Effects of acclimation temperature and exposure time on the scope for growth of the blackfoot Pāua (Haliotis iris)
Thuy T. Nguyen
A
B
Abstract
Climate change and increased seawater temperatures can greatly affect physiological processes and growth of marine ectotherms, including the blackfoot haliotid, Pāua (Haliotis iris). Scope for growth (SFG) is a laboratory-derived measure of the energy available for growth but this has not been examined in Pāua.
To measure SFG of seasonally collected Pāua and their haemolymph parameters at constant acclimation temperatures of 12, 15, 18 and 21°C for 28 days.
Energy available for growth was measured from kelp food and losses due to respiration and ammonia excretion calculated on Days 1, 14, 21 and 28 of acclimation. Haemolymph parameters were also measured.
After 3 days of acclimation, SFG was highly variable. Following 2 weeks of acclimation, SFG was positive for all temperatures. Respiration and excretion energies depended on both acclimation temperature and time. Haemolymph parameters were affected by acclimation temperature.
Pāua have limited ability to acclimate to a temperature 21°C suggesting that they would not grow effectively at this temperature.
This research suggests that adult Pāua can be adversely affected by increased seawater temperature, resulting from climate change and this could affect their future growth and distribution.
Keywords: acclimation capacity, biochemistry, energy, global warming, haemolymph, Haliotis iris, Pāua, physiology.
Introduction
Global warming is one of the greatest environmental challenges that affect marine organisms and ecosystems (Pachauri et al. 2014). Although increasing temperature is known to significantly affect the activity, physiology and energy balance of marine ectotherms (Bayne and Newell 1983; Cossins and Bowler 1987; Somero 2012), prolonged periods of energy imbalance could affect an organism’s growth, reproduction and performance. Some marine molluscs, however, can adapt, acclimate or acclimatise to changing temperatures, which helps them maintain energy balance (Peck et al. 2014; Mardones et al. 2022). Those species with poor acclimation capacity may be unable to adjust to increased temperatures associated with climate change, global warming and marine heatwaves. Temperature can also affect the feeding rate, the amount of energy absorbed from food, rates of metabolism and ammonia excretion, functions that are used to determine the scope for the growth (SFG) (Hawkins 1995; Britz et al. 1997; Hooper et al. 2007). Within an organisms’ usual temperature range, these rates usually rise with increasing temperature, whereas outside the optimum range, the rates can rapidly reduce (Gillooly et al. 2001; Hernández-Sandoval et al. 2018). SFG has been used to evaluate the available energy for growth and reproduction after fulfilling other necessary biological processes (Genoni and Pahl-Wostl 1991). It is regarded as a good predictor of actual growth in invertebrates and provides a rapid and quantitative assessment of the energy status of organisms (Beiras et al. 1994; Baillieul et al. 1996). Also, SFG has been used to indicate the ability of an organism to cope with future stress (Baillieul et al. 1996). The effects of temperature on SFG has been carried out in several marine molluscs including the juvenile scallops (Argopecten purpuratus; González et al. 2002), the blue mussel (Mytilus edulis; Widdows 1976; Mubiana and Blust 2007), the Red Sea mussel (Brachidontes pharaonis; Sarà et al. 2008) and the marine scavenging gastropod (Nassarius festivus; Zhang et al. 2016). In addition, several studies have investigated the effects of temperature on the growth of abalone species, including South African abalone (Haliotis midae; Britz et al. 1997), blackfoot Pāua (H. iris; Searle et al. 2006) and disc abalone (H. discus hannai) in South Korea (Kong et al. 2017).
Increasing exposure temperature can also affect the haemolymph of marine ectotherms including haemocyanin, protein, sodium and potassium. Haemolymph protein includes haemocyanin, which is a major component, hormones, nutritional peptides and immune components (Fredrick and Ravichandran 2012). In molluscs, haemocyanin transports oxygen and assists in immune responses (Jaenicke et al. 2009). Elevating temperature can raise haemocyanin to transport more oxygen to the tissue, thus increasing haemolymph protein (Behrens et al. 2002; Giomi and Pörtner 2013). Lu et al. (2016) found that temperature affected both haemolymph sodium and potassium concentrations of the gazami crab (Portunus trituberculatus). By contrast, Einarson (1993) found that temperature did not affect haemolymph sodium and potassium concentrations in the amphipod (Gammarus oceanicus).
The abalone (Haliotis iris, hereafter Pāua) is one of three species of haliotid in New Zealand, contributing a large and critical cultural, recreational and economic resource (Prince 2005). They live both in intertidal and subtidal zones with abundant seaweed, their main food resource. Living in coastal zones, they are frequently exposed to daily and seasonal temperature changes. Like other haliotids, Pāua growth depends on size, food, temperature and environment (Stuart and Brown 1994; Steinarsson and Imsland 2003; Bansemer et al. 2016). However, there is no information on the effects of temperature on SFG of this species and associated changes to their haemolymph parameters including ionic concentration, haemocyanin and protein levels. Furthermore, in New Zealand, the current distribution of Pāua extends from Whangarei in the north to Stewart Island in the south, where the average maximum seawater temperatures in summer range between 14.3 and 21.4°C. Along the northern coastline, where average temperatures are ~19°C, populations are often stunted with adults reaching only 90–100-mm shell length, below the value of 125-mm shell length required for legal collection. Stunted populations can also occur elsewhere and arise due to a combination of genetic and environmental factors (Naylor and Andrew 2004; Naylor et al. 2006; Venter et al. 2018). The aims of this study were to measure: (1) SFG of Pāua and their haemolymph parameters at constant acclimation temperatures of 12, 15, 18 and 21°C; and (2) determine the effects of 28 days of acclimation on various physiological parameters. The three lower temperatures selected for this experiment were in the range of sea surface temperature in Christchurch, Canterbury, New Zealand where Pāua were acclimatised (Table 1), including 12°C presenting winter (June to August), 15°C representing spring and autumn (September to November and March to May) and 18°C representing summer (November to February). The acclimation to 21°C has been used in other studies to assess the potential response to increased temperature due to ocean warming as a result of climate change. The Pāua were collected from the field seasonally and thus were seasonally acclimatised close to the new exposure acclimation temperature. The results from this study provide a basic framework for physiological research on Pāua and information about the potential physiological processes to predict the ability of this species to adapt to increased seawater temperatures associated with climate change.
Season | Average seasonal temperature variation (°C) | Minimum and maximum seasonal temperature variation (°C) | Month of collection | Sea temperature in intertidal and subtidal zones at time of collection (°C) | Experimental acclimation temperature (°C) | |
---|---|---|---|---|---|---|
Winter | 9.5 | 6.0–12.0 | June | 10.5 | 12 | |
Spring | 11.9 | 8.2–17.2 | April | 12.7 | 15 | |
Autumn | 14.6 | 10.6–18.8 | November | 15.6 | 18 | |
Summer | 16.7 | 13.4–21.1 | February | 18.7 | 21 |
Materials and methods
Animal collection and acclimation
Pāua of both sexes (80–100 mm in shell length, 124.1 ± 19.9 g) were collected by snorkelling in Little Port Cooper (Lyttelton), Christchurch (S43°613′ E172°816′). The average maximal shell length for this population between 2018 and 2020 was 112.4 mm. Pāua were collected for specific acclimation temperatures close to seasonal values from Lyttelton Harbour (Table 1). They were then transported in a chilly bin in aerated seawater to the aquarium at the University of Canterbury where they were tagged and held in recirculating natural seawater (salinity ~33‰) system for at least 3 days to reduce possible stress. Temperatures during transportation and in the aquarium were maintained similar at temperatures to the original ambient temperature where Pāua were caught (Table 1). After that, Pāua were transferred to control rooms with smaller recirculating seawater systems. Temperatures were raised ~2°C day−1 by adjusting constant temperature rooms until they reached the target water temperatures (i.e. 12, 15, 18 or 21°C). For each acclimation temperature, there were four tank replicates. Each replicate was a 60-L tank (with Pāua) connected to another 60-L tank (without Pāua) by a submersible pump (Moray 1300, 1100 L h−1, China) to maintain nature sea water recirculation. Water from the upper tanks flowed through 50-μm polishing filter pads to trap particles in the water before going to the lower tanks. Air stones were put in the lower tanks to oxygenate the water. The light-dark cycle was 12–12 h. Pāua were fed seaweed (Undaria pinnatifida or Macrocystis pyrifera) at 15% body weight every other day. Salinity, dissolved oxygen, pH and ammonia concentration were monitored and maintained at ~33‰ ± 2, 95% ± 5, 8.2 ± 4 and under 0.25 mg L−1. Water was exchanged for 50% every second day. They were stocked at a rate of 8–10 Pāua per tank.
Experimental design
The experiment was designed to estimate the effects of acclimation at four constant temperatures (12, 15, 18 or 21°C) recorded at four different periods (Table 1) and acclimation times up to 28 days (measured on Days 1, 14, 21 and 28) on physiological responses (absorption efficiency, absorbed energy, respiration energy, ammonia excretion energy and SFG) of Pāua and their haemolymph parameters (haemocyanin, protein, sodium and potassium). A group of 32 Pāua was used for each temperature treatment (except for 18°C where 24 Pāua were used). Eight replicate Pāua were measured for each time interval within each temperature treatment. At 18°C, physiological responses were not measured for the Pāua acclimated on Day 1 because the time and facilities were limited.
Measurement of physiological responses using the energy balance equation and performance was evaluated in terms of scope for growth (SFG):
where Ab is the rate of energy absorbed from food obtained in experiments (J g−1 DW h−1), R is rate of energy lost to respiration obtained in experiments (J g−1 DW h−1), U is a rate of energy lost to ammonia excretion obtained in experiments (J g−1 DW h−1) (Vinberg 1960; Zhang et al. 2016).
Pāua were fasted for 36 h before the experiment and transferred separately into 4-L chambers with window nets for water exchange and fed with 12 g of fresh seaweed (Undaria pinnatifida), which had been cleaned of epiphytes and dried with paper towels. After 24 h, the unconsumed seaweed and faeces were collected separately. Absorption efficiency was evaluated following the method by Conover (1966). The percentage organic content of faeces collected from each of the Pāua was measured. The faeces were collected by filtering water from a vacuum funnel onto 0.45-μm Whatman GF/C. The glass filter papers were then rinsed with distilled water to eliminate the salts. The material on the glass fibre filters was dried to constant weight for 48 h at 55°C, and then ashed at 450°C for 4.5 h to determine the ash-free dry weight of faeces. The unconsumed seaweed was also dried at 55°C for 48 h before ashing at 450°C for 4.5 h to determine the ash-free dry weight of the food.
Absorption efficiency (%) was calculated with the equation:
where F is the fraction lost by ashing uneaten food, and E is the fraction lost by ashing the faeces.
Ab was calculated using the equation:
where rate of food consumption was measured in grams; 1 g dry weight of Undaria pinnatifida was equivalent to 2.37 kcal (9916 J) (Lamare and Wing 2001). Calorific values Calories were converted into joules (1 cal = 4.184 J).
Respiration energy was measured ~1 h after the feeding experiment had been completed. Filtered seawater (0.45 μm) was aerated for at least 1 h to achieve 100% oxygen saturation. Pāua were transferred individually to closed-box cylindrical acrylic respirometers. They were left for 30 min in the respirometers containing saturated filtered seawater to allow them to settle before measurements were taken. The small submersible water pumps (model-04301, 378 L h−1, Taiwan) were set up within the respirometers to ensure constant mixing of seawater. An oxygen electrode (FireSting, Pyroscience, Germany) was housed in a rubber bung inserted into a hole in the lid of each respirometer, connected to a Powerlab 4ST device (ADInstruments, Australia) to record data by using LabChart 7 software (ver. 7.3.8, ADInstruments, see https://www.adinstruments.com/support/downloads/windows/labchart-0). Before the respirometers were closed, all of the air bubbles were eliminated. Two respirometers were placed in 30-L flow through a water bath to maintain temperatures within ±0.2°C of the required setpoint. The dissolved oxygen in the respirometers was kept above 75% to prevent hypoxia from influencing metabolic rates (Harris et al. 1999). The amount of oxygen consumed by each Pāua was calculated as MO2 (μg O2 g−1 h−1), and 1 μg O2 g−1 h−1 was equivalent to the energy loss of 0.01398 J g−1 h−1 (Elliot and Davison 1975).
where a is the oxygen capacitance of water (μmol L−1 mm−1 Hg) (temperature and salinity dependent), ΔPO2 is the change in oxygen partial pressure (mm Hg) over a set period time (t, h), V is the volume of the respirometer (L) (accounting for the volume of the Pāua), and w is the weight of the Pāua (g).
Ammonia excretion energy was determined parallel in individual respiration chambers. Water samples of ~5 mL were collected at the beginning and the end of the period. They were then stored at −80°C until analysis. Ammonia excretion energy was determined using the salicylate method developed by the University of Canterbury. The samples were analysed using a Unicam 8625 UV/Vis spectrophotometer at a wavelength of 650 nm. The ammonia excretion (µg NH4-N g−1 h−1) energy of the sample was calculated using:
where AC is ammonia concentration (μg g−1 h−1), V is the volume of the respirometer (L) (accounting for the volume of the Pāua), and w is the weight of the Pāua (g). 1 μg NH4-N g−1 h−1 was equivalent to the energy loss of 0.02485 J g−1 h−1 (Elliot and Davison 1975).
For determination of the O:N ratio, oxygen consumption and ammonia excretion values were converted into their atomic equivalents (Hawkins et al. 2002):
The O:N ratio between 3 and 16 indicates that metabolism is mainly based on proteins. A ratio from 16 to 60 indicates a mixture of protein and lipid substrate (Mayzaud and Conover 1988).
After measuring the physiological responses, blood samples from Pāua acclimated at 12, 15 and 21°C were collected to measure haemocyanin, haemolymph protein, haemolymph sodium and haemolymph potassium. Approximately 1 mL of haemolymph sample was drawn from the pedal sinus using a 1- or 2-mL syringe and 23-gauge needle. The haemolymph samples were dispensed into 1.5-mL Eppendorf centrifuge tubes, mixed by inversion and centrifuged at 960g for 15 min at 4°C to remove blood cells. The supernatant was transferred into fresh tubes to store frozen at −80°C until analysis. The frozen samples were thawed, mixed and re-centrifuged at 960g for 2 min at 4°C prior to analysis. Pāua were then euthanased by placing them in the freezer before the tissue and shell were separated and dried to constant weight at 45°C for at least 2 days to calculate the soft dried tissue weight (DW).
The haemocyanin samples were diluted 51-fold in aerated buffer EDTA 10 mmol L−1, pH = 8.8–9, in 1-cm2 polystyrene cuvettes. Haemocyanin level was then determined from the absorbance at 346 nm using the Unicam 8625 UV/Vis spectrophotometer with a D2 lamp. Haemocyanin concentrations (Hcy) were calculated using a practical extinction coefficient determined from copper analysis (EmM Hcy, 1 cm = 22.84 for millimolar Hcy measured in a 1-cm cuvette) (Behrens et al. 2002; Ragg and Taylor 2006):
Protein concentrations were also estimated by a similar procedure of Hcy from the absorbance at 280 nm. The haemolymph protein samples were diluted in the same buffer of haemocyanin with a dilution factor of 102 in 1-mL quartz micro cuvettes. Protein concentrations were expressed as grams per litre of equivalent bovine serum albumen (BSA) solution, using the extinction coefficient (E1%, 1 cm = 6.67 for 1% solutions, i.e. 1 g/100 mL, measured in a 1-cm cuvette) (Sterman and Foster 1956; Wang and Annunziata 2007).
Haemolymph sodium (Na+) and potassium (K+) were determined using a classic flame photometer (model 410, Sherwood, UK). For the sodium measurements, 100 μL of haemolymph was diluted 500 times, whereas the haemolymph was diluted by a dilution factor of 60 for the potassium measurements. The flame photometer for Na+ was calibrated using solutions of 0.25, 0.50, 0.75, 1.00 and 1.25 mM. For K+, the flame photometer was calibrated using solutions of 0.05, 0.10, 0.15, 0.20 and 0.25 mM. These standards were used to determine calibration curves, and subsequent calibration equations, which were applied to calculate the ionic results.
Statistical analysis
The R statistical program was used for all analyses (ver. 2021.09.0, R Foundation for Statistical Computing, Vienna, Austria, see https://www.r-project.org/). Two-way ANOVA was used to compare SFG, physiological responses and haemolymph parameters of Pāua between different acclimation temperatures and exposure times. The assumptions of normality and homogeneity of variances were tested for all variables by viewing quantile-quantile normality plots and histograms of the model residuals. If the main effects (acclimation temperature and exposure time) were significant (P ≤ 0.05), then Tukey’s Honest Significant Difference (HSD) post hoc test was applied to detect significant differences between treatment means. When a significant interaction between main effects was observed, plots were used to interpret the interaction effects.
Results
The overall, the survival rate in the experiments was 99.99%. Pāua appeared healthy, showing normal clamping and other behaviours.
Absorption efficiency
Acclimation temperature and exposure time affected absorption efficiency (Fig. 1a). Values were generally above 70%, with the exception being for Pāua 3 days after they had been collected from the field in summer (February). There was significant variation at the start of the acclimation period when the absorption efficiency in the 15°C acclimation being remarkably high (96.4%) and that for the 21°C acclimation was very low (53.4%). From day 14 onwards, there were not many differences in the absorption efficiency of Pāua at different acclimation temperatures. Both acclimation temperature and exposure time affected absorption efficiency, and there was a significant interaction between these factors (P < 0.001, Table 2).
Mean physiological responses and SFG of Pāua acclimated at 12, 15, 18 and 21°C, measured multiple times over an acclimation period of 28 days for (a) absorption efficiencies, (b) absorbed energy, (c) excretion energy, (d) respiration energy, (e) scope for growth, and (f) N:O ratio. Bars depict the mean and error bars are ±s.e. from eight replicates per treatment.
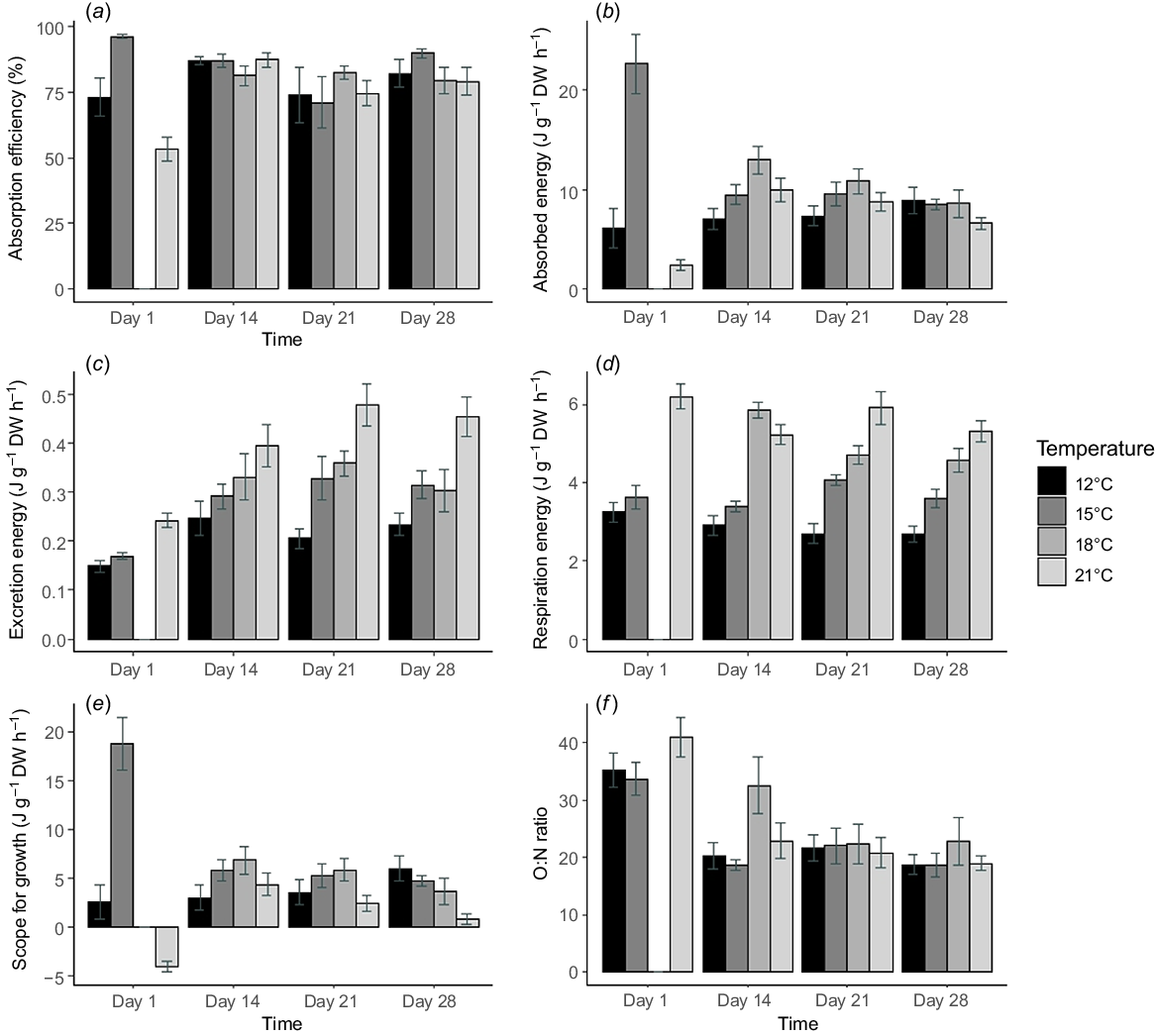
Variable | Temperature effect | Time effect | Interaction | |||||||
---|---|---|---|---|---|---|---|---|---|---|
d.f. | F-value | P | d.f. | F-value | P | d.f. | F-value | P | ||
Absorption efficiency | 3 | 4.97 | 0.003** | 3 | 4.26 | 0.007** | 8 | 4.64 | <0.001*** | |
Ab | 3 | 14.01 | <0.001*** | 3 | 2.28 | 0.84 | 8 | 11.03 | <0.001*** | |
R | 3 | 84.29 | <0.001*** | 3 | 2.67 | 0.05 * | 8 | 1.56 | 0.14 | |
U | 3 | 24.59 | <0.001*** | 3 | 16.42 | <0.001*** | 8 | 1.41 | 0.20 | |
SFG | 3 | 20.64 | <0.001*** | 3 | 1.51 | 0.22 | 8 | 11.59 | <0.001*** | |
O:N ratio | 3 | 0.76 | 0.52 | 3 | 24.34 | <0.001*** | 8 | 1.05 | 0.401 | |
Haemocyanin | 2 | 8.37 | <0.001*** | 3 | 1.54 | 0.21 | 6 | 0.90 | 0.50 | |
Haemolymph protein | 2 | 8.95 | <0.001*** | 3 | 0.39 | 0.76 | 6 | 0.69 | 0.66 | |
Haemolymph sodium | 2 | 36.60 | <0.001*** | 3 | 1.47 | 0.23 | 6 | 1.26 | 0.28 | |
Haemolymph potassium | 2 | 2.95 | 0.06 | 3 | 1.39 | 0.25 | 6 | 0.33 | 0.92 |
SFG equation: SFG = Ab − (R + U), where Ab is the rate of energy absorbed from food obtained in experiments (J g−1 DW h−1), R is the rate of energy lost to respiration obtained in experiments (J g−1 DW h−1), U is the rate of energy lost to ammonia excretion (J g−1 DW h−1). Values shown in bold and with an asterisk indicate that they are statistically significant (*, P ≤ 0.05;, **, P < 0.01; and ***, P < 0.001).
Energy absorbed from food
The energy absorbed from food (J g−1 DW h−1) varied widely on Day 1, with values in the 15°C acclimation 3.7 and 9.6 times higher than that for the 12 and 21°C acclimation treatments respectively. After 2 weeks of acclimation, the absorbed energy was more stable (Fig. 1b). The energy absorption response depended on both acclimation temperature and exposure time, and there was a significant interaction effect between the two factors (P < 0.001, Table 2).
Respiration energy
The energy required for respiration (J g−1 DW h−1) increased with higher temperatures and changed over exposure time. Pāua acclimated to 21°C consumed the highest amount of oxygen at 6.22 ± 0.90 J g−1 DW h−1, whereas that of 12°C was lowest, at 2.77 ± 0.67 J g−1 DW h−1 (Fig. 1d). The values accounted for the highest proportion of energy absorbed, increasing with rising acclimation temperatures of ~36, ~40, ~49 and ~69% at 12, 15, 18 and 21°C respectively. The respiration energy was significantly affected by acclimation temperature and exposure time (P < 0.001, Table 2).
Ammonia excretion energy
The ammonia excretion energy (J g−1 DW h−1) of Pāua increased from Days 1 to 14 before remaining fairly stable until Day 28 (Fig. 2c). During 4 weeks of acclimation, both temperature (P < 0.001, Table 2) and acclimation time (P < 0.001) influenced the ammonia excretion energy significantly, but there was no interaction between them (P < 0.203). However, these values only accounted for a small amount of the absorbed energy, ranging from 2.9 to 5.6%.
Haemolymph responses of Pāua acclimated at 12, 15 and 21°C, measured multiple times over an acclimation period of 28 days for (a) haemocyanin concentration, (b) haemolymph protein, (c) haemolymph sodium and (d) haemolymph potassium. Bars depict the mean and error bars are ±s.e. from eight replicates per treatment.
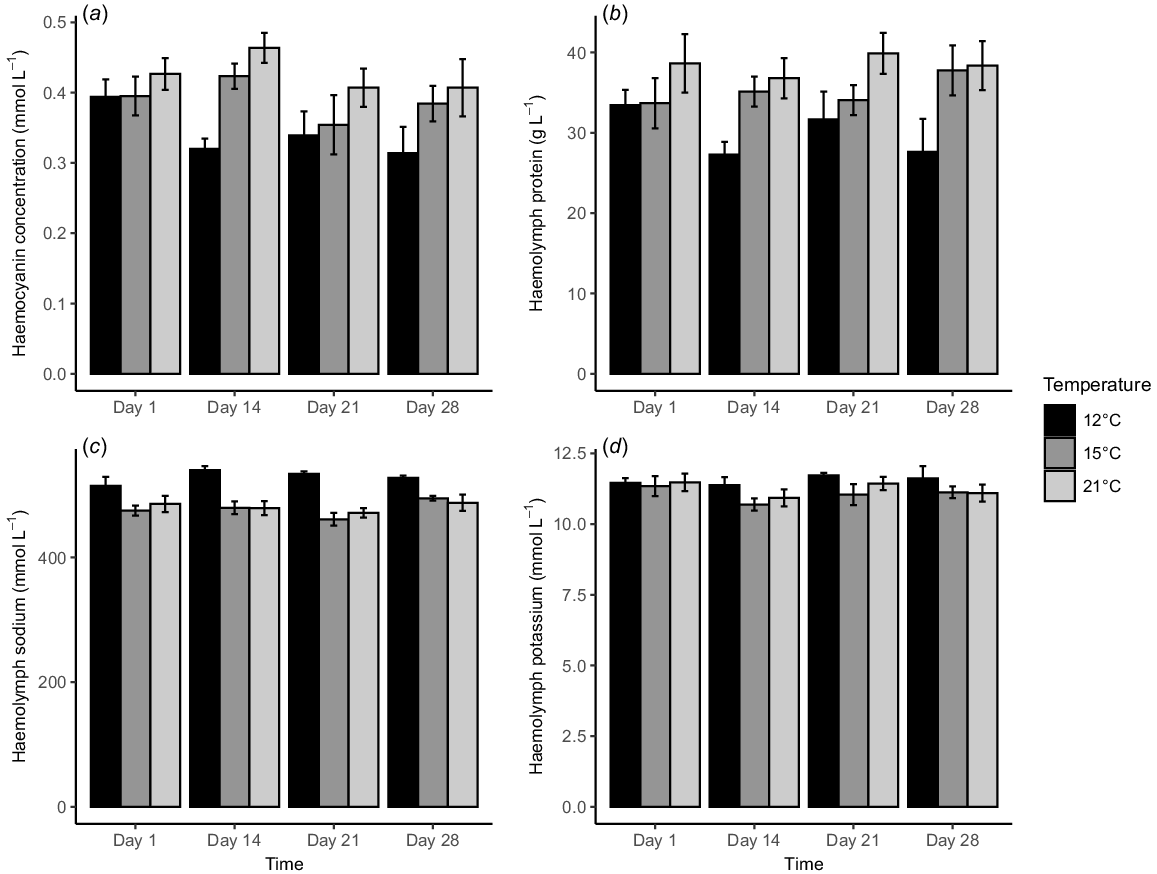
Scope for growth
The SFG (J g−1 DW h−1) of Pāua varied for the different acclimation temperatures and exposure times (Fig. 1e). On Day 1 after collection from the field in spring, the SFG in the 15°C acclimation was very high (18.76 J g−1 DW h−1) and Day 1 values for those collected in summer for the 21°C was negative (−4.10 J g−1 DW h−1). After a 2-week acclimation period, SFG became more stable and the positive SFG values were obtained for Pāua at all acclimation temperatures. The SFG of Pāua acclimated at 21°C was lowest on Day 28. For the 18°C acclimation, SFG was highest on Day 14 and reduced until Day 28. By contrast, the values for 12°C increased during the acclimation period and became highest in all groups on Day 28. The SFG of Pāua acclimated at 15°C remained almost unchanged after 2 weeks’ acclimation. During the 4 weeks, the SFG of Pāua was significantly affected by an interaction between acclimation temperature and exposure time (P < 0.001, Table 2). The significant interaction was due to considerable variation on Day 1.
O:N ratio
After the 2-week acclimation period, the ratio ranged from 18.7 to 32.5, with the highest value at 18°C. This ratio was generally similar between 21 and 28 days (Fig. 1f). Time but not acclimation temperature significantly affected the O:N ratio (P < 0.001, Table 2). The values on Day 1 were significantly higher than those for Days 14, 21 and 28 (Tukey’s HSD test, P < 0.001). All other groups were similar (Tukey’s HSD test, P > 0.05).
Haemocyanin concentration and haemolymph protein
Acclimation temperature and exposure time had similar effects on haemocyanin concentration (mmol L−1) and haemolymph protein (g L−1). They all increased with rising acclimation temperatures, with the highest at 21°C and the lowest at 12°C (Fig. 2a, b). They were affected significantly by acclimation temperature (P < 0.001, Table 2) but not by exposure time (P > 0.05). Also, there was no interaction between the two factors. Haemocyanin concentration at 12°C was significantly lower than 21°C (Tukey’s HSD test, P < 0.001). By contrast, haemolymph protein at 12°C was significantly lower than at 15 and 21°C (Tukey’s HSD test, P < 0.05).
Haemolymph sodium and potassium
Both haemolymph sodium (mmol L−1) and potassium (mmol L−1) were highest at 12°C (Fig. 2c, d). Temperature had a significant effect on the haemolymph sodium (P < 0.001, Table 1), but acclimation time did not (P > 0.001). There was no interaction between the two factors. The levels for Pāua acclimated at 12°C were significantly higher than at other temperatures (Tukey’s HSD test, P < 0.001). However, neither temperature nor time affected haemolymph potassium (P > 0.05, Table 2).
Discussion
The energy relationships of New Zealand Pāua were highly variable for individuals collected from the field and acclimated to four constant temperatures between 12 and 21°C for 28 days. Like other molluscs living in the shallow coastal environment, Pāua are exposed to daily, tidal and seasonal temperature variations and these most likely affect their growth and reproduction. Other variables likely to affect these changes include the availability and quality of food, space requirements and water quality including oxygen levels. Temperature, however, remains a key factor directly and indirectly affecting growth in haliotids (González et al. 2002; Vosloo et al. 2013; Hooper et al. 2014; Kang et al. 2019).
Seasonal physiological responses of field-collected Pāua
It is well known that the physiology of molluscs can show adaptations to seasonal changes in their environment and these results in seasonal differences in reproduction, fecundity and growth (Vilchis et al. 2005; Shin et al. 2020; Oliveira et al. 2021). Such seasonal adjustments have been shown in metabolism, oxygen uptake, ammonia excretion and digestive enzymes (Bayne and Newell 1983). In such studies, the main factors thought to affect these changes include fluctuating or constant exposure temperature, high temperatures or food availability or quality. In the present study, seasonally collected Pāua maintained for 1 day at a constant temperature close to their field temperature had variable feeding, respiration and excretion rates. Pāua collected in spring had the highest energy intake and those collected in summer had the highest energy loss due to respiration. Calculations for the SFG of these Pāua were low for winter collected individuals, highest for spring collected individuals and negative for summer collected individuals. Negative values for SFG have been recorded for other haliotids in other studies (Vosloo and Vosloo 2010). For Pāua these values most likely reflect the field conditions where, during spring, high food availability and temperature conditions close to 15°C are most likely optimal for metabolism, gonad development and spawning. High temperatures over summer are consistent with higher oxygen demands and limited food intake most likely resulting in impaired SFG. If summer collected individuals are not in good physiological health then they may not adapt quickly to laboratory conditions that are two degrees above the seawater temperature at the time of collection.
Absorbed energy and absorption efficiency
For field collected haliotids, absorption efficiencies usually exceed 50% depending on food types (Peck et al. 1987) but in laboratory experiments or in aquaculture, where a single favoured algal food is supplied, the expectation is that absorption efficiency would be higher (Bansemer et al. 2016). In the present study after 2 weeks of acclimation, absorption efficiencies for Pāua were similar to other studies. For example, values for the European abalone H. tuberculata were between 78 and 81% (Peck et al. 1987), for the green abalone, H. fulgens 84.9% (Farías et al. 2003) and for Thai abalone H. asinina, 22–42 mm in length, the average absorption efficiency was 81.04% (Ganmanee et al. 2010).
The energy obtained by grazers from their algal food depends upon its availability, its nutritional value, the feeding rate and the ability of the animal to digest it (Barkai and Griffiths 1987). All of these variables showed variability in the field and linked directly or indirectly with temperature change. The grazing rate of molluscs generally increases with exposure temperature up to a certain limit that is regarded as the optimal temperature. It then declines as in H. midae (Britz et al. 1997). The optimum temperature differs amongst haliotid species depending on species and habitat characteristics. In summer, for H. tuberulata, the amount of energy absorbed from food was greater than H. fulgens but the opposite was found in winter. In the present study, after 2 weeks of acclimation to constant temperatures absorbed energy increased with exposure temperature up to 18°C but decreased in the 21°C acclimation. Following a further 2 weeks of acclimation, the values were broadly similar for all acclimation temperatures. Over the 28-day time period, Pāua acclimated to 12°C had the most stable energy uptake generally increasing over time.
Respiration and ammonia excretion energies
Consistent with numerous other studies on molluscs, the energy consumed in respiration and ammonia excretion energies of Pāua increased markedly with acclimation temperature (McBride et al. 2001; Kang et al. 2019) and also varied over time. The two factors acted independently and values in the current study were generally higher than those from other haliotids. These differences may be due to body size or the exposure temperatures investigated. For H. tuberculata, Lopez and Tyler (2006) found that energy lost due to respiration and ammonia excretion increased greatly with the temperature from 15 to 22°C. They also found that respiration energy depended on size, accounting for 31% of the energy intake for the 10–20-mm length group, and 50.7% for the 50–60-mm group. By contrast, ammonia excretion comprised 0.9% of energy intake for the small up to 3.5% for the larger group.
Barkai and Griffiths (1988) reported that less than 1% of the energy uptake of the South African haliotid (H. midae) was lost by ammonia excretion and ~32% of energy was spent on respiration. Similarly, for Thai abalone H. asinina between 22 and 42 mm, more than 30% of the energy obtained was used for metabolism and from 0.8 to 1.8% spent for ammonia excretion (Ganmanee et al. 2010). In the present study, energy lost by respiration was 36% of intake for animals kept at 12°C, and this increased to over 40% at the higher temperatures of 15 and 18°C. At the highest temperature (21°C) energy lost through respiration was 69%, indicating that this temperature is well above optimal for this species.
For adults of the South African H.midae, Vosloo and Vosloo (2010), acclimation of cold-acclimated individuals to 16, 19 and 22°C for 1 month decreased both their specific oxygen consumption and nitrogen excretion at higher temperatures. This ability to adapt was not the same response recorded for oxygen uptake in Pāua acclimated between 12 and 21°C over a period of 28 days. By contrast, the oxygen uptake of Pāua acclimated to 21°C remained high throughout the acclimation period, confirming the limited ability to adapt to this higher temperature regime.
The O:N ratio is useful in determining the energy sources used in the metabolism of marine ectotherms. In the present study the O:N ratio for field-collected Pāua was between 33 and 41, values similar to H. fulgens, with an average value of 21.6 and 47.0 for large individuals and smaller individuals respectively (Farías et al. 2003). For Pāua, values decreased after 2 weeks of acclimation then remained at levels close to 20 for the following 14 days. Generally, the O:N ratio of Pāua was lower than other haliotids. The O:N ratio of H. discus hannai juveniles was higher than H. iris, ranging from 32.1 ± 1.4 to 38.1 ± 1.8, with a mean of 34.3 ± 0.3 (González et al. 2010). These findings suggest that, in the field, Pāua use both proteins and lipids to produce energy and that during the stable 28-day acclimation period, there was a shift towards utilisation of protein.
Scope for growth
Scope for growth (SFG), the difference between energy gains and losses and the energy available for shell, somatic and reproductive growth, has been used as a predictor of actual growth in many molluscs including bivalves (Widdows and Johnson 1988) but the relationship is less well known for haliotids. For Pāua both acclimation temperature and exposure time had interactive effects on the SFG.
Although Pāua acclimated at 12, 15, 18 and 21°C all had a positive SFG following 2 weeks of acclimation to constant temperatures, the value decreased considerably following 28 days of acclimation at 21°C. This is most likely due to the increased demands of oxygen to maintain metabolism at this temperature (Guppy and Withers 1999; Shao et al. 2015). In the 21°C acclimation, there was a steady decline over the acclimation period indicating that this acclimation temperature is above the optimal level. The positive values for SFG for the 12, 15 and 18°C acclimations suggest these haliotids could grow and mature at these lower seawater temperatures in New Zealand.
SFG values recorded previously for abalone are both higher and lower than those recorded here for Pāua (Ganmanee et al. 2010; González et al. 2010). Some of the differences can be explained by exposure temperature and others on body size; for example for H. asinina, feeding on an artificial diet at 28°C, the SFG increased with size for three size classes between 22- and 42-mm length (approximately 2–25 g) giving SFG estimates between 2.9 and 5.7 J g−1 DW h−1. SFG dependence on size was also found in juvenile green abalone H. fulgens, ranging from 99.8 to 173.7 J abalone−1 day−1 for abalone between 10 and 50 mm at 16°C, using a diet formulation (Farías et al. 2003). Also, the SFG of juvenile European abalone H. tuberculata was 33.9 cal g−1 DW day−1 (~5.91 J g−1 DW h−1) at 15°C when fed on Ulva lactuca (Peck et al. 1987) was a little lower than was found in the current research. The effects of temperature on the SFG have also been investigated in some other species including other molluscs and crustaceans (Yurista 1999; Navarro et al. 2002). These results were consistent with those for Pāua, where, within the optimal temperature range, SFG reached the highest values.
Haemolymph properties
Haemolymph is the circulatory fluid in molluscs transporting oxygen, nutrients, hormones, proteins and immune cells. It contains haemocyanin that accounts for a large proportion of haemolymph proteins (Fredrick and Ravichandran 2012). Both haemolymph haemocyanin and protein levels of Pāua showed a similar response after 14 days of acclimation, with levels increasing with higher acclimation temperatures from 12 to 21°C. The elevation of haemocyanin levels is consistent with the ability of Pāua to maintain high levels of oxygen uptake at temperatures above 20°C. This ability, however, may not be present in juveniles as Wells et al. (1998) reported that aerobic metabolism is limited above 20°C owing to a limitation of their ability for oxygen storage in haemocyanin. These results contrast with research on the marsh isopod Saduria entomon where the haemocyanin concentration remained almost unchanged with increasing temperatures (Borecka et al. 2016).
Total blood protein has been applied as a health status indicator in marine invertebrates (Paschke et al. 2010) and when haemocyanin levels increase due to increased temperatures, haemolymph proteins are also expected to increase. Studies on the effects of heat stress on juvenile haliotids (hybrid Haliotis laevigata × Haliotis rubra) found that haemolymph protein was not significantly affected by increasing temperature from 16 to 26°C after 1 week of acclimation (Hooper et al. 2014). These results suggest that protein regulation in haliotids may differ from other invertebrates.
For Pāua, haemolymph sodium was affected by temperature with the highest values at the lowest acclimation temperature of 12°C. However, haemolymph potassium was similar for Pāua acclimated between 12 and 21°C. Day et al. (2010) found that both haemolymph sodium and potassium of an Australian haliotid increased as exposure temperature decreased. In contrast, in another study on farmed Australian haliotids (hybrid Haliotis laevigata × H. rubra), Hooper et al. (2014), found that haemolymph protein and haemolymph sodium were unaffected by increasing temperature from 16 to 26°C after 1 week of acclimation. The results of that study are similar to the results on the oyster drill (Thais haenzastonza) where Hildreth and Stickle (1980) reported that temperature did not affect haemolymph sodium and potassium of this species.
Ecological context
Like all molluscs, Pāua show seasonal adaptations in their physiology as demonstrated by their patterns of growth and reproduction (Hooker et al. 1997). This is consistent with other haliotids, including those in aquaculture that also show adaptations to multiple stressors including hypoxia, air exposure and elevated temperatures (Rogers-Bennett et al. 2010; Ragg and Watts 2015). Previous research on oxygen uptake in haliotids suggests that the long and short-term responses to elevated temperatures are both species and location specific (Hooper et al. 2014). It also depends on the exposure temperature and whether this is within its normal environmental range.
The laboratory acclimation responses of Pāua are consistent with their seasonal responses and geographical distribution. Because Paūa cannot significantly reduce their energy expenditure due to respiration with increasing temperature they are therefore vulnerable to long periods of seawater temperatures above 18°C. This could induce an energy imbalance at high temperatures, in which the metabolic energy expenses of Pāua exceed their energy gain. In turn, this could affect their growth and reproduction. As a result, Pāua will be likely vulnerable to increasing global warming and marine heatwaves (Morash and Alter 2016; Markle and Kozak 2018).
Some haliotid species appear better able than others to adapt to changing environmental temperatures (Kang et al. 2019). For example, Alter et al. (2017) found that H. laevigata and H. laevigata × H. rubra hybrids have thermal acclimation capacity, but H. rubra do not. In other studies, Barkai and Griffiths (1987), investigated H. midae in South Africa and found that the respiration rate of abalone in the field was highly affected by temperature and did not have an acclimatory adjustment of its metabolism, which rose significantly during the summer. However, studies on the same haliotid suggested this species reduced the specific oxygen consumption and nitrogen excretion as temperature increased (Vosloo and Vosloo 2010). In a later study, Vosloo et al. (2013) suggest that 19°C is the temperature acclimation limit for winter-acclimated H. midae and that above this temperature energy is reallocated to survival mechanisms instead of growth. It follows then that adult Pāua show similar thermal acclimatory responses to juvenile H. midae from South Africa.
The inability of Pāua to reduce energy expenditure at seawater temperatures of 21°C suggests that, in the event of future seawater warming, there is likely to be a southern shift in the distributions to more favourable temperatures. Throughout New Zealand, however, Pāua growth differs with seawater temperatures and on small scales with higher growth on promontories than within bays (Naylor and Andrew 2004; Haist 2018). In addition, ocean warming and marine heatwaves are major threats to marine biodiversity, including effects on primary productivity and kelp forest, which provide food for Pāua (Arafeh-Dalmau et al. 2019; Rogers-Bennett and Catton 2019; Thomsen et al. 2019; Rogers-Bennett et al. 2021). Thus, there may be direct and indirect effects of climate change. The present study highlighted the likely inability of adult Paūa to survive long-term exposure to increased seawater temperatures ~21°C and this vulnerability confirms research by Aalto et al. (2020), which has suggested that, in haliotids, adults are the life stage that is most sensitive to environmental stress. In the current study, if Pāua return back to lower temperatures after a short exposure to 21°C, they may get to recover quickly. This is because they are acclimatised at lower temperature. Therefore, in aquaculture systems for Pāua, adults most likely should managed below 21°C. Also, using high quality foods or varied food sources may help Pāua to survive and grow at elevated temperatures.
Pāua in this study were maintained under the same laboratory conditions with high water quality and enough food. However, space may have been limited in the experimental tanks. Handling could also have resulted in variations of in the physiology of Pāua in this study. Future studies should explore these factors in combination with temperature effects affecting the SFG of Pāua.
In conclusion, the findings from the present study suggest that in the future, if warming seawater temperatures due to climate change result in exceeding 21°C for a long period, this could act as a severe stress to adult Pāua. The results confirm that energy relationships are affected by acclimation temperature and time. At 21°C, energy expenditure exceeded energy uptake suggesting that there would be insufficient energy for growth. Because growth is affected by other factors, then similar studies need to be undertaken using combinations of stressors including reduced oxygen levels and salinity. It would also be useful to examine the responses of juvenile Pāua living in low intertidal habitats with more variable temperature extremes and different food characteristics.
Data availability
The data that support this study will be shared upon reasonable request to the corresponding author.
Declaration of funding
This project was supported by Brian Mason Trust Grant. The project is part of a PhD thesis, funded by a Manaaki New Zealand Scholarship awarded to Thuy Thi Nguyen under the supervision of John Pirker, Islay Marsden and William Davison. The funding bodies and project partners had no role in the design, data collection, analysis or interpretation of data.
Acknowledgements
The authors are thankful for the field support of Renny Bishop, for the technical laboratory support of Johnathan Hill and Jan McKenzie.
References
Aalto EA, Barry JP, Boch CA, Litvin SY, Micheli F, Woodson CB, De Leo GA (2020) Abalone populations are most sensitive to environmental stress effects on adult individuals. Marine Ecology Progress Series 643, 75-85.
| Crossref | Google Scholar |
Alter K, Andrewartha SJ, Morash AJ, Clark TD, Hellicar AD, León RI, Elliott NG (2017) Hybrid abalone are more robust to multi-stressor environments than pure parental species. Aquaculture 478, 25-34.
| Crossref | Google Scholar |
Arafeh-Dalmau N, Montaño-Moctezuma G, Martinez JA, Beas-Luna R, Schoeman DS, Torres-Moye G (2019) Extreme marine heatwaves alter kelp forest community near its equatorward distribution limit. Frontiers in Marine Science 6, 499.
| Crossref | Google Scholar |
Baillieul M, Selens M, Blust R (1996) Scope for growth and fitness of daphnia magna in salinity-stressed conditions. Functional Ecology 10(2), 227-233.
| Crossref | Google Scholar |
Bansemer MS, Qin JG, Harris JO, Duong DN, Hoang TH, Howarth GS, Stone DAJ (2016) Growth and feed utilisation of greenlip abalone (Haliotis laevigata) fed nutrient enriched macroalgae. Aquaculture 452, 62-68.
| Crossref | Google Scholar |
Barkai R, Griffiths CL (1987) Consumption, absorption efficiency, respiration and excretion in the South African abalone Haliotis midae. South African Journal of Marine Science 5(1), 523-529.
| Crossref | Google Scholar |
Barkai R, Griffiths CL (1988) An energy budget for the South African abalone Haliotis midae Linnaeus. Journal of Molluscan Studies 54(1), 43-51.
| Crossref | Google Scholar |
Bayne BL, Newell RC (1983) Physiological energetics of marine molluscs. In ‘The Mollusca’. (Eds ASM Saleuddin, KM Wilbur) pp. 407–515. (Academic Press: Cambridge, MA, USA) doi:10.1016/B978-0-12-751404-8.50017-7
Behrens JW, Elias JP, Taylor HH, Weber RE (2002) The archaeogastropod mollusc Haliotis iris: tissue and blood metabolites and allosteric regulation of haemocyanin function. Journal of Experimental Biology 205, 253-263.
| Crossref | Google Scholar | PubMed |
Beiras R, Camacho AP, Albentosa M (1994) Comparison of the scope for growth with the growth performance of Ostrea edulis seed reared at different food concentrations in an open-flow system. Marine Biology 119(2), 227-233.
| Crossref | Google Scholar |
Borecka A, Janas U, Kendzierska H (2016) The combined effect of temperature and salinity changes on osmoregulation and haemocyanin concentration in Saduria entomon (Linnaeus, 1758). Marine Biology Research 12(3), 316-322.
| Crossref | Google Scholar |
Britz PJ, Hecht T, Mangold S (1997) Effect of temperature on growth, feed consumption and nutritional indices of Haliotis midae fed a formulated diet. Aquaculture 152(1–4), 191-203.
| Crossref | Google Scholar |
Conover RJ (1966) Assimilation of organic matter by zooplankton. Limnology and Oceanography 11(3), 338-345.
| Crossref | Google Scholar |
Day R, Hooper C, Benkendorff K, Slocombe R, Handlinger J (2010) Investigations on the immunology of stressed abalone. Project Number 2004/233. (University of Melbourne: Melbourne, Vic., Australia) Available at https://www.frdc.com.au/sites/default/files/products/2004-233-DLD.pdf
Einarson S (1993) Effects of temperature, seawater osmolality and season on oxygen consumption and osmoregulation of the amphipod Gammarus oceanicus. Marine Biology 117(4), 599-606.
| Crossref | Google Scholar |
Elliot JM, Davison W (1975) Energy equivalents of oxygen consumption in animal energetics. Oecologia 201, 195-201.
| Crossref | Google Scholar |
Farías A, García-Esquivel Z, Viana MT (2003) Physiological energetics of the green abalone, Haliotis fulgens, fed on a balanced diet. Journal of Experimental Marine Biology and Ecology 289(2), 263-276.
| Crossref | Google Scholar |
Fredrick WS, Ravichandran S (2012) Hemolymph proteins in marine crustaceans. Asian Pacific Journal of Tropical Biomedicine 2(6), 496-502.
| Crossref | Google Scholar | PubMed |
Ganmanee M, Sirirustananun N, Jarayabhand P (2010) Energy budget of the thai abalone Haliotis asinina reared in a semiclosed recirculating land-based system. Journal of Shellfish Research 29(3), 637-642.
| Crossref | Google Scholar |
Genoni GP, Pahl-Wostl C (1991) Measurement of scope for change in ascendency for short-term assessment of community stress. Canadian Journal of Fisheries and Aquatic Sciences 48, 968-974.
| Crossref | Google Scholar |
Gillooly JF, Brown JH, West GB, Savage VM, Charnov EL (2001) Effects of size and temperature on metabolic rate. Science 293, 2248-2251.
| Crossref | Google Scholar | PubMed |
Giomi F, Pörtner H-O (2013) A role for haemolymph oxygen capacity in heat tolerance of eurythermal crabs. Frontiers in Physiology 4, 110.
| Crossref | Google Scholar |
González ML, López DA, Pérez MC, Castro JM (2002) Effect of temperature on the scope for growth in juvenile scallops Argopecten purpuratus (Lamark, 1819). Aquaculture International 10(4), 339-348.
| Crossref | Google Scholar |
González GG, Brokordt KB, Winkler FE (2010) Repeatability of physiological traits in juvenile Pacific abalone, Haliotis discus hannai. Marine Biology 157(10), 2195-2203.
| Crossref | Google Scholar |
Guppy M, Withers P (1999) Metabolic depression in animals: physiological perspectives and biochemical generalizations. Biological Reviews 74(1), 1-40.
| Crossref | Google Scholar | PubMed |
Haist V (2018) Analysis of paua maturity and growth. New Zealand Fisheries Assessment Report 2018/21. Available at https://fs.fish.govt.nz/Doc/24606/FAR-2018-21-Paua-Maturity-and-Growth.pdf.ashx
Harris JO, Maguire GB, Edwards SJ, Johns DR (1999) Low dissolved oxygen reduces growth rate and oxygen consumption rate of juvenile greenlip abalone, Haliotis laevigata Donovan. Aquaculture 174, 265-278.
| Crossref | Google Scholar |
Hawkins AJS (1995) Effects of temperature change on ectotherm metabolism and evolution: metabolic and physiological interrelations underlying the superiority of multi-locus heterozygotes in heterogeneous environments. Journal of Thermal Biology 20(1–2), 23-33.
| Crossref | Google Scholar |
Hawkins AJS, Duarte P, Fang JG, Pascoe PL, Zhang JH, Zhang XL, Zhu MY (2002) A functional model of responsive suspension-feeding and growth in bivalve shellfish, configured and validated for the scallop Chlamys farreri during culture in China. Journal of Experimental Marine Biology and Ecology 281, 13-40.
| Crossref | Google Scholar |
Hernández-Sandoval P, Díaz F, Re-Araujo AD, López-Sánchez JA, Martínez-Valenzuela MDC, García-Guerrero M, Rosas C (2018) Thermal preference, critical thermal limits, oxygen routine consumption and active metabolic scope of Macrobrachium tenellum (Smith, 1871) maintained at different acclimation temperatures. Latin American Journal of Aquatic Research 46(3), 558-569.
| Crossref | Google Scholar |
Hildreth JE, Stickle WB (1980) The effects of temperature and salinity on the osmotic composition of the Southern oyster drill, Thais Haemastoma. The Biological Bulletin 159(1), 148-161.
| Crossref | Google Scholar |
Hooker SH, Creese RG, Jeffs AG (1997) Growth and demography of paua Haliotis iris (Mollusca: Gastropoda) in northeastern New Zealand. Molluscan Research 18, 299-311.
| Crossref | Google Scholar |
Hooper C, Day R, Slocombe R, Handlinger J, Benkendorff K (2007) Stress and immune responses in abalone: limitations in current knowledge and investigative methods based on other models. Fish & Shellfish Immunology 22(4), 363-379.
| Crossref | Google Scholar | PubMed |
Hooper C, Day R, Slocombe R, Benkendorff K, Handlinger J, Goulias J (2014) Effects of severe heat stress on immune function, biochemistry and histopathology in farmed Australian abalone (hybrid Haliotis laevigata × Haliotis rubra). Aquaculture 432, 26-37.
| Crossref | Google Scholar |
Jaenicke E, Fraune S, May S, Irmak P, Augustin R, Meesters C, Decker H, Zimmer M (2009) Is activated hemocyanin instead of phenoloxidase involved in immune response in woodlice? Developmental & Comparative Immunology 33(10), 1055-1063.
| Crossref | Google Scholar | PubMed |
Kang HY, Lee Y-J, Lee Y-J, Song W-Y, Kim T-I, Lee W-C, Kim TY, Kang C-K (2019) Physiological responses of the abalone Haliotis discus hannai to daily and seasonal temperature variations. Scientific Reports 9(1), 8019.
| Crossref | Google Scholar |
Kong N, Liu X, Li J, Mu W, Lian J, Xue Y, Li Q (2017) Effects of temperature and salinity on survival, growth and DNA methylation of juvenile Pacific abalone, Haliotis discus hannai Ino. Chinese Journal of Oceanology and Limnology 35(5), 1248-1258.
| Crossref | Google Scholar |
Lamare MD, Wing SR (2001) Calorific content of New Zealand marine macrophytes. New Zealand Journal of Marine and Freshwater Research 35, 335-341.
| Crossref | Google Scholar |
Lopez LM, Tyler P (2006) Energy budget of cultured female abalone Haliotis tuberculata (L.). Journal of Shellfish Research 25(2), 385-389.
| Crossref | Google Scholar |
Lu Y, Wang F, Li L, Dong S (2016) Responses of metabolism and haemolymph ions of swimming crab Portunus trituberculatus to thermal stresses: a comparative study between air and water. Aquaculture Research 47(9), 2989-3000.
| Crossref | Google Scholar |
Mardones ML, Thatje S, Fenberg PB, Hauton C (2022) The short and long-term implications of warming and increased sea water pCO2 on the physiological response of a temperate neogastropod species. Marine Biology 169,, 3.
| Crossref | Google Scholar |
Markle TM, Kozak KH (2018) Low acclimation capacity of narrow-ranging thermal specialists exposes susceptibility to global climate change. Ecology and Evolution 8, 4644-4656.
| Crossref | Google Scholar | PubMed |
Mayzaud P, Conover RJ (1988) O:N atomic ratio as a tool to describe zooplankton metabolism. Marine Ecology Progress Series 45, 289-302.
| Crossref | Google Scholar |
McBride SC, Rotem E, Ben-Ezra D, Shpigel M (2001) Seasonal energetics of Haliotis fulgens (Philippi) and Haliotis tuberculata (L.). Journal of Shellfish Research 20(2), 659-665.
| Google Scholar |
Morash AJ, Alter K (2016) Effects of environmental and farm stress on abalone physiology: perspectives for abalone aquaculture in the face of global climate change. Reviews in Aquaculture 8, 342-368.
| Crossref | Google Scholar |
Mubiana VK, Blust R (2007) Effects of temperature on scope for growth and accumulation of Cd, Co, Cu and Pb by the marine bivalve Mytilus edulis. Marine Environmental Research 63(3), 219-235.
| Crossref | Google Scholar | PubMed |
Navarro JM, Leiva GE, Gallardo CS, Varela C (2002) Influence of diet and temperature on physiological energetics of Chorus giganteus (Gastropoda: Muricidae) during reproductive conditioning. New Zealand Journal of Marine and Freshwater Research 36(2), 321-332.
| Crossref | Google Scholar |
Naylor JR, Andrew NL (2004) Productivity and response to fishing of stunted paua stocks. New Zealand Fisheries Assessment Report 2004B1. (Ministry of Fisheries) Available at https://docs.niwa.co.nz/library/public/FAR2004-31.pdf
Naylor JR, Andrew NL, Kim SW (2006) Demographic variation in the New Zealand abalone Haliotis iris. Marine and Freshwater Research 57(2), 215-224.
| Crossref | Google Scholar |
Oliveira GF, Siregar H, Queiroga H, Peteiro LG (2021) Main drivers of fecundity variability of mussels along a latitudinal gradient: lessons to apply for future climate change scenarios. Journal of Marine Science and Engineering 9, 759.
| Crossref | Google Scholar |
Pachauri RK, Allen MR, Barros VR, Broome J, Cramer W, Christ R, Church JA, Clarke L, Dahe Q, Dasgupta P, Dubash NK, Edenhofer O, Elgizouli I, Field CB, Forster P, Friedlingstein P, Fuglestvedt J, Gomez-Echeverri L, Hallegatte S, Hegerl G, Howden M, Jiang K, Jimenez Cisneros B, Kattsov V, Lee H, Mach KJ, Marotzke J, Mastrandrea MD, Meyer L, Minx J, Mulugetta Y, O’Brien K, Oppenheimer M, Pereira JJ, Pichs-Madruga R, Plattner G-K, Pörtner H-O, Power SB, Preston B, Ravindranath NH, Reisinger A, Riahi K, Rusticucci M, Scholes R, Seyboth K, Sokona Y, Stavins R, Stocker TF, Tschakert P, van Vuuren D, van Ypersele J-P (2014) Climate change 2014, synthesis report. Contribution of Working Groups I, II and III to the Fifth Assessment Report of the Intergovernmental Panel on Climate Change. (Intergovernmental Panel on Climate Change: Geneva, Switzerland) Available at https://www.ipcc.ch/site/assets/uploads/2018/02/SYR_AR5_FINAL_full.pdf
Paschke K, Cumillaf JP, Loyola S, Gebauer P, Urbina M, Chimal ME, Pascual C, Rosas C (2010) Effect of dissolved oxygen level on respiratory metabolism, nutritional physiology, and immune condition of southern king crab Lithodes santolla (Molina, 1782) (Decapoda, Lithodidae). Marine Biology 157, 7-18.
| Crossref | Google Scholar |
Peck LS, Culley MB, Helm MM (1987) A laboratory energy budget for the ormer Haliotis tuberculata L. Journal of Experimental Marine Biology and Ecology 106(2), 103-123.
| Crossref | Google Scholar |
Peck LS, Morley SA, Richard J, Clark MS (2014) Acclimation and thermal tolerance in Antarctic marine ectotherms. The Journal of Experimental Biology 217, 16-22.
| Crossref | Google Scholar |
Prince J (2005) Combating the tyranny of scale for Haliotids: micro-management for microstocks. Marine Science 76(2), 557-577 Available at http://researchrepository.murdoch.edu.au/id/eprint/24520.
| Google Scholar |
Ragg NLC, Taylor HH (2006) Oxygen uptake, diffusion limitation, and diffusing capacity of the bipectinate gills of the abalone, Haliotis iris (Mollusca: Prosobranchia). Comparative Biochemistry and Physiology – A. Molecular & Integrative Physiology 143, 299-306.
| Crossref | Google Scholar |
Ragg NLC, Watts E (2015) Physiological indicators of stress and morbidity in commercially handled abalone Haliotis iris. Journal of Shellfish Research 34(2), 455-467.
| Crossref | Google Scholar |
Rogers-Bennett L, Catton CA (2019) Marine heat wave and multiple stressors tip bull kelp forest to sea urchin barrens. Scientific Reports 9(1), 15050.
| Crossref | Google Scholar |
Rogers-Bennett L, Dondanville RF, Moore JD, Vilchis LI (2010) Response of red abalone reproduction to warm water, starvation, and disease stressors: implications of ocean warming. Journal of Shellfish Research 29(3), 599-611.
| Crossref | Google Scholar |
Rogers-Bennett L, Klamt R, Catton CA (2021) Survivors of climate driven abalone mass mortality exhibit declines in health and reproduction following kelp forest collapse. Frontiers in Marine Science 8, 725134.
| Crossref | Google Scholar |
Sarà G, Romano C, Widdows J, Staff FJ (2008) Effect of salinity and temperature on feeding physiology and scope for growth of an invasive species (Brachidontes pharaonis – MOLLUSCA: BIVALVIA) within the Mediterranean sea. Journal of Experimental Marine Biology and Ecology 363(1–2), 130-136.
| Crossref | Google Scholar |
Sea Temperature Info (2022) Christchurch water temperature by month. Available at https://seatemperature.info/new-zealand/christchurch-water-temperature.html [Verified 28 May 2022]
Searle T, Roberts RD, Lokman PM (2006) Effects of temperature on growth of juvenile blackfoot abalone, Haliotis iris Gmelin. Aquaculture Research 37(14), 1441-1449.
| Crossref | Google Scholar |
Shao Y, Li C, Chen X, Zhang P, Li Y, Li T, Jiang J (2015) Metabolomic responses of sea cucumber Apostichopus japonicus to thermal stresses. Aquaculture 435, 390-397.
| Crossref | Google Scholar |
Shin SR, Kim HJ, Lee DH, Kim H, Sohn YC, Kim JW, Lee JS (2020) Gonadal maturation and main spawning period of Haliotis gigantea (Gastropoda: Haliotidae). Development & Reproduction 24(2), 79-88.
| Crossref | Google Scholar | PubMed |
Somero GN (2012) The physiology of global change: linking patterns to mechanisms. Annual Review of Marine Science 4, 39-61.
| Crossref | Google Scholar | PubMed |
Steinarsson A, Imsland AK (2003) Size dependent variation in optimum growth temperature of red abalone (Haliotis rufescens). Aquaculture 224(1–4), 353-362.
| Crossref | Google Scholar |
Sterman MD, Foster JF (1956) Conformation changes in bovine plasma albumin associated with hydrogen ion and urea binding. I. Intrinsic viscosity and optical rotation 1,2. Journal of the American Chemical Society 78(15), 3652-3656.
| Crossref | Google Scholar |
Stuart MD, Brown MT (1994) Growth and diet of cultivated black-footed abalone, Haliotis iris (Martyn). Aquaculture 127(4), 329-337.
| Crossref | Google Scholar |
Thomsen MS, Mondardini L, Alestra T, Gerrity S, Tait L, South PM, Lilley SA, Schiel DR (2019) Local extinction of bull kelp (Durvillaea spp.) due to a marine heatwave. Frontiers in Marine Science 6, 84.
| Crossref | Google Scholar |
Venter L, Loots DT, Vosloo A, Jansen van Rensburg P, Lindeque JZ (2018) Abalone growth and associated aspects: now from a metabolic perspective. Reviews in Aquaculture 10(2), 451-473.
| Crossref | Google Scholar |
Vilchis LI, Tegner MJ, Moore JD, Friedman CS, Riser KL, Robbins TT, Dayton PK (2005) Ocean warming effects on growth, reproduction, and survivorship of southern California abalone. Ecological Applications 15(2), 469-480.
| Crossref | Google Scholar |
Vosloo D, Vosloo A (2010) Response of cold-acclimated, farmed South African abalone (Haliotis midae) to short-term and long-term changes in temperature. Journal of Thermal Biology 35(7), 317-323.
| Crossref | Google Scholar |
Vosloo D, Vosloo A, Morillion EJ, Samuels JN, Sommer P (2013) Metabolic readjustment in juvenile South African abalone (Haliotis midae) acclimated to combinations of temperature and dissolved oxygen levels. Journal of Thermal Biology 38(7), 458-466.
| Crossref | Google Scholar |
Wang Y, Annunziata O (2007) Comparison between Protein-polyethylene Glycol (PEG) interactions and the effect of PEG on protein-protein interactions using the liquid-liquid phase transition. Journal of Physical Chemistry B 111(5), 1222-1230.
| Crossref | Google Scholar | PubMed |
Wells RMG, Baldwin J, Speed SR, Weber RE (1998) Haemocyanin function in the New Zealand abalones Haliotis iris and H. australis: relationships between oxygen-binding properties, muscle metabolism and habitat. Marine and Freshwater Research 49(2), 143-149.
| Crossref | Google Scholar |
Widdows J (1976) Physiological adaptation of Mytilus edulis to cyclic temperatures. Journal of Comparative Physiology 105(2), 115-128.
| Crossref | Google Scholar |
Widdows J, Johnson D (1988) Physiological energetics of Mytilus edulis: scope for growth. Marine Ecology Progress Series 46, 113-121.
| Crossref | Google Scholar |
Yurista PM (1999) Temperature-dependent energy budget of an Arctic Cladoceran, Daphnia middendorffiana. Freshwater Biology 42(1), 21-34.
| Crossref | Google Scholar |
Zhang H, Shin PKS, Cheung SG (2016) Physiological responses and scope for growth in a marine scavenging gastropod, Nassarius festivus (Powys, 1835), are affected by salinity and temperature but not by ocean acidification. ICES Journal of Marine Science 73(3), 814-824.
| Crossref | Google Scholar |