Impacts of necrotising disease on the Endangered cauliflower soft coral (Dendronephthya australis)
Rosemary Kate Steinberg





A
B
C
D
Abstract
Diseases have affected coral populations worldwide, leading to population declines and requiring active restoration efforts.
Describe population and individual impacts of necrotising disease in the Endangered octocoral Dendronephthya australis.
We quantified population loss and recruitment by using reference photos, survey and GPS mapping and described disease lesions by using histopathology.
From December 2019 to January 2020, we observed polyp loss, necrotic lesions and loss of large colonies of D. australis at Botany Bay, New South Wales, Australia. By September 2020, only a few scattered recruits remained, and all large colonies were lost. Histopathology of colonies sampled in January 2020 confirmed that the disease had resulted in necrosis, gastrovascular canal collapse and internal colony integrity loss, leading to mortality. New recruits were recorded within 10 months of disease onset, and large colonies within 18 months.
Although the necrotising disease had significant impacts on both the individual and population level, natural recruitment began quickly. As such, unlike in other populations, restoration is not currently required in the Bare Island D. australis population.
The extent of disease impact at the individual and population levels suggests that monitoring for lesions should be undertaken before developing conservation and restoration strategies for this species.
Keywords: alcyonacea, Botany Bay, coral disease, disease, disease histology, marine disease, octocoral, temperate, temperate reef.
Introduction
Marine diseases are one of the greatest threats to marine habitat-forming species in the Anthropocene, with disease having driven declines and extirpations across taxa including corals, seagrass and macroalgae (Hughes 1994; Campbell et al. 2014; Zannella et al. 2017; Qiu et al. 2019). Disease impacts on habitat-forming species not only threaten the physical structure of these systems, but can also have bottom-up effects on a wide range of associated fauna (Hoegh-Guldberg and Bruno 2010) and can alter interactions between habitat-forming species and their predators (Campbell et al. 2014). Diseases in marine habitat-forming species have been more clearly documented in some groups than in others. Algal, seagrass, bivalve and stony and gorgonian coral diseases are well studied (Peters et al. 1983; Bally and Garrabou 2007; Bruno et al. 2007; Case et al. 2011; Zannella et al. 2017), whereas diseases in groups such as alcyonacean octocorals are poorly described (Work and Meteyer 2014; Weil et al. 2015). Unfortunately, like stony corals, octocorals are under increasing threat from climate change and disease, but disease in this group has been well-documented only in gorgonian octocorals (Weil et al. 2015; Steinberg et al. 2020).
Octocorals provide invaluable ecosystem services to marine species in all climate zones and depths (Fabricius and Alderslade 2001; Steinberg et al. 2020). Invertebrates use octocorals as refuge, grazing substrate and food (Greene 2008; Finlay-Jones et al. 2021). As many octocorals have complex branching morphologies, they are habitat for invertebrates including gastropods, ophiuroids, copepods, amphipods and other arthropods (Bayer 1961; Muzik 1982; Wendt et al. 1985; Greene 2008; Poulos et al. 2013; Maggioni et al. 2020; Finlay-Jones et al. 2021). Octocorals also support vertebrate species, providing important shelter for seahorses and a food source for butterflyfish (Lourie and Randall 2003; Pratchett 2007; Harasti et al. 2014). In temperate Australia, habitats dominated by the Endangered octocoral Dendronephthya australis have been found to have a higher biodiversity value than do surrounding sand, seagrass and sponge garden habitats (Poulos et al. 2013); and in the Great Barrier Reef, fish diversity increased with increasing octocoral, but not stony coral, cover (Epstein and Kingsford 2019). As such, loss of octocorals from benthic habitats due to stressors such as disease may significantly affect mobile species biodiversity.
Histopathology can be used to characterise the cellular characteristics of lesions (Work and Meteyer 2014). Unlike many stony corals, octocorals have a pronounced inflammatory response, making them excellent subjects for histology (Dennis et al. 2020). Gorgonian octocorals also have clearly defined granular amoebocyte immune cells that show up well under haematoxylin and eosin staining (Mydlarz et al. 2008). The majority of research into octocoral diseases has focused on gorgonian sea fans and little is known about histopathology of alcyonacean octocorals (Mydlarz et al. 2008; Tracy et al. 2018, 2021; Dennis et al. 2020; Calderón-Hernández et al. 2021; but see Slattery et al. 2013). Octocoral colonies are made up of polyps connected by coenenchyme, which capture and digest food, and the gastrovascular canals and siphonophores, which move water through the colony and maintain the colony shape. The gastrodermal layer lines the interior structures, and the epidermis lines the outside of the colony; between the two cell layers is the mesoglea, a layer of connective tissue and sclerites. Disruption of these structures can be indicative of predation or disease, such as visible aggregations of pathogens or necrotic tissues (Mydlarz et al. 2008; Work et al. 2012; Work and Aeby 2014; Raymundo et al. 2016; Tracy et al. 2018, 2021; Dennis et al. 2020; Calderón-Hernández et al. 2021). Documenting changes in tissue health and the proportions of tissue types can characterise the damage to the colony caused by disease.
The octocoral D. australis has been listed as an Endangered species because of ongoing declines across New South Wales (NSW), Australia. As such, monitoring of known populations is critical for understanding further declines or potential recovery (Harasti 2016; Larkin et al. 2021a; NSW Fisheries Scientific Committee 2021). Octocoral populations can naturally regenerate after declines through recruitment of larvae from donor populations or through clonal reproduction, providing environmental conditions are suitable (Steinberg et al. 2020). If the habitat is no longer suitable for the affected species, or if no donor populations exist for the affected population, restoration of the habitat or species may be required (McDonald et al. 2016; Steinberg et al. 2020). For example, populations of D. australis in Port Stephens, NSW, are not regenerating naturally and declines have continued for several years, suggesting that restoration is needed (Harasti 2016; Larkin et al. 2021a). In fact, successful aquaculture and transplantation trials have already begun in these highly disturbed habitats (Larkin et al. 2021b, 2023a). In Botany Bay, it is unclear whether populations would regenerate naturally following loss of colonies.
Here, we aimed to map the decline of D. australis in Botany Bay, NSW, and subsequent natural recruitment; and document the impact of field-observed lesions on individual colonies through histopathology. With this information, we hope to understand the reason for the D. australis population decline at Bare Island and determine whether or not assisted regeneration is needed for population recovery.
Materials and methods
Survey and collection of D. australis
The sponge gardens of Bare Island in Botany Bay, New South Wales, Australia (33°59′31″S, 151°13′55″E, Fig. 1) comprise at least 14 sponge species and 2 octocoral species (Poore et al. 2000). Dense aggregations of the Endangered octocoral D. australis are intermixed with the sponge gardens on western rock platforms that include a lower and upper platform. The study sites include these two rock platforms that make up the main Botany Bay population of D. australis and a search area from the platforms to 200 m seaward of the rock platforms. Although scattered colonies are found throughout the Bare Island area, we did not observe any other areas with aggregations. The lower platform was located at 10 m and the upper platform was located at 8-m depth. The lower platform perimeter measures 72.7 m and the area measures 312 m2. The upper platform perimeter measures 94.3 m, and the area measures 347 m2. These platforms were chosen as the study sites because they have the largest known aggregations of D. australis at Bare Island.
Initial observations and photographs of D. australis colonies were collected during pre-survey dives at the study sites at Bare Island on 30 January, 6 March and 19 March 2018, and photographs of colonies were taken. Initial lesions on D. australis colonies at the Bare Island study sites were then observed in late December 2019 (J. Turnbull, pers. obs.) and SCUBA surveys of the platforms were conducted on 19 January 2020, 23 September 2020, 9 October 2020, 9 March 2021 and 30 April 2021, to document changes following that observation. Mapping was conducted along with surveys on 9 October 2020, 9 March 2021 and 30 April 2021, when a GPS unit became available. For further details and mapping results, see the Supplementary material (Methods, Results and Fig. S1). Before mapping, number of colonies on each platform was recorded, but exact locations could not be determined (Fig. S2, S3). To ensure that all colonies on both platforms were accounted for, surveys began on the upper platform and the entire platform was swum in pairs less than 1 m above the substrate, after which the edge of the platform was re-surveyed because the majority of colonies were found on the platform edges. This process was then repeated on the lower platform. Overall, surveys took ~40 min. Colonies were classified as extra small (<5 cm tall), small (5–10 cm tall), medium (11–20 cm tall) or large (>20 cm tall). It should be noted that these corals are highly contractile with the tide, and as such ‘medium’ and ‘large’ size classes were difficult to differentiate between tides (Davis et al. 2015). Because retracted colonies are smaller than inflated colonies, size-class bins were halved when colonies were retracted to account for semi-retracted colonies, although colonies can retract much more than this (Davis et al. 2015). To account for this, survey dives were undertaken at mid-tide, when colonies were expected to be inflated; however, this expectation was not always met. Even when fully retracted, large colonies do not look like small colonies because the large number of branches are clearly visible. As such, best judgment sometime was required when determining size classes.
Impact of disease on individual coral
D. australis branches were collected using 12.5-cm blunt-tipped surgical scissors and placed in individual bags. Four branches of healthy D. australis were collected by SCUBA from Bare Island on 6 March 2018 and 19 March 2018, and five branches were collected from healthy colonies and four from diseased colonies on 19 January 2020. Healthy colonies were defined as those with no visible lesions and normal extension (Fig. 2a), whereas diseased colonies were defined as those with visible missing polyps (Fig. 2b) or visible necrosis (Fig. 2c). The entirety of one small necrotic colony that was no longer attached to the benthos was also collected on 19 January 2020. Colonies collected on 19 January 2020 were used for histological analysis. Branches ranged from 4 to 9 cm long. Collection causes no lasting damage to the colonies (Larkin et al. 2023a). Whole colonies were not taken as has been previously done (e.g. Corry et al. 2018) because the population of D. australis at Bare Island is small compared with other populations and removal of entire colonies was likely to cause harm to the population.
Progression of Dendronephthya australis lesions. (a) Healthy D. australis colony, (b) initial lesions observed on 22 December 2019 with close-up of gastropod inset, and (c) secondary lesions with characteristic colony trunk necrosis.
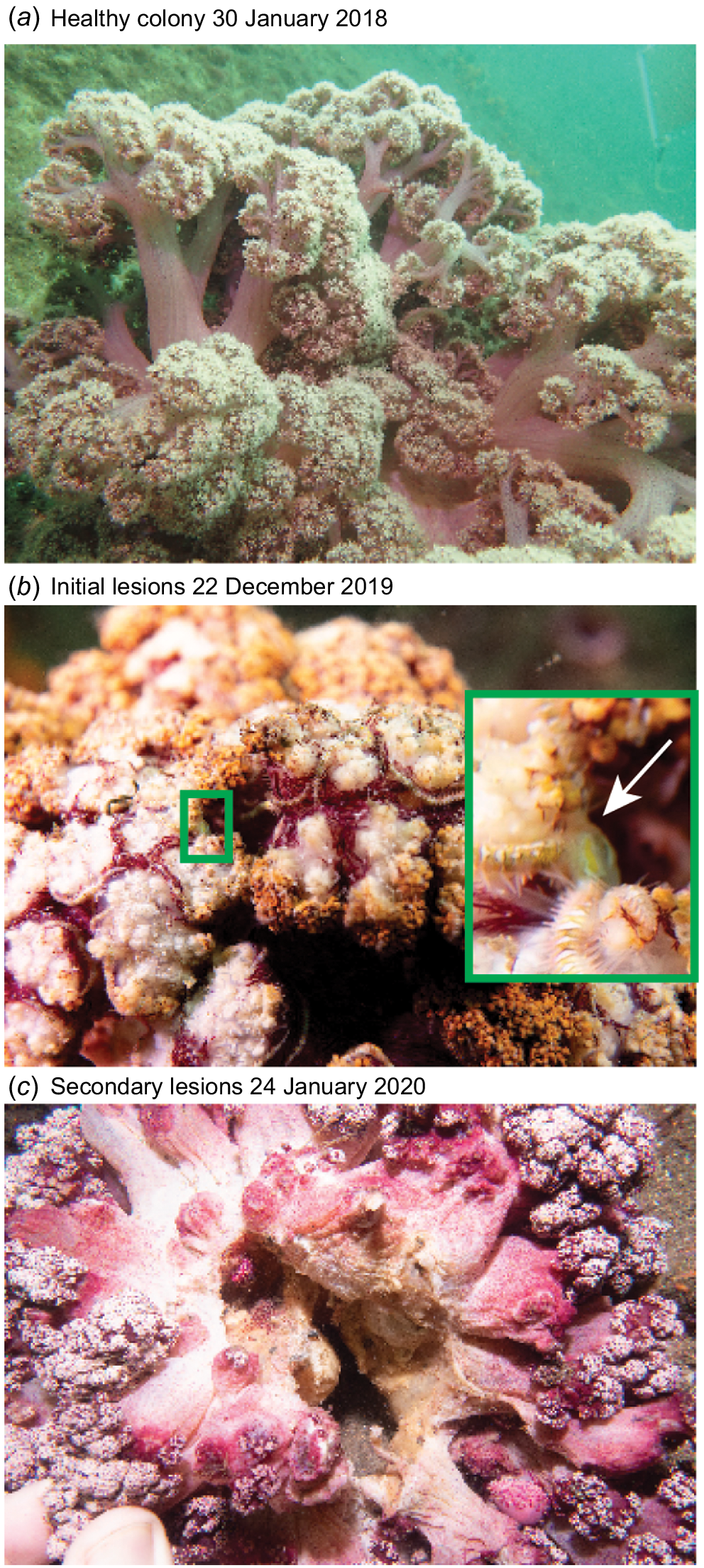
All samples were collected under New South Wales Department of Primary Industries permit number P13/0007-2.0 and OUT18/2054.
To prepare the samples collected on 19 January 2020 for histology, the branches were placed in seawater after epifauna were removed and formaldehyde was added at 10% of seawater volume. Samples remained in formalin for 1 month before being transferred to 70% ethanol. All healthy samples were subsampled twice for histology, including one stalk sample and one polyp sample. Damaged samples were subsampled from one to five times, because all sections of visibly damaged tissue were sampled with a margin of visually healthy surrounding tissue. All samples were decalcified in 20% w/v EDTA solution over 12 days, with the solution being changed every weekday for a total of 10 changes as per Wada et al. (2016). Samples were then rinsed in reverse osmosis (RO) water and dried in 70% ethanol for at least 2 weeks as per Tracy et al. (2021). Samples were embedded in paraffin, sliced 4 μm thick, and paired serial sections were stained with haematoxylin and eosin (H&E) and Masson’s trichrome as per Mandelberg et al. (2016).
Structures within D. australis were identified by referencing Fabricius and Alderslade (2001), Mandelberg et al. (2016) and Garra et al. (2020). Histology and subgross histological examination were performed on a subset of samples, with one stalk and one polyp section per colony. Histology was examined on slides stained with H&E, whereas subgross histological analysis was performed on paired slides stained with Masson’s trichrome because this stain has a greater colour contrast. The polyp section with the most polyps was chosen, and stalk sections were chosen at random. On histology, we documented collapsed gastrovascular canals, expansion, attenuation, or sclerite proliferation, necrosis or loss of the gastrodermis, epidermis, and mesentery filaments, hyperplasia of the epidermis, and discoloured (brown or eosinophilic) cells or mesoglea.
All histological slices were analysed with the quantitative pathology and bio-image analysis program QuPath (ver. 0.2.3, see https://qupath.github.io/; Bankhead et al. 2017), which allows for whole slide image analysis. The program was trained on four images (two from each health state, i.e. damaged and visibly healthy) to distinguish among three tissue classes, namely, gastrodermal and epidermal cells, mesoglea, and empty spaces left by gastrovascular canals and decalcified sclerites. The three tissue classes were different colours when stained with Masson’s trichrome; gastrodermal and epidermal cells were red, mesoglea was blue, and gastrovascular canals and sclerites were white because the spaces do not contain tissue. Regions of interest (ROI) were defined as the tissue and all open space within the tissue so that gastrovascular canals and sclerites could be quantified whereas background colour outside the coral was disregarded. As QuPath could not distinguish sclerites and mesoglea by colour, sclerites were counted manually. To do so, the images were opened in QuPath with a 250 × 250-μm2 grid overlay. Ten random 4 × 4 grid-square areas (1 × 1 mm2) were selected, with five including the sclerite-dense outer layer and five excluding this area. To account for large sclerites (longer than 1 mm), any sclerite with 50% or more of its area with the grid was counted.
Differences in proportions of tissue class (gastrodermal and epidermal cells, mesoglea, and gastrovascular canals and sclerites) between health states within stalk and polyps as determined by QuPath analysis were examined using a generalised linear mixed model (GLMM) with the package glmmTMB (ver. 1.1.7, see https://cran.r-project.org/package=glmmTMB; Brooks et al. 2017), by using a Gaussian distribution, residuals were checked graphically using the package DHARMa (ver. 0.4.6, F. Hartig, see https://cran.r-project.org/package=DHARMa/), and pairwise comparisons were made using the package emmeans (ver. 1.8.7, R. Lenth, H. Singmann, J. Love, P. Buerkner and M. Herve, see https://cran.r-project.org/package=emmeans). The response variable (percentage of the coral that was in each tissue class of gastrodermal and epidermal cells, mesoglea, and gastrovascular canals and sclerites space) was log transformed for analyses of stalk slices to meet model assumptions. Because there were two slices of coral per slide and multiple slices per sample, slide number was a random effect nested within slice, which was nested within the random effect of sample number. Differences in the number of sclerites between damaged and undamaged colonies was examined using a GLMM, by using a Poisson distribution in the package lme4 (ver. 1.1-34, see https://CRAN.R-project.org/package=lme4; Bates et al. 2015), with colony number, area (edge or centre) and body section (stalk or polyps) being included as random factors. Residuals were checked and pairwise analyses performed as above. All analyses were performed in R (ver. 4.0.5 (2021-03-31), R Foundation for Statistical Computing, Vienna, Austria, see https://www.r-project.org/). All plots were produced using the package ggplot2 (ver. 3.4.2, see https://CRAN.R-project.org/package=ggplot2; Wickham 2011).
Results
Survey of D. australis colonies
Healthy D. australis colonies have a multi-stalked, cauliflower-like growth form with full, polyp-filled crowns (Fig. 2a). Damaged colonies presented with different lesions through time. Initial lesions were characterised by whole colony retraction during mid-tide and large numbers of missing polyps. Secondary lesions were first observed on 24 January 2020 and were characterised by retracted colonies with large patches of dark brown or black necrotic tissue, missing branches and necrotic holes through the centre of colonies (Fig. 2c). The black necrotic tissue dissociated from the colonies with slight water movement, and underneath the tissue was light brown against the usually healthy pink of the corals (Fig. 2c). Colonies with either lesion type are hereafter referred to as ‘damaged’. Because gastropods are known to predate on D. australis (Davis et al. 2018; Finlay-Jones et al. 2021), presence of gastropods was noted. An unknown gastropod was photographed during onset of initial lesions (Fig. 2b, inset), and several egg cowries, Globovula cavanaghi, were observed laying eggs on branches (Fig. S4); however, no predation by the cowries was noted.
Survey results are presented in Table 1 and Fig. 3a, b (also see Fig. S1, S2 and S3). Before 24 January 2020, photographs were taken incidentally to sample collection and the number of colonies photographed is reported in Table 1, and the photographs presented in Fig. S2 and S3, but density is not calculated as the photographs are likely underestimate the full population. For mapping results, please see the Results in the Supplementary material.
Date | Observation type | Number recorded | Density per 100 m2 | |
---|---|---|---|---|
30 January 2018 | Sampling | 19 | – | |
6 March 2018 | Sampling | 15 | – | |
19 March 2018 | Sampling | 15 | – | |
24 January 2020 | Survey | 9 | 1.4 | |
23 September 2020 | Survey | 0 | 0 | |
9 October 2020 | Survey | 13 | 1.9 | |
9 March 2021 | Survey | 25 | 3.8 | |
30 April 2021 | Survey | 39 | 6.9 |
Those recorded during sampling do not represent the total number of colonies present because they were counted on photographs that were incidental to sampling effort, because such density cannot be calculated.
Populations of Dendronephthya australis before (25 September 2018) and after (23 September 2020) lesions and population decline. (a) A photo of a field of D. australis on the upper platform on 25 September 2018; at least 15 large colonies can be seen. (b) Four landscape photographs of the upper and lower platforms on 23 September 2020; no D. australis can be seen. Photographs are representative of relative abundance of D. australis and were not taken in the same position and orientation.
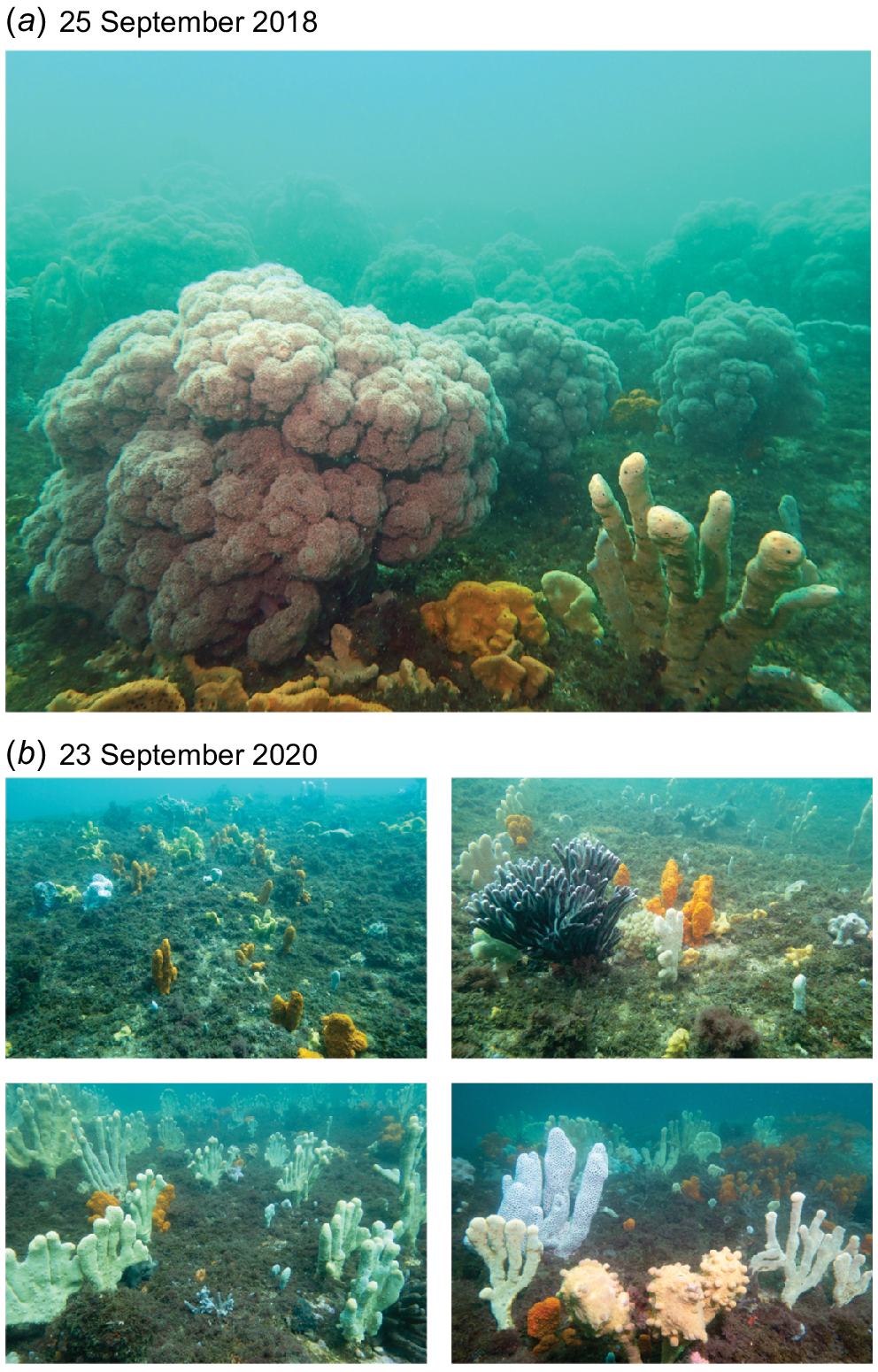
Impact of disease on individual coral
Results of histological examination of 10 H&E-stained slides of visually healthy (Fig. 4a–f, i) and seven slides of damaged (Fig. 4g, h, j–l) specimens are presented in Table 2. No signs of bacterial aggregation, fungal filaments or other pathogens were noted on histological sections. Because D. australis is aposymbiotic, Symbiodiniaceae were not examined during histology.
Histological examination of H&E-stained slices of Dendronephthya australis examining (a, d, g, j) stalk tissue, (b, e, h, k, l) polyp tissue, and (c, f, i, m) reproductive tissues at (a, f) low magnification, (b, d, g, h) mid-magnification, and (c, i–m) high magnification. (a, d) Examples of healthy D. australis stalk tissue; labels are as follows: mg, mesoglea; gvc, gastrovascular canal; ed, epidermis; gd, gastrodermis; mf, mesentery filament; sc, sclerite. (b, e) Examples of healthy D. australis polyp tissue; additional labels are as follows: pol, polyp; te, tentacle; ap, actinopharynx. (c) Spermaries found in a visibly healthy specimen. (f, i) Maturing brooding larvae within a gastrovascular canal of a visibly healthy colony; additional labels are as follows: bl, brooding larvae; e-hm, eosinophilic hyaline membrane. Note the bright red eosinophilic hyaline membrane, which is abnormal. (g, j) Examples of damaged D. australis stalk tissue. Note the expanded mesoglea (e-mg), collapsed gastrovascular canals (c-gvc), and necrotic–inflammatory gastrodermis (n-i gd) and epidermis (n-i ed). (h, k, l) Examples of damaged D. australis polyp tissue. Note the necrotic polyp (n-pol), the necrotic–inflammatory epidermis, necrotic mesoglea (n-gm), brown cells (br-c), and the atrophied actinopharynx, whose cells appear cuboidal as opposed to the healthy columnar configuration. (m) Brooding larvae at approximately the four-cell stage.
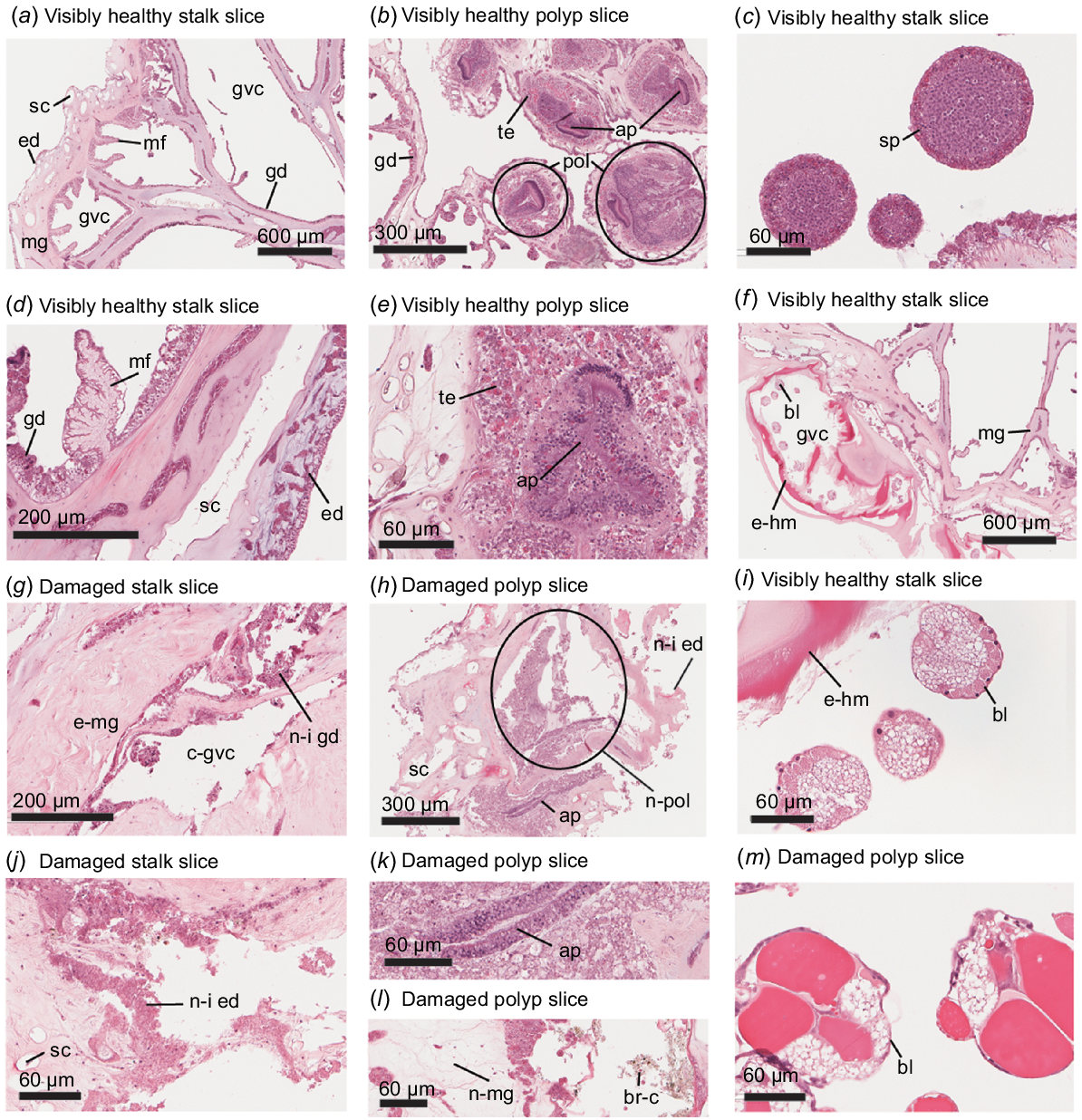
Characteristic | Healthy (10 total) | Damaged (7 total) | |
---|---|---|---|
Collapsed gastrovascular canals | 1 | 6 | |
Expansion of mesoglea | 1 | 5 | |
Cellular dissociation | 2 | 7 | |
Necrosis or loss of gastrodermis | 2 | 7 | |
Necrosis or loss of epidermis | 1 | 7 | |
Necrosis or loss of mesentery filaments | 1 | 6 | |
Hyperplasia of epidermis | 2 | 3 | |
Brown cells | 0 | 3 | |
Eosinophilic cells | 0 | 1 | |
Eosinophilic mesoglea | 2 | 0 | |
Brooding larvae | 1 | 1 |
The number of colonies exhibiting each characteristic out of 10 for healthy colonies and out of 7 for damaged colonies.
In all subgross histology figures, the gastrodermis and epidermis are stained red, whereas the mesoglea is stained blue (Fig. 5 and Fig. S5). Stalk and polyp tissue composition differed among the classes of tissue and there was an interaction between health state and tissue class (ANOVA, P < 0.05), but there was no difference between the health state alone (ANOVA, P > 0.05). All tissue classes (mesoglea, gastrodermal and epidermal cells, and empty space left by gastrovascular canals and sclerites) were significantly different between damaged and visibly healthy individuals (ANOVA, P < 0.05). For stalk and polyp slices, there was a significantly higher proportion of mesoglea in damaged than visibly healthy individuals, and significantly fewer gastrodermal and epidermal cells or empty space (Fig. 6a, b, Table 3). There was no significant difference in the number of sclerites between damaged and undamaged colonies (Table 4).
Subgross examination of Masson’s trichrome-stained micrographs of Dendronephthya australis. (a) Subgross micrograph of a visibly healthy stalk. Note the honeycomb-like structure of tissues and gastrovascular canals. (b) Subgross micrograph of a lesioned stalk. Note collapsed gastrovascular canals, increased mesoglea, possible hyperplastic epidermis and damage extending beyond lesion site. (c) Subgross micrograph of visibly healthy polyps. Note clearly defined polyps and honeycomb-like structure of tissues and gastrovascular canals. (d, e) Subgross micrographs of lesioned polyps. In (d), note increased size of gastrovascular canals, attenuation or atrophy of mesentery filaments, and thinning of mesoglea. In (e), note loss of eosinophilia of polyp tips, collapsed gastrovascular canals, a possible increase in sclerites and damage extending beyond lesion site. Structures are labelled as follows: pol, polyps; ed, epidermis; gd, gastrodermis; mg, mesoglea; gvc, gastrovascular canal; mf, mesentery filament; L, lesion site.
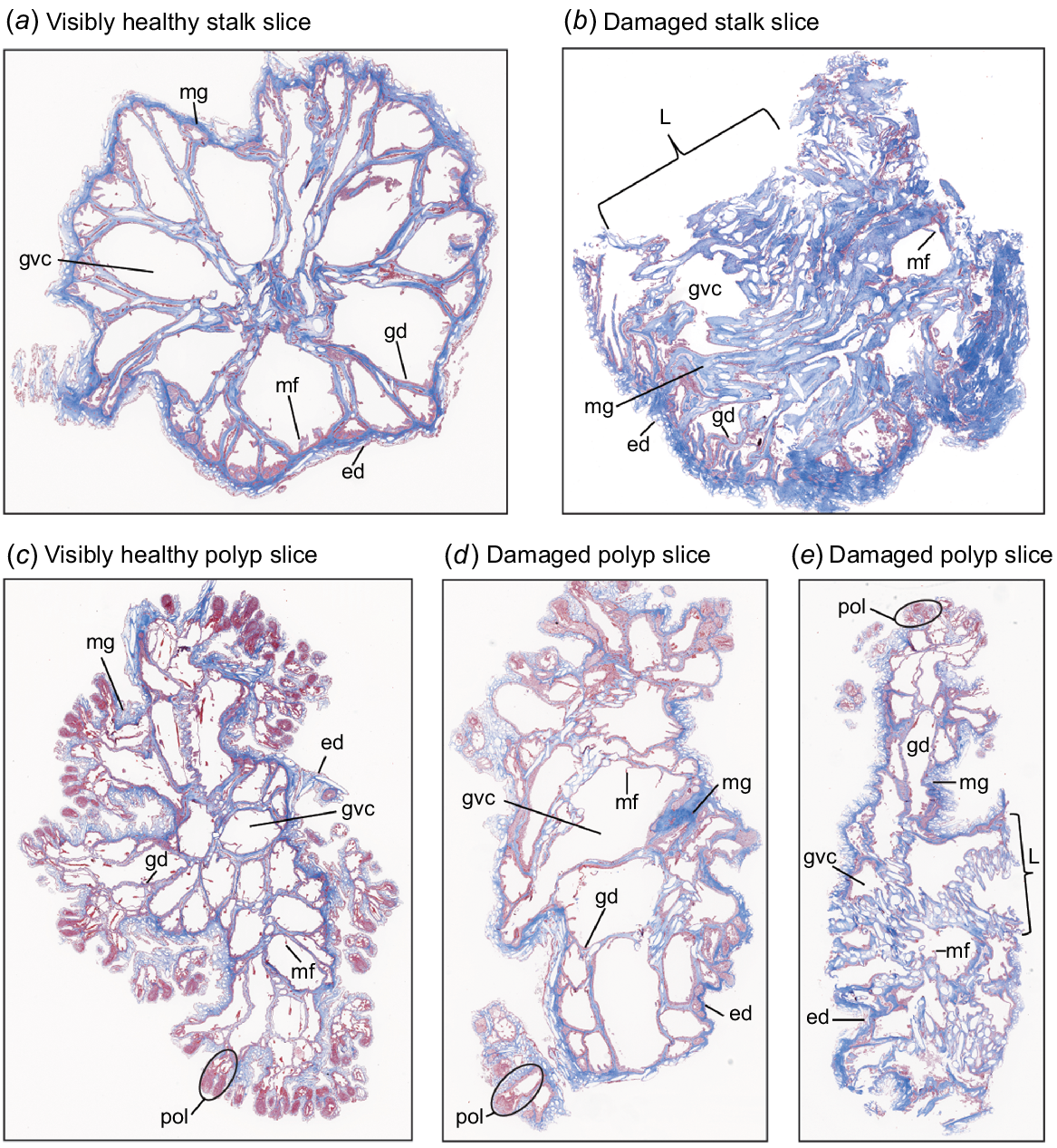
Boxplots of Dendronephthya australis subgross histology analyses contrasting damaged and undamaged tissues for different tissue types. (a) Percentage of region of interest (ROI) area, which includes the entire coral slice and all empty space left by gastrovascular canals and sclerites within the slice, of tissue composition of stalk slices as quantified in QuPath, and (b) tissue composition of polyp slices as quantified in QuPath. Significance between visual health conditions is denoted as follows: *, P < 0.05; **, P < 0.005; ***, P < 0.0005; all slice types and tissue types were significantly different.
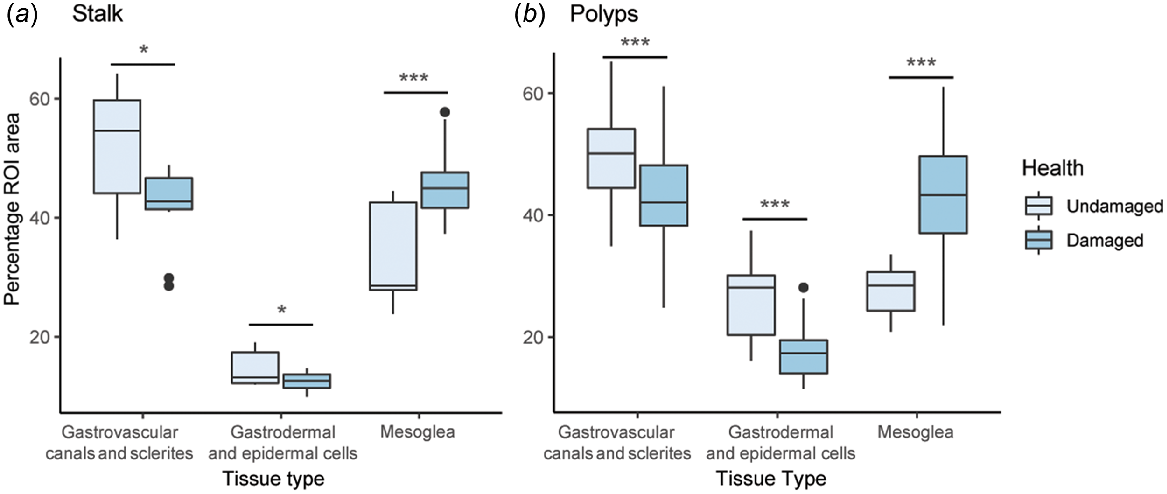
Factor tested | Body slices | Polyp slices | |||||
---|---|---|---|---|---|---|---|
GLMM | χ 2 | d.f. | Pr(>χ 2) | χ 2 | d.f. | Pr(>χ 2) | |
Health | 0.07 | 1 | 0.8 | 0 | 1 | 0.99 | |
Class | 634.1 | 2 | <2e−16 | 473.9 | 2 | <2e−16 | |
Health × class | 41.6 | 2 | 1.4e−7 | 118.6 | 2 | <2e−16 | |
Pairwise | t-ratio | d.f. | P | t-ratio | d.f. | P | |
Background: damaged–undamaged | −2.7 | 56 | 0.008 | −3.8 | 218 | 0.0002 | |
Dermal cells: damaged–undamaged | −2.1 | 56 | 0.04 | −5.0 | 218 | <0.0001 | |
Mesoglea: damaged–undamaged | 4.4 | 56 | <0.0001 | 8.9 | 218 | <0.0001 |
Significant P-values are indicated in bold.
Discussion
We documented a population decline associated with the appearance of necrotic lesions and subsequent recruitment in the Endangered cauliflower soft coral, D. australis, at Bare Island, Botany Bay, Australia. Polyp lesions were recorded in December of 2019, followed by larger, necrotic lesions that extended into the trunk of colonies in January 2020, and no large colonies were surveyed on the study sites by September 2020. Although disease may have played a role in the observed declines, other factors, including bushfires, could also have been the cause. Lesions were associated with changes in the structure of the colonies, including collapsed gastrovascular canals, expanded mesoglea and significant necrosis. After loss of all large colonies, natural recruitment of D. australis was recorded within 10 months of the onset of disease and large colonies were recorded within 18 months, suggesting that natural recruitment and growth rates of D. australis are quite high at Bare Island. Unfortunately, little is known about diseases and their consequences in this Endangered species. We found that disease is a serious threat to D. australis, suggesting that disease monitoring should be undertaken during survey of this species. Development of interventions may need to become a management priority if further disease and loss is discovered.
D. australis declines and recovery
Although systematic surveys were not conducted prior to observation of lesions at Bare Island, collection of distal branches had been ongoing since 6 March 2018. During this time, the population appeared stable, with dense aggregations across both platforms as exemplified in Fig. 3. Unfortunately, all large colonies at Bare Island were lost between December 2019 and September 2020. December 2019 and January 2020 were part of an El Niño cycle in eastern Australia, which saw high temperatures, low rainfall, coral bleaching in the Great Barrier Reef and severe bushfires in NSW that affected estuarine benthic habitats (Barros et al. 2022; Gissing et al. 2022), including shifting benthic communities (Bracewell et al. 2023), and may have affected the estuarine habitats at Bare Island. Population loss has also occurred in other estuaries; notably, two populations in Port Stephens, NSW, Australia (Seahorse Gardens and Pipeline) declined by 96 and 73% respectively, between 2009 and 2015 and these declines are ongoing (Harasti 2016; Larkin et al. 2021a). Although the Bare Island population is relatively small compared to Port Stephens, declines here suggest that colonies outside the main population centre in Port Stephens are also vulnerable to disturbances and should be included in monitoring efforts. In our study, the declines do not appear to be caused by physical smothering or damage from boating equipment as has been recorded previously (Harasti 2016; Larkin et al. 2021a). Instead, losses resulted after lesions from an unknown source. Sclerite proliferation can occur around predation lesions (Calderón-Hernández et al. 2021), but no evidence of proliferation was found in this study. In addition, no pathogens were noted on histological sections, suggesting that the disease was either caused by a non-infectious agent or the infectious agent is not observable on light microscopy and a higher resolution technique, such as electron microscopy, could possibly identify the cause. Additionally, the declines coincided with the Black Summer bushfires of 2019, which significantly affected estuarine environments and could have affected D. australis in Botany Bay (Barros et al. 2022; Bracewell et al. 2023). As such, D. australis appears to be vulnerable to declines from multiple stressors across its range.
The initial lesions recorded on D. australis at Bare Island are consistent with previously described predation lesions by fish or gastropods (Griffith 1994; Davis et al. 2018; Garra et al. 2020). Previous records of predation lesions of D. australis have shown that colonies retract and lose feeding opportunities (Davis et al. 2018) but have not led to disease as reported in this study. In addition, D. australis appears to be attractive habitat for gastropods, with nubbins of D. australis colonised by cowries within 13 days of transplantation to a novel habitat (Larkin et al. 2021b). In the tropics, octocorals are often consumed by fishes, but these bites heal quickly and have not lead to disease, although predation lesions have become locally necrotic (Pratchett 2007; Garra et al. 2020). Conversely, gastropod predation on stony corals has been found to spread disease among colonies (Nicolet et al. 2013, 2018). We did not directly observe predation on D. australis, with only a single gastropod being captured in photos of the damaged colonies and Globovula cavanagh observed laying eggs but not actively feeding, and no fish predation was observed. Previous studies have also not found evidence of predation by Globuvola cavanagh (Corry et al. 2018; Finlay-Jones et al. 2021), although another study observed predation (Larkin et al. 2021b) and other species of egg cowries are known octocoral predators (Bennett 1971; Bowden et al. 1978; Coll et al. 1983; Griffith 1994). It is also possible that the newly hatched cowries may predate on the octocoral tissue, although monitoring of hatching and early behaviour is needed to test this. It is possible that predation made the colonies more susceptible to another disturbance that was not documented, such as a major storm or flood event, or that the colonies were stressed because of early necrotising disease, which made them vulnerable to predation. It is also possible that even though the lesions are consistent with predation, they were early symptoms of the necrotising disease. Further work on the possible interaction between predation and disease by remote-camera surveys (e.g. Losey et al. 1994), regular surveys of colonies and possible predators to detect population spikes and better documentation of the response of D. australis to predation (e.g. Raymundo et al. 2016) would significantly further our understanding of the possible link among predation, disease and population declines in D. australis. Finally, experimental studies to understand how D. australis responds to trauma at the microscopic level (e.g. Rodríguez-Villalobos et al. 2016) would allow for understanding of whether the lesions observed were caused by predation.
Recruitment of new colonies to the study sites at Bare Island began quickly, with new colonies being observed only 10 months after the first observation of disease. Within 15 months of disease observation, large colonies were present and within 18 months, over 35 colonies had grown in the affected area. Other octocoral species recover slowly from disturbance, including the Endangered Mediterranean precious red coral Corallium rubrum, which may take over 30 years to reach pre-disturbance population structure (Tsounis et al. 2006; Bruckner 2009; Montero-Serra et al. 2018). By contrast, soft octocorals such as xeniids and Carijoa spp. grow quickly and can even become invasive (Concepcion et al. 2010; Ruiz Allais et al. 2014; Sánchez and Ballesteros 2014; Ruiz-Allais et al. 2021). Structures that appear to be developing brooding larvae were observed during gross histological analysis (Permata et al. 2000; Marlow and Martindale 2007), and D. australis along with other temperate Dendronephthya breed during the warm months and can reproduce asexually by dropping polyp bundles (Dahan and Benayahu 1997; Hwang and Song 2007; Larkin et al. 2023b). As such, the recovery in population numbers documented in our study may be due to breeding during the austral summer or clonal reproduction. Interestingly, recent work has found that D. australis is likely to broadcast spawn (Larkin et al. 2023b), suggesting that, like Pocillopora spp., D. australis may be capable of multiple modes of reproduction (Smith et al. 2019). In Port Stephens, D. australis releases gametes at neap tide in February and March (Larkin et al. 2023b); so, if colonies at Bare Island follow similar patterns, then many newly recruited colonies surveyed in September of 2020 may be sexual recruits from surrounding non-affected colonies.
Impact of disease on individual corals
Damaged colonies of D. australis sloughed necrotic tissue during collection and histopathology found that the tissue had significant gastrodermal and epidermal necrosis, collapse of gastrovascular canals, and expansion or attenuation of the mesoglea, all of which are likely to have led to loss of regular function. Interestingly, one visibly healthy colony had eosinophilic hyaline membrane, which is abnormal, but was not found in any of the damaged colonies. As observed in M. capitata infected with white syndrome and Sinularia spp. infected with Sinularia tissue loss disease (STLD; Wainwright 2011; Slattery et al. 2013; Work and Meteyer 2014), structures of D. australis became disorganised, took on a ‘shredded’ appearance, and gastrodermal and epidermal cells lost cohesion with connective tissues. In the field, necrosis was clearly present at the centre of infected colonies, but the surrounding tissues appeared unaffected. Under histology, structures of diseased D. australis were damaged and necrotic throughout the slice, suggesting that the disease had travelled throughout the internal structures. Stony coral and gorgonian diseases often spread from a single point to create a lesion (Work and Aeby 2011; Work et al. 2012; Dennis et al. 2020; Sharp et al. 2020). Here, the colonies collected had advanced lesions and their point of origin could not be identified. Previously, similar necrosis and loss of epithelial and gastrodermal cells was found in the hybrid octocoral Sinularia maxima × polydactyla, although tissue that was adjacent to disease lesions maintained its structure (Slattery et al. 2013). Conversely, we found that the interior of colonies was significantly affected by the disease beyond the lesion site. This disease may be difficult to identify in early stages in the field because it appears to target internal structures before external ones, opposite to what has previously been observed in coral diseases.
The D. australis necrotising disease caused significant changes in the tissue composition of the corals that are likely to have affected the ability of the colony to function. The collapse of gastrovascular canals, and overall loss of structure within the colonies, suggests that the disease severely affected the ability of D. australis colonies to circulate water and expand polyps (Davis et al. 2015). In the most severely affected colonies, the gastrovascular canals were no longer distinguishable from other perforate structures (e.g. sclerites) and it is likely that they could not continue their role as the vascular system of the coral. Because these structures are responsible for the extension of coral polyps, and D. australis is entirely heterotrophic, this loss could lead to starvation of the colony (Fabricius and Alderslade 2001; Davis et al. 2015; Mandelberg et al. 2016). Polyp tissues were also affected, with gastrodermal and epidermal cells in the tentacles and digestive systems losing cohesion, often to the point that polyp structures such as the actinopharynx and tentacles could no longer be distinguished. This loss of function would have impeded individual colony recovery because the corals would have been unable to feed even after eliminating the disease.
Potential for natural recovery and restoration
D. australis is distributed in shallow, protected estuaries and bays from Jervis Bay to Port Stephens, NSW. In Port Stephens, where populations are largest, mapping and modelling of appropriate habitat found that D. australis prefers sandy substrates within 6.5 km of the estuary mouth in strong current, in moderate depths of 3–18 m, and with a fairly shallow seafloor slope (Poulos et al. 2016). Although a large portion of the southern shore of Port Stephens meets these criteria, D. australis inhabits only a small portion of habitat with appropriate conditions (Poulos et al. 2016). The habitat in Botany Bay is different from that in Port Stephens in several ways, meaning that the model developed for Port Stephens cannot be extrapolated without modification. In Botany Bay, the colonies were found on large rocky platforms with a thin layer of algal–sediment mat on top. Attempting to remove small colonies from the substrate was not possible and it was concluded that the colonies were attached to the platform below or firmly embedded in the algal matrix. The largest aggregation of D. australis at Botany Bay is the one surveyed in this study at Bare Island, but scattered colonies are often encountered throughout the Bare Island area (R. K. Steinberg and J. Turnbull, pers. obs.). In Port Stephens, all D. australis colonies were associated with sand, sponge and seagrass habitats (Poulos et al. 2016), whereas in Botany Bay, colonies were exclusively found in sponge garden habitat. Survey of the benthic habitat requirements of D. australis across its range and determination of substrate attachment method in each habitat type would allow for modelling of potential D. australis colonies or restoration locations across its range. Root-like processes of Dendronephthya spp. can attach to solid objects and anchor in purely soft sediments (Barneah et al. 2002; Larkin et al. 2023a); however, it is unclear whether one method is preferable over the other for natural or assisted regeneration. Understanding the preferred habitat of this Endangered species outside of Port Stephens would greatly enhance our understanding of their ecology, and could allow for establishing populations in areas of Port Stephens that may be at a lower risk for sand inundation.
Even though Botany Bay populations of D. australis did experience disease and all large colonies were lost, natural recruitment has already begun and, unlike in Port Stephens, the Botany Bay population is not currently likely to require active restoration interventions. At present, it is unclear why the population at Botany Bay is showing signs of natural recovery, whereas the populations in Port Stephens are not, although it may partially be due to the cause of declines. In Port Stephens, declines were caused by sedimentation and smothering (Harasti 2016; Larkin et al. 2021a), which have also severely affected other species elsewhere (Erftemeijer et al. 2012; Jones et al. 2019), whereas in Botany Bay disease appears to have played an important role in declines. Understanding the differences among the characteristics of the environment, D. australis colonies and causes of declines, such as substrate composition, flow regimes, sedimentation rate, larval characteristics, reproductive traits and growth rates, would greatly enhance our understanding of the recovery potential of this charismatic species across its range and allow for modelling of both assisted and unassisted population recovery. Continued monitoring, especially during building and maintenance of marine infrastructure, is also critical to maintaining the health of D. australis populations. Overall, although active restoration interventions are not currently needed at Bare Island, disease outbreaks are expected to become more frequent under climate change (Maynard et al. 2015). As such, understanding disease dynamics and restoration methods for this species is critical for the continued health of populations not only in Botany Bay but across temperate eastern Australia.
Data availability
Histological slides of Dendronephthya australis are freely available on ScienceDB at https://doi.org/10.57760/sciencedb.09644 (Steinberg 2023). This paper forms part of the PhD thesis of Steinberg (2022).
Declaration of funding
This work was funded by an Australian Government Research Training Program scholarship awarded to Rosemary K. Steinberg and by the University of New South Wales.
Acknowledgements
We extend our respects to the Kameygal and Bedegal people who are the traditional owners of the land on which this research was conducted. We thank Fei Shang from the UNSW Biological Specimen Preparation Laboratory for embedding, slicing and staining of samples. We thank Iveta Slapetova and Florence Tomastig from UNSW BMIF for training on the slide scanner and the analysis programs. We thank Talia Stelling-Wood for reading early drafts of the manuscript. We thank Eve Slavich from UNSW Stats Central for her invaluable help with statistical analyses.
References
Bally M, Garrabou J (2007) Thermodependent bacterial pathogens and mass mortalities in temperate benthic communities: a new case of emerging disease linked to climate change. Global Change Biology 13, 2078-2088.
| Crossref | Google Scholar |
Bankhead P, Loughrey MB, Fernández JA, Dombrowski Y, McArt DG, Dunne PD, McQuaid S, Gray RT, Murray LJ, Coleman HG, James JA, Salto-Tellez M, Hamilton PW (2017) QuPath: open source software for digital pathology image analysis. Scientific Reports 7, 16878.
| Crossref | Google Scholar |
Barneah O, Malik Z, Benayahu Y (2002) Attachment to the substrate by soft coral fragments: desmocyte development, structure, and function. Invertebrate Biology 121, 81-90.
| Crossref | Google Scholar |
Barros TL, Bracewell SA, Mayer-Pinto M, Dafforn KA, Simpson SL, Farrell M, Johnston EL (2022) Wildfires cause rapid changes to estuarine benthic habitat. Environmental Pollution 308, 119571.
| Crossref | Google Scholar | PubMed |
Bates D, Mächler M, Bolker B, Walker S (2015) Fitting linear mixed-effects models using lme4. Journal of Statistical Software 67, 1-48.
| Crossref | Google Scholar |
Bowden BF, Coll JC, Hicks W, Kazlauskas R, Mitchell SJ (1978) Studies of Australian soft corals. X. The isolation of epoxyisoneocembrene-A from Sinularia grayi and isoneocembrene-A from Sarcophyton ehrenbergi. Australian Journal of Chemistry 31, 2707-2712.
| Crossref | Google Scholar |
Bracewell SA, Barros TL, Mayer-Pinto M, Dafforn KA, Simpson SL, Johnston EL (2023) Contaminant pulse following wildfire is associated with shifts in estuarine benthic communities. Environmental Pollution 316, 120533.
| Crossref | Google Scholar | PubMed |
Brooks ME, Kristensen K, van Benthem KJ, Magnusson A, Berg CW, Nielsen A, Skaug HJ, Machler M, Bolker BM (2017) glmmTMB balances speed and flexibility among packages for zero-inflated generalized linear mixed modeling. The R Journal 9, 378-400.
| Crossref | Google Scholar |
Bruckner AW (2009) Rate and extent of decline in Corallium (pink and red coral) populations: existing data meet the requirements for a CITES Appendix II listing. Marine Ecology Progress Series 397, 319-332.
| Crossref | Google Scholar |
Bruno JF, Selig ER, Casey KS, Page CA, Willis BL, Harvell CD, Sweatman H, Melendy AM (2007) Thermal stress and coral cover as drivers of coral disease outbreaks. PLoS Biology 5, e124.
| Crossref | Google Scholar | PubMed |
Calderón-Hernández A, Urbina-Villalobos A, Mora-Barboza C, Morales JA, Fernández-García C, Cortés J (2021) Lesions in octocorals of the Costa Rican Caribbean during the 2015–2016 El Niño. Coral Reefs 40, 1167-1179.
| Crossref | Google Scholar |
Campbell AH, Marzinelli EM, Vergés A, Coleman MA, Steinberg PD (2014) Towards restoration of missing underwater forests. PLoS ONE 9, e84106.
| Crossref | Google Scholar |
Case RJ, Longford SR, Campbell AH, Low A, Tujula N, Steinberg PD, Kjelleberg S (2011) Temperature induced bacterial virulence and bleaching disease in a chemically defended marine macroalga. Environmental Microbiology 13, 529-537.
| Crossref | Google Scholar | PubMed |
Coll JC, Tapiolas DM, Bowden BF, Webb L, Marsh H (1983) Transformation of soft coral (Coelenterata: Octocorallia) terpenes by Ovula ovum (Mollusca: Prosobranchia). Marine Biology 74, 35-40.
| Crossref | Google Scholar |
Concepcion GT, Kahng SE, Crepeau MW, Franklin EC, Coles SL, Toonen RJ (2010) Resolving natural ranges and marine invasions in a globally distributed octocoral (genus Carijoa). Marine Ecology Progress Series 401, 113-127.
| Crossref | Google Scholar |
Corry M, Harasti D, Gaston T, Mazumder D, Cresswell T, Moltschaniwskyj N (2018) Functional role of the soft coral Dendronephthya australis in the benthic food web of temperate estuaries. Marine Ecology Progress Series 593, 61-72.
| Crossref | Google Scholar |
Dahan M, Benayahu Y (1997) Clonal propagation by the azooxanthellate octocoral Dendronephthya hemprichi. Coral Reefs 16, 5-12.
| Crossref | Google Scholar |
Davis TR, Harasti D, Smith SDA (2015) Extension of Dendronephthya australis soft corals in tidal current flows. Marine Biology 162, 2155-2159.
| Crossref | Google Scholar |
Davis TR, Harasti D, Smith SDA (2018) Responses of Dendronephthya australis to predation by Dermatobranchus sp. nudibranchs. Marine and Freshwater Research 69, 186-190.
| Crossref | Google Scholar |
Dennis MM, Becker AAMJ, Freeman MA (2020) Pathology of multifocal purple spots, a nonspecific lesion morphology of Caribbean sea fans Gorgonia spp. Diseases of Aquatic Organisms 141, 79-89.
| Crossref | Google Scholar | PubMed |
Epstein HE, Kingsford MJ (2019) Are soft coral habitats unfavourable? A closer look at the association between reef fishes and their habitat. Environmental Biology of Fishes 102, 479–497. 10.1007/s10641-019-0845-4
Erftemeijer PLA, Riegl B, Hoeksema BW, Todd PA (2012) Environmental impacts of dredging and other sediment disturbances on corals: a review. Marine Pollution Bulletin 64, 1737-1765.
| Crossref | Google Scholar | PubMed |
Finlay-Jones H, Raoult V, Harasti D, Gaston TF (2021) What eats a cauliflower coral? An assessment of predation on the endangered temperate soft coral, Dendronepthya australis. Marine and Freshwater Research 73, 307-318.
| Crossref | Google Scholar |
Garra S, Hall A, Kingsford MJ (2020) The effects of predation on the condition of soft corals. Coral Reefs 39, 1329-1343.
| Crossref | Google Scholar |
Gissing A, Timms M, Browning S, Crompton R, McAneney J (2022) Compound natural disasters in Australia: a historical analysis. Environmental Hazards 21, 159-173.
| Crossref | Google Scholar |
Griffith JK (1994) Predation on soft corals (Octocorallia: Alcyonacea) on the Great Barrier Reef, Australia. Marine and Freshwater Research 45, 1281-1284.
| Crossref | Google Scholar |
Harasti D (2016) Declining seahorse populations linked to loss of essential marine habitats. Marine Ecology Progress Series 546, 173-181.
| Crossref | Google Scholar |
Harasti D, Martin-Smith K, Gladstone W (2014) Ontogenetic and sex-based differences in habitat preferences and site fidelity of White’s seahorse Hippocampus whitei. Journal of Fish Biology 85, 1413-1428.
| Crossref | Google Scholar | PubMed |
Hoegh-Guldberg O, Bruno JF (2010) The impact of climate change on the world’s marine ecosystems. Science 328, 1523-1528.
| Crossref | Google Scholar |
Hughes TP (1994) Catastrophes, phase shifts, and large-scale degradation of a Caribbean coral reef. Science 265, 1547-1551.
| Crossref | Google Scholar |
Hwang S-J, Song J-I (2007) Reproductive biology and larval development of the temperate soft coral Dendronephthya gigantea (Alcyonacea: Nephtheidae). Marine Biology 152, 273-284.
| Crossref | Google Scholar |
Jones R, Fisher R, Bessell-Browne P (2019) Sediment deposition and coral smothering. PLoS ONE 14, e0216248.
| Crossref | Google Scholar |
Larkin MF, Davis TR, Harasti D, Cadiou G, Poulos DE, Smith SDA (2021a) The rapid decline of an Endangered temperate soft coral species. Estuarine, Coastal and Shelf Science 255, 107364.
| Crossref | Google Scholar |
Larkin MF, Harasti D, Davis TR, Smith SDA (2021b) If you plant it, they will come: rapid recruitment of habitat-dependent marine invertebrates to transplanted fragments of an endangered soft coral species. Diversity 13, 79.
| Crossref | Google Scholar |
Larkin MF, Davis TR, Harasti D, Benkendorff K, Smith SDA (2023a) Substantial advancement in aquaria rearing methods to assist recovery of an Endangered soft coral. Aquatic Conservation: Marine and Freshwater Ecosystems 33, 1-14.
| Crossref | Google Scholar |
Larkin MF, Davis TR, Harasti D, Smith SDA, Ainsworth TD, Benkendorff K (2023b) A glimmer of hope for an Endangered temperate soft coral: the first observations of reproductive strategies and early life cycle of Dendronephthya australis (Octocorallia: Malacalcyonacea). Marine Biology 170, 146.
| Crossref | Google Scholar |
Losey GS, Balazs GH, Privitera LA (1994) Cleaning symbiosis between the wrasse, Thalassoma duperry, and the green turtle, Chelonia mydas. Copeia 1994, 684-690.
| Crossref | Google Scholar |
Lourie SA, Randall JE (2003) A new pygmy seahorse, Hippocampus denise (Teleostei: Sygnathidae), from the Indo-Pacific. Zoological Studies 42, 284-291.
| Google Scholar |
Maggioni D, Montano S, Voigt O, Seveso D, Galli P (2020) A mesophotic hotel: the octocoral Bebryce cf. grandicalyx as a host. Ecology 101, e02950.
| Crossref | Google Scholar |
Mandelberg Y, Benayahu D, Benayahu Y (2016) Octocoral Sarcophyton auritum Verseveldt & Benayahu, 1978: microanatomy and presence of collagen fibers. The Biological Bulletin 230, 68-77.
| Crossref | Google Scholar | PubMed |
Marlow HQ, Martindale MQ (2007) Embryonic development in two species of scleractinian coral embryos: Symbiodinium localization and mode of gastrulation. Evolution & Development 9, 355-367.
| Crossref | Google Scholar | PubMed |
Maynard J, van Hooidonk R, Eakin CM, Puotinen M, Garren M, Williams G, Heron SF, Lamb J, Weil E, Willis B, Harvell CD (2015) Projections of climate conditions that increase coral disease susceptibility and pathogen abundance and virulence. Nature Climate Change 5, 688-694.
| Crossref | Google Scholar |
Montero-Serra I, Garrabou J, Doak DF, Figuerola L, Hereu B, Ledoux JB, Linares C (2018) Accounting for life-history strategies and timescales in marine restoration. Conservation Letters 11, e12341.
| Crossref | Google Scholar |
Mydlarz LD, Holthouse SF, Peters EC, Harvell CD (2008) Cellular responses in sea fan corals: granular amoebocytes react to pathogen and climate stressors. PLoS ONE 3, e1811.
| Crossref | Google Scholar |
Nicolet KJ, Hoogenboom MO, Gardiner NM, Pratchett MS, Willis BL (2013) The corallivorous invertebrate Drupella aids in transmission of brown band disease on the Great Barrier Reef. Coral Reefs 32, 585-595.
| Crossref | Google Scholar |
Nicolet KJ, Chong-Seng KM, Pratchett MS, Willis BL, Hoogenboom MO (2018) Predation scars may influence host susceptibility to pathogens: evaluating the role of corallivores as vectors of coral disease. Scientific Reports 8, 5258.
| Crossref | Google Scholar |
NSW Fisheries Scientific Committee (2021) Final determination: cauliflower soft coral Dendronephthya australis. (Fisheries Scientific Committee) Available at https://www.dpi.nsw.gov.au/__data/assets/pdf_file/0007/1277782/Final-determination-D.australis-.pdf
Permata WD, Kinzie RA, Hidaka M (2000) Histological studies on the origin of planulae of the coral Pocillopora damicornis. Marine Ecology Progress Series 200, 191-200.
| Crossref | Google Scholar |
Peters EC, Oprandy JJ, Yevich PP (1983) Possible causal agent of ‘white band disease’ in caribbean acroporid corals. Journal of Invertebrate Pathology 41, 394-396.
| Crossref | Google Scholar |
Poore AGB, Watson MJ, de Nys R, Lowry JK, Steinberg PD (2000) Patterns of host use among alga- and sponge-associated amphipods. Marine Ecology Progress Series 208, 183-196.
| Crossref | Google Scholar |
Poulos DE, Harasti D, Gallen C, Booth DJ (2013) Biodiversity value of a geographically restricted soft coral species within a temperate estuary. Aquatic Conservation: Marine and Freshwater Ecosystems 23, 838-849.
| Crossref | Google Scholar |
Poulos DE, Gallen C, Davis T, Booth DJ, Harasti D (2016) Distribution and spatial modelling of a soft coral habitat in the Port Stephens-Great Lakes Marine Park: implications for management. Marine and Freshwater Research 67, 256-265.
| Crossref | Google Scholar |
Pratchett MS (2007) Dietary selection by coral-feeding butterflyfishes (chaetodontidae) on the great barrier reef, Australia. The Raffles Bulletin of Zoology 2014, 171-176.
| Google Scholar |
Qiu Z, Coleman MA, Provost E, Campbell AH, Kelaher BP, Dalton SJ, Thomas T, Steinberg PD, Marzinelli EM (2019) Future climate change is predicted to affect the microbiome and condition of habitat-forming kelp. Proceedings of the Royal Society B: Biological Sciences 286, 20181887.
| Crossref | Google Scholar |
Raymundo LJ, Work TM, Miller RL, Lozada-Misa PL (2016) Effects of Coralliophila violacea on tissue loss in the scleractinian corals Porites spp. depend on host response. Diseases of Aquatic Organisms 119, 75-83.
| Crossref | Google Scholar | PubMed |
Rodríguez-Villalobos JC, Work TM, Calderon-Aguilera LE (2016) Wound repair in Pocillopora. Journal of Invertebrate Pathology 139, 1-5.
| Crossref | Google Scholar | PubMed |
Ruiz Allais JP, Amaro ME, McFadden CS, Halasz A, Benayahu Y (2014) The first incidence of an alien soft coral of the family Xeniidae in the Caribbean, an invasion in eastern Venezuelan coral communities. Coral Reefs 33, 287.
| Crossref | Google Scholar |
Ruiz-Allais JP, Benayahu Y, Lasso-Alcalá OM (2021) The invasive octocoral Unomia stolonifera (Alcyonacea, Xeniidae) is dominating the benthos in the southeastern Caribbean Sea. Memoria de la Fundación La Salle de Ciencias Naturales 79, 63-80.
| Crossref | Google Scholar |
Sánchez JA, Ballesteros D (2014) The invasive snowflake coral (Carijoa riisei) in the tropical eastern Pacific, Colombia. Revista de Biología Tropical 62, 199-207.
| Crossref | Google Scholar |
Sharp W, Maxwell K, Smith K, Hunt J (2020) Investigating the ongoing coral disease outbreak in the Florida Keys: continued SCTLD monitoring at middle and lower Florida Keys, experimental coral restoration of SCTLD-susceptible coral species, and assessing the prevalence of SCTLD on intermediate reef habitat. Florida Department of Environmental Protection Award 24, Florida Fish & Wildlife Conservation Commission.
Slattery M, Renegar DA, Gochfeld DJ (2013) Direct and indirect effects of a new disease of alcyonacean soft corals. Coral Reefs 32, 879-889.
| Crossref | Google Scholar |
Smith HA, Moya A, Cantin NE, van Oppen MJH, Torda G (2019) Observations of simultaneous sperm release and larval planulation suggest reproductive assurance in the coral Pocillopora acuta. Frontiers in Marine Science 6, 362.
| Crossref | Google Scholar |
Steinberg RK (2023) Histological slides of Dendronephthya australis. V2. (Science Data Bank) [Data files, updated 2023-11-03] doi:10.57760/sciencedb.09644
Steinberg RK, Dafforn KA, Ainsworth T, Johnston EL (2020) Know thy anemone: a review of threats to octocorals and anemones and opportunities for their restoration. Frontiers in Marine Science 7, 590.
| Crossref | Google Scholar |
Tracy AM, Weil E, Harvell CD (2018) Octocoral co-infection as a balance between host immunity and host environment. Oecologia 186, 743-753.
| Crossref | Google Scholar | PubMed |
Tracy AM, Weil E, Burge CA (2021) Ecological factors mediate immunity and parasitic co-infection in sea fan octocorals. Frontiers in Immunology 11, 608066.
| Crossref | Google Scholar |
Tsounis G, Rossi S, Gili J-M, Arntz W (2006) Population structure of an exploited benthic cnidarian: the case study of red coral (Corallium rubrum L.). Marine Biology 149, 1059-1070.
| Crossref | Google Scholar |
Wada N, Pollock FJ, Willis BL, Ainsworth T, Mano N, Bourne DG (2016) In situ visualization of bacterial populations in coral tissues: pitfalls and solutions. PeerJ 4, e2424.
| Crossref | Google Scholar |
Wainwright D (2011) Halifax Park/Fly Point sand accumulation study. R.N2128.001.01, prepared for Port Stephens – Great Lakes Marine Park, BMT WBM Pty Ltd, Newcastle, NSW, Australia Available at https://indopacificimages.com/wp-content/uploads/2021/09/Halifax-Fly-Point-Sand-Accumulation-Study.pdf
Wendt PH, van Dolah RF, O’Rourke CB (1985) A comparative study of the invertebrate macrofauna associated with seven sponge and coral species collected from the South Atlantic Bight. Journal of the Elisha Mitchell Scientific Society 101, 187-203.
| Google Scholar |
Wickham H (2011) ggplot2. WIREs Computational Statistics 3, 180-185.
| Crossref | Google Scholar |
Work TM, Aeby GS (2011) Pathology of tissue loss (white syndrome) in Acropora sp. corals from the Central Pacific. Journal of Invertebrate Pathology 107, 127-131.
| Crossref | Google Scholar | PubMed |
Work TM, Aeby GS (2014) Microbial aggregates within tissues infect a diversity of corals throughout the Indo-Pacific. Marine Ecology Progress Series 500, 1-9.
| Crossref | Google Scholar |
Work T, Meteyer C (2014) To understand coral disease, look at coral cells. EcoHealth 11, 610-618.
| Crossref | Google Scholar | PubMed |
Work TM, Russell R, Aeby GS (2012) Tissue loss (white syndrome) in the coral Montipora capitata is a dynamic disease with multiple host responses and potential causes. Proceedings of the Royal Society of London – B. Biological Sciences 279, 4334-4341.
| Crossref | Google Scholar |
Zannella C, Mosca F, Mariani F, Franci G, Folliero V, Galdiero M, Tiscar PG, Galdiero M (2017) Microbial diseases of bivalve mollusks: infections, immunology and antimicrobial defense. Marine Drugs 15, 182.
| Crossref | Google Scholar |