenies. Where possible, we attempted to sample at least two species per currently accepted genus, including representatives of the two most divergent clades and a representative of each formally recognised section. Representatives of all putative clades were sampled for putatively polyphyletic and paraphyletic genera (Baeckea sens. lat., Darwinia Rudge, Homoranthus A.Cunn. ex Schauer, Malleostemon J.W.Green, Verticordia DC.). We attempted to sample all morphologically orphaned groups of Baeckea that remain unplaced following the revisions of Rye and co-authors (mostly belonging to subtribe Hysterobaeckineae Rye & Peter G.Wilson; Rye et al. 2020). We therefore aimed to capture a near-complete sampling of Chamelaucieae to approximately sectional level. We also attempted to sample from the geographic extent of each genus to maximise representation for biogeographic signal; however, species-level phylogenies are unavailable for many genera, limiting assessment of whether any phylogenetically or biogeographically important clades were unsampled. We also sampled six outgroup taxa in the tribes Osbornieae (Osbornia F.Muell.), Lindsayomyrteae (Lindsayomyrtus B.Hyland & Steenis), Eucalypteae (Eucalyptus L’Hér.) and the sister tribe Leptospermeae (2× Leptospermum J.R.Forst. & G.Forst. and 1× Pericalymma (Endl.) Endl.; Wilson and Heslewood 2023). We supplemented the dataset with 35 samples from the Maurin et al. (2021) Myrtales dataset generated as part of the Plant and Fungal Trees of Life initiative (PAFTOL; www.paftol.org).
Species | Subtribe | Voucher | Collector number | GAP bioplatform ID | |
---|---|---|---|---|---|
Actinodium cunninghamii Schauer | Chamelauciinae | PERTH 9339078 | A.Webb AW 09118 | 376940 | |
Aluta maisonneuvei (F.Muell.) Rye & Trudgen subsp. maisonneuvei | Alutinae | PERTH 9274251 | S.van Leeuwen et al. PBS 5695 | 376941 | |
Anticoryne melanosperma Rye | Hysterobaeckeinae | PERTH 7782586 | T.Erickson TEE 230 | 376942 | |
Anticoryne ovalifolia (F.Muell.) Rye | Triplarininae | PERTH 8991758 | J.A.Cochrane 8396 | ERR7599928 | |
Astartea aspera Schauer | Astarteinae | UNSWDB11225 | P.G.Wilson UNSW23732 | 376977 | |
Astartea astarteoides (Benth.) Rye | Astarteinae | NSW495906 | P.G.Wilson 1407 | 376975 | |
Astartea fascicularis (Labill.) DC. | Astarteinae | K000065808 | A.Strid 21207 | ERR5034123 | |
Astus tetragonus (Benth.) Trudgen & Rye | Triplarininae | K000065809 | M.E.Trudgen 22485 | ERR5034124 | |
Austrobaeckea uncinella (Benth.) Rye | Hysterobaeckeinae | PERTH 8489432 | M.Hislop 4192 | 376974 | |
Austrobaeckea verrucosa (Turcz.) Rye | Hysterobaeckeinae | NSW612780 | P.G.Wilson 1638 | 376896 | |
Babingtonia camphorosmae (Endl.) Lindl. | Hysterobaeckeinae | K000065811 | G.J.Keighery; B.J.Keighery 335 | ERR5034126 | |
Babingtonia fascifolia Rye | Hysterobaeckeinae | PERTH 9111131 | A.Chant 2057 | 376943 | |
Baeckea elderiana E.Pritz. | Hysterobaeckeinae | PERTH 8935343 | G.Byrne 5990 | 376944 | |
Baeckea muricata C.A.Gardner | Hysterobaeckeinae | PERTH 8997012 | R.Meissner; B.Bayliss 5673 | 376945 | |
Baeckea pentagonantha F.Muell. | Hysterobaeckeinae | PERTH 8837260 | B.L.Rye 290163 | 376946 | |
Baeckea robusta F.Muell. | Hysterobaeckeinae | PERTH 8224188 | M.E.Trudgen MET 22148 | 376947 | |
Baeckea sp. Dudawa (M.E.Trudgen MET 5369) | Hysterobaeckeinae | PERTH 8906343 | K.R.Thiele 5190 | 376948 | |
Baeckea sp. London Bridge (M.E.Trudgen 5393) | Hysterobaeckeinae | PERTH 8561362 | W.A.Thompson; N.B.Sheehy 405 | 376949 | |
Baeckea sp. Murchison River (M.E.Trudgen 12009) | Hysterobaeckeinae | PERTH 6989888 | G.Cassis; M.Wall; C.Symonds; C.Weirauch 9–79 | 376950 | |
Baeckea sp. Stirling Range (H.Steedman s.n. 03/1933) | Hysterobaeckeinae | K000065838 | C.A.Gardner; H.Steedman s.n. | ERR5034889 | |
Baeckea sp. Walkaway (A.S.George 11249) | Hysterobaeckeinae | PERTH 8341524 | V.Westcott 4 A | 376951 | |
Baeckea sp. Walyahmoning (M.E.Trudgen 5412) | Hysterobaeckeinae | PERTH 8215103 | M.E.Trudgen MET 23344 A | 376952 | |
Baeckea staminosa E.Pritz. | Hysterobaeckeinae | PERTH 8208875 | M.E.Trudgen MET 22010 | 376953 | |
Baeckea subcuneata F.Muell. | Hysterobaeckeinae | PERTH 8497095 | G.Woodman; K.Kershaw K Opp 24 | 376954 | |
Balaustion pulcherrimum Hook. | Hysterobaeckeinae | K000065813 | J.Coleby-Williams 232 | ERR5034127 | |
Calytrix exstipulata DC. | Calytricinae | MBA 2728.1 | I.D.Fox 1078 | 376913 | |
Calytrix leschenaultii (Schauer) Benth. | Calytricinae | K000696972 | M.J.M.Christenhusz 7029 | ERR5034778 | |
Chamelaucium ciliatum Desf. | Chamelauciinae | PERTH 9098909 | G.Byrne 6946 | 376927 | |
Chamelaucium uncinatum Schauer | Chamelauciinae | K000065816 | B.L.Rye; M.E.Trudgen 250703 | ERR5034129 | |
Cheyniana microphylla (C.A.Gardner) Rye | Hysterobaeckeinae | K000065817 | L.S.J.Sweedman 8070 | ERR5034880 | |
Cheyniana rhodella Rye & Trudgen | Hysterobaeckeinae | PERTH 8312419 | J.Brooker BB 42 | 376955 | |
Cyathostemon ambiguus (F.Muell.) Rye & Trudgen | Astarteinae | K000065819 | L.Sweedman; M.Baldwin 5703 | ERR5034882 | |
Cyathostemon gracilis Trudgen & Rye | Astarteinae | NSW608059 | P.G.Wilson 1644 | 376897 | |
Darwinia diosmoides (DC.) Benth. | Chamelauciinae | PERTH 9171177 | E.Massenbauer 763 | 376930 | |
Darwinia fascicularis Rudge subsp. fascicularis | Chamelauciinae | NSW909627 | J.M.Cohen 8 | 376918 | |
Darwinia meeboldii C.A.Gardner | Chamelauciinae | QRS 124248.1 | B.Hyland 16600 | 376908 | |
Darwinia micropetala (F.Muell.) Benth. | Chamelauciinae | NSW197012 | A.N.Rodd 5891 | 376919 | |
Darwinia speciosa (Meisn.) Benth. | Chamelauciinae | K000696937 | M.J.M.Christenhusz 6994 | ERR5034776 | |
Enekbatus cryptandroides (F.Muell.) Trudgen & Rye | Rinziinae | K000065820 | M.M.Cole 49 | ERR5034883 | |
Enekbatus sessilis Trudgen & Rye | Rinziinae | PERTH 6764274 | B.L.Rye; M.E.Trudgen BLR 239026 | 376958 | |
Ericomyrtus serpyllifolia (Turcz.) Rye | Hysterobaeckeinae | K000696961 | M.J.M.Christenhusz 7018 | ERR5034777 | |
Ericomyrtus serpyllifolia (Turcz.) Rye | Hysterobaeckeinae | K000065821 | B.L.Rye; M.E.Trudgen 241056 | ERR5034884 | |
Eucalyptus microcorys F.Muell. | Outgroup | NSW333995 | R.Coveny; P.Hind 7764 | ERR5101695 | |
Euryomyrtus denticulata (Maiden & Betche) Trudgen | Rinziinae | NSW879990 | J.Miles 1301 | 376898 | |
Euryomyrtus maidenii (Ewart & Jean White) Trudgen | Rinziinae | K000065823 | R.J.Cranfield 1513 | ERR5034130 | |
Euryomyrtus recurva Trudgen | Rinziinae | PERTH 8633134 | R.Davis; A.Brown RD 12427 | 376956 | |
Harmogia densifolia (Sm.) Schauer | Hysterobaeckeinae | NSW1003373 | S.L.Jalaluddin 61 | 376899 | |
Homalocalyx aureus (C.A.Gardner) Craven | Calytricinae | PERTH 8928185 | K.R.Thiele 5111 | 376957 | |
Homalocalyx polyandrus (F.Muell.) Benth. | Calytricinae | NSW492985 | P.G.Wilson 1544 | 376982 | |
Homalocalyx thryptomenoides (F.Muell.) Craven | Calytricinae | NSW608516 | P.G.Wilson 1614 | 376989 | |
Homoranthus darwinioides (Maiden & Betche) Cheel | Chamelauciinae | QRS 11141.1 | K.C.Ingram s.n. | 376912 | |
Homoranthus homoranthoides (F.Muell.) Craven & S.R.Jones | Chamelauciinae | NSW448059 | P.C.Jobson 5762; K.M.Downs | 376920 | |
Homoranthus thomasii (F.Muell.) Craven & S.R.Jones | Chamelauciinae | K000065825 | L.M.Copeland; K.Hastenteufel 2921 | ERR5034885 | |
Homoranthus tropicus Byrnes | Chamelauciinae | CNS 140415.1 | A.Ford 6044 | 376917 | |
Homoranthus virgatus A.Cunn. ex Schauer | Chamelauciinae | NSW706370 | P.G.Wilson 1709 | 376921 | |
Hypocalymma angustifolium (Endl.) Schauer | Astarteinae | NA | K.W.Dixon s.n. | ERR5033722 | |
Hypocalymma puniceum C.A.Gardner | Astarteinae | NSW448547 | P.G.Wilson 1500 | 376906 | |
Hypocalymma robustum (Endl.) Lindl. | Astarteinae | PERTH 8507562 | R.J.Cranfield 25813 | 376959 | |
Hypocalymma verticillare Rye | Astarteinae | PERTH 7131240 | B.L.Rye; E.D.Middleton BLR 250505 | 376960 | |
Hysterobaeckea aff. behrii | Hysterobaeckeinae | CANB 892274.2 | D.E.Albrecht 14664 | 376990 | |
Hysterobaeckea behrii (Schltdl.) Rye | Hysterobaeckeinae | NSW442319 | P.G.Wilson 1495 | 376905 | |
Hysterobaeckea ochropetala subsp. ochropetala (F.Muell.) Rye | Hysterobaeckeinae | PERTH 8911096 | G.Cockerton; D.Brassington; D.Leach WB 38590 | 376961 | |
Hysterobaeckea petraea Rye | Hysterobaeckeinae | NSW418671 | P.G.Wilson 1388 | 376904 | |
Hysterobaeckea tuberculata (Trudgen) Rye | Hysterobaeckeinae | NSW607950 | P.G.Wilson 1587 | 376985 | |
Kardomia jucunda (S.T.Blake) Peter G.Wilson | Hysterobaeckeinae | K000065827 | L.S.Smith 14108 | ERR5034132 | |
Kardomia odontocalyx (A.R.Bean) Peter G.Wilson | Hysterobaeckeinae | NSW622797 | L.M.Copeland 3328 | 376979 | |
Kardomia squarrulosa (Domin) Peter G.Wilson | Hysterobaeckeinae | NSW495858 | B.R.Jackes 2201 | 376901 | |
Leptospermopsis erubescens (Schauer) Peter G.Wilson | Outgroup | NA | R.J.Smith; L.Sweedman; S.Hopper; N.McQuoid; R.Garlett 295 | ERR5034013 | |
Leptospermum polygalifolium subsp. tropicum Joy Thomps. | Outgroup | CNS 143594.1 | B.S.Wannan 6676 | 376911 | |
Lindsayomyrtus racemoides (Greves) Craven | Outgroup | QRS 119161.1 | B.Gray 7662 | 376910 | |
Malleostemon pentagonus Rye & Trudgen | Hysterobaeckeinae | PERTH 7757778 | M.E.Trudgen; M.Guest MET 22545 | 376962 | |
Malleostemon pustulatus Rye | Hysterobaeckeinae | K000065833 | L.S.J.Sweedman 7506 | ERR5084304 | |
Malleostemon roseus (E.Pritz.) J.W.Green | Hysterobaeckeinae | NSW414891 | P.G.Wilson 1385 | 376991 | |
Malleostemon sp. Adelong (G.J.Keighery 11825) | Hysterobaeckeinae | PERTH 4505395 | D.True; C.McChesney DET TTR 2/31 | 376963 | |
Malleostemon sp. Officer Basin (D.Pearson 350) | Hysterobaeckeinae | PERTH 8179417 | S.Reiffer SRE 024 | 376964 | |
Malleostemon tuberculatus (E.Pritz.) J.W.Green | Hysterobaeckeinae | NSW448519 | P.G.Wilson 1530 | 376903 | |
Micromyrtus delicata A.R.Bean | Micromyrtinae | CNS 141177.1 | R.L.Jago 7512 | 376915 | |
Micromyrtus elobata (F.Muell.) Benth. subsp. elobata | Micromyrtinae | NSW608165 | P.G.Wilson 1633 | 376988 | |
Micromyrtus flava (J.W.Green) Rye & Peter G.Wilson | Micromyrtinae | K000065818 | J.Coleby-Williams 137 | ERR5034881 | |
Micromyrtus gracilis A.R.Bean | Micromyrtinae | NSW494544 | P.G.Wilson 1557 | 376983 | |
Micromyrtus obovata (Turcz.) J.W.Green | Micromyrtinae | K000065834 | B.L.Rye; M.E.Trudgen 231087 | ERR5034887 | |
Micromyrtus serrulata J.W.Green | Micromyrtinae | NSW608513 | P.G.Wilson 1605 | 376986 | |
Ochrosperma lineare (C.T.White) Trudgen | Ochrosperminae | K000065837 | L.M.Copeland 3722 | ERR5034137 | |
Ochrosperma sulcatum A.R.Bean | Ochrosperminae | NSW495861 | G.J.Leach 4681 | 376984 | |
Osbornia octodonta F.Muell. | Outgroup | QRS 91700.1 | J.R.Clarkson 7414 | 376909 | |
Oxymyrrhine coronata Rye & Trudgen | Hysterobaeckeinae | PERTH 8811903 | K.R.Thiele 4427 | 376965 | |
Pericalymma spongiocaule Cranfield | Outgroup | K000065839 | R.J.Cranfield 8988 | ERR5034138 | |
Pileanthus filifolius Meisn. | Chamelauciinae | NSW448572 | P.G.Wilson 1513 | 376907 | |
Pileanthus limacis Labill. | Chamelauciinae | K000065840 | L.S.J.Sweedman 6744 | ERR5034890 | |
Rinzia carnosa (S.Moore) Trudgen | Rinziinae | K000065843 | F.M.C.Schock 122866 | ERR5034891 | |
Rinzia ericaea (F.Muell. ex Benth.) Rye | Rinziinae | NSW441865 | P.G.Wilson 1493 | 376976 | |
Rinzia fumana Schauer | Rinziinae | PERTH 8332061 | K.R.Thiele 3886 | 376966 | |
Rinzia icosandra (F.Muell. ex Benth.) Rye | Rinziinae | PERTH 8197369 | M.Trudgen; B.Moyle MET 22535 A | 376967 | |
Rinzia longifolia Turcz. | Rinziinae | PERTH 9100423 | E.M.Sandiford EMS 2325 | 376968 | |
Rinzia polystemonea (F.Muell.) Rye | Rinziinae | NSW541599 | D.E.Albrecht 9391 | 376969 | |
Rinzia torquata Rye & Trudgen | Rinziinae | NSW608660 | P.G.Wilson 1664 | 376970 | |
Sannantha angusta (A.R.Bean) Peter G.Wilson | Hysterobaeckeinae | CNS 131921.1 | A.Ford 5572 | 376914 | |
Sannantha crassa (A.R.Bean) Peter G.Wilson | Hysterobaeckeinae | K000065844 | R.Johnstone; L.Gannon 3480 | ERR5034892 | |
Sannantha virgata (J.R.Forst. & G.Forst.) Peter G.Wilson | Hysterobaeckeinae | K000065824 | J.M.Veillon 7498 | ERR5034131 | |
Scholtzia involucrata (Endl.) Druce | Hysterobaeckeinae | PERTH 7891415 | K.R.Thiele 3159 | 376971 | |
Scholtzia sp. Geraldton (F.Lullfitz L 3216) | Hysterobaeckeinae | PERTH 5527996 | S.Patrick 3363 | 376972 | |
Scholtzia uberiflora F.Muell. | Hysterobaeckeinae | K000065845 | M.E.Trudgen 21667 | ERR5034893 | |
Seorsus clavifolius (C.A.Gardner) Rye & Trudgen | Astarteinae | K000065846 | J.A.Cochrane 4838 | ERR5034141 | |
Seorsus intratropicus (F.Muell.) Rye & Trudgen | Astarteinae | NSW519753 | A.A.Mitchell 7017 | 376902 | |
Stenostegia congesta A.R.Bean | Stenostegiinae | K000843452 | I.Cowie; C.Mangion 7320 | ERR5034899 | |
Tetrapora preissiana Schauer | Hysterobaeckeinae | K000065848 | N.N.Donner 2800 | ERR5034894 | |
Thryptomene australis Endl. subsp. australis | Thryptomeninae | K000065849 | B.L.Rye; M.E.Trudgen 250702 | ERR5034895 | |
Thryptomene hexandra C.T.White | Thryptomeninae | NSW494411 | P.G.Wilson 1540 | 376980 | |
Thryptomene oligandra F.Muell. | Thryptomeninae | MBA 8244.1 | I.D.Fox 2185 | 376916 | |
Thryptomene parviflora (F.Muell. ex Benth.) Domin | Thryptomeninae | NSW494536 | P.G.Wilson 1554 | 376981 | |
Thryptomene planiflora Rye | Thryptomeninae | NSW607938 | P.G.Wilson 1670 | 376987 | |
Thryptomene remota A.R.Bean | Thryptomeninae | NSW615197 | K.Brennan 5982 | 376900 | |
Triplarina nitchaga A.R.Bean | Triplarininae | NSW808824 | B.S.Wannan 1903 | 376978 | |
Triplarina paludosa A.R.Bean | Triplarininae | K000065850 | A.R.Bean 14257 | ERR5034896 | |
Verticordia brownii (Desf.) DC. | Chamelauciinae | PERTH 8437998 | C.D.Turley; R.M.Hoggart NO. 10/12-11 | 376932 | |
Verticordia cooloomia A.S.George | Chamelauciinae | PERTH 8764727 | L.S.J.Sweedman 7910 | 376938 | |
Verticordia cunninghamii Schauer | Chamelauciinae | PERTH 8356815 | P.Docherty 170 | 376926 | |
Verticordia dasystylis A.S.George subsp. dasystylis | Chamelauciinae | PERTH 8284962 | S.Reiffer SRE 520 | 376929 | |
Verticordia fastigiata Turcz. | Chamelauciinae | PERTH 9098240 | G.Byrne 6783 | 376924 | |
Verticordia habrantha Schauer | Chamelauciinae | PERTH 9097325 | G.Byrne 6933 | 376928 | |
Verticordia helmsii S.Moore | Chamelauciinae | PERTH 8798591 | N.Murdock NM 129 | 376925 | |
Verticordia humilis Benth. | Chamelauciinae | PERTH 8962731 | M.Hislop 4611 | 376923 | |
Verticordia insignis Endl. subsp. insignis | Chamelauciinae | PERTH 8866058 | G.J.Keighery; B.J.Keighery 1996 | 376931 | |
Verticordia minutiflora F.Muell. | Chamelauciinae | PERTH 8734194 | G.Byrne 5054 | 376922 | |
Verticordia monadelpha var. callitricha (Meisn.) A.S.George | Chamelauciinae | PERTH 8709726 | L.S.J.Sweedman 8397 | 376933 | |
Verticordia nitens (Lindl.) Endl. | Chamelauciinae | PERTH 8121818 | D.A.Mickle; M.L.Swinburn 394 | 376937 | |
Verticordia ovalifolia Meisn. | Chamelauciinae | PERTH 8789789 | G.Byrne 4403 | 376973 | |
Verticordia oxylepis Turcz. | Chamelauciinae | PERTH 7893752 | K.R.Thiele 3430 | 376939 | |
Verticordia pennigera Endl. | Chamelauciinae | PERTH 9199071 | G.J.Keighery; B.J.Keighery 2561 | 376936 | |
Verticordia picta Endl. | Chamelauciinae | PERTH 8855390 | F.Hort; J.Hort FH 4068 | 376934 | |
Verticordia plumosa (Desf.) Druce var. plumosa | Chamelauciinae | PERTH 8924856 | G.Byrne 5781 | 376935 |
In the GAP bioplatform column, ERR samples correspond to PAFTOL sequence data.
DNA extractions were performed using QIAGEN DNEasy Plant Minikits (Hilden, Germany). All other laboratory work was completed at the Australian Genome Research Facility, Melbourne. Genomic DNA extracts were fragmented enzymatically to ~350 base pairs, except in samples that were already sheared through natural degradation. DNA libraries were prepared with NEBNextUltra II FS Library Prep Kit (New England Biolabs, Ipswich, MA, USA). Hybridisation capture was carried out on pools of 12–16 libraries, enriched using the Angiosperms353 v1 bait kit (Johnson et al. 2019) with V5 chemistry; probes were produced by myBaits target capture kit (Daicel Arbor Biosciences, Ann Arbor, MI, USA; catalogue number #308108.v5). After hybridisation capture, enriched libraries were sequenced on Illumina NovaSeq. 6000 SP (Illumina Inc., San Diego, CA, USA) with v1.5 chemistry and generating 150-bp paired-end reads.
Bioinformatic data processing
High-throughput 150-bp paired-end and combined unpaired reads were imported into CLC Genomics Workbench (ver. 20.0.2, see https://digitalinsights.qiagen.com/) for trimming using a quality score limit of 0.05 (Phred score ~13) and discarding reads with length <50 nucleotides. The cleaned reads for each individual were randomly sampled to 4 million reads, to reduce computational burden for downstream processing steps.
The cleaned reads were used to recover the Angiosperms353 target sequences using HybPiper (ver. 2.1.1, see https://github.com/mossmatters/HybPiper; Johnson et al. 2016) with a target file comprising sequences of Leptospermum erubescens Schauer, Pericalymma spongiocaule Cranfield and Eucalyptus grandis W.Hill, sourced from the Kew Tree of Life Explorer (Baker et al. 2021). The HybPiper ‘assemble’ command was run using BLASTx (ver. 2.14.0; Camacho et al. 2009) to map reads to the translated target sequences and the mapped reads were subsequently assembled de novo using SPAdes (ver. 4.0.0, see https://github.com/ablab/spades; Bankevich et al. 2012). Coding sequences (hereafter exons) were extracted using exonerate (Slater and Birney 2005), and the regions flanking the exons (i.e. introns and untranslated regions, UTRs) were recovered using the ‘--run_intronerate’ flag and combined with the exons to form supercontig sequences. The ‘paralog_retriever’ command was used to recover putatively paralogous exon sequences.
We used the Paragone (ver. 1.0.0, see https://github.com/chrisjackson-pellicle/ParaGone) pipeline to process the HybPiper output (Jackson et al. 2023) that provides an implementation of the orthology inference approach developed by Yang and Smith (2014). Following multiple sequence alignment, Paragone uses HmmCleaner (ver. 0.180750, see https://metacpan.org/dist/Bio-MUST-Apps-HmmCleaner; Di Franco et al. 2019), TreeShrink (ver. 1.3.9, see https://github.com/uym2/TreeShrink; Mai and Mirarab 2018) and TrimAl (ver. 1.5, see http://trimal.cgenomics.org/; Capella-Gutiérrez et al. 2009) to remove sequencing and alignment errors, and rogue taxa from multiple sequence alignments. We used the monophyletic outgroups (MO) algorithm to recover putatively orthologous gene alignments for the exon data using an internal outgroup file comprising Leptospermum erubescens, Leptospermum polygalifolium Salisb. and Pericalymma spongiocaule. Paragone was used to generate cleaned sequence alignments (i.e. all the pre-paralogy resolution subcommands were run) for the supercontig data. We used the MAFFT (ver. 7.471, see https://mafft.cbrc.jp/alignment/software/; Katoh and Standley 2013) ‘mafft_auto’ algorithm, gene tree inference was performed using FastTree 2 (ver. 2.1.1, see http://www.microbesonline.org/fasttree; Price et al. 2010) and TreeShrink was run with a q value of 0.2 for the alignment steps. Alignments with fewer than 20 sequences were removed from downstream analyses.
Phylogenetic analyses
The exon and supercontig gene alignments output from Paragone were each concatenated using Geneious Prime (ver. 2022.0.1, see https://www.geneious.com) and we used IQ-TREE (ver. 2.2.0, http://www.iqtree.org/; Nguyen et al. 2015; Chernomor et al. 2016) to generate maximum likelihood (ML) phylogenies. ModelFinder (ver. 1.0, see http://www.iqtree.org/ModelFinder/; Kalyaanamoorthy et al. 2017) was used to estimate the best partitioning scheme and models (–MFP + MERGE) with the relaxed hierarchical clustering algorithm (Lanfear et al. 2014) restricting the search to the top 10% of partition merging schemes (–rclust 10) for the concatenated data. Branch support was estimated using the best partitioning scheme with 1000 ultrafast bootstrap replicates (Minh et al. 2013) and the IQ-TREE implementation of the approximate likelihood ratio test (aLRT; Guindon et al. 2010), with interpretation of support following Minh et al. (2013) and recommendations in the IQ-TREE manual (see http://www.iqtree.org/doc/Frequently-Asked-Questions).
In addition to the concatenated analyses, we estimated coalescent-based species trees for the exon and supercontig data sets using weighted ASTRAL (wASTRAL; Zhang and Mirarab 2022) as implemented in Accurate Species Tree Estimator (ASTER, ver. 1.19, see https://github.com/chaoszhang/ASTER). We inferred gene trees for each locus using IQ-TREE with support estimated using the approximate Bayes test (Anisimova et al. 2011). The wASTRAL algorithm attempts to account for gene tree uncertainty in the species tree estimates by weighting to reduce the impact of long terminal branches and low branch support. We followed the authors’ recommendations (see https://github.com/chaoszhang/ASTER/blob/master/tutorial/wastral.md) for upper and lower thresholds for local Bayesian support (1.0 and 0.33 respectively) and used local posterior probability (LPP) to measure internal branch support for the species tree estimate.
Both the concatenated and coalescent based species trees were further interrogated using quartet sampling (QS; Pease et al. 2018). The QS approach subsamples quartets from the target tree and alignment to produce four metrics: Quartet Concordance (QC), Quartet Differential (QD), Quartet Informativeness (QI) and Quartet Fidelity (QF). Taken together, QS scores describe the degree of topological variation in the data (Pease et al. 2018), providing a valuable alternative to measures such as bootstrap that can be a poor measure of variation in the data, particularly among large data sets (Lanfear and Hahn 2024). We ran QS analyses with 500 replicates and a log-likelihood cut-off of 2.
We focused on the supercontig concatenated dataset for our phylogenetic dating, biogeographic and diversification analyses as this is more phylogenetically informative (double the total alignment length) than the exon dataset. We used the concatenated as opposed to the coalescent topology as our QS analyses indicated that low QC support along the backbone is due to low phylogenetic informativeness of individual genes rather than significant conflict or skewed discordance (Pease et al. 2018). Inaccurate or poorly resolved gene trees may limit the reconstruction of a more accurate species tree than those from concatenation approaches (Gatesy and Springer 2014). Furthermore, studies have shown that concatenation approaches can perform equally well or even better than coalescent approaches that attempt to account for incomplete lineage sorting (Tonini et al. 2015).
Phylogenetic dating
We used BEAST (ver. 2.4.7, see https://www.beast2.org/; Bouckaert et al. 2014) for divergence age estimates. Although there are pollen microfossils of Chamelaucieae (Thornhill et al. 2012, 2015), these cannot be confidently assigned to genera or groups within the tribe due to homoplasy and a lack of diagnostic characters. We therefore used the Chamelaucieae + Leptospermeae crown age (uniform prior: 47.8–103 Ma) from Thornhill et al. (2015), based on an Eocene Myrtaceidites Cookson & K.M.Pike fossil (Thornhill and Macphail 2012), as a secondary calibration in the BEAST analyses, following Nge et al. (2022). The maximum age constraint for our analyses was set to 103 Ma for Myrtaceae based on the stem age of the family from the BEAST analyses of an angiosperm-wide study with 238 fossil calibrations (Ramírez-Barahona et al. 2020).
We used a gene-shopping approach using the SortaDate pipeline (see https://github.com/FePhyFoFum/sortadate; Smith et al. 2018) to account for computational limitations and achieve adequate convergence (>200 effective sample size; ESS) from the BEAST analyses. We selected a subset of the most clock-like loci of the 300+ nuclear markers using SortaDate (root-to-tip variance filtering option). We tested for the effects of different subset sizes (10, 20 and 30 loci) on divergence age estimates. As the effects of subset sizes are minimal, we used the 30 most clock-like loci as the main dataset for this study, as a balance between including more loci while achieving computationally feasible convergence in BEAST (see Supplementary Table S1). We constrained the starting topology using a chronogram obtained from treePL (ver. 1.0, see https://github.com/blackrim/treePL; Smith and O’Meara 2012). The input topology for treePL was obtained using IQ-TREE. The treePL topology was calibrated using the same secondary calibration points for our BEAST analysis, allowing the runs to initialise successfully, as the specified calibration nodes are required to overlap with the respective nodes in the starting topology. We also conducted sensitivity tests for the dating analyses. We tested the effects of genetic locus sampling on our dating analyses by conducting BEAST analyses on subsets of the 10, 20 and 30 most clock-like loci from the gene-shopping approach. As divergence age estimates were not significantly different for these subsets (Supplementary Fig. S1 and Table S1), we used the 30-gene subset for subsequent biogeographic and diversification analyses.
The configuration XML file for BEAST was created using BEAUti (ver. 2.4.7, see https://beast.community/beauti), with the 30 most clock-like loci concatenated as the input alignment and a constrained treePL starting topology provided as a Newick tree file. The GTR + G substitution model was applied for the entire alignment (Abadi et al. 2019). The relaxed log-normal clock model and birth–death tree priors were chosen in BEAUti. Secondary calibrations were implemented as uniform priors, with the 95% confidence intervals (CI) from Thornhill et al. (2015) specified as minimum and maximum bounds. Three independent BEAST runs were performed with 50 million Markov Chain Monte Carlo (MCMC) chains each, to ensure convergence towards a global instead of a local optimum, sampling every 1000th generation. Convergence (>200 ESS) for each run was assessed using Tracer (ver. 1.6, A. Rambaut, M. A. Suchard, D. Xie and A. J. Drummond, see https://github.com/beast-dev/tracer/releases/tag/v1.6) with the log files as input. The first 20% MCMC chains for each run were discarded as burn-in. The output tree files from the three runs were subsequently combined using LogCombiner (ver. 2.4.7, see https://beast.community/logcombiner) and annotated in TreeAnnotator (ver. 2.4.7, see https://www.beast2.org/treeannotator/). The output dated phylogenies were visualised in FigTree (ver. 1.4.3, A. Rambaut see https://github.com/rambaut/figtree/releases) and final figures edited in Inkscape (ver. 1.2, see https://inkscape.org, accessed April 2024). All outgroups were pruned from the dated BEAST phylogeny using the ‘drop.tip’ function in the R package ape (ver. 5.3, see http://ape-package.ird.fr/; Paradis et al. 2004) prior to biogeography and diversification analyses, as we focused on the crown diversification of the tribe in this study. Investigating the stem diversification of Chamelaucieae would require a more extensive and complete sampling of sister outgroups than is in scope for this paper.
Biogeographic analyses
The geographic distribution of each sequenced taxon was determined using vouchered herbarium records obtained from the Australasian Virtual Herbarium (see http://avh.chah.org.au, accessed March 2023) and cross-validated with Florabase for recent taxonomic changes of Western Australian taxa (see https://florabase.dbca.wa.gov.au, accessed April 2023). Cultivated and erroneous records (e.g. outliers, records in the ocean) were excluded. We delimited six geographic regions that largely correspond to present-day Australian biomes (Table 2): south-west Western Australia (W; Mediterranean climate), arid Eremaean interior (A), eastern Australia (E; temperate to subtropical), northern Australia including the Kimberley and Top End regions (N; monsoonal), Cape York peninsula and north-eastern Australia (C; monsoonal, wet tropical and subtropical) and the semi-arid transition zone between W and A (T). Region boundaries were based on the Interim Biogeographic Regionalisation of Australia (IBRA; Environment Australia 2000), following previous studies with some modifications (see Supplementary Table S3; Cook et al. 2015; Nge et al. 2022, 2023). As many Chamelaucieae endemic or near-endemic taxa occur around the semi-arid periphery of south-west Western Australia (i.e. part of the Eremaean but not strictly in the arid interior), we delimited the two adjacent IBRA regions (Yalgoo and Coolgardie) as a separate region (T). We separated regions N and C across the Gulf of Carpentaria for northern Australia, as this has been demonstrated to be a significant biogeographic barrier for many taxa (Bowman et al. 2010). The New Caledonian Sannantha virgata (J.R.Forst. & G.Forst.) Peter G.Wilson was coded as occurring in eastern Australia, to limit the number of biogeographic regions due to computational constraints and because the study focuses on biogeographic disjunctions within Australia.
Biogeographic regions | Coding abbreviation | |
---|---|---|
South-west Western Australia | W | |
Arid Eremaean zone | A | |
Transitional semi-arid zone between W and A | T | |
Eastern Australia | E | |
Northern Australia (Kimberley + Top End) | N | |
Cape York and north-eastern Australia | C |
Biogeographic analyses conducted using BioGeoBEARS (ver. 1.1, N. J. Matzke, see https://github.com/nmatzke/Matzke_R_binaries; Matzke 2013) in R (ver. 3.5.1, R Foundation for Statistical Computing, Vienna, Austria, see https://www.r-project.org/) inferred relative probabilities of ancestral areas for each node in the dated phylogeny. We included variations of the dispersal–extinction–cladogenesis (DEC; Ree and Smith 2008) and dispersal–vicariance (DIVA; Ronquist 1997) models. We excluded BAYAREA models (Landis et al. 2013) as these do not account for vicariance (Pontes-Nogueira et al. 2021). As in previous studies, we included additional model parameters such as jump dispersal (j), dispersal as a function of geographic distance (x) and time stratified parameters that included information on how respective regions across Australia have shifted through time (Nge et al. 2022, 2023). We implemented a scenario of progressive aridification and climate cooling since the Oligocene (32 Ma) by progressively larger geographic dispersal distances between regions (W+T, N+C, E), with an expanding arid centre as a barrier for dispersal (see Supplementary Table S4). Results from the biogeographic analyses were compared using Akaike Information Criterion scores (AIC; Akaike 1974) in the BioGeoBEARS package to determine the best model for our dataset.
Homalocalyx F.Muell., Ochrosperma Trudgen, and Calytrix were underrepresented geographically compared with other genera in our study. To account for this, we conducted two separate BioGeoBEARS analyses with different geographic codings for these genera. The first analysis used actual geographic coding of the sampled species. The second took into account results from a previously published study by Nge et al. (2022) with near-complete taxonomic sampling and complete geographic representation for Calytrix, and unpublished data for Homalocalyx and Ochrosperma (with complete sampling of all species for both) that inferred widespread ancestral areas for these genera. Accordingly, the second coding gave Calytrix, Homalocalyx and Ochrosperma widespread distributions. Results from both coding regimes were similar and we focus on the second analysis as we believe this is a more accurate representation of the biogeographic history of these genera (see the Supplementary material for details, Fig. S2). We also conducted a sensitivity analysis for BioGeoBEARS with the wASTRAL (supercontig) topology instead of the IQ-TREE topology, dated using treePL instead of BEAST or starBEAST due to computational constraints. This analysis also used the widespread geographic distribution coding for Homalocalyx, Ochrosperma and Calytrix.
We estimated the number and directions of dispersal–vicariant patterns to test H2 (asymmetrical dispersal patterns) in BioGeoBEARS using biogeographic stochastic mapping (BSM; Matzke 2015). Event frequencies were taken from the mean of event counts across 50 BSMs.
Diversification analyses
We applied the paleoenvironmental birth–death models of Condamine et al. (2013) using RPANDA (ver. 1.8, see https://cran.r-project.org/package=RPANDA; Morlon et al. 2016) in R to test for climate-dependent diversification patterns (H3). We compared pure birth (no extinction), constant birth–death, and time-dependent diversification models with other birth–death models that incorporated global temperature and sea-level changes. Variations in speciation and extinction rates (constant v. exponential) were also included as part of the model testing (Table 3), resulting in 14 different models. We chose the best-fitting model as the one with the highest AIC weight (out of a value of 1).
Model abbreviations | Model | Mean AIC weights | |
---|---|---|---|
BCST | Pure birth | 0 | |
BCSTDCST | Constant birth–death | 0 | |
BTimeVar | Speciation varies exponentially through time without extinction | 0.0 | |
BTimeVarDCST | Speciation varies exponentially through time with constant extinction | 0.0 | |
BCSTDTimeVar | Constant speciation and extinction varies exponentially through time | 0.0 | |
BTimeVarDTimeVar | Both speciation and extinction vary exponentially through time | 0.0 | |
BTempVar | Speciation varies exponentially with temperature without extinction | 0.7 | |
BTempVarDCST | Speciation varies exponentially with temperature and extinction is constant | 0.2 | |
BCSTDTempVar | Constant speciation and extinction varies exponentially with temperature | 0.0 | |
BTempVarDTempVar | Both speciation and extinction vary exponentially with temperature | 0.1 | |
BSeaLevelVar | Speciation varies exponentially with SeaLevel without extinction | 0 | |
BSeaLevelVarDCST | Speciation varies exponentially with SeaLevel and extinction is constant | 0 | |
BCSTDSeaLevelVar | Constant speciation and extinction varies exponentially with SeaLevel | 0 | |
BSeaLevelVarDSeaLevelVar | Both speciation and extinction vary exponentially with SeaLevel | 0 |
Heatmap colours represent low–high numerical values (blue–white–red; least to most likely), with the top three best-fitting models highlighted in red.
We used Bayesian analyses of macroevolutionary mixtures (BAMM; Rabosky 2014) on our dated BEAST phylogeny to test for diversification rate-shifts (H4). Three sampling fractions were specified to test the effects of incomplete taxonomic sampling: (1) the sampling fraction for each subtribe was specified for BAMM (Supplementary Table S5), (2) a global sampling fraction for the entire Chamelaucieae tribe was specified based on the total number of described species from Rye et al. (2020) (18.8%, 124/660 spp.) and (3) a more conservative global sampling fraction for the tribe based on the total number of described and phased-named taxa from Rye et al. (2020) (15%, 124/810 spp.). The BAMM analyses were run for 5 million and 20 million MCMC generations for the global sampling and subtribal sampling fractions respectively, to achieve convergence (>200 ESS). Convergence of each run was assessed using BAMMtools (ver. 2.1.10, see https://cran.r-project.org/package=BAMMtools; Rabosky et al. 2014) in R. The first 10% of BAMM runs was discarded as burn-in. Credibility diversification rate shifts were also determined using BAMMtools, with two different expected rate shifts as our priors: (1) one expected rate shift event as recommended for smaller phylogenies with <200 tips, and (2) three expected rate shifts to account for the wider unsampled diversity of the tribe (660+ species).
We also used GeoHiSSE (ver. 1.9.6; Caetano et al. 2018) in R to compare diversification rates between south-west Western Australia and other regions (i.e. geographic-dependent diversification). GeoHiSSE implements 36 models to test geographic-dependent speciation or extinction and hidden states (Supplementary Table S6). As GeoHiSSE only analyses binary traits, the geographic coding used in our biogeographic analyses were collapsed to two regions (W and non-W). All taxa with occurrence records in W (even non-endemics) were coded as ‘W’. AIC scores were used to determine the best-fitting model.
Results
Data set construction
The average number of reads that were mapped to target sequences using HybPiper was ~340,000 comprising ~10% of our subsampled sequence reads (Supplementary Table S2). In general, gene recovery was high across samples averaging ~310 genes with sequences and ~255 genes recovered with at least 50% of the target length. After paralog filtering, 295 loci were retrieved from the MO option in Paragone. The final exon and supercontig alignments are 155,922 bp (292 loci) and 367,371 (341 loci) in length respectively, including 131 taxa.
Phylogenomic relationships and divergence time of Chamelaucieae
We use the following terms to discuss support values in our phylogenetic trees: (1) nodes with aLRT = 100, BS = 100, LPP = 1, QC/QD/QI = 1/NA/1 are fully supported, (2) nodes with aLRT > 80, BS > 95 LPP > 0.85, QC > 0.5 are strongly supported, (3) nodes with aLRT 80, BS 95, LPP 0.85–0.6, QC 0.3–0.5 are moderately supported, and (4) nodes with aLRT < 80, BS < 95, LPP < 0.6, QC < 0.3 are weakly supported. Support values for nodes across Chamelaucieae are generally higher for the supercontigs than the exons dataset. In general, we recovered backbone topologies with strongly supported values from aLRT/BS/LPP and weaker support from QS.
Several notable differences in topology and support values were detected across different analyses (concatenated v. coalescent) and from different datasets (exon v. supercontig) (Fig. 2, 3). Despite these differences, there are several consistent findings from our results. Chamelaucieae was recovered as monophyletic, sister to Leptospermeae with full support in all analyses across both datasets. Ochrospermatinae is recovered as the sister taxon to all other Chamelaucieae subtribes, albeit with weak support across analyses in both datasets. Rinziinae sens. lat. is non-monophyletic with strong to moderate support across all analyses and both datasets.
Phylogenetic relationships of subtribes in Chamelaucieae based on concatenated (IQ-TREE) and coalescent (ASTRAL) approaches for supercontig and exon datasets. (a) IQ-TREE and (b) ASTRAL topologies based on the supercontig dataset. (c) IQ-TREE and (d) ASTRAL topologies based on the exons dataset. Support values are based on approximate likelihood ratio tests (aLRT)/ultrafast bootstrap (BS) for IQ-TREE and local posterior probability (LPP) for ASTRAL. Nodes have full support (100/100/1; aLRT/BS/LPP) unless otherwise highlighted with red circles, and support values shown. Rinziinae* includes Enekbatus and Rinzia, and Rinziinae** includes Euryomyrtus.
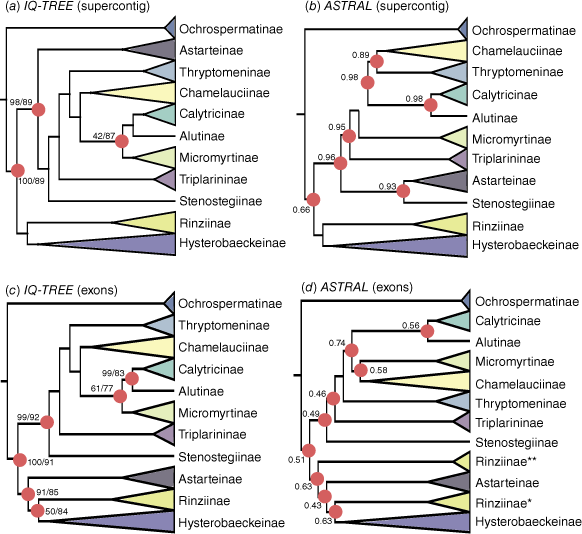
Maximum likelihood IQ-TREE phylogeny of Chamelaucieae based on the supercontig dataset. Support values are approximate likelihood ratio tests (aLRT) and ultrafast bootstrap (BS). Nodes with full support (100/100, aLRT/BS) are indicated with black circles. Nodes without full support are highlighted as red circles, with actual support values shown. Cladogram depicts phylogenetic relationships across subtribes, and Clade A, Clade B.
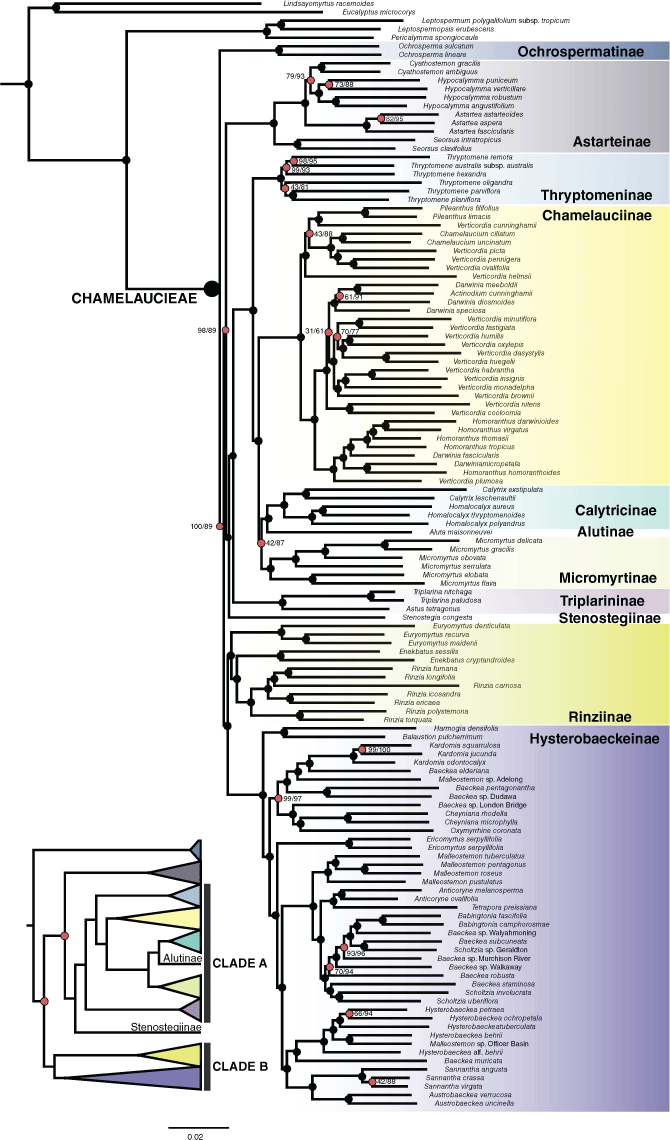
All subtribes except for Rinziinae sens. lat. are recovered as monophyletic with strong support based on concatenated and coalescent support values. Support for these relationships was lower based on the quartet sampling approach, with most subtribes (6/11) recovered as monophyletic with strong support from the exon dataset. This increased to eight subtribes with strong support based on the supercontig dataset (with monophyly of Micromyrtinae and Thryptomeninae respectively now strongly supported). The remaining subtribes are recovered as monophyletic with moderate to weak support from quartet sampling.
Clade A, consisting of five subtribes (Chamelauciinae, Calytricinae, Alutinae, Micromyrtinae and Thryptomeninae), had moderate to strong support across analyses and the two datasets (Fig. 2). Clade B (Hysterobaeckeinae and Rinziinae sens. str.) was recovered with strong support from the supercontig dataset but moderate to weak support from the exon dataset, across both concatenated and coalescent approaches. The phylogenetic placements of Stenostegiinae and Astarteinae are uncertain. The placement of Stenostegiinae alternates from being either sister to the remainder of Clade A (supercontig IQ-TREE, exon ASTRAL, exon IQ-TREE) or sister to Astarteinae (supercontig ASTRAL), with high to full support from aLRT/BS/LPP and weak support from QC. Astarteinae either forms a grade with Clade A (supercontig dataset) or is sister to Clade B (exon dataset), with strong to moderate support from aLRT/BS/LPP and weak support from QC. Different topologies were recovered from the exon dataset depending on the analysis method. The concatenated approach showed Rinziinae sens. str. to be non-monophyletic, with Euryomyrtus Schauer forming a grade with Astarteinae, the rest of Rinziinae sens. str. (Enekbatus Trudgen & Rye, Rinzia Schauer) and Hysterobaeckeinae. By contrast, the coalescent approach showed Astarteinae forming a grade with Clade A (Rinziinae sens. str. as monophyletic and Hysterobaeckeinae).
The topological placement of subtribe Micromyrtinae also alternates within Clade A, with differing topologies from concatenated and coalescent approaches across both datasets. Resulting IQ-TREEs showed that the subtribe is recovered as sister to Alutinae + Calytricinae from the supercontig dataset and sister to Calytricinae only from the exon dataset, with weak support (aLRT/BS/LPP/QC). However, in coalescent ASTRAL trees, Micromyrtinae is sister to Chamelauciinae with weak to strong (LPP = 0.98 from supercontig dataset) support.
Several genera in Chamelaucieae including Homoranthus, Verticordia, Darwinia, Baeckea sens. lat., Malleostemon and Hysterobaeckea (Nied.) Rye were recovered as non-monophyletic with full to strong support based on aLRT/BS/LPP but with low QC values.
The stem age of Chamelaucieae was estimated at 63.5 Ma (95% confidence interval; CI: 47.8–88.8 Ma) and the crown radiation at 42.4 Ma (95% CI: 31.2–61.1 Ma; Fig. 4). All subtribes diverged and radiated in the Oligocene to early Miocene (20–33 Ma; Fig. 4). The New Caledonian Sannantha virgata diverged from sister Australian relatives at c. 6.7 Ma (95% CI: 4.1–9.7 Ma).
Maximum likelihood ancestral range estimation of Chamelaucieae (Myrtaceae) based on the best model DIVA + j + x with time stratification (dotted lines) from BioGeoBEARS. Phylogenetic relationships shown are based on the IQ-TREE supercontig topology. Dated phylogeny was constructed with secondary calibrations implemented in BEAST, with 30 top clock-like loci subset from the Angio353 bait kit. Pie diagrams at nodes show relative probability of ancestral states, with common area combinations highlighted in colours and the remainder in grey. See Supplementary Table S7 for all possible area combinations included. The map shows region delimitation across the Australian continent based on IBRA regions: south-west Western Australia (W), semi-arid adjacent transition zone (T), arid centre (A), eastern Australia (E), Cape York and north-eastern Australia (C), and northern Australia (N) that includes the Kimberley and Top End. The red line highlights the Eocene–Oligocene boundary (33 Ma). Subtribes are highlighted in different colours. Sannantha virgata is a New Caledonian endemic.
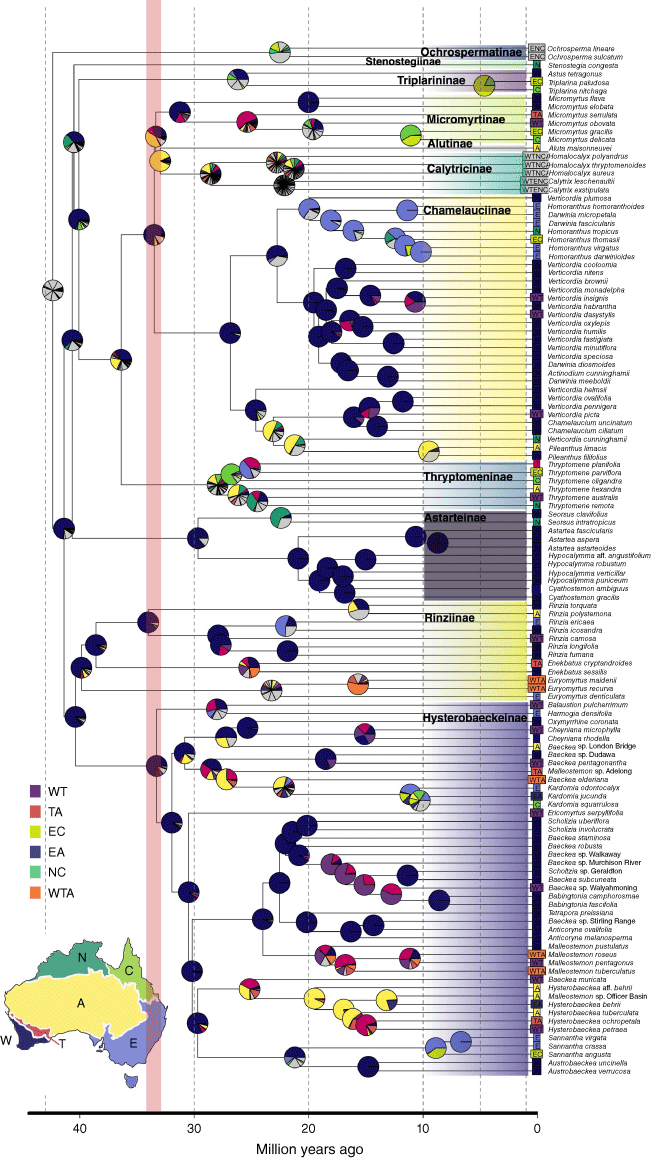
Biogeography
The best-fitting biogeographic model with the lowest AIC score was DIVA + j + x, with the addition of aridification scenario time stratified distance matrices (Supplementary Table S7). The ancestral area for Chamelaucieae was unresolved, with near equal probability of ancestral area occurring across multiple regions of the continent, supporting H1 (Fig. 1, Supplementary Table S8, Fig. S13). However, the ancestral area of Chamelaucieae excluding subtribe Ochrospermatinae (sister to remainder of the tribe) was estimated to be south-west Western Australia (region W; Probability, P = 0.99, node126). Similarly, the ancestral area of the deeper backbone divergences was predominantly estimated to be W (Fig. 2). Furthermore, 5 of the 11 sampled subtribes had the ancestral area estimated to be W: Micromyrtinae, Chamelauciinae, Astarteinae, Rinziinae and Hysterobaeckeinae. The ancestral area for Alutinae + Calytricinae + Micromyrtinae was estimated to be in central Australia (region A; P = 0.58, node 234). The biogeographic results from our wASTRAL topology yielded largely similar results, of widespread ancestral distribution for Chamelaucieae and W as the ancestral area for the deeper backbone divergences (Supplementary Fig. S3). A notable difference between our main results (IQ-TREE topology) and the sensitivity analysis (wASTRAL topology) is the ancestral area of Stenostegiinae and Astarteinae. This was recovered as region N from the wASTRAL topology but W from the IQ-TREE topology due to different placements of the two subtribes in the respective phylogenies (Fig. 4, S3).
Directionality and type of biogeographic events
Most biogeographic events comprised dispersals (49%, 104/213) followed by within-area speciation (42%, 89/213) (Table 4). Very few vicariance events (10%, 20/213) were inferred. Range expansion dispersals were more common (42%, 90/213) than founder events (7%, 14/213).
Mode | Type | Mean (s.d.) | Percentage | |
---|---|---|---|---|
Within-area speciation | Sympatric speciation | 88.86 (2.04) | 41.8 | |
Speciation–subset | 0 | 0.0 | ||
Dispersal | Founder events (j) | 13.72 (2.64) | 6.5 | |
Range expansions (d) | 89.52 (3.65) | 42.1 | ||
Range contractions (e) | 0 | 0.0 | ||
Vicariance | Vicariance | 20.42 (2.82) | 9.6 | |
Total | 212.52 | 100.0 |
Heatmap colours represent low–high values (blue–red).
The direction of dispersal events was found to be strongly asymmetrical, in support of H2 and dominated by dispersals from south-west Western Australia to the semi-arid transition zone (T; 22% of all dispersal events) and arid interior (A; 12%) (Fig. 5, Table 5). Multiple independent dispersal events from W to T occurred in Chamelaucieae including Verticordia sens. lat., Balaustion Hook., Cheyniana Rye and Malleostemon (Fig. 4). Overall, south-west Western Australia was the largest source area of dispersals (49% of all dispersal events) and the semi-arid transition zone the largest sink (33%). The central arid region served as an important connecting area for other parts of Australia, with dispersals recorded outwards to all other regions (Table 5). Dispersals out of northern (N) and eastern (E) Australia were limited, confined to only dispersals into Cape York and north-eastern Australia (C). Dispersal modes also differed between regions: dispersals from W to T were largely range expansions (21%), with very few long-distance founder events (1%). By contrast, dispersal rates from W to A were more similar among range expansions (8%) and founder events (3%). Not surprisingly, range expansions occurred more frequently between adjacent areas (e.g. W and T).
Summary of dispersal events (range expansion and founder events) and associated directionality for Chamelaucieae across Australia, estimated with biogeographic stochastic mapping in BioGeoBEARS (Table 3). Size of arrows represents frequency of dispersal events, red being the most common (W → T). Dashed arrows are the least common dispersal events and events with a frequency of less than 1 are not shown. See Table 5 for frequencies of dispersal events for each area. Letters represent different regions across Australia based on IBRA regions: south-west Western Australia (W), semi-arid adjacent transition zone (T), arid centre (A), eastern Australia (E), Cape York and north-eastern Australia (C) and northern Australia (N) that includes the Kimberley and Top End.
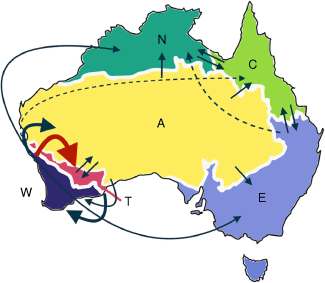
All dispersal | W | T | E | N | C | A | Total | Percentage source | |
---|---|---|---|---|---|---|---|---|---|
W | 0 | 22.06 | 5.24 | 4.14 | 2.42 | 11.54 | 45.4 | 49.0 | |
T | 8.22 | 0 | 0.44 | 0.54 | 1.18 | 4.42 | 14.8 | 16.0 | |
E | 0.4 | 0.26 | 0 | 1.82 | 4.38 | 0.96 | 7.82 | 8.4 | |
N | 0.72 | 0.56 | 0.94 | 0 | 3.02 | 0.86 | 6.1 | 6.6 | |
C | 0.48 | 1.22 | 5.34 | 3.36 | 0 | 0.96 | 11.36 | 12.3 | |
A | 3.2 | 6.44 | 3.06 | 2.32 | 2.74 | 0 | 17.76 | 19.2 | |
Total | 13.02 | 30.54 | 15.02 | 12.18 | 13.74 | 18.74 | 103.24 | ||
Percentage sink | 14.1 | 33.0 | 16.2 | 13.2 | 14.8 | 20.2 |
Founder events | W | T | E | N | C | A | Total | Percentage source | |
---|---|---|---|---|---|---|---|---|---|
W | 0 | 1 | 1.84 | 2.18 | 0.48 | 3.16 | 8.66 | 34.6 | |
T | 0.08 | 0 | 0.12 | 0.04 | 0.26 | 0.22 | 0.72 | 2.9 | |
E | 0.04 | 0.08 | 0 | 0.5 | 0.44 | 0 | 1.06 | 4.2 | |
N | 0.14 | 0.02 | 0.02 | 0 | 0.1 | 0.14 | 0.42 | 1.7 | |
C | 0.04 | 0.56 | 0.06 | 0.02 | 0 | 0.04 | 0.72 | 2.9 | |
A | 0.46 | 0.68 | 0.2 | 0.4 | 0.4 | 0 | 2.14 | 8.5 | |
Total | 0.76 | 2.34 | 2.24 | 3.14 | 1.68 | 3.56 | 13.72 | ||
Percentage sink | 3.0 | 9.3 | 8.9 | 12.5 | 6.7 | 14.2 |
Range expansions | W | T | E | N | C | A | Total | Percentage source | |
---|---|---|---|---|---|---|---|---|---|
W | 0 | 21.06 | 3.4 | 1.96 | 1.94 | 8.38 | 36.74 | 54.4 | |
T | 8.14 | 0 | 0.32 | 0.5 | 0.92 | 4.2 | 14.08 | 20.9 | |
E | 0.36 | 0.18 | 0 | 1.32 | 3.94 | 0.96 | 6.76 | 10.0 | |
N | 0.58 | 0.54 | 0.92 | 0 | 2.92 | 0.72 | 5.68 | 8.4 | |
C | 0.44 | 0.66 | 5.28 | 3.34 | 0 | 0.92 | 10.64 | 15.8 | |
A | 2.74 | 5.76 | 2.86 | 1.92 | 2.34 | 0 | 15.62 | 23.1 | |
Total | 12.26 | 28.2 | 12.78 | 9.04 | 12.06 | 15.18 | 89.52 | ||
Percentage sink | 18.2 | 41.8 | 18.9 | 13.4 | 17.9 | 22.5 |
Total dispersal counts are derived from summing founder (long-distance dispersal) and range expansion events for each category. The relative percentages of source and sink areas are also presented. Heatmap colours represent low–high numerical values (blue–red). Regions across the Australian continent are based on the IBRA classification scheme: south-west Western Australia (W), semi-arid adjacent transition zone (T), arid centre (A), eastern Australia (E), Cape York and north-eastern Australia (C) and northern Australia (N) that includes the Kimberley and Top End.
Temperature-dependent speciation and diversification rate shifts in Chamelaucieae
Temperature-dependent diversification models were overwhelmingly the best-fitting models in the RPANDA analyses, supporting H3 (Table 3). The model with the highest AIC weight (0.66) has speciation varying exponentially with temperature without extinction. In addition, the second- and third-best models were also temperature-dependent, with speciation varying exponentially with temperature and extinction constant (AIC weight 0.23), and both speciation and extinction varying exponentially with temperature (AIC weight 0.08). Speciation rates declined from the initial crown radiation in the Eocene (c. 40 Ma) until after the E-O boundary at 34 Ma (Fig. 6). The speciation rate remained relatively constant thereafter until an increase during the Middle Miocene Climatic Optimum (MMCO; 14–17.5 Ma) and subsequent decline towards the present (Fig. 6). A declining speciation trend towards the present was also found in our BAMM analyses across all different sampling fraction and rate shift regimes (Fig. 6, Supplementary Fig. S4–S7).
Diversification dynamics of Chamelaucieae. (a) Temperature-dependent paleoenvironmental model showing speciation decline towards the present. The Eocene–Oligocene boundary is marked with a red column. (b) Declining speciation rates shown from BAMM analyses. (c) The most credible diversification rate shift configuration from BAMM showing a rate shift at the crown of Chamelaucieae, with sampling fractions specified for each subtribe and an expected rate shift prior of 1.
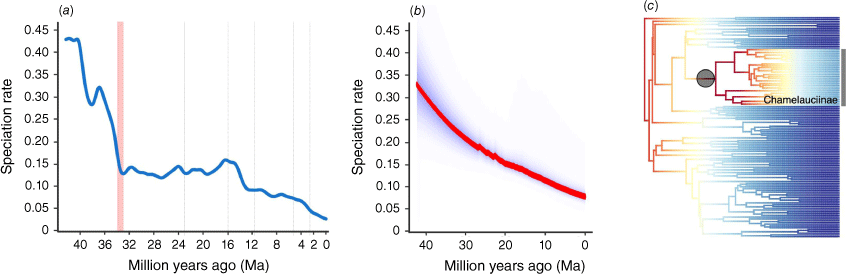
One diversification rate increase was detected, at the crown radiation of Chamelauciinae in the late Oligocene to Miocene (c. 26.85 Ma, 95% CI: 19.1–37.98 Ma), by the BAMM analyses with subsampling fractions specified for each subtribe (Fig. 6, Supplementary Fig. S8–S12). The increased radiation of this subtribe initially occurred in south-west Western Australia based on our BioGeoBEARS results, with subsequent dispersal and smaller radiations in eastern Australia and into the arid interior (Fig. 4). By contrast, no rate shifts were detected when we used a global sampling fraction (both actual and conservative regimes; Fig. S8–S12 and Table S9). Our GeoHiSSE results indicate that model 11 (weighed AIC = 0.5/1) was the best-fitting model, followed by model 17 (weighed AIC = 0.38/1; Table S5). Taken together, these results support H4 (lack of diversification rate shifts in south-west Western Australia).
Discussion
We present the most densely sampled phylogenomic analysis for Chamelaucieae to date, including 10 of the 11 previously named subtribes and 36 of the 37 genera. We provide a better resolved backbone and additional insights into inter-subtribal relationships for Chamelaucieae than in previous studies. We show that the initial crown radiation of the tribe occurred in the Eocene, with diversification of subtribes after the E-O boundary at 33 Ma. This well-resolved phylogeny allowed us to infer the biogeographic history and diversification dynamics of the tribe.
Phylogenetic relationships
Our study presents different topologies for Chamelaucieae depending on the dataset used (exon v. supercontig) and phylogenetic method applied (coalescent or concatenated), with differing levels of support depending on the metric used (see results above). Coalescent and concatenated approaches have increasingly been shown to potentially yield differing topologies even from the same phylogenomic dataset (e.g. Antonelli et al. 2021; Helmstetter et al. 2024, 2025; Nge et al. 2024b; Zuntini et al. 2024). Similarly, differing support values may be obtained from different metrics. Our topologies generally have high aLRT/BS (concatenated) and LPP (coalescent) support values, in contrast to low QC values (indicative of weak majority but not conflicting support for alternate topologies). Although having high LPP support in contrast to low QC values might appear counterintuitive, this is not unusual as these are calculated differently (Sayyari and Mirarab 2016; Pease et al. 2018). The LPP is recommended as the general measure of support for the species topology given that this indicates the probability of a selected node being the true node given the set of gene trees available, assuming the presence of incomplete lineage sorting (ILS; Sayyari and Mirarab 2016). Low QC values along the backbone topologies of Chamelaucieae along with high QD and QI values are likely due to a rapid radiation in the early evolutionary history of the tribe – due to poor phylogenetic informativeness (low QC) likely due to ILS, rather than discordant skew or conflict (low QD) or poor genetic sampling (low QI) (Pease et al. 2018). Ultimately, each different methodological approach has inherent strengths and limitations, and should be assessed critically for each individual case (Joyce et al. 2024, in press). Nevertheless, we argue that these discordances in our topologies should not significantly affect our biogeographic results for several reasons. All subtribes apart from Ochrospermatinae have higher species richness in W, resulting in all backbone nodes apart from the crown node having W as ancestral. The unresolved ancestral area of the Chamelaucieae crown group would likely remain true as Ochrosperma is consistently recovered as the earliest diverging lineage in all phylogenomic analyses, albeit with lower support from the coalescent analyses. All analyses recovered similar topologies within subtribes that were strongly supported as monophyletic (except Rinziinae sens. lat.).
Our phylogenomic trees have some notable differences from previous results based largely on Sanger plastid and rDNA ETS sequence data. All subtribes were recovered as monophyletic except for Rinziinae sens. lat., in which Astus Trudgen & Rye and Triplarina Raf. are separated from Rinziinae sens. str. (Rinzia, Enekbatus and Euryomyrtus), a result foreshadowed by Rye et al. (2020) based on weak support for the combined clade, compared to strong support for each of the two subclades. Ochrosperma was recovered as sister to the remaining Chamelaucieae in all cases. Ochrosperma has the ancestral anther type (dorsifixed, versatile) and what is possibly the ancestral seed type (reniform) that may justify the position as sister to the remainder of the tribe. However, Calytricinae is not sister to Ochrosperma in our study, in contrast to Rye et al. (2020). We inferred Calytricinae as sister to Alutinae in all analyses. In Rye et al. (2020), Alutinae and Micromyrtinae were sister taxa, in contrast to our study in which both tribes were not recovered as sister in every analysis. These incongruences between nuclear (our study) and plastid + ribosomal ETS topologies from previous studies suggest the presence of non-bifurcating evolution in the early evolutionary history of the tribe, likely attributable to incomplete lineage sorting, hybridisation or a combination of both (Folk et al. 2017; Nge et al. 2021a; McLay et al. 2023). Low or negative QC and low QD values noted for Homoranthus and Darwinia also indicate the presence of reticulation among shallower nodes, supporting previous claims of recent hybridisation between extant species of Chamelaucieae (Briggs 1964; Rye et al. 2013; Rye 2022).
Baeckea sens. lat. is non-monophyletic in our topology, confirming the findings of others based on plastid data (Lam et al. 2002; Rye et al. 2020). Baeckea sens. str. is the only genus missing from the tribal sampling. Previous phylogenies did not resolve the position, e.g. within a large polytomy of subtribes in Rye et al. (2020). All of the orphaned ‘Baeckea’ species belong to Hysterobaeckeinae. Some of these orphaned Baeckea species require evaluation as to whether inclusion in existing sister genera is possible or the description of new genera is required: B. elderiana E.Pritz. (sister to Kardomia Peter G.Wilson), B. muricata C.A.Gardner (sister to Hysterobaeckea on a long branch), B. pentagonantha F.Muell. + B. sp. Dudawa (sister to the Kardomia–B. elderiana clade) and B. sp. London Bridge (sister to Cheyniana + Oxymyrrhine Schauer). Other orphaned Baeckea species are discussed below.
Micromyrtus flava (J.W.Green) Rye & Peter G.Wilson is recovered nested within other species of the genus, supporting the recent recombination from the previous monotypic genus Corynanthera J.W.Green (Rye and Wilson 2022).
Scholtzia Schauer is paraphyletic with respect to Babingtonia Lindl. and several orphaned Baeckea species due to the presence of S. sp. Geraldton amongst the grade of Baeckea orphans leading to Babingtonia. A number of orphaned Baeckea species (B. robusta F.Muell., B. staminosa E.Pritz., B. subcuneata F.Muell., B. sp. Walkaway and B. sp. Walyahmoning) are phylogenetically placed in the subtribe Hysterobaeckeinae (rather than Baeckeinae) in the Scholtzia clade, with Babingtonia apparently nested within this, and require further investigation to establish whether the latter two genera require recircumscription or whether additional genera should be erected for the atypical species. Baeckea staminosa in particular may be best placed in Scholtzia as this was resolved sister to two core Scholtzia samples, and has 2-lobed anthers (a common state in Scholtzia) and 2 collateral ovules per loculus, (1 or 2 superposed ovules per loculus in Scholtzia) in comparison with 4 or more ovules per loculus in other members of the Hysterobaeckeinae.
Malleostemon is monophyletic with the removal of two species that are morphologically atypical. Malleostemon sp. Adelong falls sister to Kardomia + Baeckea elderiana, requiring further assessment of generic limits in this clade, potentially requiring a new genus. Malleostemon sp. Officer Basin falls within Hysterobaeckea, consistent with its morphology (Rye 2016) and the species should be described in this genus (B. L. Rye, in prep.).
The genera Darwinia, Homoranthus and Verticordia were also recovered as non-monophyletic in our study, and this is supported by densely sampled ribosomal and plastid data (M. D. Barrett, unpubl. data). In particular, the three Western Australian Darwinia species (D. diosmoides (DC.) Benth., D. meeboldii C.A.Gardner and D. speciosa (Meisn.) Benth.) were paraphyletic with Actinodium Schauer nested within, and remote from the eastern Australian species (D. fascicularis Rudge and D. micropetala (F.Muell.) Benth.) that grouped with Homoranthus, a relationship previously noted by Lam et al. (2002). The core of Homoranthus is monophyletic but the genus is rendered polyphyletic by the somewhat divergent position of the aberrant H. homoranthoides (F.Muell.) Craven & S.R.Jones that has previously been placed in a monotypic genus Schuermannia F.Muell. (Mueller 1853). Our study is the first to include multiple Verticordia species and the genus is resolved as grossly polyphyletic with the genera Actinodium, Chamelaucium, Darwinia, Homoranthus and Pileanthus Labill. nested within Verticordia. Detailed taxonomic revision and new circumscriptions for subtribe Chamelauciinae are in progress and will be published in due course.
The polymorphic genus Rinzia was comprehensively sampled for this study with seven species representing all five sections, including type species of four of the sections (R. carnosa (S.Moore) Trudgen, type of sect. Mesostemon Rye; R. fumana Schauer, type of the autonymic sect. Rinzia; R. longifolia Turcz., type of sect. Discolora Rye; and R. polystemonea, type of sect. Polyandra Rye). We were unable to sample R. orientalis Rye, the type of sect. Semasperma Rye (Rye 2017) but the section is represented by two other species, R. ericaea (F.Muell. ex Benth.) Rye and R. icosandra (F.Muell. ex Benth.) Rye. Despite the morphological diversity within Rinzia, the genus is confirmed as monophyletic, an important conclusion given previous suggestions that more than one genus should be recognised (Rye 2017). However, a uniting morphological apomorphy for Rinzia is not known and the genus is defined within subtribe Rinziinae by exclusion from the more uniform Euryomyrtus and Enekbatus.
The widespread, variable genus Hysterobaeckea is resolved as monophyletic, with the inclusion of Malleostemon sp. Officer Basin in that genus (B. L. Rye, in prep.). The most-divergent species in the genus is Baeckea aff. behrii, restricted to a small patch of granite on Mount Ziel in central Australia, the highest peak west of the Great Dividing Range and disjunct from the remainder of Hysterobaeckea to the south. This disjunction could possibly have resulted from an early vicariance event as central Australia dried, prior to the genus Hysterobaeckea reinvading the arid zone from the south. Hysterobaeckea is an unusual example of a primarily arid zone genus, occurring from south-eastern Australia across the north of the Nullarbor Plain and in arid margins of south-western Australia.
Species with 1-locular, indehiscent fruits, i.e. nuts, with a terminal style (the old tribe Chamelaucieae sensu Johnson and Briggs 1984, i.e. the clade comprising subtribes Alutinae, Calytricinae, Chamelauciinae, Micromyrtinae and Thryptomeninae here) are resolved as monophyletic with molecular data for the first time. Atypical nuts with the style inserted in a depression occur in Malleostemon (all species), Scholtzia (in 2 species only, most species having a multi-locular, indehiscent fruit), 1–2 species of Astartea DC., Hysterobaeckea and Babingtonia (the 3 latter genera usually with a multi-locular dehiscent fruit, i.e. a capsule). In all these taxa the presence of styles inserted in pits at the nut apex hints at a more recent ancestry from capsules.
All subtribes are supported by morphological characters, as provided below for the new subtribe Triplarininae and more narrowly circumscribed subtribe Rinziinae, and as previously provided (Rye et al. 2020) for the other 10 subtribes.
Cheyniana, Oxymyrrhine and Baeckea sp. London Bridge all have very reduced ±globular anthers, lacking an enlarged connective gland, in agreement with the positioning within a single clade. Baeckea sp. London Bridge may require recognition in a distinct genus as this orphaned species has unusual dark reddish flowers that may help support such a conclusion.
Bird pollination arose independently at least five times within Chamelaucieae (Keighery 1982; Brundrett et al. 2024), with a radiation of bird-pollinated bell inflorescences (at least 35 species in Darwinia; subtribe Chamelauciinae), best known for the Mountain Bells in the Stirling Range of south-west Western Australia. The stem divergence of the Mountain Bell clade (represented by Darwinia meeboldii in our study) from Actinodium occurred in the Miocene c. 13 Ma (95% CI: 8.9–18.6 Ma) but dozens of putatively bird-pollinated Darwinia species were not sampled, preventing reconstruction of bird pollinating syndromes in this genus. Other presumably bird-pollinated species occur sporadically in Verticordia sensu lato (13 species suggested to be bird-pollinated by Brundrett et al. 2024, e.g. the sampled V. humilis Benth. and several other species not sampled e.g. V. grandis J.Drumm.) and Chamelaucium (at least four unsampled species; Brundrett et al. 2024). In other subtribes of Chamelaucieae, only two independent origins of bird-pollinated lineages are noted in the subtribe Hysterobaeckeinae, with only one extant species per lineage – Balaustion pulcherrimum Hook. and Cheyniana microphylla (C.A.Gardner) Rye (Rye 2009). In view of the striking radiation of the Mountain Bells, the lack of radiations in some other bird-pollinated Chamelaucieae is puzzling and warrants further investigation.
Widespread ancestral area and continental vicariance
The ancestral area for Chamelaucieae is unresolved in our analyses, with equal probabilities of different area combinations. Most of these (80%+) have more than one region, suggesting that the ancestral area for the tribe was widespread across Australia historically, thus supporting the Peripheral Vicariance Hypothesis (PVH; H1). Early divergences within the tribe were vicariant events across the northern, eastern and south-western parts of Australia: Ochrosperma (regions E/N), Stenostegia A.R.Bean (N) and the remaining Chamelaucieae (W as ancestral). Our results therefore support an emerging pattern of peripheral vicariance recovered from multiple Australian mesic plant groups (Nge et al. 2022), suggesting that PVH is a dominant biogeographic signal in the Australian flora. Suggestions or explicit recovery of this pattern have been found for Nicotiana L. (Ladiges et al. 2012), Boronia Sm. (Duretto and Ladiges 1998) and Rhamnaceae (Ladiges et al. 2005; Nge et al. 2021b, 2023).
Interestingly, except for the ancestral node of Chamelaucieae, all nodes in the backbone had south-west Western Australia (W) as ancestral. Other biogeographic studies of Australian plants have also shown W to be ancestral (Mast and Givnish 2002; Jabaily et al. 2014; Lamont et al. 2016; Nauheimer et al. 2018). However, Ladiges et al. (2012) and McLay et al. (2016) cautioned that these findings may be artefactual and this was supported by biogeographic sensitivity tests of Nge et al. (2022). W was estimated to be ancestral for Calytrix (Myrtaceae) when default biogeographic models were applied in BioGeoBEARS, without the addition of dispersal distance parameters and time stratification but when these were added the ancestral area was widespread (Nge et al. 2022). However, our study has W as ancestral for most Chamelaucieae clades even with the addition of distance and time stratified parameters in the models. A large proportion of the tribe could well have originated in W, congruent with an earlier presence and subsequent dominance of sclerophyllous elements replacing rainforest in that region since the Eocene (Hill 1998, 2017; Hill and Brodribb 2001; Crisp and Cook 2013). Alternatively, severe extinction of Chamelaucieae lineages in other parts of Australia may have erased vicariant signals for this group (Sanmartín and Meseguer 2016). Indeed, our paleoenvironmental diversification results indicate that there have likely been significant extinctions (or at the very least, a decrease in speciation) in Chamelaucieae during the Eocene–Oligocene. Testing for the effects of extinction as a parameter in biogeographic models is worth further investigation, e.g. with the application of PhyBears (N. J. Matzke, unpubl. data).
We found that most clades in Chamelaucieae diversified within the respective geographic regions (within-area speciation), with few dispersal events between regions. This has also been shown from previous studies on Calytrix (Nge et al. 2022), Cryptandra (Nge et al. 2023), Pomaderris (Nge et al. 2021b), Banksia L.f. (Cardillo and Pratt 2013) and Goodeniaceae (Jabaily et al. 2014). Clades that correspond to different geographic regions of Australia with little biogeographic exchange is a common pattern across the Australian flora – a pattern that can even be seen from phylogenetic studies without an explicit biogeographic analytical component (e.g. de Lange et al. 2010; Clowes et al. 2022; Duretto et al. 2023; Orel et al. 2023; Wilson and Heslewood 2023). Vicariance in southern Australia has been attributed to the uplift of the calcareous Nullarbor Plain in the Miocene (14 Ma; Crisp and Cook 2007). This edaphic barrier limits dispersal between the mesic regions of southern Australia, resulting in more within-area speciation rather than dispersal events across the barrier. However, the Nullarbor uplift is clearly not the only driver for these geographic patterns, as vicariances were not restricted to the Miocene. For example, we infer a divergence between eastern Australian Homoranthus and south-west Western Australia Verticordia sens. str. at c. 22.8 Ma (95% CI: 16.4–32.3 Ma), preceding the uplift of the Nullarbor at 14 Ma. We believe that peripheral vicariance, argued above and in previous studies, is a more convincing explanation.
Asymmetrical dispersal into arid Australia and speciation decline
Chamelaucieae has a surprisingly high proportion of dispersals amongst all biogeographic events (47%; Table 4). In a separate study of Calytrix within the tribe, dispersals accounted for only 21% of events (Nge et al. 2022), as was found in a global study of Solanaceae (20% in Dupin et al. 2017). The high proportion of dispersals in Chamelaucieae is largely driven by range expansions rather than founder events, mainly from W to the adjacent T (Table 5). We show that transitions from W to the semi-arid (T) and arid (A) interior are common in Chamelaucieae, supporting H2. Similar patterns have also been noted in Hakea Schrad. & J.C.Wendl. (Cardillo et al. 2017), Triodia R.Br. (Toon et al. 2015), Lomandra Labill. (Gunn et al. 2020, 2024), Schoenus L. (Elliott et al. 2021), Goodeniaceae (Jabaily et al. 2014) and Cryptandra (Nge et al. 2023) but not for Pomaderris (Nge et al. 2021b). There are three likely reasons for the dispersal bias. Firstly, many lineages in the mediterranean W are dry adapted and may therefore be pre-adapted to colonise the drier habitats of T and A (Cardillo et al. 2017), whereas many lineages in eastern Australia inhabit wetter temperate and subtropical areas. However, few transitions from mediterranean southern South Australia (as part of eastern Australia) into the arid zone have been recovered. Hopper (1979) suggested that the transition zone of W is a fertile area for recent allopatric speciation due to greater fragmentation of habitats and populations from past climatic change than in the arid zone and wetter areas of W. Secondly, high species and lineage diversity in W may simply mean that the region has more potential colonising lineages to disperse into adjacent arid regions than regions with more depauperate floras. Thirdly, West Wind Drift may bias dispersals from west to east in continental Australia and the wider Southern Hemisphere (Sanmartín et al. 2007; Nauheimer et al. 2018). Dispersal towards the east is also evident in our phylogenetic placement of Sannantha Peter G.Wilson, with the New Caledonian endemic (S. virgata) nested in an eastern Australian clade.
Our study provides counterevidence to the hypothesis of Australian arid flora being primarily derived from dispersal of coastal floras that were pre-adapted to xeric conditions (Burbidge 1960; Schodde 1989; Crisp et al. 1999; Ladiges et al. 2011). Although this may be true for some taxa, as hypothesised for Amaranthaceae and Chenopodiaceae (Kadereit et al. 2005; Cabrera et al. 2011), we show that arid zone lineages in Chamelaucieae are mainly derived from adjacent regions, in Hysterobaeckeinae, Chamelauciinae, Rinziinae sens. str. and Calytricinae (Nge et al. 2022) but appear to be absent in Astarteinae. Species in Hypocalymma (Endl.) Endl. and Astartea (Astarteinae) are largely restricted to the mesic fringes of south-west Western Australia, where most inhabit swampy habitats (Rye 2013; Rye et al. 2013; Keighery et al. 2023). Another genus from this subtribe, Cyathostemon Turcz., does reach inland well into T and almost reaches to the border with A. This subtribe is likely limited by the requirement for wetter niches, either from transitions of more dry-adapted ancestors similar to most other present-day Chamelaucieae or persistence in a wetter niche from the past.
Despite frequent transitions from south-west Western Australia to more arid areas, these did not often result in radiations in the arid zone. Only one clade (Hysterobaeckea) shows convincing evidence of an arid (semi-arid) radiation in the Miocene (c. 20 Ma). We acknowledge that more extensive sampling may be required to further test this pattern for other potential clades, e.g. in Rinziinae and Pileanthus–Chamelaucium–Verticordia subg. Eperephes A.S.George. However, this is an emerging pattern in multiple sclerophyllous mesic Australian plant groups (Cardillo and Pratt 2013; Cardillo et al. 2017; Elliott et al. 2021; Habib et al. 2022; Nge et al. 2022; Gunn et al. 2024). The previous dominant narrative of sclerophyllous plant radiations coinciding with aridification episodes in the Miocene (Crisp et al. 2004; Crayn et al. 2006) should be revisited. As a result of denser species-level sampling, we know that multiple transitions from mesic regions into the arid zone have occurred for plants in Australia but these lineages often fail to radiate, with only a few examples of arid radiations having been documented (Kadereit et al. 2005; Cabrera et al. 2011; Dodsworth et al. 2020; Renner et al. 2020; Čalasan et al. 2022). We also show a declining trend of speciation with increasing aridification towards the present, similar to Cryptandra (Nge et al. 2023), Acacia Mill. (Renner et al. 2020) and a wide range of other sclerophyllous flora in Australia (F. J. Nge, unpubl. data). Even arid elements of the Australian biota (Hancock et al. 2018; Hammer et al. 2021; Tiatragul et al. 2023) show decreases in speciation towards the present, suggesting that the consequences for speciation during intensifying Pliocene and Pleistocene aridification (Martin 2006; Byrne et al. 2008; Byrne and Murphy 2020) have been severe. We detect an increase in speciation for Chamelaucieae during the relatively mild MMCO (15–17 Ma), followed by further speciation decline, accelerated during the Pliocene and coinciding with increasing aridification during that time (Martin 2006; Fujioka et al. 2005, 2009). Whether synchronous radiations of sclerophyllous elements in the Miocene across Australia are a response to increased seasonality, warming in the MMCO, gradual aridification or other factors, these warrant further testing using diversification models. However, we also note that the strongly asymmetrical sampling of the Chamelaucieae, weighted toward geographic representation and deeper divergences (genus or subtribal level), will strongly under-represent recent species-level divergence events in this study. A more complete species-level phylogeny may significantly alter conclusions about the effect of increasing aridity for Chamelaucieae.
Our study did not focus explicitly on biome evolution of Chamelaucieae, as incorporating the evolution of specific biomes into biogeographic models (e.g. Landis et al. 2021) requires additional simulations and information beyond the scope of this study. The initiation of the formation of Mediterranean climates in temperate Australia is unclear, although Beard (1977); Lamont and He (2017) suggested that Mediterranean climates first appeared in north-western Australia during the Oligocene (30 Ma) and subsequently moved southwards as the continent drifted north. The precise timing of the commencement of Australia’s northern monsoonal climate has also been contentious (Bowman et al. 2010).
Many clades in Chamelaucieae were already widespread prior to the likely initiation of mediterranean, monsoonal and arid biomes in Australia. Hence surmising that lineages would have transitioned and adapted to these newer biomes from older ones is reasonable. Present-day biomes largely correspond to the geographic regions in our study, with some minor differences. The mesic Murray Basin region of South Australia (SA) and parts of western Victoria (Vic.) have a mediterranean climate similar to south-west Western Australia. Despite this, southern Australia (SA + Vic.) is relatively depauperate in Chamelaucieae, with only a few species, e.g. Homoranthus homoranthoides, Rinzia ericaea, Micromyrtus ciliata (Sm.) Druce, Thryptomene micrantha J.D.Hook. and T. ericaea F.Muell present in the region. One of the two south-eastern taxa sequenced in our study (Homoranthus homoranthoides) shared affinities with other eastern Australian taxa that occupy non-Mediterranean biomes whereas Rinzia ericaea belonged in a clade with western species. Hence, some of the biogeographic connections of South Australian Mediterranean lineages are driven by geographic proximity with other mesic regions instead of biome fidelity with south-west Western Australia, a similar pattern found in other plant groups (Nge et al. 2021b, 2022, 2023; Gunn et al. 2024). Micromyrtus delicata A.R.Bean, found in the Wet Tropics region of Queensland, did not make a biome transition into rainforests, as this species is found in xeric open woodlands on sparse volcanic substrates (Bean 1997). In general, many species in Chamelaucieae are confined to skeletal oligotrophic substrates across a wide range of biomes. These include outlying taxa found in New Caledonia (Sannantha virgata) and Asia (species of Seorsus Rye & Trudgen; Rye and Trudgen 2008; Wilson and Heslewood 2014). Incorporation of biotic traits with biome evolution modelling (Bouchenak-Khelladi et al. 2015; Onstein et al. 2015; Onstein and Linder 2016; Landis et al. 2021) is a promising future avenue to test for interactions between these factors in the evolution of Chamelaucieae.
Eocene–Oligocene pulse event and lineage persistence in south-west Western Australia
We show that the crown radiation of Chamelaucieae pre-dates the E-O boundary and that there is a significant decline in speciation rate after the initial crown radiation until shortly after the E-O boundary pulse event, supporting H3 (Nge et al. 2020). Paleoenvironmental diversification models show that diversification of Chamelaucieae has been most affected by temperature changes, as opposed to other factors (e.g. time, sea-level). The tribe diversified more rapidly during a historically warmer climate in the Eocene and declined as the climate cooled towards the present. The rapid declining trend between the Eocene and the E-O boundary may be due to the effects of extinction during the E-O boundary pulse event pushing back age estimates of node divergence (Crisp and Cook 2009) or this may represent actual decline during that period. Untangling these two explanations will require further investigation. Our study differs from the findings of Nge et al. (2020) that showed an increase in diversification for Chamelaucieae during the E-O boundary. We believe that our current study, with more comprehensive sampling and a better-resolved dated phylogeny, offers a more accurate estimate of diversification in the tribe. Therefore we show that Chamelaucieae exhibits a similar diversification decline at the E-O boundary to other Australian plant groups (Nge et al. 2020). Crayn et al. (2006) showed that crown radiation of the temperate clade of Elaeocarpaceae (formerly Tremandraceae) occurred immediately after the E-O boundary, as has been documented for several Australian animal groups (Schweizer et al. 2011; Almeida et al. 2012; Kayaalp et al. 2013; Brennan and Oliver 2017; Marki et al. 2019). These studies reinforce the importance of the E-O boundary extinction pulse event as a significant driver for the evolution and diversification of our biota.
Our BAMM analyses detected only one diversification rate shift in south-west Western Australia, despite most extant species and lineages occurring in that region (supporting H4). This finding, along with our non-significant GeoHiSSE results, strongly indicates that high species diversity in south-west Western Australia is best attributed to more time for species accumulation rather than elevated diversification rates. The lack of diversification rate shifts is common in the south-west Western Australian flora (F. J. Nge, unpubl. data), although additional sampling of species-rich subtribes for Chamelaucieae may very likely reveal other rate shifts not detected in this study (Cusimano and Renner 2010). Diversification rate shifts in Australian plants have mostly been linked to trait adaptations to oligotrophic environments (Jordan et al. 2008; Carpenter et al. 2014; Onstein et al. 2016; Hayes et al. 2021), aridity (Renner et al. 2020, 2021; Hua et al. 2022) or fire (Crisp et al. 2011; Thornhill et al. 2019; Lamont et al. 2020) rather than being strictly geographically dependent.
Our dated phylogeny of Chamelaucieae also reveals several long-persisting lineages (isolated, long-branched, species-poor lineages; Nge et al. 2023). These include Ericomyrtus serpyllifolia (Turcz.) Rye (30.5 Ma; 95% CI: 23.0–45.1 Ma; three species of Ericomyrtus Turcz. not sampled), Aluta maisonneuvei (F.Muell.) Rye & Trudgen (33.0 Ma; 95% CI: 23.4–46.3 Ma; four other species of Aluta Rye & Trudgen not sampled) and Stenostegia congesta A.R.Bean (40.5 Ma, 95% CI: 29.6–57.8 Ma; monotypic, apparently ‘relictual’ and highly localised), although we note that, other than the monotypic Stenostegia A.R.Bean, the divergence age of these lineages may change significantly with additional ingroup sampling. Long-persisting lineages have also been detected in south-west Western Australian Cryptandra (Nge et al. 2023), Calytrix (Nge et al. 2022), Tremandra R.Br. ex DC. (Crayn et al. 2006), Franklandia R.Br. (Sauquet et al. 2009) and others (Hopper and Gioia 2004), and have been linked to the tectonic and climatic stability of the region (OCBILs; Hopper 2009; Hopper et al. 2021).
Conclusion
The phylogenomic approach using Angiosperms353 has allowed us to better resolve subtribal relationships across the spectacular Chamelaucieae tribe, resulting in an updated classification and description of an additional subtribe (total of 12 subtribes including Triplarininae). Despite these advances, the phylogenetic placements of Stenostegiinae, Astarteinae and Micromyrtinae remain equivocal and resolution of these relationships should be a focus of future research. Biogeographically, Chamelaucieae adds to the growing literature highlighting the importance of PVH, and E-O boundary pulse event in the diversification and evolution of the Australian flora. Additional taxonomic sampling at the species level should yield additional insights into more recent diversification dynamics for this tribe and the wider Australian flora more broadly.
Taxonomy
Following strong support for the non-monophyly of Rinziinae, the alternate solution of removing Astus and Triplarina, and recognising these in a separate tribe (foreshadowed by Rye et al. 2020) is enacted here. The following key can be appended as an additional step to the key to subtribes in Rye et al. (2020), with the lead to Rinziinae at step 10(a) leading to ‘11’ below instead. Numbering of subtribes in the definition below follows that of Rye et al. (2020) with the addition of a twelfth tribe Triplarininae.
11. | Hypanthium tending to be pentagonal in TS. Stamens all antisepalous or (in 2 species) with one stamen opposite each sepal and petal. Fruit 2- or 3-valvate; seeds 0.5–1.6 mm long, without an aril, also lacking a large flat hilum (when the fruit is 3-valvate the seeds are only 0.5–0.8 mm long)...12. Triplarininae (see definition below) |
11: | Hypanthium circular in TS. Stamens all antipetalous or opposite both the sepals and petals. Fruit either 3-valvate or (in Enekbatus) indehiscent; seeds 1–2.3 mm long, arillate or with a large flat hilum in most species (except when the fruit is indehiscent)...9. Rinziinae (see redefinition below) |
9. Myrtaceae tribe Chamelaucieae subtribe Rinziinae
Myrtaceae tribe Chamelaucieae subtribe Rinziinae Rye & Peter G.Wilson, Australian Systematic Botany 33: 203 (2020).
Type: Rinzia Schauer
Mostly prostrate or small shrubs to 1 m, rarely to 2.5 m tall, glabrous, sometimes with adventitious roots. Leaves opposite, decussate, small, usually shortly petiolate. Peduncles 1- or 2-flowered. Bracteoles usually persistent. Pedicels absent to distinctly longer than peduncles. Flowers 5-merous; hypanthium circular in TS. Sepals much shorter than petals. Petals white or pink. Staminodes usually absent, rarely numerous. Stamens 3–30, all antipetalous or opposite both the petals and sepals. Anthers dorsifixed, versatile or attached on front of broad filament; thecae parallel, longitudinally dehiscent; connective gland free. Ovary 2–5-locular; placentas ±sessile; ovules 2–10(–12) per loculus. Style base deeply inset in depression. Fruits inferior to largely superior, indehiscent (in Enekbatus) or dehiscent by 3 valves. Seeds 1–2.3 mm long, reniform or rarely obovoid, arillate or with a large flat hilum in most species (except when in an indehiscent fruit); testa crustaceous, usually thick (thin when fruit is indehiscent). x = 11.
Subtribe Rinziinae has 3 genera and 44 species, with most occurring in south-western Australia. This subtribe also occurs in south-eastern Australia, including Tasmania and has a single representative in the central mainland.
1. | Flowers ±sessile. Ovary 2–5-locular; ovules usually 2 per loculus, collateral or superposed. Fruits indehiscent...Enekbatus Flowers stalked. Ovary 3-locular; ovules 2–12 per loculus, collateral or in 2 rows. Fruits dehiscent. |
2. | Leaves with parallel venation usually visible on upper surface. Peduncles 0.5–9 mm long, usually about as long as to distinctly longer than the pedicels. Antipetalous colleters forming prominent groups (absent in one species)...Euryomyrtus Leaf venation obscure or not as above. Peduncles 0–0.6(–1) mm long, usually much shorter than the pedicels. Antipetalous colleters inconspicuous or absent...Rinzia |
12. Myrtaceae tribe Chamelaucieae subtribe Triplarininae
Myrtaceae tribe Chamelaucieae subtribe Triplarininae Rye & M.D.Barrett, subtr. nov.
Type: Triplarina Raf.
Small to tall shrubs (up to 3.5 m high), glabrous. Leaves opposite, decussate, small, shortly petiolate. Peduncles solitary in the axils, 1–4-flowered; secondary axes (when present) often long. Bracteoles shed early in most species. Pedicels almost absent to much longer than peduncles. Flowers 5-merous; hypanthium tending to be pentagonal in TS. Sepals much shorter than to slightly exceeding petals. Petals white or rarely pink. Staminodes few or absent. Stamens 8–20, apparently in a single series, all antisepalous in most species (rarely with one stamen opposite each sepal and petal). Filaments free, narrow. Anthers dorsifixed, versatile; thecae parallel, longitudinally dehiscent; connective gland free, dorsal-subterminal. Ovary inferior, 3-locular; placentas ±sessile; ovules 2–14 per loculus. Style base deeply inset in depression. Fruits half- or largely inferior, 3-valvate or heterocarpidic, the latter kind with two valvate loculi and a single indehiscent loculus borne at a lower level. Seeds reniform, 0.5–1.6 mm long, without an aril or large hilum; testa crustaceous, tuberculate or colliculate. x = unknown.
Triplarininae is distinguished by the following combination of characters: hypanthium tending to be pentagonal in TS; stamens 8–20, apparently in a single series, all antisepalous in most species, free; anthers dorsifixed, versatile, with parallel, longitudinally dehiscent thecae; connective gland free, dorsal-subterminal; fruits 3-valvate or heterocarpidic; seeds reniform, without an aril, also lacking a large hilum, with a tuberculate or colliculate testa.
This new subtribe comprises 2 genera and 11 species. Triplariniae is endemic to mainland Australia and has a disjunct distribution, with the larger genus (Triplarina) occurring along the east coast and the smaller genus (Astus) occurring in the south-west.
1. | Stamens 11–20. Fruits 3-valvate, with all loculi at the same level. Seeds 0.5–0.8 mm long. Occurring in eastern Australia...Triplarina Stamens 8–12. Fruits 2-valvate, with loculi at two overlapping levels, the lower level with an indehiscent loculus and upper level with 2 dehiscent loculi. Seeds 1–1.6 mm long. Occurring in south-western Australia...Astus |
Data availability
Sequence data are available in the Bioplatforms Australia web portal and associated sequence ID are listed in Table 1 for each newly sequenced sample.
Conflicts of interest
Peter Wilson, Kevin Thiele and Michelle Waycott are Associate Editors for Australian Systematic Botany but did not at any stage have editor-level access to this manuscript while in peer review, as is the standard practice when handling manuscripts submitted by an editor to this journal. Australian Systematic Botany encourages its editors to publish in the journal and they are kept totally separate from the decision-making processes for their manuscripts. The authors have no further conflicts of interest to declare.
Declaration of funding
This study was funded by Bioplatforms Australia through the Australian Government National Collaborative Research Infrastructure Strategy (NCRIS), with additional contributions from the Botanic Gardens and Herbaria.
Acknowledgements
We acknowledge the contribution of the Genomics for Australian Plants Framework Initiative consortium (see https://www.genomicsforaustralianplants.com/consortium/) in the generation of data used in this publication. The initiative is supported by funding from Bioplatforms Australia (enabled by NCRIS), the Ian Potter Foundation, Royal Botanic Gardens Foundation (Vic.), Royal Botanic Gardens Victoria, the Royal Botanic Gardens and Domain Trust, the Council of Heads of Australasian Herbaria, CSIRO, Centre for Australian National Biodiversity Research and the Department of Biodiversity, Conservation and Attractions, Western Australia. In particular, we thank Mabel Lum for technical support. We acknowledge the Plant and Fungal Trees of Life (PAFTOL) project at the Royal Botanic Gardens Kew that has enabled the sharing of and public access to sequence data. We thank the handling editor Dan Murphy, the nomenclature editor Anna Monro and two anonymous reviewers for feedback that helped improve the manuscript.
References
Abadi S, Azouri D, Pupko T, Mayrose I (2019) Model selection may not be a mandatory step for phylogeny reconstruction. Nature Communications 10, 934.
| Crossref | Google Scholar | PubMed |
Akaike H (1974) A new look at the statistical model identification. IEEE Transactions on Automatic Control 19, 716-723.
| Crossref | Google Scholar |
Almeida EAB, Pie MR, Brady SG, Danforth BN (2012) Biogeography and diversification of colletid bees (Hymenoptera: Colletidae): emerging patterns from the southern end of the world. Journal of Biogeography 39, 526-544.
| Crossref | Google Scholar |
Anisimova M, Gil M, Dufayard J-F, Dessimoz C, Gascuel O (2011) Survey of branch support methods demonstrates accuracy, power, and robustness of fast likelihood-based approximation schemes. Systematic Biology 60, 685-699.
| Crossref | Google Scholar | PubMed |
Antonelli A, Clarkson JJ, Kainulainen K, Maurin O, Brewer GE, Davis AP, Epitawalage N, Goyder DJ, Livshultz T, Persson C (2021) Settling a family feud: a high-level phylogenomic framework for the Gentianales based on 353 nuclear genes and partial plastomes. American Journal of Botany 108, 1143-1165.
| Crossref | Google Scholar | PubMed |
Baker WJ, Bailey P, Barber V, Barker A, Bellot S, Bishop D, Botigué LR, Brewer G, Carruthers T, Clarkson JJ, Cook J, Cowan RS, Dodsworth S, Epitawalage N, Françoso E, Gallego B, Johnson MG, Kim JT, Leempoel K, Maurin O, Mcginnie C, Pokorny L, Roy S, Stone M, Toledo E, Wickett NJ, Zuntini AR, Eiserhardt WL, Kersey PJ, Leitch IJ, Forest F (2021) A comprehensive phylogenomic platform for exploring the angiosperm Tree of Life. Systematic Biology 71, 301-319.
| Crossref | Google Scholar |
Bankevich A, Nurk S, Antipov D, Gurevich AA, Dvorkin M, Kulikov AS, Lesin VM, Nikolenko SI, Pham S, Prjibelski AD (2012) SPAdes: a new genome assembly algorithm and its applications to single-cell sequencing. Journal of Computational Biology 19, 455-477.
| Crossref | Google Scholar | PubMed |
Barker NP, Weston PH, Rutschmann F, Sauquet H (2007) Molecular dating of the ‘Gondwanan’ plant family Proteaceae is only partially congruent with the timing of the break-up of Gondwana. Journal of Biogeography 34, 2012-2027.
| Crossref | Google Scholar |
Bean A (1997) A revision of Micromyrtus Benth.(Myrtaceae) in Queensland. Austrobaileya 4, 455-476.
| Crossref | Google Scholar |
Beard JS (1977) Tertiary evolution of the Australian flora in the light of latitudinal movements of the continent. Journal of Biogeography 4, 111-118.
| Crossref | Google Scholar |
Birch JL, Keeley SC (2013) Dispersal pathways across the Pacific: the historical biogeography of Astelia s.l. (Asteliaceae, Asparagales). Journal of Biogeography 40, 1914-1927.
| Crossref | Google Scholar |
Bouchenak-Khelladi Y, Onstein RE, Xing Y, Schwery O, Linder HP (2015) On the complexity of triggering evolutionary radiations. New Phytologist 207, 313-326.
| Crossref | Google Scholar | PubMed |
Bouckaert R, Heled J, Kühnert D, Vaughan T, Wu C-H, Xie D, Suchard MA, Rambaut A, Drummond AJ (2014) BEAST 2: a software platform for Bayesian evolutionary analysis. PLoS Computational Biology 10, e1003537.
| Crossref | Google Scholar | PubMed |
Bowman DMJS, Brown GK, Braby MF, Brown JR, Cook LG, Crisp MD, Ford F, Haberle S, Hughes J, Isagi Y (2010) Biogeography of the Australian monsoon tropics. Journal of Biogeography 37, 201-216.
| Crossref | Google Scholar |
Brennan IG, Oliver PM (2017) Mass turnover and recovery dynamics of a diverse Australian continental radiation. Evolution 71, 1352-1365.
| Crossref | Google Scholar | PubMed |
Briggs BG (1964) The control of interspecific hybridization in Darwinia. Evolution 18, 292-303.
| Crossref | Google Scholar |
Briggs BG, Johnson LAS (1979) Evolution in Myrtaceae: evidence from inflorescence structure. Proceedings of the Linnean Society of New South Wales 102, 157-256.
| Google Scholar |
Brundrett MC, Ladd PG, Keighery GJ (2024) Pollination strategies are exceptionally complex in southwestern Australia – a globally significant ancient biodiversity hotspot. Australian Journal of Botany 72, BT23007.
| Crossref | Google Scholar |
Burbidge N (1960) The phytogeography of the Australian region. Australian Journal of Botany 8, 75-211.
| Crossref | Google Scholar |
Byrne M (2008) Evidence for multiple refugia at different time scales during Pleistocene climatic oscillations in southern Australia inferred from phylogeography. Quaternary Science Reviews 27, 2576-2585.
| Crossref | Google Scholar |
Byrne M, Murphy DJ (2020) The origins and evolutionary history of xerophytic vegetation in Australia. Australian Journal of Botany 68, 195-207.
| Crossref | Google Scholar |
Byrne M, Yeates DK, Joseph L, Kearney M, Bowler J, Williams MAJ, Cooper S, Donnellan SC, Keogh JS, Leys R, Melville J, Murphy DJ, Porch N, Wyrwoll KH (2008) Birth of a biome: insights into the assembly and maintenance of the Australian arid zone biota. Molecular Ecology 17, 4398-4417.
| Crossref | Google Scholar | PubMed |
Cabrera J, Jacobs SWL, Kadereit G (2011) Biogeography of Camphorosmeae (Chenopodiaceae): tracking the Tertiary history of Australian aridification. Telopea 13, 313-326.
| Crossref | Google Scholar |
Caetano DS, O’Meara BC, Beaulieu JM (2018) Hidden state models improve state-dependent diversification approaches, including biogeographical models. Evolution 72, 2308-2324.
| Crossref | Google Scholar | PubMed |
Čalasan AŽ, Hammen S, Sukhorukov AP, McDonald JT, Brignone NF, Böhnert T, Kadereit G (2022) From continental Asia into the world: global historical biogeography of the saltbush genus Atriplex (Chenopodieae, Chenopodioideae, Amaranthaceae). Perspectives in Plant Ecology, Evolution and Systematics 54, 125660.
| Crossref | Google Scholar |
Camacho C, Coulouris G, Avagyan V, Ma N, Papadopoulos J, Bealer K, Madden TL (2009) BLAST+: architecture and applications. BMC Bioinformatics 10, 421.
| Crossref | Google Scholar |
Capella-Gutiérrez S, Silla-Martínez JM, Gabaldón T (2009) trimAl: a tool for automated alignment trimming in large-scale phylogenetic analyses. Bioinformatics 25, 1972-1973.
| Crossref | Google Scholar | PubMed |
Cardillo M, Pratt R (2013) Evolution of a hotspot genus: geographic variation in speciation and extinction rates in Banksia (Proteaceae). BMC Evolutionary Biology 13, 155.
| Crossref | Google Scholar | PubMed |
Cardillo M, Weston PH, Reynolds ZK, Olde PM, Mast AR, Lemmon EM, Lemmon AR, Bromham L (2017) The phylogeny and biogeography of Hakea (Proteaceae) reveals the role of biome shifts in a continental plant radiation. Evolution 71, 1928-1943.
| Crossref | Google Scholar | PubMed |
Carpenter RJ, McLoughlin S, Hill RS, McNamara KJ, Jordan GJ (2014) Early evidence of xeromorphy in angiosperms: stomatal encryption in a new Eocene species of Banksia (Proteaceae) from Western Australia. American Journal of Botany 101, 1486-1497.
| Crossref | Google Scholar | PubMed |
Chernomor O, Von Haeseler A, Minh BQ (2016) Terrace aware data structure for phylogenomic inference from supermatrices. Systematic Biology 65, 997-1008.
| Crossref | Google Scholar | PubMed |
Clowes C, Fowler RM, Fahey PS, Kellermann J, Brown GK, Bayly MJ (2022) Big trees of small baskets: phylogeny of the Australian genus Spyridium (Rhamnaceae: Pomaderreae), focusing on biogeographic patterns and species circumscriptions. Australian Systematic Botany 35, 95-119.
| Crossref | Google Scholar |
Condamine FL, Rolland J, Morlon H (2013) Macroevolutionary perspectives to environmental change. Ecology Letters 16, 72-85.
| Crossref | Google Scholar | PubMed |
Cook LG, Hardy NB, Crisp MD (2015) Three explanations for biodiversity hotspots: small range size, geographical overlap and time for species accumulation. An Australian case study. New Phytologist 207, 390-400.
| Crossref | Google Scholar | PubMed |
Cowling RM, Potts AJ, Bradshaw PL, Colville J, Arianoutsou M, Ferrier S, Forest F, Fyllas NM, Hopper SD, Ojeda F (2015) Variation in plant diversity in mediterranean-climate ecosystems: the role of climatic and topographical stability. Journal of Biogeography 42, 552-564.
| Crossref | Google Scholar |
Crayn DM, Rossetto M, Maynard DJ (2006) Molecular phylogeny and dating reveals an Oligo-Miocene radiation of dry-adapted shrubs (former Tremandraceae) from rainforest tree progenitors (Elaeocarpaceae) in Australia. American Journal of Botany 93, 1328-1342.
| Crossref | Google Scholar | PubMed |
Crayn DM, Costion C, Harrington MG (2015) The Sahul–Sunda floristic exchange: dated molecular phylogenies document Cenozoic intercontinental dispersal dynamics. Journal of Biogeography 42, 11-24.
| Crossref | Google Scholar |
Crisp MD, Cook LG (2007) A congruent molecular signature of vicariance across multiple plant lineages. Molecular Phylogenetics and Evolution 43, 1106-1117.
| Crossref | Google Scholar | PubMed |
Crisp MD, Cook LG (2009) Explosive radiation or cryptic mass extinction? Interpreting signatures in molecular phylogenies. Evolution 63, 2257-2265.
| Crossref | Google Scholar | PubMed |
Crisp MD, Cook LG (2013) How was the Australian flora assembled over the last 65 million years? A molecular phylogenetic perspective. Annual Review of Ecology, Evolution, and Systematics 44, 303-324.
| Crossref | Google Scholar |
Crisp MD, West JG, Linder HP (1999) Biogeography of the terrestrial flora. Flora of Australia 1, 321-367.
| Google Scholar |
Crisp MD, Cook L, Steane D (2004) Radiation of the Australian flora: what can comparisons of molecular phylogenies across multiple taxa tell us about the evolution of diversity in present-day communities? Philosophical Transactions of the Royal Society of London B 359, 1551-1571.
| Crossref | Google Scholar | PubMed |
Crisp MD, Burrows GE, Cook LG, Thornhill AH, Bowman DMJS (2011) Flammable biomes dominated by eucalypts originated at the Cretaceous–Palaeogene boundary. Nature Communications 2, 193.
| Crossref | Google Scholar | PubMed |
Crisp MD, Minh BQ, Choi B, Edwards RD, Hereward J, Kulheim C, Lin YP, Meusemann K, Thornhill AH, Toon A (2024) Perianth evolution and implications for generic delimitation in the eucalypts (Myrtaceae), including the description of the new genus, Blakella. Journal of Systematics and Evolution 62, 942-962.
| Crossref | Google Scholar |
Cusimano N, Renner SS (2010) Slowdowns in diversification rates from real phylogenies may not be real. Systematic Biology 59, 458-464.
| Crossref | Google Scholar | PubMed |
Davis RW, Rye BL (2020) Life on the rocks: Darwinia sphaerica (Myrtaceae: Chamelaucieae), a new species currently known from one granite outcrop. Nuytsia 31, 233-237.
| Crossref | Google Scholar |
de Lange PJ, Smissen RD, Wagstaff SJ, Keeling DJ, Murray BG, Toelken HR (2010) A molecular phylogeny and infrageneric classification for Kunzea (Myrtaceae) inferred from rDNA ITS and ETS sequences. Australian Systematic Botany 23, 309-319.
| Crossref | Google Scholar |
Di Franco A, Poujol R, Baurain D, Philippe H (2019) Evaluating the usefulness of alignment filtering methods to reduce the impact of errors on evolutionary inferences. BMC Evolutionary Biology 19, 21.
| Crossref | Google Scholar |
Dodsworth S, Christenhusz MJ, Conran JG, Guignard MS, Knapp S, Struebig M, Leitch AR, Chase MW (2020) Extensive plastid-nuclear discordance in a recent radiation of Nicotiana section Suaveolentes (Solanaceae). Botanical Journal of the Linnean Society 193, 546-559.
| Crossref | Google Scholar |
Dröllner M, Barham M, Kirkland CL, Danišík M, Bourdet J, Schulz M, Aspandiar M (2023) Directly dating Plio-Pleistocene climate change in the terrestrial record. Geophysical Research Letters 50, e2023GL102928.
| Crossref | Google Scholar |
Dupin J, Matzke NJ, Särkinen T, Knapp S, Olmstead RG, Bohs L, Smith SD (2017) Bayesian estimation of the global biogeographical history of the Solanaceae. Journal of Biogeography 44, 887-899.
| Crossref | Google Scholar |
Duretto MF, Ladiges PY (1998) A cladistic analysis of Boronia section Valvatae (Rutaceae). Australian Systematic Botany 11, 636-665.
| Crossref | Google Scholar |
Duretto MF, Heslewood MM, Bayly MJ (2023) A molecular phylogeny of Boronia (Rutaceae): placement of enigmatic taxa and a revised infrageneric classification. Australian Systematic Botany 36, 81-106.
| Crossref | Google Scholar |
Elliott TL, van Mazijk R, Barrett RL, Bruhl JJ, Joly S, Muthaphuli N, Wilson KL, Muasya AM (2021) Global dispersal and diversification of the genus Schoenus (Cyperaceae) from the Western Australian biodiversity hotspot. Journal of Systematics and Evolution 59, 791-808.
| Crossref | Google Scholar |
Folk RA, Mandel JR, Freudenstein JV (2017) Ancestral gene flow and parallel organellar genome capture result in extreme phylogenomic discord in a lineage of angiosperms. Systematic Biology 66, 320-337.
| Crossref | Google Scholar | PubMed |
Fujioka T, Chappell J, Honda M, Yatsevich I, Fifield K, Fabel D (2005) Global cooling initiated stony deserts in central Australia 2–4 Ma, dated by cosmogenic 21Ne-10Be. Geology 33, 993-996.
| Crossref | Google Scholar |
Fujioka T, Chappell J, Fifield LK, Rhodes EJ (2009) Australian desert dune fields initiated with Pliocene–Pleistocene global climatic shift. Geology 37, 51-54.
| Crossref | Google Scholar |
Gadek PA, Wilson PG, Quinn CJ (1996) Phylogenetic reconstruction in Myrtaceae using matK, with particular reference to the position of Psiloxylon and Heteropyxis. Australian Systematic Botany 9, 283-290.
| Crossref | Google Scholar |
Gatesy J, Springer MS (2014) Phylogenetic analysis at deep timescales: unreliable gene trees, bypassed hidden support, and the coalescence/concatalescence conundrum. Molecular Phylogenetics and Evolution 80, 231-266.
| Crossref | Google Scholar | PubMed |
Gemmill CEC, Allan GJ, Wagner WL, Zimmer EA (2002) Evolution of insular Pacific Pittosporum (Pittosporaceae): origin of the Hawaiian radiation. Molecular Phylogenetics and Evolution 22, 31-42.
| Crossref | Google Scholar | PubMed |
González-Orozco CE, Laffan SW, Miller JT (2011) Spatial distribution of species richness and endemism of the genus Acacia in Australia. Australian Journal of Botany 59, 601-609.
| Crossref | Google Scholar |
González-Orozco CE, Thornhill AH, Knerr N, Laffan S, Miller JT (2014) Biogeographical regions and phytogeography of the eucalypts. Diversity and Distributions 20, 46-58.
| Crossref | Google Scholar |
Guindon S, Dufayard J-F, Lefort V, Anisimova M, Hordijk W, Gascuel O (2010) New algorithms and methods to estimate maximum-likelihood phylogenies: assessing the performance of PhyML 3.0. Systematic Biology 59, 307-321.
| Crossref | Google Scholar | PubMed |
Gunn BF, Murphy DJ, Walsh NG, Conran JG, Pires JC, Macfarlane TD, Birch JL (2020) Evolution of Lomandroideae: multiple origins of polyploidy and biome occupancy in Australia. Molecular Phylogenetics and Evolution 149, 106836.
| Crossref | Google Scholar | PubMed |
Gunn BF, Murphy DJ, Walsh NG, Conran JG, Pires JC, Macfarlane TD, Crisp MD, Cook LG, Birch JL (2024) Genomic data resolve phylogenetic relationships of Australian mat-rushes, Lomandra (Asparagaceae: Lomandroideae). Botanical Journal of the Linnean Society 204, 1-22.
| Crossref | Google Scholar |
Habib S, Gong Y, Dong S, Lindstrom A, Stevenson DW, Liu Y, Wu H, Zhang S (2022) Phylotranscriptomics reveal the spatio-temporal distribution and morphological evolution of Macrozamia, an Australian endemic genus of Cycadales. Annals of Botany 130, 671-685.
| Crossref | Google Scholar | PubMed |
Hammer TA, Renton M, Mucina L, Thiele KR (2021) Arid Australia as a source of plant diversity: the origin and climatic evolution of Ptilotus (Amaranthaceae). Australian Systematic Botany 34, 570-586.
| Crossref | Google Scholar |
Hammer TA, Toelken HR, Thiele KR (2022) Hibbertia advena (Dillenijaceae), a new and rare species from Queensland with transcontinental affinities. Australian Journal of Taxonomy 9, 1-5.
| Crossref | Google Scholar |
Hancock LP, Obbens F, Moore AJ, Thiele K, de Vos JM, West J, Holtum JAM, Edwards EJ (2018) Phylogeny, evolution, and biogeographic history of Calandrinia (Montiaceae). American Journal of Botany 105, 1021-1034.
| Crossref | Google Scholar | PubMed |
Hayes PE, Nge FJ, Cramer MD, Finnegan P, Fu P, Hopper SD, Oliveira RS, Turner BL, Zemunik G, Zhong H, Lambers H (2021) Traits related to efficient acquisition and use of phosphorus drive diversification in Proteaceae in phosphorus-impoverished landscapes. Plant and Soil 462, 67-88.
| Crossref | Google Scholar |
Helmstetter AJ, Ezedin Z, Lirio EJ, SM Oliveira, Chatrou LW, Erkens RHJ, Larridon I, Leempoel K, Maurin O, Roy S, Zuntini AR, Baker WJ, Couvreur TLP, Forest F, Sauquet H (2024) Towards a phylogenomic classification of Magnoliidae. bioRxiv 2024, 2024.01.09.574948 [Preprint, published 10 January 2024].
| Crossref | Google Scholar |
Helmstetter AJ, Ezedin Z, Lirio EJ, SM Oliveira, Chatrou LW, Erkens RHJ, Larridon I, Leempoel K, Maurin O, Roy S, Zuntini AR, Baker WJ, Couvreur TLP, Forest F, Sauquet H (2025) Towards a phylogenomic classification of magnoliids. American Journal of Botany 112(1), e16451.
| Crossref | Google Scholar |
Herbert TD (2023) The mid-Pleistocene climate transition. Annual Review of Earth and Planetary Sciences 51, 389-418.
| Crossref | Google Scholar |
Hill RS (1998) Fossil evidence for the onset of xeromorphy and scleromorphy in Australian Proteaceae. Australian Systematic Botany 11, 391-400.
| Crossref | Google Scholar |
Hill RS (2017) ‘History of the Australian vegetation: Cretaceous to Recent.’ (The University of Adelaide Press) 10.20851/australian-vegetation
Hill R, Brodribb T (2001) Macrofossil evidence for the onset of xeromorphy in Australian Casuarinaceae and tribe Banksieae (Proteaceae). Journal of Mediterranean Ecology 2, 127-136.
| Google Scholar |
Hopper SD (1979) Biogeographical aspects of speciation in the southwest Australian flora. Annual Review of Ecology and Systematics 10, 399-422.
| Crossref | Google Scholar |
Hopper SD (2009) OCBIL theory: towards an integrated understanding of the evolution, ecology and conservation of biodiversity on old, climatically buffered, infertile landscapes. Plant and Soil 322, 49-86.
| Crossref | Google Scholar |
Hopper SD, Gioia P (2004) The Southwest Australian Floristic Region: evolution and conservation of a global hot spot of biodiversity. Annual Review of Ecology, Evolution, and Systematics 35, 623-650.
| Crossref | Google Scholar |
Hopper SD, Lambers H, Silveira FA, Fiedler PL (2021) OCBIL theory examined: reassessing evolution, ecology and conservation in the world’s ancient, climatically buffered and infertile landscapes. Biological Journal of the Linnean Society 133, 266-296.
| Crossref | Google Scholar |
Hua X, Cardillo M, Bromham L (2022) Adapting to extremes: reconstructing evolution in response to changing climate over time and space in the diverse Australian plant genus Acacia. Journal of Biogeography 49, 727-738.
| Crossref | Google Scholar |
Hutchinson DK, Coxall HK, Lunt DJ, Steinthorsdottir M, De Boer AM, Baatsen M, von der Heydt A, Huber M, Kennedy-Asser AT, Kunzmann L (2020) The Eocene–Oligocene transition: a review of marine and terrestrial proxy data, models and model–data comparisons. Climate of the Past Discussions 17, 269-315.
| Crossref | Google Scholar |
Jabaily RS, Shepherd KA, Gardner AG, Gustafsson MHG, Howarth DG, Motley TJ (2014) Historical biogeography of the predominantly Australian plant family Goodeniaceae. Journal of Biogeography 41, 2057-2067.
| Crossref | Google Scholar |
Jackson C, McLay T, Schmidt-Lebuhn AN (2023) hybpiper-nf and paragone-nf: containerization and additional options for target capture assembly and paralog resolution. Applications in Plant Sciences 11, e11532.
| Crossref | Google Scholar | PubMed |
Johnson MG, Gardner EM, Liu Y, Medina R, Goffinet B, Shaw AJ, Zerega NJ, Wickett NJ (2016) HybPiper: extracting coding sequence and introns for phylogenetics from high-throughput sequencing reads using target enrichment. Applications in Plant Sciences 4, 1600016.
| Crossref | Google Scholar | PubMed |
Johnson MG, Pokorny L, Dodsworth S, Botigué LR, Cowan RS, Devault A, Eiserhardt WL, Epitawalage N, Forest F, Kim JT, Leebens-Mack JH, Leitch IJ, Maurin O, Soltis DE, Soltis PS, Wong GK, Baker WJ, Wickett NJ (2019) A universal probe set for targeted sequencing of 353 nuclear genes from any flowering plant designed using k-medoids clustering. Systematic Biology 68, 594-606.
| Crossref | Google Scholar | PubMed |
Jordan GJ, Weston PH, Carpenter RJ, Dillon RA, Brodribb TJ (2008) The evolutionary relations of sunken, covered, and encrypted stomata to dry habitats in Proteaceae. American Journal of Botany 95, 521-530.
| Crossref | Google Scholar | PubMed |
Joyce EM, Schmidt-Lebuhn AN, Orel HK, Nge FJ, Anderson BM, Hammer TA, McLay TGB (2024) Navigating phylogenetic conflict and evolutionary inference in plants with target capture data. EcoEvoRxiv [Preprint, published 28 May 2024].
| Crossref | Google Scholar |
Joyce EM, Schmidt-Lebuhn AN, Orel HK, Nge FJ, Anderson BM, Hammer TA, McLay TGB (in press) Navigating phylogenetic conflict and evolutionary inference in plants with target capture data. Australian Systematic Biology. 10.1071/SB24011
Kadereit G, Gotzek D, Freitag H (2005) Origin and age of Australian Chenopodiaceae. Organisms Diversity & Evolution 5, 59-80.
| Google Scholar |
Kalyaanamoorthy S, Minh BQ, Wong TKF, Von Haeseler A, Jermiin LS (2017) ModelFinder: fast model selection for accurate phylogenetic estimates. Nature Methods 14, 587-589.
| Crossref | Google Scholar | PubMed |
Katoh K, Standley DM (2013) MAFFT multiple sequence alignment software version 7: improvements in performance and usability. Molecular Biology and Evolution 30, 772-780.
| Crossref | Google Scholar | PubMed |
Kayaalp P, Schwarz MP, Stevens MI (2013) Rapid diversification in Australia and two dispersals out of Australia in the globally distributed bee genus, Hylaeus (Colletidae: Hylaeinae). Molecular Phylogenetics and Evolution 66, 668-678.
| Crossref | Google Scholar | PubMed |
Keighery GJ, Rye BL, Tauss C (2023) Update to the taxonomy of Hypocalymma sect. Hypocalymma (Myrtaceae: Chamelaucieae), including hybrids and new species. Nuytsia 34, 21-60.
| Crossref | Google Scholar |
Keppel G, Nge FJ, Ibanez T (2023) Slowing taxon cycle can explain biodiversity patterns on islands: insights into the biogeography of the tropical South Pacific from molecular data. Journal of Systematics and Evolution 62, 201-214.
| Crossref | Google Scholar |
Korasidis VA, Wallace MW, Wagstaff BE, Hill RS (2019) Terrestrial cooling record through the Eocene–Oligocene transition of Australia. Global and Planetary Change 173, 61-72.
| Crossref | Google Scholar |
Ladiges PY, Cantrill D (2007) New Caledonia–Australian connections: biogeographic patterns and geology. Australian Systematic Botany 20, 383-389.
| Crossref | Google Scholar |
Ladiges PY, Udovicic F, Nelson G (2003) Australian biogeographical connections and the phylogeny of large genera in the plant family Myrtaceae. Journal of Biogeography 30, 989-998.
| Crossref | Google Scholar |
Ladiges PY, Kellermann J, Nelson G, Humphries CJ, Udovicic F (2005) Historical biogeography of Australian Rhamnaceae, tribe Pomaderreae. Journal of Biogeography 32, 1909-1919.
| Crossref | Google Scholar |
Ladiges PY, Ariati SR, Murphy DJ (2006) Biogeography of the Acacia victoriae, pyrifolia and murrayana species groups in arid Australia. Journal of Arid Environments 66, 462-476.
| Crossref | Google Scholar |
Ladiges PY, Marks CE, Nelson G (2011) Biogeography of Nicotiana section Suaveolentes (Solanaceae) reveals geographical tracks in arid Australia. Journal of Biogeography 38, 2066-2077.
| Crossref | Google Scholar |
Ladiges PY, Bayly MJ, Nelson G (2012) Searching for ancestral areas and artifactual centers of origin in biogeography: with comment on east–west patterns across southern Australia. Systematic Biology 61, 703-708.
| Crossref | Google Scholar | PubMed |
Lam N, Wilson PG, Heslewood MM, Quinn CJ (2002) A phylogenetic analysis of the Chamelaucium alliance (Myrtaceae). Australian Systematic Botany 15, 535-543.
| Crossref | Google Scholar |
Lamont BB, He T (2017) When did a Mediterranean-type climate originate in southwestern Australia? Global and Planetary Change 156, 46-58.
| Crossref | Google Scholar |
Lamont BB, He T, Lim SL (2016) Hakea, the world’s most sclerophyllous genus, arose in southwestern Australian heathland and diversified throughout Australia over the past 12 million years. Australian Journal of Botany 64, 77-88.
| Crossref | Google Scholar |
Lamont BB, Pausas JG, He T, Witkowski ET, Hanley ME (2020) Fire as a selective agent for both serotiny and nonserotiny over space and time. Critical Reviews in Plant Sciences 39, 140-172.
| Crossref | Google Scholar |
Landis MJ, Matzke NJ, Moore BR, Huelsenbeck JP (2013) Bayesian analysis of biogeography when the number of areas is large. Systematic Biology 62, 789-804.
| Crossref | Google Scholar | PubMed |
Landis M, Edwards EJ, Donoghue MJ (2021) Modeling phylogenetic biome shifts on a planet with a past. Systematic Biology 70, 86-107.
| Crossref | Google Scholar | PubMed |
Lanfear R, Hahn MW (2024) The meaning and measure of concordance factors in phylogenomics. Molecular Biology and Evolution 41(11), msae214.
| Crossref | Google Scholar |
Lanfear R, Calcott B, Kainer D, Mayer C, Stamatakis A (2014) Selecting optimal partitioning schemes for phylogenomic datasets. BMC Evolutionary Biology 14, 82.
| Crossref | Google Scholar | PubMed |
Lauretano V, Kennedy-Asser AT, Korasidis VA, Wallace MW, Valdes PJ, Lunt DJ, Pancost RD, Naafs BDA (2021) Eocene to Oligocene terrestrial Southern Hemisphere cooling caused by declining pCO2. Nature Geoscience 14, 659-664.
| Crossref | Google Scholar |
Lockhart PJ, McLenachan PA, Havell D, Glenny D, Huson D, Jensen U (2001) Phylogeny, radiation, and transoceanic dispersal of New Zealand alpine buttercups: molecular evidence under split decomposition. Annals of the Missouri Botanical Garden 88, 458-477.
| Crossref | Google Scholar |
Mai U, Mirarab S (2018) TreeShrink: fast and accurate detection of outlier long branches in collections of phylogenetic trees. BMC Genomics 19, 272.
| Crossref | Google Scholar | PubMed |
Mairal M, Pokorny L, Aldasoro JJ, Alarcón M, Sanmartín I (2015) Ancient vicariance and climate-driven extinction explain continental-wide disjunctions in Africa: the case of the Rand Flora genus Canarina (Campanulaceae). Molecular Ecology 24, 1335-1354.
| Crossref | Google Scholar | PubMed |
Marki PZ, Kennedy JD, Cooney CR, Rahbek C, Fjeldså J (2019) Adaptive radiation and the evolution of nectarivory in a large songbird clade. Evolution 73, 1226-1240.
| Crossref | Google Scholar | PubMed |
Martin H (2006) Cenozoic climatic change and the development of the arid vegetation in Australia. Journal of Arid Environments 66, 533-563.
| Crossref | Google Scholar |
Mast AR, Givnish TJ (2002) Historical biogeography and the origin of stomatal distributions in Banksia and Dryandra (Proteaceae) based on their cpDNA phylogeny. American Journal of Botany 89, 1311-1323.
| Crossref | Google Scholar | PubMed |
Matzke NJ (2013) Probabilistic historical biogeography: new models for founder-event speciation, imperfect detection, and fossils allow improved accuracy and model-testing. Frontiers of Biogeography 5(4), 242-248.
| Crossref | Google Scholar |
Matzke NJ (2015) Stochastic mapping under biogeographical models. Available at http://phylo.wikidot.com/biogeobears#stochastic_mapping [Verified 10 October 2019]
Maurin O, Anest A, Bellot S, Biffin E, Brewer G, Charles-Dominique T, Cowan RS, Dodsworth S, Epitawalage N, Gallego B, Gliaretta A, Goldenberg R, Gonçalves DJP, Graham S, Hoch P, Mazine F, Low YW, McGinnie C, Michelangeli FA, Morris S, Penneys DS, Pérez Escobar OA, Pillon Y, Turner IM, Vasconcelos T, Wilson PG, Zuntini AR, Baker WJ, Forest F, Lucas E (2021) A nuclear phylogenomic study of the angiosperm order Myrtales, exploring the potential and limitations of the universal Angiosperms353 probe set. American Journal of Botany 108, 1087-1111.
| Crossref | Google Scholar | PubMed |
McLay TGB, Bayly MJ, Ladiges PY (2016) Is south-western Western Australia a centre of origin for eastern Australian taxa or is the centre an artefact of a method of analysis? A comment on Hakea and its supposed divergence over the past 12 million years. Australian Systematic Botany 29, 87-94.
| Crossref | Google Scholar |
McLay TG, Fowler RM, Fahey PS, Murphy DJ, Udovicic F, Cantrill DJ, Bayly MJ (2023) Phylogenomics reveals extreme gene tree discordance in a lineage of dominant trees: hybridization, introgression, and incomplete lineage sorting blur deep evolutionary relationships despite clear species groupings in Eucalyptus subgenus Eudesmia. Molecular Phylogenetics and Evolution 187, 107869.
| Crossref | Google Scholar | PubMed |
Meudt HM, Lockhart PJ, Bryant D (2009) Species delimitation and phylogeny of a New Zealand plant species radiation. BMC Evolutionary Biology 9, 111.
| Crossref | Google Scholar | PubMed |
Milner ML, Weston PH, Rossetto M, Crisp MD (2015) Biogeography of the Gondwanan genus Lomatia (Proteaceae): vicariance at continental and intercontinental scales. Journal of Biogeography 42, 2440-2451.
| Crossref | Google Scholar |
Minh BQ, Nguyen MAT, Von Haeseler A (2013) Ultrafast approximation for phylogenetic bootstrap. Molecular Biology and Evolution 30, 1188-1195.
| Crossref | Google Scholar | PubMed |
Morlon H, Lewitus E, Condamine FL, Manceau M, Clavel J, Drury J (2016) RPANDA: an R package for macroevolutionary analyses on phylogenetic trees. Methods in Ecology and Evolution 7, 589-597.
| Crossref | Google Scholar |
Mueller F (1853) Diagnoses et descriptiones plantarum novarum, quas in Nova Hollandia. Linnaea: Ein Journal für die Botanik in Ihrem Ganzen Umfange, Oder Beiträge zur Pflanzenkunde 25, 386-387.
| Google Scholar |
Nauheimer L, Boyce PC, Renner SS (2012) Giant taro and its relatives: a phylogeny of the large genus Alocasia (Araceae) sheds light on Miocene floristic exchange in the Malesian region. Molecular Phylogenetics and Evolution 63, 43-51.
| Crossref | Google Scholar | PubMed |
Nauheimer L, Schley RJ, Clements MA, Micheneau C, Nargar K (2018) Australasian orchid biogeography at continental scale: molecular phylogenetic insights from the sun orchids (Thelymitra, Orchidaceae). Molecular Phylogenetics and Evolution 127, 304-319.
| Crossref | Google Scholar | PubMed |
Nge FJ, Thiele KR (2022) Calytrix calingiri, a new species from the Calytrix acutifolia species group (Myrtaceae: Chamelaucieae). Nuytsia 33, 251-261.
| Crossref | Google Scholar |
Nge FJ, Biffin E, Thiele KR, Waycott M (2020) Extinction pulse at Eocene–Oligocene boundary drives diversification dynamics of the two Australian temperate floras. Proceedings of the Royal Society of London – B. Biological Sciences 287, 20192546.
| Crossref | Google Scholar | PubMed |
Nge FJ, Biffin E, Thiele KR, Waycott M (2021a) Reticulate evolution, ancient chloroplast haplotypes, and rapid radiation of the Australian plant genus Adenanthos (Proteaceae). Frontiers in Ecology and Evolution 8, 616741.
| Crossref | Google Scholar |
Nge FJ, Kellermann J, Biffin E, Waycott M, Thiele KR (2021b) Historical biogeography of Pomaderris (Rhamnaceae): continental vicariance in Australia and repeated independent dispersals to New Zealand. Molecular Phylogenetics and Evolution 158, 107085.
| Crossref | Google Scholar |
Nge FJ, Biffin E, Waycott M, Thiele KR (2022) Phylogenomics and continental biogeographic disjunctions: insight from the Australian starflowers (Calytrix). American Journal of Botany 109, 221-232.
| Crossref | Google Scholar |
Nge FJ, Kellermann J, Biffin E, Thiele KR, Waycott M (2023) Rise and fall of a continental mesic radiation in Australia: spine evolution, biogeography, and diversification of Cryptandra (Rhamnaceae: Pomaderreae). Botanical Journal of the Linnean Society 204, 327-342.
| Crossref | Google Scholar |
Nge FJ, Hammer TA, Vasconcelos T, Biffin E, Kellermann J, Waycott M (2024a) Polyploidy linked with species richness but not diversification rates or niche breadth in Australian Pomaderreae (Rhamnaceae). Annals of Botany 2024, mcae181 [Corrected proof, published 23 October 2024].
| Crossref | Google Scholar |
Nge FJ, Chaowasku T, Damthongdee A, Wiya C, Soulé VRC, Rodrigues‐Vaz C, Bruy D, Mariac C, Chatrou LW, Chen J, Choo LM, Dagallier LMJ, Erkens RHJ, Johnson DM, Leeratiwong C, Lobão AQ, Lopes JC, Martínez‐Velarde MF, Munzinger J, Murray NA, Neo WL, Rakotoarinivo M, Ortiz‐Rodriguez AE, Sonké B, Thomas DC, Wieringa JJ, Couvreur T (2024b) Complete genus‐level phylogenomics and new subtribal classification of the pantropical plant family Annonaceae. Taxon 73(6), 1341-1369.
| Crossref | Google Scholar |
Nguyen L-T, Schmidt HA, Von Haeseler A, Minh BQ (2015) IQ-TREE: a fast and effective stochastic algorithm for estimating maximum-likelihood phylogenies. Molecular Biology and Evolution 32, 268-274.
| Crossref | Google Scholar | PubMed |
Onstein RE, Linder HP (2016) Beyond climate: convergence in fast evolving sclerophylls in Cape and Australian Rhamnaceae predates the mediterranean climate. Journal of Ecology 104, 665-677.
| Crossref | Google Scholar |
Onstein RE, Carter RJ, Xing Y, Richardson JE, Linder HP (2015) Do Mediterranean-type ecosystems have a common history? Insights from the buckthorn family (Rhamnaceae). Evolution 69, 756-771.
| Crossref | Google Scholar | PubMed |
Onstein RE, Jordan GJ, Sauquet H, Weston PH, Bouchenak-Khelladi Y, Carpenter RJ, Linder HP (2016) Evolutionary radiations of Proteaceae are triggered by the interaction between traits and climates in open habitats. Global Ecology and Biogeography 25, 1239-1251.
| Crossref | Google Scholar |
Orel HK, McLay TGB, Neal WC, Forster PI, Bayly MJ (2023) Plastid phylogenomics of the Eriostemon group (Rutaceae; Zanthoxyloideae): support for major clades and investigation of a backbone polytomy. Australian Systematic Botany 36, 355-385.
| Crossref | Google Scholar |
Paradis E, Claude J, Strimmer K (2004) APE: analyses of phylogenetics and evolution in R language. Bioinformatics 20, 289-290.
| Crossref | Google Scholar | PubMed |
Pease JB, Brown JW, Walker JF, Hinchliff CE, Smith SA (2018) Quartet sampling distinguishes lack of support from conflicting support in the green plant tree of life. American Journal of Botany 105, 385-403.
| Crossref | Google Scholar | PubMed |
Pokorny L, Riina R, Mairal M, Meseguer AS, Culshaw V, Cendoya J, Serrano M, Carbajal R, Ortiz S, Heuertz M (2015) Living on the edge: timing of Rand Flora disjunctions congruent with ongoing aridification in Africa. Frontiers in Genetics 6, 154.
| Crossref | Google Scholar | PubMed |
Pole M (1994) The New Zealand flora-entirely long-distance dispersal? Journal of Biogeography 21, 625-635.
| Crossref | Google Scholar |
Pontes-Nogueira M, Martins M, Alencar LR, Sawaya RJ (2021) The role of vicariance and dispersal on the temporal range dynamics of forest vipers in the Neotropical region. PLoS ONE 16, e0257519.
| Crossref | Google Scholar | PubMed |
Prentice E, Knerr N, Schmidt-Lebuhn AN, González-Orozco CE, Bui EN, Laffan S, Miller JT (2017) Do soil and climate properties drive biogeography of the Australian proteaceae? Plant and Soil 417, 317-329.
| Crossref | Google Scholar |
Price MN, Dehal PS, Arkin AP (2010) FastTree 2 – approximately maximum-likelihood trees for large alignments. PLoS ONE 5, e9490.
| Crossref | Google Scholar | PubMed |
Rabosky DL (2014) Automatic detection of key innovations, rate shifts, and diversity-dependence on phylogenetic trees. PLoS ONE 9, e89543.
| Crossref | Google Scholar | PubMed |
Rabosky DL, Grundler M, Anderson C, Title P, Shi JJ, Brown JW, Huang H, Larson JG (2014) BAMMtools: an R package for the analysis of evolutionary dynamics on phylogenetic trees. Methods in Ecology and Evolution 5, 701-707.
| Crossref | Google Scholar |
Ramírez-Barahona S, Sauquet H, Magallón S (2020) The delayed and geographically heterogeneous diversification of flowering plant families. Nature Ecology & Evolution 4, 1232-1238.
| Crossref | Google Scholar | PubMed |
Ree RH, Smith SA (2008) Maximum likelihood inference of geographic range evolution by dispersal, local extinction, and cladogenesis. Systematic Biology 57, 4-14.
| Crossref | Google Scholar | PubMed |
Renner MAM, Foster CSP, Miller JT, Murphy DJ (2020) Increased diversification rates are coupled with higher rates of climate space exploration in Australian Acacia (Caesalpinioideae). New Phytologist 226, 609-622.
| Crossref | Google Scholar | PubMed |
Renner MAM, Foster CSP, Miller JT, Murphy DJ (2021) Phyllodes and bipinnate leaves of Acacia exhibit contemporary continental-scale environmental correlation and evolutionary transition-rate heterogeneity. Australian Systematic Botany 34, 595-608.
| Crossref | Google Scholar |
Ronquist F (1997) Dispersal–vicariance analysis: a new approach to the quantification of historical biogeography. Systematic Biology 46, 195-203.
| Crossref | Google Scholar |
Rossetto M, Crayn D, Ford A, Ridgeway P, Rymer P (2007) The comparative study of range-wide genetic structure across related, co-distributed rainforest trees reveals contrasting evolutionary histories. Australian Journal of Botany 55, 416-424.
| Crossref | Google Scholar |
Rye BL (2009) A reduced circumscription of Balaustion and description of the new genus Cheyniana (Myrtaceae: Chamelaucieae). Nuytsia 19, 129-148.
| Crossref | Google Scholar |
Rye BL (2013) A revision of the south-western Australian genus Astartea (Myrtaceae: Chamelaucieae). Nuytsia 23, 189-269.
| Crossref | Google Scholar |
Rye BL (2016) An update to the taxonomy of some Western Australian genera of Myrtaceae tribe Chamelaucieae. 4. Malleostemon. Nuytsia 27, 103-120.
| Crossref | Google Scholar |
Rye BL (2017) An expanded circumscription and new infrageneric classification of Rinzia (Myrtaceae: Chamelaucieae). Nuytsia 28, 39-93.
| Crossref | Google Scholar |
Rye BL (2020) Description of the rare Goldfields Laceflower, Thryptomene planiflora (Myrtaceae: Chamelaucieae). Nuytsia 31,.
| Crossref | Google Scholar |
Rye BL (2021) Austrobaeckea, a new south-western Australian genus of Myrtaceae (Chamelaucieae: Hysterobaeckeinae). Nuytsia 32, 173-197.
| Crossref | Google Scholar |
Rye BL (2022) An expanded circumscription and revision of the Western Australian genus Balaustion (Myrtaceae: Chamelaucieae: Hysterobaeckeinae). Nuytsia 33, 149-204.
| Crossref | Google Scholar |
Rye BL (2024) New taxa and a key for Thryptomene (Myrtaceae: Chamelaucieae: Thryptomeninae). Nuytsia 35, 101-135.
| Crossref | Google Scholar |
Rye BL, Barrett MD (2020) A new species that’s worth its salt: Verticordia elizabethiae (Myrtaceae: Chamelaucieae), a salt-tolerant rarity from semi-arid Western Australia. Nuytsia 31, 259-263.
| Crossref | Google Scholar |
Rye BL, Trudgen ME (2008) Seorsus, a new Gondwanan genus of Myrtaceae with a disjunct distribution in Borneo and Australia. Nuytsia 18, 235-257.
| Crossref | Google Scholar |
Rye BL, Wilson PG (2022) Reduction of Corynanthera to the synonymy of Micromyrtus (Myrtaceae: Chamelaucieae: Micromyrtinae). Nuytsia 33, 321-324.
| Crossref | Google Scholar |
Rye BL, Wilson PG, Keighery GJ (2013) A revision of the species of Hypocalymma (Myrtaceae: Chamelaucieae) with smooth or colliculate seeds. Nuytsia 23, 283-312.
| Crossref | Google Scholar |
Rye BL, Wilson PG, Heslewood MM, Perkins AJ, Thiele KR (2020) A new subtribal classification of Myrtaceae tribe Chamelaucieae. Australian Systematic Botany 33, 191-206.
| Crossref | Google Scholar |
Rye BL, Keighery GJ, Barrett MD (2022) Description of a new south-western Australian plant group, Hypocalymma sect. Grandiflora (Myrtaceae: Chamelaucieae: Astarteinae). Nuytsia 33, 233-249.
| Crossref | Google Scholar |
Sanmartín I, Meseguer AS (2016) Extinction in phylogenetics and biogeography: from timetrees to patterns of biotic assemblage. Frontiers in Genetics 7, 35.
| Crossref | Google Scholar | PubMed |
Sanmartín I, Wanntorp L, Winkworth RC (2007) West Wind Drift revisited: testing for directional dispersal in the Southern Hemisphere using event-based tree fitting. Journal of Biogeography 34, 398-416.
| Crossref | Google Scholar |
Sauquet H, Weston PH, Anderson CL, Barker NP, Cantrill DJ, Mast AR, Savolainen V (2009) Contrasted patterns of hyperdiversification in Mediterranean hotspots. Proceedings of the National Academy of Sciences 106, 221-225.
| Crossref | Google Scholar | PubMed |
Sayyari E, Mirarab S (2016) Fast coalescent-based computation of local branch support from quartet frequencies. Molecular Biology and Evolution 33, 1654-1668.
| Crossref | Google Scholar | PubMed |
Schmidt-Lebuhn AN, Bovill J (2021) Phylogenomic data reveal four major clades of Australian Gnaphalieae (Asteraceae). Taxon 70, 1020-1034.
| Crossref | Google Scholar |
Schodde R (1989) Origins, radiations and sifting in the Australasian biota: changing concepts from new data and old. Australian Systematic Botanical Society Newsletter 60, 2-11.
| Google Scholar |
Schweizer M, Seehausen O, Hertwig ST (2011) Macroevolutionary patterns in the diversification of parrots: effects of climate change, geological events and key innovations. Journal of Biogeography 38, 2176-2194.
| Crossref | Google Scholar |
Simpson L, Cantrill DJ, Byrne M, Allnutt TR, King GJ, Lum M, Al Bkhetan Z, Andrew RL, Baker W, Barrett MD, Batley J, Berry O, Bink RM, Bragg JG, Broadhurst L, Brown GK, Bruhl JJ, Edwards RJ, Ferguson S, Forest F, Gustafsson J, Hammer TA, Holmes GD, Jackson CJ, James EA, Jones A, Kersey PJ, Leitch IJ, Maurin O, McLay TGB, Murphy DJ, Nargar K, Nauheimer L, Sauquet H, Schmidt-Lebuhn AN, Shepherd KA, Syme AE, Waycott M, Wilson TC, Crayn DM (2024) The Genomics for Australian Plants (GAP) framework initiative – developing genomic resources for understanding the evolution and conservation of the Australian flora. EcoEvoRxiv [Preprint, published 9 July 2024].
| Crossref | Google Scholar |
Simpson L, Cantrill DJ, Byrne M, Allnutt TR, King GJ, Lum M, Al Bkhetan Z, Andrew RL, Baker W, Barrett MD, Batley J, Berry O, Bink RM, Bragg JG, Broadhurst L, Brown GK, Bruhl JJ, Edwards RJ, Ferguson S, Forest F, Gustafsson J, Hammer TA, Holmes GD, Jackson CJ, James EA, Jones A, Kersey PJ, Leitch IJ, Maurin O, McLay TGB, Murphy DJ, Nargar K, Nauheimer L, Sauquet H, Schmidt-Lebuhn AN, Shepherd KA, Syme AE, Waycott M, Wilson TC, Crayn DM (in press) The Genomics for Australian Plants (GAP) framework initiative – developing genomic resources for understanding the evolution and conservation of the Australian flora. Australian Systematic Botany
| Crossref | Google Scholar |
Skeels A, Cardillo M (2019) Equilibrium and non-equilibrium phases in the radiation of Hakea and the drivers of diversity in Mediterranean-type ecosystems. Evolution 73, 1392-1410.
| Crossref | Google Scholar | PubMed |
Slater GSC, Birney E (2005) Automated generation of heuristics for biological sequence comparison. BMC Bioinformatics 6, 31.
| Crossref | Google Scholar | PubMed |
Smith SA, O’Meara BC (2012) treePL: divergence time estimation using penalized likelihood for large phylogenies. Bioinformatics 28, 2689-2690.
| Crossref | Google Scholar | PubMed |
Smith SA, Brown JW, Walker JF (2018) So many genes, so little time: a practical approach to divergence-time estimation in the genomic era. PLoS One 13, e0197433.
| Crossref | Google Scholar |
Sniderman JMK, Jordan GJ, Cowling RM (2013) Fossil evidence for a hyperdiverse sclerophyll flora under a non-Mediterranean-type climate. Proceedings of the National Academy of Sciences 110, 3423-3428.
| Crossref | Google Scholar | PubMed |
Sun J, Ni X, Bi S, Wu W, Ye J, Meng J, Windley BF (2014) Synchronous turnover of flora, fauna, and climate at the Eocene–Oligocene boundary in Asia. Scientific Reports 4, 7463.
| Crossref | Google Scholar | PubMed |
Thiele KR, Keighery GJ, Nge FJ, Rye BL (2022) Three new Western Australian species related to Calytrix violacea (Myrtaceae: Chamelaucieae). Nuytsia 33, 221-232.
| Crossref | Google Scholar |
Thomas DC, Surveswaran S, Xue B, Sankowsky G, Mols JB, Keßler PJA, Saunders RMK (2012) Molecular phylogenetics and historical biogeography of the Meiogyne–Fitzalania clade (Annonaceae): generic paraphyly and late Miocene–Pliocene diversification in Australasia and the Pacific. Taxon 61, 559-575.
| Crossref | Google Scholar |
Thornhill AH, Macphail M (2012) Fossil myrtaceous pollen as evidence for the evolutionary history of Myrtaceae: a review of fossil Myrtaceidites species. Review of Palaeobotany and Palynology 176, 1-23.
| Crossref | Google Scholar |
Thornhill AH, Ho SYW, Külheim C, Crisp MD (2015) Interpreting the modern distribution of Myrtaceae using a dated molecular phylogeny. Molecular Phylogenetics and Evolution 93, 29-43.
| Crossref | Google Scholar | PubMed |
Thornhill AH, Crisp MD, Külheim C, Lam KE, Nelson LA, Yeates DK, Miller JT (2019) A dated molecular perspective of eucalypt taxonomy, evolution and diversification. Australian Systematic Botany 32, 29-48.
| Crossref | Google Scholar |
Thornhill AH, Popple LW, Carter RJ, Ho SY, Crisp MD (2012) Are pollen fossils useful for calibrating relaxed molecular clock dating of phylogenies? A comparative study using Myrtaceae. Molecular Phylogenetics and Evolution 63, 15-27.
| Crossref | Google Scholar | PubMed |
Tiatragul S, Skeels A, Keogh JS (2023) Paleoenvironmental models for Australia and the impact of aridification on blindsnake diversification. Journal of Biogeography 50, 1899-1913.
| Crossref | Google Scholar |
Tonini J, Moore A, Stern D, Shcheglovitova M, Ortí G (2015) Concatenation and species tree methods exhibit statistically indistinguishable accuracy under a range of simulated conditions. PLoS Currents 7, ecurrents.tol.34260cc27551a527b124ec5f6334b6be.
| Crossref | Google Scholar | PubMed |
Toon A, Crisp M, Gamage H, Mant J, Morris DC, Schmidt S, Cook LG (2015) Key innovation or adaptive change? A test of leaf traits using Triodiinae in Australia. Scientific Reports 5, 12398.
| Crossref | Google Scholar | PubMed |
Waycott M, van Dijk K, Biffin E (2021) A hybrid capture RNA bait set for resolving genetic and evolutionary relationships in angiosperms from deep phylogeny to intraspecific lineage hybridization. bioRxiv 2021, 2021.09.06.456727 [Preprint, published 7 September 2021].
| Crossref | Google Scholar |
Wilson PG, Heslewood MM (2014) An expanded phylogenetic analysis of Sannantha (Myrtaceae) and description of a new species. Australian Systematic Botany 27, 78-84.
| Crossref | Google Scholar |
Wilson PG, Heslewood MM (2023) Revised taxonomy of the tribe Leptospermeae (Myrtaceae) based on morphological and DNA data. Taxon 72, 550-571.
| Crossref | Google Scholar |
Wilson PG, O’Brien MM, Gadek PA, Quinn CJ (2001) Myrtaceae revisited: a reassessment of infrafamilial groups. American Journal of Botany 88, 2013-2025.
| Google Scholar | PubMed |
Winkworth RC, Wagstaff SJ, Glenny D, Lockhart PJ (2005) Evolution of the New Zealand mountain flora: origins, diversification and dispersal. Organisms Diversity & Evolution 5, 237-247.
| Crossref | Google Scholar |
Worth JR, Jordan GJ, McKinnon GE, Vaillancourt RE (2009) The major Australian cool temperate rainforest tree Nothofagus cunninghamii withstood Pleistocene glacial aridity within multiple regions: evidence from the chloroplast. New Phytologist 182, 519-532.
| Crossref | Google Scholar | PubMed |
Yang Y, Smith SA (2014) Orthology inference in nonmodel organisms using transcriptomes and low-coverage genomes: improving accuracy and matrix occupancy for phylogenomics. Molecular Biology and Evolution 31, 3081-3092.
| Crossref | Google Scholar | PubMed |
Zhang C, Mirarab S (2022) Weighting by gene tree uncertainty improves accuracy of quartet-based species trees. Molecular Biology and Evolution 39, msac215.
| Crossref | Google Scholar | PubMed |
Zuntini AR, Carruthers T, Maurin O, Bailey PC, Leempoel K, Brewer GE, Epitawalage N, Françoso E, Gallego-Paramo B, McGinnie C, Negrão R, Roy SR, Simpson L, Toledo Romero E, Barber VMA, Botigué L, Clarkson JJ, Cowan RS, Dodsworth S, Johnson MG, Kim JT, Pokorny L, Wickett NJ, Antar GM, DeBolt L, Gutierrez K, Hendriks KP, Hoewener A, Hu A-Q, Joyce EM, Kikuchi IABS, Larridon I, Larson DA, de Lírio EJ, Liu J-X, Malakasi P, Przelomska NAS, Shah T, Viruel J, Allnutt TR, Ameka GK, Andrew RL, Appelhans MS, Arista M, Ariza MJ, Arroyo J, Arthan W, Bachelier JB, Bailey CD, Barnes HF, Barrett MD, Barrett RL, Bayer RJ, Bayly MJ, Biffin E, Biggs N, Birch JL, Bogarín D, Borosova R, Bowles AMC, Boyce PC, Bramley GLC, Briggs M, Broadhurst L, Brown GK, Bruhl JJ, Bruneau A, Buerki S, Burns E, Byrne M, Cable S, Calladine A, Callmander MW, Cano Á, Cantrill DJ, Cardinal-McTeague WM, Carlsen MM, Carruthers AJA, de Castro Mateo A, Chase MW, Chatrou LW, Cheek M, Chen S, Christenhusz MJM, Christin P-A, Clements MA, Coffey SC, Conran JG, Cornejo X, Couvreur TLP, Cowie ID, Csiba L, Darbyshire I, Davidse G, Davies NMJ, Davis AP, van Dijk K, Downie SR, Duretto MF, Duvall MR, Edwards SL, Eggli U, Erkens RHJ, Escudero M, de la Estrella M, Fabriani F, Fay MF, Ferreira PDL, Ficinski SZ, Fowler RM, Frisby S, Fu L, Fulcher T, Galbany-Casals M, Gardner EM, German DA, Giaretta A, Gibernau M, Gillespie LJ, González CC, Goyder DJ, Graham SW, Grall A, Green L, Gunn BF, Gutiérrez DG, Hackel J, Haevermans T, Haigh A, Hall JC, Hall T, Harrison MJ, Hatt SA, Hidalgo O, Hodkinson TR, Holmes GD, Hopkins HCF, Jackson CJ, James SA, Jobson RW, Kadereit G, Kahandawala IM, Kainulainen K, Kato M, Kellogg EA, King GJ, Klejevskaja B, Klitgaard BB, Klopper RR, Knapp S, Koch MA, Leebens-Mack JH, Lens F, Leon CJ, Léveillé-Bourret , Lewis GP, Li D-Z, Li L, Liede-Schumann S, Livshultz T, Lorence D, Lu M, Lu-Irving P, Luber J, Lucas EJ, Luján M, Lum M, Macfarlane TD, Magdalena C, Mansano VF, Masters LE, Mayo SJ, McColl K, McDonnell AJ, McDougall AE, McLay TGB, McPherson H, Meneses RI, Merckx VSFT, Michelangeli FA, Mitchell JD, Monro AK, Moore MJ, Mueller TL, Mummenhoff K, Munzinger J, Muriel P, Murphy DJ, Nargar K, Nauheimer L, Nge FJ, Nyffeler R, Orejuela A, Ortiz EM, Palazzesi L, Peixoto AL, Pell SK, Pellicer J, Penneys DS, Perez-Escobar OA, Persson C, Pignal M, Pillon Y, Pirani JR, Plunkett GM, Powell RF, Prance GT, Puglisi C, Qin M, Rabeler RK, Rees PEJ, Renner M, Roalson EH, Rodda M, Rogers ZS, Rokni S, Rutishauser R, de Salas MF, Schaefer H, Schley RJ, Schmidt-Lebuhn A, Shapcott A, Al-Shehbaz I, Shepherd KA, Simmons MP, Simões AO, Simões ARG, Siros M, Smidt EC, Smith JF, Snow N, Soltis DE, Soltis PS, Soreng RJ, Sothers CA, Starr JR, Stevens PF, Straub SCK, Struwe L, Taylor JM, Telford IRH, Thornhill AH, Tooth I, Trias-Blasi A, Udovicic F, Utteridge TMA, Del Valle JC, Verboom GA, Vonow HP, Vorontsova MS, de Vos JM, Al-Wattar N, Waycott M, Welker CAD, White AJ, Wieringa JJ, Williamson LT, Wilson TC, Wong SY, Woods LA, Woods R, Worboys S, Xanthos M, Yang Y, Zhang Y-X, Zhou M-Y, Zmarzty S, Zuloaga FO, Antonelli A, Bellot S, Crayn DM, Grace OM, Kersey PJ, Leitch IJ, Sauquet H, Smith SA, Eiserhardt WL, Forest F, Baker WJ (2024) Phylogenomics and the rise of the angiosperms. Nature 629(8013), 843-850.
| Crossref | Google Scholar |