The effects of storm-induced events on the seasonal dynamics of epilithic algal biomass in subtropical mountain streams
Jeng-Wei Tsai A , Yi-Li Chuang B , Zih-Yi Wu B , Mei-Hwa Kuo C and Hsing-Juh Lin A B DA Graduate Institute of Ecology and Evolutionary Biology, China Medical University, Taichung 404, Taiwan.
B Department of Life Sciences and Research Center for Global Change Biology, National Chung Hsing University, Taichung 402, Taiwan.
C Department of Entomology, National Chung Hsing University, Taichung 402, Taiwan.
D Corresponding author. Email: hjlin@dragon.nchu.edu.tw
Marine and Freshwater Research 65(1) 25-38 https://doi.org/10.1071/MF13058
Submitted: 4 March 2013 Accepted: 12 June 2013 Published: 16 August 2013
Abstract
Information concerning the drivers of seasonal variation in algal biomass in subtropical mountain streams is limited. To identify the drivers of biomass dynamics for epilithic algae, a 20-month study was conducted in mountain streams in Taiwan, an area characterised by different levels of riparian vegetation coverage and agricultural activity, in which a process-based model was optimally fit to field data. We found that episodic typhoon-induced floods were the major drivers shaping the seasonal variations in algal biomass. Flow-induced detachment was frequently observed in periods of higher algal biomass. In contrast, an increased flow stimulated algal growth during periods with slower flow rates. Increased temperature stimulated algal growth at sites with an open canopy cover and higher light availability but constrained biomass at sites with dense canopy shading. Overall, scraper biomass exerted less influence on algal biomass than did environmental factors. The effects of grazing were visible only at the pristine, low-stream-order site in winter. The effects of minimal algal biomass required for recovery was comparable to environmental factors only at sites with intermediate canopy cover, moderate discharge, and higher nutrient concentrations. We suggest that agricultural activity and riparian vegetation can affect epilithic algal biomass in subtropical mountain streams.
Additional keywords: agricultural activity, discharge, dynamic model, light availability, riparian vegetation, typhoon, water temperature.
Introduction
Epilithic algae are one of the major primary producers in stream ecosystems. Changes in their community structure, biomass, and rate of primary production play key roles in determining the rate of material and energy flow and the structure of food webs in streams (Minshall 1978). They are also widely used for water quality remediation (Azim et al. 2005) and as indicators for aquatic environmental monitoring because of their high sensitivity to natural variations and anthropogenic disturbances (Hill et al. 2010). Understanding the forces driving the dynamics of epilithic algal communities in their natural environment is of critical importance for the management of stream ecosystems (Azim et al. 2005).
Tropical and subtropical mountain streams are characterised by large-scale meteorological disturbances such as monsoons, rainstorms, and typhoons (Smith et al. 2003). These influences can affect physical and biogeochemical processes, the food-web structure, and ecosystem metabolism (Webster et al. 2005; Huang et al. 2007; Yang et al. 2009). Global warming is expected to change the spatial and temporal patterns of precipitation, to increase the intensity of storms and precipitation, and to reduce the duration of sunshine in tropical and subtropical regions (Kerr 2007; Zhang et al. 2007). Increased precipitation and a greater frequency of disturbance might increase the surface runoff from watersheds to inland aquatic ecosystems such as streams, lakes, and wetlands. Alterations to the functions of ecosystems caused by episodic weather events in tropical and subtropical montane aquatic ecosystems have garnered much recent attention (Tsai et al. 2008; Jones et al. 2009; Jennings et al. 2012).
To date, most studies that have examined the effects of flow regimes on epilithic algae have been carried out in temperate streams or rivers. These studies have primarily focussed on anthropogenic or natural variability in stream and river flows (Biggs 1995; Robinson et al. 2004; Robinson and Uehlinger 2008) or on the interactions of forest cover, watershed land use, and stream water chemistry with biological activities (Carr et al. 2005; Johnson et al. 2007; O’Brien and Wehr 2010). Limited research has simultaneously assessed the contribution of flood disturbance and catchment properties to the periphyton ecology of streams (Biggs 1995). The temporal dynamics of epilithic algae in tropical and subtropical mountain streams, especially those subject to severe episodic events such as typhoons and storms, remain poorly understood (see Yang et al. 2009), and no similar research exists for subtropical mountain streams, particularly for studies using a process-based dynamic model. Changes in the intensity or frequency of tropical storms or typhoons may induce quantitative and qualitative changes in the nutrients supporting the photosynthetic activities of algae and the physical and chemical characteristics of habitats (Tsai et al. 2011; Kohler et al. 2012). Such events could strongly impact the dynamics of epilithic algae.
The spatial distribution, abundance, and species composition of epilithic algal communities are governed by hydrological patterns (Bunn and Arthington 2002; Souchon et al. 2008; Poff and Zimmerman 2010). Intermediate water flow may increase the uptake of nutrients (Biggs et al. 1998; Townsend et al. 2012) and support the recolonisation of epilithic algae (Jørgensen et al. 2000), thus increasing their biomass and/or biodiversity. In addition, very high levels of water flow may eliminate algal cover on the bedrock and sediments, reduce standing stocks, and change community structure in streams and rivers (Robinson and Uehlinger 2008). Surface runoff may increase anthropogenic nutrient loading from watersheds and thus stimulate the growth rate of algae (Lin et al. 2012), whereas terrestrially derived organic matter loading may reduce light availability and thus indirectly reduce algal biomass (Julian et al. 2008). The effects of hydrological variation on epilithic algal communities are thus difficult to predict a priori because they depend on both the discharge conditions and catchment characteristics (Biggs 1995).
While nutrient supply may increase algal biomass, particularly in streams with infrequent floods (Biggs 2000), scrapers may reduce algal biomass (Feminella and Hawkins 1995). A literature survey by Hillebrand (2002) of experiments with factorial and replicated manipulation of scraper access and nutrient supply indicated that scrapers have a greater effect (top-down) on algal biomass than do nutrients (bottom-up), though experimental design could profoundly affect the relative effects. By examining the natural abundance of stable carbon and nitrogen isotopes, it has been demonstrated that diatoms are the major food source for scrapers in some subtropical mountain streams (Chang et al. 2012; Lin et al. 2012). Aquatic insects may limit algal biomass and alter the community structure and may also exert strong indirect effects within food webs and nutrient cycling systems (Holomuzki et al. 2010).
Herein, we report a 20-month study (February 2007–September 2008) of the seasonal dynamics of epilithic algal biomass in subtropical mountain streams located in central Taiwan. These streams were located in the Chichiawan Stream (CCWS) basin and were characterised by different levels of riparian vegetation cover and agricultural activity. This study focussed on (1) determining the seasonal dynamics of the epilithic algal biomass in these subtropical mountain streams, (2) assessing how the storm-induced water flows affected the dynamics of epilithic algal biomass, and (3) examining the effects of different levels of riparian vegetation cover and agricultural activity on the dynamics of epilithic algal biomass via a process-based dynamic model. We anticipated that storm-induced high water flows would be the major driver that shapes the seasonality of epilithic algal biomass. The extent of riparian vegetation coverage and the levels of surrounding agricultural activity would shape the in-stream environment (e.g. discharge, water temperature, nutrient levels, and light availability). Interactions between the physical and chemical properties of stream ecosystems and the physiological traits of algal communities (e.g. flow-induced detachment and the temperature-dependent growth rate) would determine the seasonal growth trajectory for algae at different sites.
Materials and methods
Study site
The CCWS basin is located in the central montane region of Taiwan at 1720–2100 m elevation. The basin contains three third-order streams (Chichiawan (CCWS), Yousheng (YSS), and Kaoshan (KSS) streams) and two second-order streams (Taoshan West (TWS) and Taoshan North (TNS) streams) (Fig. 1). Streambeds were primarily composed of weathered sandstone and slate. The mean annual water temperature was 12°C (monthly averages ranged from 10 to 18°C) (Yu and Lin 2009). Annual precipitation could exceed 1600 mm, and the mean monthly rainfall was typically <100 mm in the dry season (November–April) and frequently exceeded 220 mm in the wet season (May–October). A single typhoon can drop >1000 mm of precipitation on the drainage basin, and each year, on average, 3.4 typhoons directly affect this basin (Typhoon DataBase, Taiwan Central Weather Bureau). During the study period, the basin was affected by four typhoons. These were Sepat and Krosa in August and October of 2007, respectively, and Kalmaegi, and Fung-wong in July of 2008. These typhoons were intense meteorological events and induced rapid flushing in these streams.
![]() |
The CCWS drains a basin of 76 km2 and is 15.3 km long and 3.0–35.0 m wide with a mean gradient of 130 m km–1 (Lin et al. 1990). Bed sediments contain 42% pebbles in the dry season, and 26% cobbles and 21% boulders in the wet season (Yeh 2006). The central reach of the CCWS has been developed for agricultural production and features higher mean temperatures (13°C) and nutrient concentrations. The recorded concentrations of nitrate+nitrite nitrogen (NO3+NO2-N) and total phosphorus (TP) were 4.12 and 0.024 mg L–1, respectively (Yu and Lin 2009). The TWS forms the upper reaches of the CCWS, and the surrounding vegetation consists of pristine riparian forest. The KSS is 10.6 km long with a mean gradient of 140 m km–1 and a catchment area of 40 km2. Streambed composition varied seasonally, consisting mainly of 39% pebbles and 27% cobbles in the dry season and 44% boulders in the wet season. The catchment of the KSS is covered by natural forests, and no agricultural activity occurs along the stream (Yu and Lin 2009; Hsu et al. 2010). The YSS is located downstream of the CCWS and is 11.4 km long with a mean gradient of 68 m km–1 and a catchment area of 31 km2. Its substrate is mainly composed of 39% gravel and 39% pebbles (Yeh 2006). The YSS has been channelised, and the natural riparian vegetation was replaced with farmland in the 1970s. Thus, this stream had the highest mean water temperature (16°C) and nutrient concentrations (the recorded concentrations of NO3+NO2-N and TP were 8.5 and 0.037 mg L–1, respectively) of the streams studied (Yu and Lin 2009).
Five study sites were assigned in the study basin (Fig. 1). Site 1 was located in the TWS and Site 4 in the KSS in the pristine upper reach of the CCWS basin. Both were designated to be reference sites, as the other sites were affected by anthropogenic activities. Site 2 was located in the central reach of the CCWS, Site 3 was in the downstream reach of the CCWS, approaching the confluence of the KSS and CCWS, and Site 5 was in the downstream reach of the YSS before its confluence with the CCWS. Sites were selected to reflect different coverage levels of riparian vegetation and agricultural activity (Table 1).
![]() |
Sample collection and analysis
Environmental data and biological samples were collected at monthly intervals at each site. Canopy cover and epilithic algal biomass values were recorded every two months in the wet season to account for the rapid change in the in-stream environment and every three months in the dry season. Water temperature, pH, conductivity, turbidity, and dissolved oxygen (DO) were measured with a portable sonde (model 6560; YSI Inc., Yellow Springs, OH, USA) at 07:00–10:00 hours on sampling days. Downward-directed, photosynthetically active radiation (PAR) was measured at a land-based weather station situated near Site 2. Water depth and current velocity were recorded with a velocimeter (FlowTracker Handheld-ADV, SonTek/YSI Inc.). Water samples were stored on ice for no longer than two days before being analysed for concentrations of nitrite N, nitrate N, ammonium N (NH4-N), phosphate P (PO4-P), and silica (Si) following methods of the American Public Health Association (Clesceri et al. 1998).
Epilithic algal samples were collected from randomly selected cobbles (n = 5–12) in riffles at each site, as riffles constituted >80% of the total surface area across all sites (Yeh 2006). On each cobble, a 12.5-cm2 steel frame was used to define the sampling area for the algal patch. Four algal patches were scraped off each cobble with a toothbrush, cleaned with 50–100 mL of filtered stream water, and then stored in cool, dark conditions until the laboratory analysis (Yu and Lin 2009). In the laboratory, algal samples were centrifuged for 10 min to concentrate them to 5 mL. Algal samples were then extracted for chlorophyll (chl) a (an index of algal biomass) in 90% acetone for 24 h at 4°C in the dark and analysed spectrophotometrically (Jeffrey and Humphrey 1975). Aquatic insects were collected using a Surber sampler with an area of 30.5 × 30.5 cm and a mesh size of 250 μm. Six random samples from riffles were taken at each site. These were carried back to the laboratory to determine the biomass and to identify organisms to the lowest possible taxonomic level using available keys (Merritt and Cummin 1996; Kawai and Tanida 2005). The trophic model of the CCWS has demonstrated that algivorous insects are the most abundant herbivores (Lin et al. 2012). Herbivorous fish (e.g. the shovelmouth minnow) and other algivorous invertebrates (e.g. snails) were not abundant, and the effects of grazing could be ignored. Only the biomass of algivorous insects (Lin et al. 2012) belonging to the Baetidae, Heptageniidae, Glossosomatidae, and Uenoidae was used for modelling.
Model development
We developed a mechanistic model to assess the key drivers responsible for the seasonal dynamics of epilithic algal biomass at each site based on a process-based model proposed by Uehlinger et al. (1996). To reduce model complexities and parameter uncertainties, basic model assumptions and simplifications made included the following: (1) sampling sites were considered to be independent of each other, although abiotic (nutrient concentrations) and biotic (algal biomass) conditions at one site might be related to conditions at a neighbouring site; (2) modelling was focussed on algal biomass changes that occurred in riffles to reduce the influence of biological and chemical differences between habitat types (e.g. vegetation cover, food-web structure, hydrological patterns, and light regime); and (3) because we attempted to simulate and interpret drivers that dominate the seasonal dynamics of algal biomass, variables included in the model were only those thought to influence the biomass of epilithic algae over monthly to seasonal timescales.
Seasonal dynamics of epilithic algal biomass can be calculated as a dynamic balance between net light- and nutrient-dependent growth, a flow-induced loss process, grazing loss, and natural death (Uehlinger et al. 1996) and was calculated as follows:

where y is the biomass of epilithic algae ((mg Chl a) m–2), t is time (days), μmax is the maximum instantaneous growth rate of algae (day–1), β is the coefficient of the temperature-dependent growth rate of algae (°C–1), T is the mean daily water temperature (°C), T0 is the reference water temperature (°C), Cdet is an empirical detachment coefficient (s m–3 day–1), Q is the measured discharge of the stream (m3 s–1), y0 is the minimal biomass required to recover after floods (mg m–2), m is the natural mortality of algae (%), σ is the scraper foraging efficiency (m2 g–1 day–1), and G is the scraper biomass (g m–2). NR and IR represent the governing equations for nutrient and light limitations, respectively, of algal growth. NR is formulated as the Monod equation (McCarthy 1981) and incorporates Liebig’s law for the colimitation of nitrogen, phosphorus, and silica, as follows:

where N, P, and Si are the waterborne concentrations of nitrate N, phosphate P, and silica (mg L–1), and KN, KP, and KSi are half-saturation coefficients (mg L–1), which refer to the concentrations at which growth rates of epilithic algae are one-half of the maxima for nitrate N, phosphate P, and silica, respectively. The effect of light availability on algal growth caused by riparian canopy shading was formulated as:

where k and λ refer to the shade coefficients (dimensionless), and S is the fraction of shade on the algal mat (%) caused by the riparian canopy (Rutherford and Cuddy 2005).
Model parameterisation and validation
Input parameters of irradiance, temperature, flow rate, canopy cover, biomass of algae and scrapers, and concentrations of nutrients were adapted by the measured seasonal averages. It was not possible to obtain all of the parameters required for the process-based model from field sampling and measurements as sufficient individual field experiments were not available. Some parameters could, however, be estimated from the literature, including the order of magnitude for the parameters for μmax, T0, σ, KN, KP, KSi, k, and λ (Table 2). This study adapted a preliminary dataset to estimate the best-fit values of site-specific unknown model parameters (β, y0, and Cdet) by fitting the proposed model to all of the field data using nonlinear least-squares regression. An initial set of parameters was estimated and then modified to reproduce the observed dynamics of algal biomass at each site. The remaining parameters for each site were carefully and simultaneously calibrated to agree well with the observed data.
![]() |
Validation and calibration of the model were considered successful relative to the field measurements of the temporal profiles of algal biomass at each site if model outputs were within one standard deviation of the measured mean (Jørgensen and Bendoricchio 2001). In addition, the goodness of fit was evaluated using a coefficient of efficiency (EC) (Nash and Sutcliffe 1970), computed as
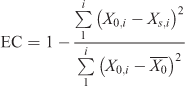
where i denotes the number of measurements, X0,i are the measured data i, and Xs,i is the output result corresponding to data point i. The goal of model calibration was to obtain an EC value as close to 1 as possible.
All modelling was performed using Matlab ver. 6.5 (Mathworks, MA, USA). All steady-state analytical solutions were derived or checked using the appropriate Matlab Symbolic Math Toolbox functions. We could not determine the time-dependent solutions analytically and thus integrated the differential equations numerically in Matlab.
Sensitivity analysis
An analysis of sensitivity was performed to assess the most sensitive components of the algal biomass model (Eqn 1) at each site over the entire study period. It was based on the sensitivity function in Uehlinger et al. (1996) and calculated as , which creates a linear approximation of the change in algal biomass, x, resulting from a 100% change in a selected forcing variable (p) in the algal biomass model (Eqn 1). Larger values of δx, p indicate a higher dependence of x on p, if there is no covariance between the selected p and other forcing variables (Uehlinger et al. 1996).
Statistical analysis
We examined Pearson’s correlations for clarifying the potential forcing variables that were highly correlated in the proposed model (–0.70 > r > 0.70) to avoid covariance between forcing variables and to identify the most significant driver of the algal biomass dynamics accompanying the sensitivity analysis. A paired t-test was used to compare the correlation of sensitivity curves between selected forcing variables. Statistical analysis was conducted using Statistica® software (StatSoft, Tulsa, OK, USA). A P < 0.05 was considered statistically significant.
Results
Seasonal variations in algal biomass and potential drivers
Water temperatures and canopy cover showed clear seasonal fluctuations during the study period. On average, temperatures were ~4°C higher at Site 5 (Fig. 2a), which was characterised by a landscape heavily modified for agricultural activities (Table 1). Canopy cover was highest at the site surrounded by pristine forest (Site 4, 59.5–87.2%) and lowest at the site with the widest open stream channel (Site 2, 3.7–34.0%) (Fig. 2b). Discharge was higher in the wet season (May–October) and lower in the dry season (November–May). Temporal peaks of discharge consistently corresponded to typhoons and rainstorms (Fig. 2c). Typhoons in August and October of 2007 and July of 2008 increased the discharge levels at Sites 4 and 5 by as much as 14–110-fold compared with calm days. High discharge levels quickly returned to normal until the next typhoon. Concentrations of nitrate N and phosphate P were higher at Sites 3 and 5 (Fig. 2d, e), which might have been due to the intensive farming activities along those stream sections. Silica concentrations were generally higher in the wet season (Fig. 2f). The seasonal dynamics of scraper biomass were highly consistent across sites. Scraper biomass peaked in winter, decreased in spring, and was almost absent in summer and autumn (Fig. 3).
![]() |
Algal biomass was characterised by a seasonal pattern corresponding to the seasonal dynamics of flow (Figs 2a and 4). Seasonal trends in algal biomass were similar among Sites 1, 2, 3, and 4 (Fig. 4a–d). Algal biomass remained low during the typhoon season (July–October) (mean algal biomass ranged from 0.81–1.21 mg m–2), increased and peaked in winter (December or January), and then rapidly dropped in early summer (from May–June) (mean algal biomass was 8.24–17.37 mg m–2 during the non-typhoon season) (Fig. 4a–d). Generally, winter peaks of algal biomass at these sites were related to low riparian cover or high light availability and low discharge rates or low detachment loss (Figs 2b, c and 4a–d). In contrast, algal biomass at Site 5 showed distinct seasonal variations. Levels increased from early spring, peaked during early summer, dramatically dropped to low levels during the typhoon season (summer and autumn), and remained at low levels during winter and early spring (Fig. 4e).
Model validation and parameterisation
Model-output values corresponded closely with the temporal trends of the field measurements of epilithic algal biomass at all study sites, with EC values for model performance >0.77 (Fig. 4a–e). Most model outputs were within the error limits (1 standard deviation) for the observed data. Underestimation of algal biomass occurred only in April and July 2007 at Site 3. These results suggest that the algal biomass models were capable of describing the seasonal patterns of epilithic algal biomass caused by various flow disturbances in the CCWS.
Several key processes that drive algal growth, Cdet, y0, and β, were quantified by optimally fitting the model to the measured data in association with known environmental variables and biological parameters (Table 2). The highest value of Cdet (31.9 s m–3 day–1) was observed at Site 5 (Fig. 5a). The highest value of y0 (11.32 mg m–2) was observed at Site 1 and was at least 3 times higher than values at other sites, which ranged from 0.78 to 3.03 mg m–2 (Fig. 5b). We found that the first measurement of algal biomass after the typhoon season at most of the study sites was lower than the estimated y0. β was negative at sites characterised by lower water temperatures and higher canopy shading (Sites 1, 3, and 4) (Figs 2a, b and 5c). In contrast, β was positive at sites with higher temperatures and light availability (Sites 2 and 5) (Figs 2a, b and 5c).
![]() |
Sensitivity analysis
The highest sensitivity function values were observed at Site 5 (436.7 × 103) (Fig. 6e), which featured the most distinctive seasonal variations of algal biomass among all of the study sites (Fig. 4e). Generally, the sensitivity curves for light availability and nutrients were strongly correlated (P < 0.001) at all of the study sites, and the estimated sensitivity function values were similar for light availability (i.e. canopy cover) and nutrient concentrations (Fig. 6a–e), although they were not statistically correlated with each other at most study sites (P > 0.05) (Table 3). This finding suggests that light and nutrients may be colimiting factors on algal growth (Fig. 6a–e). Water temperature positively correlated with canopy cover at all study sites (P = 0.01–0.0001) (Table 3). However, algal growth showed site-specific dependency on changes in temperature. Algal biomass decreased with increasing temperature at sites with denser canopy cover and lower water temperatures (Sites 1 and 4) (Figs 2a, b and 6a, d) but increasing temperature stimulated algal growth at sites with moderate or low riparian forest shading (Sites 2, 3, and 5) (Figs 2b and 6b, c, e). Values of the site-specific sensitivity function for temperature were mainly determined using estimated values of the site-specific and temperature-dependent algal growth rates (β) (Fig. 5c) in Eqn (1).
![]() |
When algal biomass was high, high discharge led to a reduction in biomass, as was observed during the winter peaks at Sites 1–4 and during the summer peaks at Site 5 (Fig. 6a–e). Overall, the effects of flow-induced detachment increased during periods of high algal biomass (Figs 4 and 6). In contrast, sensitivity function values were positively correlated with discharge in the non-typhoon periods, particularly at Site 1, which had the lowest flow rates (Figs 2c and 6a).
For all study sites, biological variables (e.g. scraper biomass and the minimal biomass required to recover) affected algal biomass less than did environmental factors (P > 0.05). Scraper biomass showed a negative correlation with flow rate (r = –0.70, P < 0.05) (Table 3), and no significant difference existed between their sensitivity curves (P > 0.05). A low flow rate in winter facilitated the control of scrapers in algal biomass at the low-stream-order, pristine Site 1 (Fig. 6a). The effects of the minimal biomass required for recovery (y0) were comparable to environmental variables (e.g. light availability, nutrient concentrations, and temperature) only at Site 3 (Fig. 6c), which featured the smallest value of y0 (Fig. 5b) and higher nitrate N and phosphate P concentrations among study sites, as well as intermediate canopy cover and moderate discharge (Fig. 2b–e).
Discussion
Predicting which factors will regulate the structure and function of algal communities remains a challenge for stream ecologists. Stevenson (1997) developed a hierarchical framework of factors affecting benthic algae in streams and postulated that better predictions can result from the understanding that higher-scale factors may constrain the effects of lower-scale factors. In this study, the critical processes shaping seasonal variations in epilithic algal biomass were evaluated by comparing proposed process-based model outputs to measured data. The results of this analysis revealed that episodic floods are the major environmental drivers that shape the seasonal dynamics of epilithic algal biomass. The findings support the hierarchical framework (Stevenson 1997) in these subtropical mountain streams. That is, the effects of water temperature, nutrients, light availability, and grazing on epilithic algal biomass were evident only after accounting for the large-scale constraints of land use, such as the levels of riparian vegetation and agricultural activity. The higher-level constraints on lower-level effects can explain the paradox of the same factor having different effects at different sites. However, our results also suggest that the lower-level physiological properties of algal communities (e.g. flow-induced detachment and temperature-dependent growth rate) should be considered when predicting how epilithic algal biomass reacts to episodic floods.
Drivers of algal biomass dynamics
The distinct seasonal trend in epilithic algal biomass (peaking in the early summer and decreasing during the typhoon season and winter) was observed only at the single site characterised by intensive agricultural activities and a channelised streambed (Site 5). On the other hand, a winter seasonal peak in algal biomass was recorded at other sites (Sites 1–4) that were surrounded by pristine or partially logged forests. To a large degree, higher temperatures (~4°C higher) appear to account for the occurrence of an early summer peak at Site 5, which featured the highest water temperature among the study sites during the spring and summer (Figs 2a and 4e). Thus, the higher growth rate of algae with temperature at Site 5 was reflected in the estimated highest positive values of the temperature constant (β) (Fig. 5c). In contrast, values of β were smaller or even negative at other sites (Fig. 5c), which might explain why there were lower levels of algal biomass in the summer and a buildup of winter biomass. However, high algal biomass (87.9 mg m–2) in the early summer of 2007 could be attributed to the high nitrate N concentration in the water at the intensive agricultural site (Site 5) (Figs 2d and 4e). When compared with the nutrient levels in July 2007, a nitrate N concentration that was ~50% lower and a temperature that was 3°C lower in May 2008 might explain the low algal biomass at Site 5 (10.5 mg m–2). This finding indicates that both higher temperatures and nutrient loading may lead to a high growth rate for epilithic algae in the early summer at the intensive agricultural site (Site 5).
The overall effect of grazing on epilithic algal biomass in the CCWS was minor. This finding was supported by the mixed trophic impacts analysis of the trophic model for the CCWS, which showed that increasing the biomass of aquatic insects would have a minor impact on epilithic algal biomass (Lin et al. 2012). Our sensitivity analysis also demonstrated that the effect of grazing was visible only at the low-stream-order, intermediate-canopy-cover, pristine Site 1 during the winter (Fig. 6a). However, the scraper biomass at Site 1 was not the highest among all of the study sites at that time (Fig. 3). Filamentous green algae are observed more frequently in open-canopy sections of CCWS that have high nitrate N concentrations in the water in the summer (Yu and Lin 2009). Stable isotope analysis has demonstrated that a relatively low proportion of filamentous green algae is being consumed by scrapers (Lin et al. 2012). This finding may explain why the effects of grazing become visible only in the upper reach of the CCWS in winter when diatoms, rather than filamentous green algae, become the dominant taxa in epilithic algal communities. However, the effect of grazing is not visible at Site 4, the other pristine, low-stream-order site, which is likely because the discharge level is higher at Site 4 relative to Site 1. High discharge levels have been shown to be the major driver reducing aquatic insect populations in the CCWS (Chiu et al. 2008).
Effects of flow on the dynamics of algal biomass
Typhoon- and rainstorm-induced events governed the seasonal dynamics of epilithic algal biomass, which explains the period of very low algal biomass during the annual typhoon season (July–October). Mechanical action from extreme floods during these periods reduced the levels of epilithic algal biomass. The highest value of Cdet (31.9 s m–3 day–1) was observed at Site 5 (Fig. 5a), suggesting that algal communities were easily affected by high storm-induced water flow and that flow-induced detachment loss largely accounted for the seasonal patterns of algal biomass. Similar results were reported in other stream and river ecosystems affected by natural and anthropogenic events (Uehlinger 1991; Uehlinger et al. 1996). This result is because shear stresses on the epilithic algal mat from the magnitude of the discharge cause detachment once the flow rate reaches a critical level (Uehlinger 1991; Uehlinger et al. 1996). However, we also found that moderate discharge levels stimulated growth and the accumulation of epilithic algal biomass in spring and early summer, as was the case at sites with slower flow rates (Site 1). The first measurement of algal biomass after the typhoon season at most study sites (Fig. 4a–e) was lower than the estimated value of the minimal biomass required for recovery (y0: Fig. 5b). This result may explain the low recovery rate of epilithic algal biomass at each site before the winter peaks (Fig. 4a–e). We found that moderate water flow stimulated algal growth in the non-typhoon periods, particularly at the site with the lowest flow rate (Site 1). Moderate current speeds might stimulate algal accumulation by reducing the thickness of the diffusion boundary layer and thus improving the uptake of CO2 and nutrients and the removal of oxygen by the algae. This process may make it easier for photosynthetic organisms to reach a higher level of chlorophyll density and biomass (Horner et al. 1990; Biggs et al. 1998; Townsend et al. 2012).
Sensitivity analysis revealed that a more-reliable mechanistic-based model for biomass dynamics during non-typhoon-induced flood periods would require a higher frequency of field sampling, particularly when regional weather patterns drive smaller floods or natural flow variability. Sensitivity function values quantify the extent of algal biomass variation caused by a change in the selected driving force (e.g. temperature, discharge, light intensity, and nutrient concentration). For example, sensitivity function values for Site 5 were ~1–2 orders of magnitude higher than for other sites, especially in periods with the highest biomass in early summer (Fig. 4e). This result may indicate that the sampling interval used might be too long to capture the details of rapid temporal changes in algal biomass before and after the seasonal peaks. An adequate sampling frequency that covers the algal dynamics before and after these critical periods could improve the validity of the proposed model.
Effects of light availability
Our results indicate that light and nutrients may have both limited the growth of epilithic algae at all study sites. Light availability is often the predominant single driver shaping temporal changes in algal biomass and productivity over the short term, especially on a weekly time scale (Robson 2005; O’Brien et al. 2009). This fact is particularly true in comparatively nutrient-poor and unproductive pristine shallow freshwater ecosystems (Karlsson et al. 2009). Because most natural and pristine streams or rivers around the world are shallow with low nutrient concentrations, a high canopy cover, and low phytoplankton biomass, light-limited benthic algae tend to dominate primary production. Light availability is commonly reported to be the dominant limiting factor, rather than nutrients or temperature, in stream and river ecosystems, especially in habitats with subsaturated irradiance (Mosisch et al. 2001; Webster et al. 2005).
In addition to the effects of scouring floods that removed algal biomass, we found that shade from the riparian canopy cover was another key driver of algal growth at all study sites. The narrow active channels and dense canopy cover of most study sites meant that riparian forests influenced light availability, with the exception of Site 2 (Table 1, Fig. 2b). Sensitivity analysis showed that the effects of light availability varied seasonally and were pronounced in the winter, with high levels of algal biomass (Fig. 6a–d). Leaf fall in the winter allowed light to reach the channel surface and had a clear effect on algal communities at most of the study sites. We also found that light availability had a profound effect at sites with higher canopy cover. Growth and production of an algal community might be affected by both short-term irradiance and previously experienced light conditions (O’Brien et al. 2009). The growth of dark-acclimated algae may show increased sensitivity to variations in incidental irradiance than do light-acclimated algal communities (O’Brien et al. 2009). We suggest that direct measurements of the irradiance intensity, the site-specific algal growth rate, and quantitative assessments of the relationship between photosynthesis and irradiance (P–I) are needed to develop a better understanding of how light availability alters the temporal dynamics of algal biomass and to examine the combined effects of light and nutrients on the growth of epilithic algae in streams (Kaiblinger et al. 2007; Torremorell et al. 2009).
Model limitations and application
In this study, a process-based biomass model was constructed and parameterised to generate a first glance at the major mechanisms or processes driving site-specific seasonal dynamics of algal biomass in subtropical mountain streams. However, the capabilities and limitations of the proposed model must be identified before extensive use of the model. This model requires a large number of site- and temporal-specific input parameters, which might not be easily accessible or even available. In general, our model performed well in describing the biomass dynamics of epilithic algae at all of the study sites during the study period. However, it clearly underestimated the 2007 biomass level at Site 3, where concentrations of phosphate P were higher than in the following year. Our model used the Monod equation, with inputs of universal light- and nutrient-utilisation constants (μmax, T0, KN, KP, KSi, k, and λ), to simulate algal biomass changes under various nutrient and light levels. However, the values of these parameters may vary widely among and within algal taxa and may even vary among individual cells as they adapt to surrounding nutrient concentrations or light regimes (Bothwell and Kilroy 2011; Veraart et al. 2008). The performance of this model is expected to improve following the collection of site-, species-, and time-specific physiological and ecological information, which would be vital for more accurate predictions.
In addition to the effects of input parameter quality and availability, appropriate measurement data profiles with an adequate sampling frequency that effectively reflect the quantitative relationships between potential drivers and algal biomass could improve the reliability of the model’s outputs. In general, this model performed well at illustrating site-specific seasonal dynamics of epilithic algae in the CCWS basin. However, a higher sampling frequency (e.g. monthly or weekly) might be required if this model is used to explore additional ecological issues or mechanisms that occur on smaller time scales. Some examples might be algal biomass reactions to, and recovery from, episodic disturbances such as storm-induced floods, dam removal, pulse inflows of high-nutrient runoff from surrounding agricultural land, and the occurrence of benthic algal blooms. In such cases, sampling intervals on a weekly or daily scale would be more appropriate for analysis.
This study presents the numerical effect of storm-induced events on epilithic algal biomass and quantitatively identified site-specific drivers of algal dynamics via a process-based model. The model performed reasonably well in modelling processes that affect epilithic algal biomass, such as interactions between discharge and biomass, underwater light availability and canopy cover, and the transition process of nutrient utilisation by algae characterised by riparian land use. Some effects were minimised in this study, including nutrient releases from sediments and neighbouring areas. It is important to emphasise that the taxonomic and physiognomic structures of epilithic algal species, and thus their biomass dynamics, can be dramatically affected by such processes (Pringle 1987). However, the model is still useful for assessing and predicting the site-specific biomass responses of epilithic algal communities to expected climate or weather scenarios. This study revealed that nutrient levels, water temperature, light availability, and flow rates have important implications for establishing ecological standards for stream, riparian, and watershed ecosystem management. These environmental properties are also important when predicting the impact of temporal and spatial changes in meteorological phenomena, such as precipitation and temperature, on site-specific dynamics of epilithic algal biomass.
Acknowledgements
We thank Ms Mei-Ru Su for assistance with equipment maintenance, data collection, and water chemical analysis. We also thank Ms Hui-Lin Chuang for her assistance in preparing the figures and tables. This work was financially supported by Shei-Pa National Park Headquarters, Miaoli County, Taiwan.
References
Azim, M. E., Verdegem, M. C. J., van Dam, A. A., and Beveridge, M. C. M. (2005). ‘Periphyton: Ecology, Exploitation and Management.’ (CABI: USA.)Biggs, B. J. F. (1995). The contribution of disturbance, catchment geology and landuse to the habitat template of periphyton in stream ecosystems. Freshwater Biology 33, 419–438.
| The contribution of disturbance, catchment geology and landuse to the habitat template of periphyton in stream ecosystems.Crossref | GoogleScholarGoogle Scholar |
Biggs, B. J. F. (2000). Eutrophication of streams and rivers: dissolved nutrient–chlorophyll relationships for benthic algae. Journal of the North American Benthological Society 19, 17–31.
| Eutrophication of streams and rivers: dissolved nutrient–chlorophyll relationships for benthic algae.Crossref | GoogleScholarGoogle Scholar |
Biggs, B. J. F., Goring, D. G., and Nikora, V. I. (1998). Subsidy and stress responses of stream periphyton to gradients in water velocity as a function of community growth. Journal of Phycology 34, 598–607.
| Subsidy and stress responses of stream periphyton to gradients in water velocity as a function of community growth.Crossref | GoogleScholarGoogle Scholar |
Bothwell, M. L., and Kilroy, C. (2011). Phosphorus limitation of the freshwater benthic diatom Didymosphenia geminata determined by the frequency of dividing cells. Freshwater Biology 56, 565–578.
| Phosphorus limitation of the freshwater benthic diatom Didymosphenia geminata determined by the frequency of dividing cells.Crossref | GoogleScholarGoogle Scholar | 1:CAS:528:DC%2BC3MXktVGgu74%3D&md5=cf699228d9a57babd8df52c785a0127dCAS |
Bunn, S. E., and Arthington, A. H. (2002). Basic principles and ecological consequences of altered flow regimes for aquatic biodiversity. Environmental Management 30, 492–507.
| Basic principles and ecological consequences of altered flow regimes for aquatic biodiversity.Crossref | GoogleScholarGoogle Scholar | 12481916PubMed |
Carr, G. M., Chambers, P. A., and Morin, A. (2005). Periphyton, water quality, and land use at multiple spatial scales in Alberta rivers. Canadian Journal of Fisheries and Aquatic Sciences 62, 1309–1319.
| Periphyton, water quality, and land use at multiple spatial scales in Alberta rivers.Crossref | GoogleScholarGoogle Scholar | 1:CAS:528:DC%2BD2MXhtV2itrzI&md5=7d7daff0b6e93bd5387c89fc5be52efaCAS |
Chang, H. H., Wu, S. H., Shao, K. T., Kao, W. Y., Maa, C. J. W., Jan, R. Q., Liu, L. L., Tzeng, C. S., Hwang, J. S., Hsieh, H. L., Kao, S. J., Chen, Y. K., and Lin, H. J. (2012). Longitudinal variation in food sources and their use by aquatic fauna along the length of a subtropical river in Taiwan. Freshwater Biology 57, 1839–1853.
| Longitudinal variation in food sources and their use by aquatic fauna along the length of a subtropical river in Taiwan.Crossref | GoogleScholarGoogle Scholar |
Chiu, M. C., Kuo, M. H., Sun, Y. H., Hong, S. Y., and Kuo, H. C. (2008). Effects of flooding on avian top-predators and their invertebrate prey in a monsoonal Taiwan stream. Freshwater Biology 53, 1335–1344.
| Effects of flooding on avian top-predators and their invertebrate prey in a monsoonal Taiwan stream.Crossref | GoogleScholarGoogle Scholar |
Clesceri, L. S., Greenberg, A. E., and Eaton, A. D. (1998). ‘Standard Methods for the Examination of Water and Wastewater.’ 20th edn. (American Public Health Association: Washington, DC.)
Feminella, J. W., and Hawkins, C. P. (1995). Interactions between stream herbivores and periphyton: a quantitative analysis of past experiments. Journal of the North American Benthological Society 14, 465–509.
| Interactions between stream herbivores and periphyton: a quantitative analysis of past experiments.Crossref | GoogleScholarGoogle Scholar |
Garnier, J., Némery, J., Billen, G., and Théry, S. (2005). Nutrient dynamics and control of eutrophication in the Marne River system: modelling the role of exchangeable phosphorus. Journal of Hydrology 304, 397–412.
| Nutrient dynamics and control of eutrophication in the Marne River system: modelling the role of exchangeable phosphorus.Crossref | GoogleScholarGoogle Scholar | 1:CAS:528:DC%2BD2MXitVGhsLs%3D&md5=d72c1fbe378745fcd7d4d6f4437a161fCAS |
Hill, W. R., Ryon, M. G., Smith, J. G., Adams, S. M., Boston, H. L., and Stewart, A. J. (2010). The role of periphyton in mediating the effects of pollution in a stream ecosystem. Environmental Management 45, 563–576.
| The role of periphyton in mediating the effects of pollution in a stream ecosystem.Crossref | GoogleScholarGoogle Scholar | 20108138PubMed |
Hillebrand, H. (2002). Top-down versus bottom-up control of autotrophic biomass: a meta-analysis on experiments with periphyton. Journal of the North American Benthological Society 21, 349–369.
| Top-down versus bottom-up control of autotrophic biomass: a meta-analysis on experiments with periphyton.Crossref | GoogleScholarGoogle Scholar |
Holomuzki, J. R., Feminella, J. W., and Power, M. E. (2010). Biotic interactions in freshwater benthic habitats. Journal of the North American Benthological Society 29, 220–244.
| Biotic interactions in freshwater benthic habitats.Crossref | GoogleScholarGoogle Scholar |
Horner, R. R., Welch, E. B., Seeley, M. R., and Jacoby, J. M. (1990). Responses of periphyton to changes in current velocity, suspended sediment and phophorus concentration. Freshwater Biology 24, 215–232.
| Responses of periphyton to changes in current velocity, suspended sediment and phophorus concentration.Crossref | GoogleScholarGoogle Scholar |
Hsu, C. B., Tzeng, C. S., Yeh, C. H., Kuan, W. H., Kuo, M. H., and Lin, H. J. (2010). Habitat use by the Formosan landlocked salmon Oncorhynchus masou formosanus. Aquatic Biology 10, 227–239.
| Habitat use by the Formosan landlocked salmon Oncorhynchus masou formosanus.Crossref | GoogleScholarGoogle Scholar |
Huang, I. Y., Lin, Y. S., Chen, C. P., and Hsieh, H. L. (2007). Food web structure of a subtropical headwater stream. Marine and Freshwater Research 58, 596–607.
| Food web structure of a subtropical headwater stream.Crossref | GoogleScholarGoogle Scholar |
Jeffrey, S. W., and Humphrey, G. F. (1975). New spectrophotometric equations for determining chlorophylls a, b, c1, and c2, in higher plants, algae and natural phytoplankton. Biochemie und Physiologie der Pflanzen 167, 191–194.
| 1:CAS:528:DyaE2MXkvFegsLw%3D&md5=6d031de1be80a55c40284c3d96722bbcCAS |
Jennings, E., Jones, S., Arvola, L., Staehr, P. A., Gaiser, E., Jones, I. D., Weathers, K. C., Weyhenmeyer, G. A., Chiu, C. Y., and De Eyto, E. (2012). Effects of weather-related episodic events in lakes: an analysis based on high-frequency data. Freshwater Biology 57, 589–601.
| Effects of weather-related episodic events in lakes: an analysis based on high-frequency data.Crossref | GoogleScholarGoogle Scholar | 1:CAS:528:DC%2BC38XjsVCqurk%3D&md5=8d8dd4e642295c8f44d498c2a78bbddbCAS |
Johnson, T., McNair, J. N., Srivastava, P., and Hart, D. D. (2007). Stream ecosystem responses to spatially variable land cover: an empirically based model for developing riparian restoration strategies. Freshwater Biology 52, 680–695.
| Stream ecosystem responses to spatially variable land cover: an empirically based model for developing riparian restoration strategies.Crossref | GoogleScholarGoogle Scholar |
Jones, S. E., Kratz, T. K., Chiu, C. Y., and McMahon, K. D. (2009). Influence of typhoons on annual CO2 flux from a subtropical, humic lake. Global Change Biology 15, 243–254.
| Influence of typhoons on annual CO2 flux from a subtropical, humic lake.Crossref | GoogleScholarGoogle Scholar |
Jørgensen, S. E., and Bendoricchio, G. (2001). ‘Fundamentals of Ecological Modelling.’ 3rd edn. (Elsevier Science Ltd: Oxford.)
Jørgensen, S. E., Patten, B. C., and Straskraba, M. (2000). Ecosystems emerging: 4. Growth. Ecological Modelling 126, 249–284.
| Ecosystems emerging: 4. Growth.Crossref | GoogleScholarGoogle Scholar |
Julian, J. P., Stanley, E. H., and Doyle, M. W. (2008). Basin-scale consequences of agricultural land use on benthic light availability and primary production along a sixth order temperate river. Ecosystems 11, 1091–1105.
| Basin-scale consequences of agricultural land use on benthic light availability and primary production along a sixth order temperate river.Crossref | GoogleScholarGoogle Scholar |
Kaiblinger, C., Greisberger, S., Teubner, K., and Dokulil, M. T. (2007). Photosynthetic efficiency as a function of thermal stratification and phytoplankton size structure in an oligotrophic alpine lake. Hydrobiologia 578, 29–36.
| Photosynthetic efficiency as a function of thermal stratification and phytoplankton size structure in an oligotrophic alpine lake.Crossref | GoogleScholarGoogle Scholar |
Karlsson, J., Byström, P., Ask, J., Ask, P., Persson, L., and Jansson, M. (2009). Light limitation of nutrient-poor lake ecosystems. Nature 460, 506–509.
| Light limitation of nutrient-poor lake ecosystems.Crossref | GoogleScholarGoogle Scholar | 1:CAS:528:DC%2BD1MXovFymu78%3D&md5=2e97cae524928fe7ce6020f4e9daa518CAS | 19626113PubMed |
Kawai, T., and Tanida, K. (2005). ‘Aquatic Insects of Japan: Manual with Keys and Illustrations.’ (Tokai University Press: Hadano.)
Kerr, R. A. (2007). Global warming is changing the world. Science 316, 188–190.
| Global warming is changing the world.Crossref | GoogleScholarGoogle Scholar | 1:CAS:528:DC%2BD2sXksVajsr8%3D&md5=35bb65c386514972fee9b2cf7ac85498CAS | 17431148PubMed |
Kohler, T. J., Heatherly, T. N., El-Sabaawi, R. W., Zandona, E., Marshall, M. C., Flecker, A. S., Pringle, C. M., Reznick, D. N., and Steven A. Thomas, S. A. (2012). Flow, nutrients, and light availability influence Neotropical epilithon biomass and stoichiometry. Freshwater Science 31, 1019–1034.
| Flow, nutrients, and light availability influence Neotropical epilithon biomass and stoichiometry.Crossref | GoogleScholarGoogle Scholar |
Lin, Y. S., Tsao, S. S., and Chang, K. H. (1990). Population and distribution of the Formosan landlocked salmon (Oncorhynchus masou formosanus) in Chichiawan Stream. Bulletin of the Institute of Zoology, Academia Sinica 29, 73–85.
Lin, H. J., Peng, T. R., Cheng, I. C., Chen, L. W., Kuo, M. H., Tzeng, C. S., Tsai, S. T., Yang, J. T., Wu, S. H., Sun, Y. H., Yu, S. F., and Kao, S. J. (2012). A trophic model of the subtropical headwater stream habitat of the Formosan landlocked salmon. Oncorhynchus formosanus. Aquatic Biology 17, 269–283.
| A trophic model of the subtropical headwater stream habitat of the Formosan landlocked salmon. Oncorhynchus formosanus.Crossref | GoogleScholarGoogle Scholar |
McCarthy, J. J. (1981). The kinetics of nutrient utilization. Canadian Bulletin of Fisheries and Aquatic Sciences 210, 211–233.
Merritt, R. W., and Cummins, K. W. (1996). ‘An Introduction to the Aquatic Insects of North America.’ (Kendall/Hunt Publishing Company: Dubuque, IA.)
Minshall, G. W. (1978). Autotrophy in stream ecosystems. Bioscience 28, 767–771.
| Autotrophy in stream ecosystems.Crossref | GoogleScholarGoogle Scholar |
Mosisch, T. D., Bunn, S. E., and Davies, P. M. (2001). The relative importance of shading and nutrients on algal production in subtropical streams. Freshwater Biology 46, 1269–1278.
| The relative importance of shading and nutrients on algal production in subtropical streams.Crossref | GoogleScholarGoogle Scholar |
Nash, J. E., and Sutcliffe, J. V. (1970). River flow forecasting through conceptual models part I – A discussion of principles. Journal of Hydrology 10, 282–290.
| River flow forecasting through conceptual models part I – A discussion of principles.Crossref | GoogleScholarGoogle Scholar |
O’Brien, P. J., and Wehr, J. D. (2010). Periphyton biomass and ecological stoichiometry in streams within an urban to rural land-use gradient. Hydrobiologia 657, 89–105.
| Periphyton biomass and ecological stoichiometry in streams within an urban to rural land-use gradient.Crossref | GoogleScholarGoogle Scholar | 1:CAS:528:DC%2BC3cXht1WmtbrI&md5=65d591c57a63709a8cf5d09df0a1a796CAS |
O’Brien, K. R., Burford, M. A., and Brookes, J. D. (2009). Effects of light history on primary productivity in a phytoplankton community dominated by the toxic cyanobacterium. Cylindrospermopsis raciborskii. Freshwater Biology 54, 272–282.
| Effects of light history on primary productivity in a phytoplankton community dominated by the toxic cyanobacterium. Cylindrospermopsis raciborskii.Crossref | GoogleScholarGoogle Scholar | 1:CAS:528:DC%2BD1MXjt1CrsLw%3D&md5=862c06e23b8d331d2db172739c1d2dbaCAS |
Poff, N. L., and Zimmerman, J. K. H. (2010). Ecological responses to altered flow regimes: a literature review to inform the science and management of environment flows. Freshwater Biology 55, 194–205.
| Ecological responses to altered flow regimes: a literature review to inform the science and management of environment flows.Crossref | GoogleScholarGoogle Scholar |
Pringle, C. M. (1987). Effects of water and substratum nutrient supplies on lotic periphyton growth: an integrated bioassay. Canadian Journal of Fisheries and Aquatic Sciences 44, 619–629.
| Effects of water and substratum nutrient supplies on lotic periphyton growth: an integrated bioassay.Crossref | GoogleScholarGoogle Scholar | 1:CAS:528:DyaL2sXkvVentr4%3D&md5=a498fdc9beca16754daabf34162a2995CAS |
Robinson, C. T., and Uehlinger, U. (2008). Experimental floods cause ecosystem regime shift in a regulated river. Ecological Applications 18, 511–526.
| Experimental floods cause ecosystem regime shift in a regulated river.Crossref | GoogleScholarGoogle Scholar | 18488612PubMed |
Robinson, C.T., Uehlinger, U., and Monaghan, M.T. (2004). Stream ecosystem response to multiple experimental floods from reservoir. River Research and Application 20, 359–377.
Robson, B. J. (2005). Representing the effects of diurnal variations in light on primary production on a seasonal scale. Ecological Modelling 186, 358–365.
| Representing the effects of diurnal variations in light on primary production on a seasonal scale.Crossref | GoogleScholarGoogle Scholar |
Rosemarin, A. S. (1982). Phosphorus nutrition of two potentially competing filamentous algae, Cladophora glomerata (L.) Kutz. and Stigeoclonium tenue (Agardh) Kutz. from Lake Ontario. Journal of Great Lakes Research 8, 66–72.
| Phosphorus nutrition of two potentially competing filamentous algae, Cladophora glomerata (L.) Kutz. and Stigeoclonium tenue (Agardh) Kutz. from Lake Ontario.Crossref | GoogleScholarGoogle Scholar | 1:CAS:528:DyaL38Xks1SmtbY%3D&md5=a748ad40a0c225459afefd20eab8c4c6CAS |
Rutherford, J. C., and Cuddy, S. M. (2005). Modelling periphyton biomass, photosynthesis and respiration in streams. Technical Report No. 23/05, CSIRO Land and Water, Canberra.
Smith, G. C., Covich, A. P., and Brasher, A. M. D. (2003). An ecological perspective on the biodiversity of tropical island streams. Bioscience 53, 1048–1051.
| An ecological perspective on the biodiversity of tropical island streams.Crossref | GoogleScholarGoogle Scholar |
Souchon, Y., Sabaton, C., Deibel, R., Reiser, D., Kershner, J., Gard, M., Katopodis, C., Leonard, P., Poff, N. L., Miller, W. J., and Lam, B. L. (2008). Detecting biological responses to flow management: missed opportunities; future directions. River Research and Applications 24, 506–518.
| Detecting biological responses to flow management: missed opportunities; future directions.Crossref | GoogleScholarGoogle Scholar |
Stevenson, R. J. (1997). Scale-dependent determinants and consequences of benthic algal heterogeneity. Journal of the North American Benthological Society 16, 248–262.
| Scale-dependent determinants and consequences of benthic algal heterogeneity.Crossref | GoogleScholarGoogle Scholar |
Torremorell, A., Llames, M. E., Perez, G. L., Escaray, R., Bustingorry, J., and Zagarese, H. (2009). Annual patterns of phytoplankton density and primary production in a large, shallow lake: the central role of light. Freshwater Biology 54, 437–449.
| Annual patterns of phytoplankton density and primary production in a large, shallow lake: the central role of light.Crossref | GoogleScholarGoogle Scholar |
Townsend, S. A., Garcia, E. A., and Douglas, M. M. (2012). The response of benthic algal biomass to nutrient addition over a range of current speeds in an oligotrophic river. Freshwater Science 31, 1233–1243.
| The response of benthic algal biomass to nutrient addition over a range of current speeds in an oligotrophic river.Crossref | GoogleScholarGoogle Scholar |
Tsai, J. W., Kratz, T. K., Hanson, P. C., Wu, J. T., Chang, W. Y. B., Arzberger, P. W., Lin, B. S., Lin, F. P., Chou, H. M., and Chiu, C. Y. (2008). Seasonal dynamics, typhoons and the regulation of lake metabolism in a subtropical humic lake. Freshwater Biology 53, 1929–1941.
| Seasonal dynamics, typhoons and the regulation of lake metabolism in a subtropical humic lake.Crossref | GoogleScholarGoogle Scholar | 1:CAS:528:DC%2BD1cXht1entbjM&md5=c049c786bf0a19188b97e610da95c258CAS |
Tsai, J. W., Kratz, T. K., Hanson, P. C., Kimura, N., Liu, W. C., Lin, F. P., Chou, H. M., Wu, J. T., and Chiu, C. Y. (2011). Metabolic changes and the resistance and resilience of a subtropical heterotrophic lake to typhoon disturbance. Canadian Journal of Fisheries and Aquatic Sciences 68, 768–780.
| Metabolic changes and the resistance and resilience of a subtropical heterotrophic lake to typhoon disturbance.Crossref | GoogleScholarGoogle Scholar |
Uehlinger, U. (1991). Spatial and temporal variability of the periphyton biomass in a prealpine river (Necker, Switzerland). Archiv fuer Hydrobiologie 123, 219–237.
Uehlinger, U., Bührer, H., and Reichert, P. (1996). Periphyton dynamics in a floodprone prealpine river: evaluation of significant processes by modeling. Freshwater Biology 36, 249–263.
| Periphyton dynamics in a floodprone prealpine river: evaluation of significant processes by modeling.Crossref | GoogleScholarGoogle Scholar |
Veraart, A. J., Romaní, A. M., Tornés, E., and Sabater, S. (2008). Algal response to nutrient enrichment in forested oligotrophic stream. Journal of Phycology 44, 564–572.
| Algal response to nutrient enrichment in forested oligotrophic stream.Crossref | GoogleScholarGoogle Scholar | 1:CAS:528:DC%2BD1cXoslequ78%3D&md5=0b319ecf26cd2c866a82ff42acff77d0CAS |
Webster, I., Rea, N., Padovan, A., Dostine, P., Townsend, S., and Cook, S. (2005). An analysis of primary production in the Daly River, a relatively unimpacted tropical stream in northern Australia. Marine and Freshwater Research 56, 303–316.
| An analysis of primary production in the Daly River, a relatively unimpacted tropical stream in northern Australia.Crossref | GoogleScholarGoogle Scholar | 1:CAS:528:DC%2BD2MXkslSrtrs%3D&md5=efd17ac52a906b772fef61337df243c4CAS |
Yang, G. Y., Tang, T., and Dudgeon, D. (2009). Spatial and seasonal variations in benthic algal assemblages in streams in monsoonal Hong Kong. Hydrobiologia 632, 189–200.
| Spatial and seasonal variations in benthic algal assemblages in streams in monsoonal Hong Kong.Crossref | GoogleScholarGoogle Scholar |
Yeh, C. H. (2006). Long-term ecological monitoring and ecosystem modeling in the Wuling area – study of channel morphology and physical habitat in Chichiawan Stream. Technical Report. Shei-Pa National Park Administration, Taichung. [In Chinese.]
Yu, S. F., and Lin, H. J. (2009). Effects of agriculture on the abundance and community structure of epilithic algae in mountain streams of subtropical Taiwan. Botanical Studies (Taipei, Taiwan) 50, 73–78.
Zhang, X., Zwiers, F. W., Hegerl, G. C., Lambert, F. H., Gillett, N. P., Solomon, S., Stott, P. A., and Nozawa, T. (2007). Detection of human influence on twentieth-century precipitation trends. Nature 448, 461–465.
| Detection of human influence on twentieth-century precipitation trends.Crossref | GoogleScholarGoogle Scholar | 1:CAS:528:DC%2BD2sXotFaju7w%3D&md5=b99ec1812ff5899c0cc8335664021440CAS | 17646832PubMed |