Do cyanogenic glucosides help sorghum manage a fluctuating nitrogen supply?
Bethany English A # , Alicia A. Quinn A # , Charles R. Warren B , Roslyn M. Gleadow

A
B
C
# These authors contributed equally to this paper
Handling Editor: Ulrike Mathesius
Abstract
Sorghum (Sorghum bicolor [L.] Moench) is an important forage crop that contains the cyanogenic glucoside dhurrin that releases hydrogen cyanide when tissue is damaged. The acyanogenic (dhurrin-free) sorghum line tcd1 was developed to eliminate the risk of cyanide poisoning from sorghum forage. However, dhurrin may also play a role in nitrogen accumulation and storage. We tested whether dhurrin offers the cyanogenic sorghum line BTx623 a growth advantage relative to tcd1, when nitrogen is limiting and variable. BTx623 and tcd1 were grown under two 42-day nitrogen treatments: high dose, low frequency (‘surge’) and low dose, high frequency (‘pulse’). BTx623 exhibited no growth advantage or disadvantage compared to tcd1 under either treatment. Young BTx623 plants had high concentrations of dhurrin for defence but rapidly recycled this during nitrogen deficiency under the surge treatment, demonstrating dhurrin’s role in both defence and nitrogen storage. At later stages, surge plants appeared to accumulate influxes of nitrogen in nitrate and amino acids but not dhurrin. There was evidence of gene expression promoting increased biosynthesis and reduced recycling of dhurrin following surge nitrogen applications but not pulse applications. These results deepen our understanding of dhurrin’s role in nitrogen metabolism and demonstrate tcd1’s potential as a safe forage.
Keywords: crop safety, cyanogenesis, dhurrin, forage, nitrogen metabolism, qPCR, resource accumulation, Sorghum bicolor.
Introduction
Sorghum: a crucial crop with a cost
Sorghum (Sorghum bicolor [L.] Moench) is a C4 cereal crop that originated in Africa but is currently grown worldwide (Ananda et al. 2020). In the African semi-tropics, sorghum is grown primarily for grain and is a crucial staple crop for food security (Mwadalu and Mwangi 2013). In high-income countries, sorghum is typically grown for feed, forage or biofuel (Ramatoulaye et al. 2016). Sorghum requires less water and fertiliser than other cereals such as maize, establishing this as a vital crop for graziers in arid and semi-arid climates, where few other forage crops can survive (Getachew et al. 2016).
Despite the value in food security, sorghum is one of over 3000 cyanogenic plant species that produce potentially hazardous metabolites called cyanogenic glucosides (Gleadow and Møller 2014; Bolarinwa et al. 2016; Wang et al. 2024). Cyanogenic glucosides are stored in plant tissues for defence against herbivory, releasing toxic hydrogen cyanide (HCN) when the tissue is damaged. The risk of cyanide poisoning is of great concern to the agriculture industry and consequently food security, with environmental factors such as climate change and drought potentially exacerbating the risk of cyanide poisoning (Gleadow and Møller 2014). Continued research into cyanogenic glucosides is therefore necessary to mitigate the risk posed.
Concentrations of cyanogenic glucosides can be quantified as HCN potential – the total amount of HCN released per gram of plant tissue consumed. HCN potentials of 0.6 mg g−1 DW and above are considered harmful to cattle (Boyd et al. 1938; Hunt and Taylor 1976), though other species are vulnerable to lower HCN potentials (Kumari et al. 2021). Sorghum produces the cyanogenic glucoside dhurrin and under most conditions, has HCN potential below 0.2 mg g−1. However, HCN potential in sorghum has been found to reach levels above 1 mg g−1, especially when young or exposed to hot, dry conditions (Nelson 1953; Rosenow and Clark 1981; O’Donnell et al. 2013).
Cyanogenic glucosides within resource storage theory
According to Chapin et al. (1990), resource storage in plants can be split into three categories: accumulation, reserves and defence. Accumulation occurs when resource supply exceeds the quantity needed for growth, resulting in the accumulation of storage compounds at no cost to growth. Reserves are storage molecules that are produced despite resources being limiting, at a short-term cost to growth. Defence compounds can be classified as storage molecules if these can be recycled to boost growth when necessary. Thus, biosynthesis and recycling of defences are regulated in a trade-off with growth when resources are limiting (Herms and Mattson 1992). The balance of such a trade-off is dependent on the plant in question, environmental conditions and resource availability.
Cyanogenic glucosides appear to fulfil the dual defence and resource storage role as described by Chapin et al. (1990). These compounds contain nitrogen and glucose, both of which are commonly limiting resources for plant growth. However, experimentally identifying reduced growth caused by cyanogenic glucoside production has proven difficult (Gleadow and Møller 2014). Gruss et al. (2022) recently found that a dhurrin-free sorghum mutant (SbEMS2447 cyp79a1) had a larger green plant area than the wildtype in the absence of herbivory but this result has not been observed in other dhurrin-free or low-dhurrin mutants (Sohail et al. 2020). In sorghum, dhurrin concentrations peak in young plants (4–12 days post-germination) (Busk and Møller 2002; Bjarnholt et al. 2018; Cowan et al. 2021; Myrans and Gleadow 2022), when plant biomass is small and damage is proportionally more costly (McKey 1974; McCall and Fordyce 2010). As sorghum continues to grow, dhurrin concentration gradually declines (O’Donnell 2012; Miller et al. 2014; Emendack et al. 2017; Cowan et al. 2021), with biosynthesis slowing and some dhurrin being recycled (Pičmanová et al. 2015; Bjarnholt et al. 2018). Recycling is therefore considered to alleviate the long-term allocation costs of producing dhurrin for defence (Møller 2010; Neilson et al. 2013; Pičmanová et al. 2015; Bjarnholt et al. 2018).
While there is evidence that cyanogenic glucosides are defences that can be recycled (Bjarnholt et al. 2018; Siegień et al. 2021), whether plants can also synthesise cyanogenic glucosides as a form of resource accumulation when an influx of nitrogen is supplied remains unknown (Blomstedt et al. 2018; Sohail et al. 2020). The development of an acyanogenic sorghum line, tcd1 (Blomstedt et al. 2012), provides an opportunity to test this knowledge gap. tcd1 contains a mutation in the gene for CYP79A1, a key enzyme in the dhurrin biosynthesis pathway, rendering the enzyme inactive (Blomstedt et al. 2012). tcd1 also has great potential in agricultural applications, with the negligible dhurrin concentrations meaning this could serve as a safe forage crop, as long as the growth rate is comparable to that of the wildtype and the associated nitrate concentrations are not above the safe threshold of 2 mg g−1 (Stuart 2012).
Aims and hypotheses
This study explores the roles of dhurrin in S. bicolor, assessing whether dhurrin is biosynthesised as a nitrogen accumulation mechanism when nitrogen is limiting and variable. This was carried out by applying the same total amount of nitrogen at two different frequencies: (1) a high dose, low frequency treatment (‘surge’) and (2) a low dose, high frequency treatment (‘pulse’). Growth was measured alongside dhurrin concentrations to identify potential growth/defence trade-off dynamics. Concentrations of other nitrogenous metabolites (nitrate and free amino acids), and total elemental nitrogen, were measured to allow a better understanding of the magnitude of dhurrin’s role relative to other nitrogen storage molecules. Expression of selected genes in the nitrogen metabolism and dhurrin biosynthesis and recycling pathways was also measured to provide insight into the effects of the nitrogen provisioning regime at the molecular level.
If dhurrin is an important component of nitrogen storage, growth rates and dhurrin concentrations will differ between treatments and genotypes. Our hypotheses were: (1) if dhurrin is produced for defence when nitrogen is limiting, BTx623 pulse plants will exhibit a short-term growth disadvantage, relative to tcd1 pulse plants, (2) if plants accumulate nitrogen in dhurrin when an influx of nitrogen is provided, BTx623 surge plants will: (a) have higher dhurrin concentrations than the pulse counterparts, and (b) exhibit a growth advantage over tcd1 surge plants, and (3) following nitrogen application, surge plants of both genotypes will upregulate nitrogen assimilation and dhurrin biosynthesis genes more than the pulse counterparts.
Materials and methods
Study genotypes
Our study used two genotypes of S. bicolor. The first genotype, BTx623, is an inbred line and the reference genome for S. bicolor. The second genotype, tcd1, is completely acyanogenic due to a mutation in the CYP79A1 gene. This was identified using the reverse genetic technique of Targeted Induced Local Lesions in Genomes (TILLING) (Blomstedt et al. 2012). tcd1 was initially backcrossed to the parent line and subsequently backcrossed to BTx623 three times and allowed to self-fertilise. Homozygous mutant lines after the third backcross were used in this experiment. Growth rate is similar in the two genotypes (Blomstedt et al. 2018).
Growth conditions
Plants were grown under controlled greenhouse conditions at Monash University (−37.91°, 145.14°) from October to December 2022. Mean temperature was 28.77 ± 0.04°C and 22.02 ± 0.04°C (day/night). Mean daytime illuminance was 10,848 ± 107 lx and mean daylength was 12.6 h. Sodium lamps (MK-1 Just-a-shade, Ablite) provided supplementary lighting for 13 h per day.
Plants were grown in 1.9 L pots. Drainage holes in the bottoms of pots were covered with polypropylene woven weed mat to allow water to drain through without the loss of substrate. Washed river sand was used as the substrate to allow nutrient solution to drain through the pot while minimising retention of nutrients. Two seeds were planted at a depth of 1 cm per pot, in a total of 200 pots. Pots were watered to saturation every day for the first 12 days post-sowing and subsequently every second day for the remainder of the experiment. Seedlings were grown for 17 days post-sowing before being thinned to one plant per pot and treatments commenced.
Nitrogen treatments
To test whether dhurrin acts as a storage mechanism for an influx nitrogen, two nitrogen treatments were implemented in four 10-day cycles. For each genotype, 43 plants were assigned to each treatment. To reduce microenvironment effects, plants were organised in randomised complete blocks and pot positions were rotated once per week. Both treatments used modified Long Ashton’s nutrient solution (Miller et al. 2014) with nitrate and ammonia levels adjusted to a 3:1 ratio (Table S1 in supplementary information). The first treatment, referred to herein as the ‘pulse’ treatment, involved the application of a 2 mM nitrogen solution on days 2, 4, 6, 8 and 10 of each cycle. The second treatment, referred to herein as the ‘surge’ treatment, involved the application of a 10 mM nitrogen solution on day 10 of each cycle. This resulted in an equal total nitrogen application per 10-day cycle across treatments but at different application frequencies and intensities. Treatments were selected to make nitrogen a limiting resource, in terms of total nitrogen application over the duration of the experiment but not so low as to kill our study plants, based on a previous study using the same sorghum lines (Blomstedt et al. 2018). The nutrient solutions also provided other essential nutrients. To account for this, a third nutrient solution (0 mM surge supplement) was created with no nitrate or ammonia but with all other nutrients (Table S2 in supplementary information). This surge supplement was provided to surge plants on days 2, 4, 6, and 8 of each cycle, and functioned to balance all other constituents of the solution and ensure that the observed differences between plants were solely due to nitrogen application. Plants were watered with nutrient solution to saturation; initially with 125 mL per plant and increased as required (Table S2 in supplementary information).
Nutrient solution was also required prior to the start of the experiment. Plants exhibited signs of yellowing, potentially indicating nutrient deficiency by 13 days post-sowing (Clark et al. 1981). As a result, all plants were saturated with the pulse nutrient solution (2 mM nitrogen). This was repeated 15 days post-sowing as yellowing was still evident.
Harvesting and growth measurements
Five destructive harvests were performed over the course of the experiment. The first, a baseline harvest, was performed on day 17 post-sowing. As there were still two seedlings per pot at this stage, any defective or sickly plants were discarded. From the remaining healthy plants, 10 BTx623 and 10 tcd1 plants were randomly selected for the baseline harvest. Each plant was removed, had the roots washed and was oven dried at 60°C for 72 h. Any remaining pots with two plants had one plant randomly selected and removed. The following day, the first nitrogen treatment cycle commenced (day 1 of treatment). The remaining four harvests were performed 2 days after each surge treatment application, on days 12, 22, 32 and 42 of treatment. At each timepoint, 10 blocks were randomly selected, with 10 replicates per genotype × treatment combination harvested per timepoint (Table S2 in supplementary information). Plants were separated into roots, sheaths, and green and senesced leaves. Leaves were considered senesced if more than 50% of the surface area was discoloured. All tissue was dried in ovens at 60°C for at least 72 h before the biomass was measured. Biomass measurements and leaf counts included both green and senesced leaves.
Biochemical analysis
To prepare samples for biochemical analysis, dried tissue was cut into ~1 cm pieces and finely ground using a TissueLyser II (Qiagen). Each homogenised sample contained the entirety of an individual plant’s green leaves, sheaths or roots. Senesced leaves were excluded from biochemical analysis.
Samples from all tissue types were measured using the evolved cyanide method (Woodrow et al. 2002; Gleadow et al. 2012; Quinn et al. 2022) to calculate the quantity of HCN released by hydrolysing all dhurrin present (HCN potential). 10 mg of dried, ground tissue was placed in a vial, to which 300 μL of 2 mg mL−1 beta-glucosidase (G0395, Sigma-Aldrich) in 0.1 M trisodium citrate buffer was added. A 200 μL Eppendorf tube containing 200 μL of 1 M sodium hydroxide was placed in the vial before the vial was sealed and frozen. Frozen samples were defrosted and refrozen to ensure complete disruption of tissue. Samples were incubated at 37°C for 15 h. Evolved HCN is released from the plant tissue and trapped in the sodium hydroxide solution during this process. A 50 μL aliquot of the sodium hydroxide solution was subsequently reacted with 0.5 M acetic acid (50 μL), succinimide reagent (125 μL) and barbituric acid (50 μL) in a 96-well plate. Plates were incubated at room temperature for 15 min before absorbance was measured at 590 nm using a FLUOstar Galaxy plate reader and compared to a standard curve.
Nitrate concentration was measured using a method adapted from O’Donnell et al. (2013). Dried, ground tissue (15 mg) was diluted in 500 μL of distilled water and shaken in an Eppendorf tube containing a ball bearing. Samples were shaken for 4 min in a TissueLyser II, incubated for 1 h at 45°C and centrifuged for 15 min at 16,000g before the supernatant was extracted. In a 96-well plate, 5 μL of sample was mixed with 20 μL of salicylic acid/sulfuric acid solution before incubating at room temperature for 20 min. 300 μL of 3 M sodium hydroxide was subsequently added. Once the plate had cooled, absorbance was measured at 410 nm using a FLUOstar Galaxy plate reader. Potassium nitrate (P8394, Sigma-Aldrich) was used as the standard.
Concentration of free amino acids was measured using a method adapted from Moore and Stein (1954), and Jones et al. (2002). Dried, ground tissue (15 mg) was diluted in 500 μL of distilled water and shaken in an Eppendorf tube containing a ball bearing. Samples were shaken for 4 min in a TissueLyser II, incubated for 1 h at 45°C and centrifuged for 15 min at 16,000g. The supernatant was subsequently extracted. In a 96-well plate, 80 μL of diluted sample was mixed with 60 μL 2% ninhydrin reagent solution (N7285, Sigma-Aldrich) before incubating at 80°C for 30 min. Next, 80 μL 50% ethanol solution was added and absorbance was measured at 590 nm using a FLUOstar Galaxy plate reader. Glycine (AJA1083, Ajax Finechem) was used as the standard.
Total elemental nitrogen ([N]; %) was measured by the Monash Analytical Platform, Monash University (Clayton, Vic, Australia), in leaf, sheath and root tissue from plants harvested after 12 and 42 days of treatment (n = 5). Results were used to calculate mass of nitrogen in each organ (leaf/sheath/root Npool; mg), each plant (total Npool; mg) and subsequently, nitrogen productivity (ANplant; g day−1 gN−1), and allocation of nitrogen to dhurrin (dhurrin-N/N; %) and nitrate (nitrate-N/N; %) using the following equations:
Statistical analysis
Statistical analyses were performed in GraphPad Prism 9.0.1 (GraphPad Software, San Diego, CA, USA). Biomass, number of leaves, HCN potential, proportion of nitrogen allocated to nitrate, nitrogen productivity, and concentrations of nitrate, amino acids and elemental nitrogen were analysed by three-way ANOVA, using genotype, treatment and timepoint as the explanatory variables. Within timepoints, leaf, sheath, root and total Npool were analysed by two-way ANOVA, using genotype and treatment as the explanatory variables. Allocation of nitrogen to dhurrin in BTx623 was analysed by two-way ANOVA, using timepoint and treatment as the explanatory variables. Where necessary, skewness of data was reduced via log-transformation (leaf, sheath and root nitrate concentrations, and sheath and root amino acid concentrations) or square root-transformation (leaf, sheath and root HCN potential). Where significant effects were found in ANOVAs, Tukey’s tests were performed to identify significant differences among groups. For all analyses, P < 0.05 was considered significant. ANOVA results are presented in Table S3 in supplementary information.
Gene expression
Leaf tissue was collected for RNA extraction from three plants per genotype × treatment combination. 100 mg samples were taken from the mid-section of the youngest fully expanded leaf and the expanding leaf, and snap frozen in liquid nitrogen and stored at −80°C until needed. Samples were collected slightly before, and 24 and 48 h after, the application of nutrient solution on day 40 of treatments. Three genes were selected for analysis: CYP79A1, nitrilase 4B2 (NIT4B2) and glutamine synthetase 2 (GS2). CYP79A1 is involved in the rate-determining first step of the dhurrin biosynthesis pathway. GS2 is involved in primary assimilation of nitrogen through the glutamine-glutamate (GS-GOGAT) cycle. NIT4B2 is involved in the dhurrin recycling pathway that enables the recovery of nitrogen without releasing HCN (Bjarnholt et al. 2018).
Samples were ground using a bead mill cooled with dry ice (FastPrep, MP-Biomedicals). RNA was extracted with 1 mL TRIzol reagent (15596018, Invitrogen) and resuspended in RNase-free water. RNA concentration and purity were determined using a NanoDrop spectrophotometer (ND-1000, Thermo Fisher Scientific) and integrity checked on a 1.5% agarose-TAE gel. 1 μg total RNA was incubated with DNase (RQ1 DNase, Promega) and used directly for cDNA synthesis using SuperScript III Reverse Transcriptase (Invitrogen) with Oligo dT primers according to manufacturer instructions (Table S4 in supplementary information).
Transcript levels for CYP79A1 (Sobic.001G012300), NIT4B2 (Sobic.004G225100) and GS2 (Sobic.006G249400), normalised to ubiquitin (Sobic.001G311100) and β-actin (Sobic.001G112600), were determined by qPCR using a Light Cycler 480II (Roche). SensiFast SYBR Green No-ROX kit (Bioline) was used for the qPCR reaction mix and reactions were run on 384-well plates set up using an EpMotion 5075 Robot (Eppendorf). On each plate, samples were measured in triplicate alongside a set of gene-specific standards, no-template controls and no-reverse transcriptase controls to check for DNA contamination. Mean normalised expression was calculated for the expanded leaf and the expanding leaf using the Q-Gene method (Simon 2003) and analysed by three-way ANOVA in R version 4.3.2 (R Core Team 2023). Tukey’s tests were performed to identify significant differences among groups. Figures were generated using GraphPad Prism 9.0.1.
Results
Biomass and leaves
Genotype had no significant effect on biomass but treatment and timepoint, and their interaction, did (Table S3 in supplementary information). Mean biomass followed a very similar trend in BTx623 and tcd1, increasing significantly between each timepoint under both treatments but being significantly higher in pulse plants than surge plants at every timepoint other than at 12 days of treatment. There was no timepoint at which mean biomass of either genotype significantly differed from the other, within treatments and timepoints (Fig. 1a, b). Biomass of plants in the baseline harvest did not significantly differ between genotypes either (Table S5 in supplementary information).
(a, b) Whole plant biomass and (c, d) number of leaves of S. bicolor genotypes BTx623 and tcd1, grown under two nitrogen treatments: low dose, high frequency (pulse; black symbols and letters) and high dose, low frequency (surge; grey symbols and letters), measured at four timepoints. Graph shows means ± s.e.m. (n = 10). Different letters indicate a significant difference, analysed by three-way ANOVA and Tukey’s tests (P < 0.05).
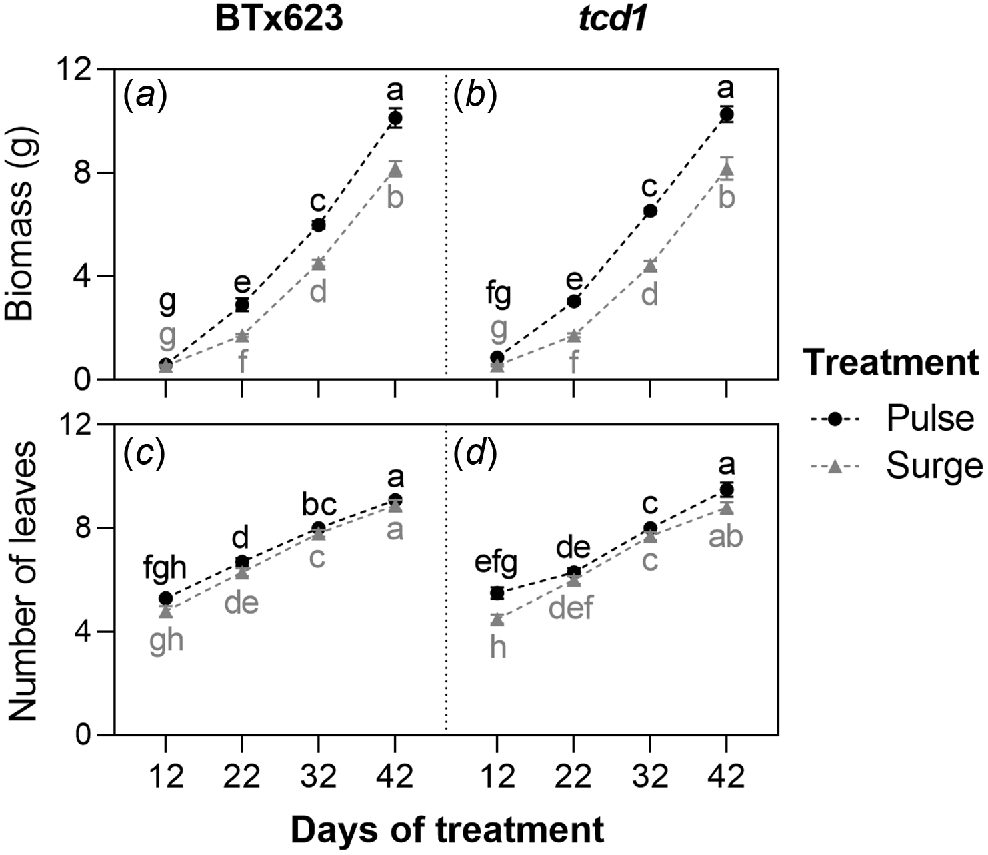
Genotype had no significant effect on number of leaves but treatment and timepoint did (Table S3 in supplementary information). There was no timepoint at which mean number of leaves significantly differed between genotypes, within treatments. In BTx623, mean number of leaves was never significantly different between treatments at any timepoint. After 12 days of treatment, mean number of leaves ranged from 4.5 to 5.5 across genotypes and treatments. After 42 days of treatment, mean number of leaves ranged from 8.8 to 9.5 across genotypes and treatments (Fig. 1c, d).
HCN potential
HCN potential was negligible in tcd1 across all tissues and harvests, with no significant differences among treatments or timepoints (Fig. 2). BTx623, in contrast, exhibited variation among treatments and timepoints.
Hydrogen cyanide (HCN) potential in (a, b) leaf, (c, d) stem and (e, f) root tissue for S. bicolor genotypes BTx623 and tcd1, grown under two nitrogen treatments: low dose, high frequency (pulse; black symbols and letters) and high dose, low frequency (surge; grey symbols and letters), measured at four timepoints. Graph shows means ± s.e.m. (n = 10). Within organs, different letters indicate a significant difference, analysed by three-way ANOVA and Tukey’s tests (P < 0.05).
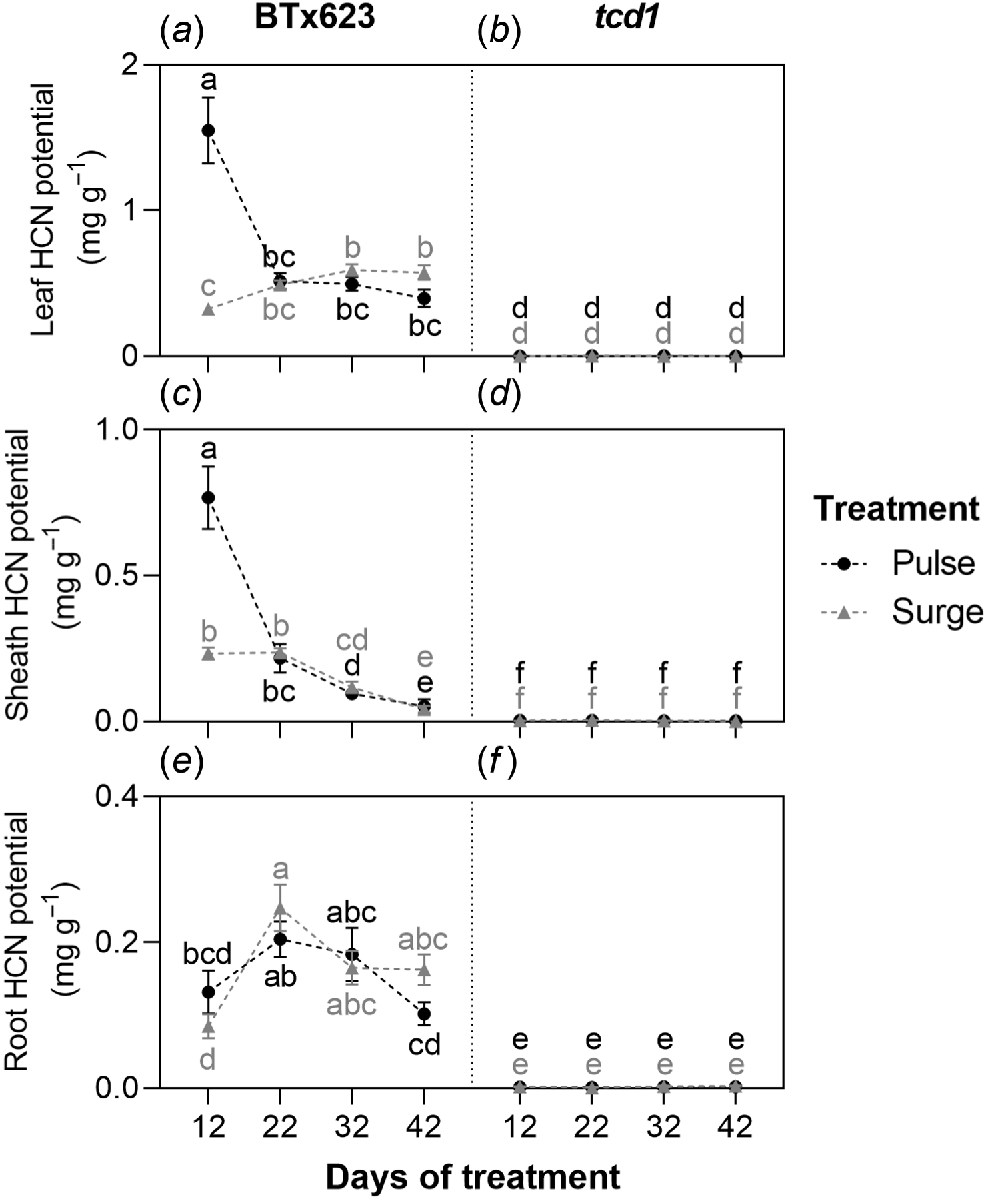
The largest range in BTx623’s HCN potential among timepoints and treatments was found in the leaves. At the baseline harvest, mean leaf HCN potential was 1.47 mg g−1 (Table S5 in supplementary information). Mean leaf HCN potential in pulse plants (1.55 mg g−1) was significantly higher than that of surge plants (0.32 mg g−1) by 12 days into the treatment period. Mean leaf HCN potential concentration of pulse plants had declined to 0.52 mg g−1 by 22 days of treatment while that of surge plants was 0.49 mg g−1 – there was no longer a significant difference between treatments. Similarity between surge and pulse plants’ leaf HCN potential was maintained for the remainder of the experiment, with small changes in means but no significant differences found between treatments on days 32 or 42. At 32 and 42 days of treatment, leaf HCN potential in surge plants was significantly higher than at the 12-day mark (Fig. 2a).
BTx623’s sheath HCN potential followed a similar trend, with pulse plants having a high mean HCN potential at 12 days of treatment (0.77 mg g−1) that subsequently decreased significantly between each timepoint thereafter. In surge plants, sheath HCN potential also decreased significantly over the course of the experiment, but had a much lower peak than in pulse plants (0.24 mg g−1 after 22 days of treatment) (Fig. 2c).
Mean root HCN potential in BTx623 remained low in both treatment groups throughout the experiment (ranging between 0.09 and 0.25 mg g−1), with no evidence of a consistent decline over time (Fig. 2e).
Nitrate
Nitrate concentrations showed contrasting trends to HCN potential, with consistently low levels found in the leaves (ranging between 1.3 and 3.3 mg g−1) rather than the roots. Genotype had no significant effect on nitrate concentrations in sheaths or roots but timepoint and treatment, and their associated interaction, did (Table S3 in supplementary information). Sheath nitrate concentration in surge plants peaked at 12 days of treatment in both genotypes (8.9 mg g−1 in BTx623, 7.9 mg g−1 in tcd1) before decreasing significantly over time. Root nitrate concentration peaked at 22 days of treatment in surge plants in both genotypes (6.0 mg g−1 in BTx623, 7.3 mg g−1 in tcd1) before decreasing significantly over time. Within genotypes, neither sheath nor root nitrate concentration varied significantly over time in pulse plants (Fig. 3).
Nitrate concentration in (a, b) leaf, (c, d) stem and (e, f) root tissue for S. bicolor genotypes BTx623 and tcd1, grown under two nitrogen treatments: low dose, high frequency (pulse; black symbols and letters) and high dose, low frequency (surge; grey symbols and letters), measured at four timepoints. Graph shows means ± s.e.m. (n = 10). Within organs, different letters indicate a significant difference, analysed by three-way ANOVA and Tukey’s tests (P < 0.05).
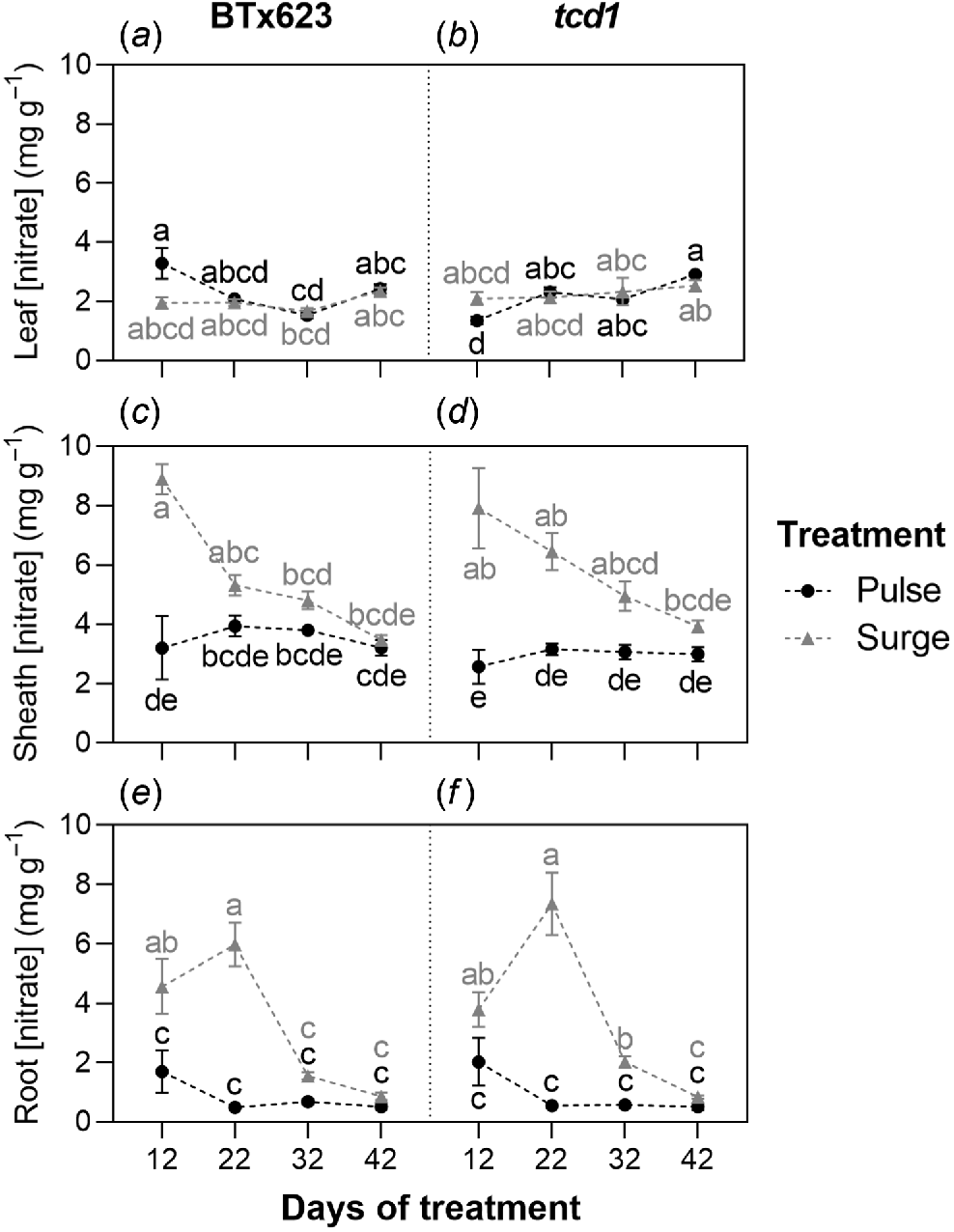
Amino acids
Free amino acid concentrations followed similar trends in both genotypes, with significant differences found over time and between treatments. No significant genotype effects were found in leaf and sheath concentrations. Leaf amino acid concentration spiked at 22 days of treatment in both genotypes under the surge treatment (8.4 mg g−1 in BTx623, 8.0 mg g−1 in tcd1) but remained relatively low in both genotypes under the pulse treatment (1.4–4.0 mg g−1). Sheaths showed a similar pattern, with concentrations already high in both genotypes under the surge treatment by the 12-day mark (8.8 mg g−1 in BTx623, 8.4 mg g−1 in tcd1) and remaining high at the 22-day mark (8.8 mg g−1 in BTx623, 9.0 mg g−1 in tcd1) before gradually declining for the remainder of the treatment period. Sheath amino acid concentrations also declined over time in both genotypes under the pulse treatment but peak amino acid concentrations were again much lower in both genotypes for this treatment (4.2 mg g−1 in BTx623, 3.4 mg g−1 in tcd1). Root amino acid concentrations did not follow a similar trend to the aboveground organs, with levels fluctuating somewhat but only ranging between 1.0 and 3.4 mg g−1 across all genotypes, treatments and timepoints, and rarely varying significantly (Fig. 4).
Concentration of free amino acids in (a, b) leaf, (c, d) stem and (e, f) root tissue of S. bicolor genotypes BTx623 and tcd1, grown under two nitrogen treatments: low dose, high frequency (pulse; black symbols and letters) and high dose, low frequency (surge; grey symbols and letters), measured at four timepoints. Graph shows means ± s.e.m. (n = 10). Within organs, different letters indicate a significant difference, analysed by three-way ANOVA and Tukey’s tests (P < 0.05).
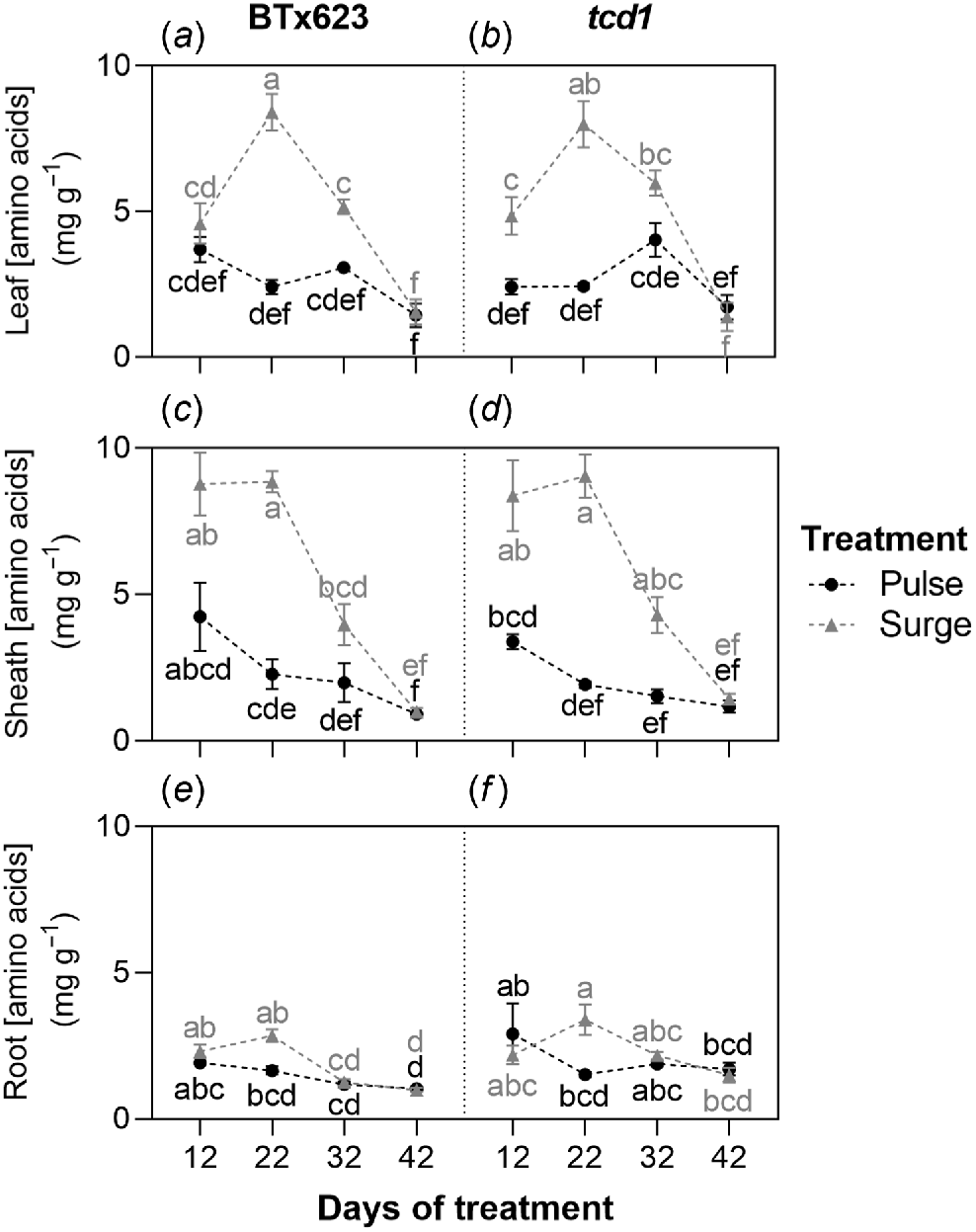
Total elemental nitrogen and allocation
Total elemental nitrogen concentration was measured in leaf, stem and root tissue. Across timepoints, treatments and genotypes, mean nitrogen concentration was consistently highest in leaf tissue. After 12 days of treatment, mean leaf nitrogen concentrations were significantly higher in BTx623 pulse plants than in BTx623 surge plants and tcd1 pulse plants. After 42 days of treatment, there were no significant differences in nitrogen concentration between genotypes or treatments within organs. After 12 days of treatment, total Npool was significantly higher in pulse plants than surge plants but this did not differ significantly between genotypes. After 42 days of treatment, total Npool did not differ significantly between treatments or genotypes (Table 1). Npool in individual organs also never differed significantly between the genotypes, within timepoints and treatment groups (Table S6 in supplementary information).
Days of treatment | Genotype | Treatment | Leaf [nitrogen] (%) | Sheath [nitrogen] (%) | Root [nitrogen] (%) | Total Npool (mg) | Nitrogen productivity (g day−1 gN−1) | |
---|---|---|---|---|---|---|---|---|
12 | BTx623 | Pulse | 3.48 ± 0.21a | 1.93 ± 0.11b | 1.12 ± 0.10a | 14.35 ± 1.20a | 1.65 ± 0.11ab | |
Surge | 2.75 ± 0.12b | 2.70 ± 0.10a | 1.28 ± 0.05a | 9.90 ± 0.77b | 1.70 ± 0.04ab | |||
tcd1 | Pulse | 2.76 ± 0.08b | 1.53 ± 0.08c | 1.06 ± 0.09ab | 16.56 ± 0.84a | 1.89 ± 0.11a | ||
Surge | 2.52 ± 0.07bc | 2.14 ± 0.09b | 1.16 ± 0.03a | 10.76 ± 0.40b | 1.88 ± 0.03a | |||
42 | BTx623 | Pulse | 1.57 ± 0.12d | 0.71 ± 0.10d | 0.71 ± 0.05c | 97.06 ± 6.56a | 1.80 ± 0.17ab | |
Surge | 1.84 ± 0.11d | 0.97 ± 0.07d | 0.85 ± 0.03bc | 100.69 ± 5.56a | 1.40 ± 0.04b | |||
tcd1 | Pulse | 1.56 ± 0.16d | 0.58 ± 0.05d | 0.65 ± 0.02c | 90.15 ± 4.81a | 1.91 ± 0.11a | ||
Surge | 2.01 ± 0.05cd | 0.84 ± 0.08d | 0.80 ± 0.02c | 101.54 ± 1.50a | 1.39 ± 0.05b |
Values are means ± s.e.m. (n = 5). Npool data were analysed by two-way ANOVA and Tukey’s tests (P < 0.05), and within timepoints, values with different superscript letters are significantly different. All other parameters were analysed by three-way ANOVA and Tukey’s tests (P < 0.05) and within organs, values with different letters are significantly different.
The proportion of nitrogen allocated to dhurrin was not calculated in tcd1 because its HCN potentials were consistently negligible. In BTx623, mean nitrogen allocation to dhurrin was significantly higher after 12 days of treatment than after 42 (Table S3 in supplementary information). Within timepoints, nitrogen allocation to dhurrin significantly differed between the surge (9.92%) and pulse (22.61%) plants after 12 days of treatment but did not significantly differ between treatments (1.04% in surge plants, 1.08% in pulse plants) after 42 days of treatment (Fig. 5a).
Proportion of nitrogen allocated to (a) dhurrin in BTx623 and (b) nitrate in BTx623 and tcd1 grown under two nitrogen treatments: low dose, high frequency (pulse; black symbols and letters) and high dose, low frequency (surge; grey symbols and letters), measured at two timepoints. Columns show means ± s.e.m. (n = 5). Different letters indicate a significant difference, analysed by three-way ANOVA and Tukey’s tests (P < 0.05).
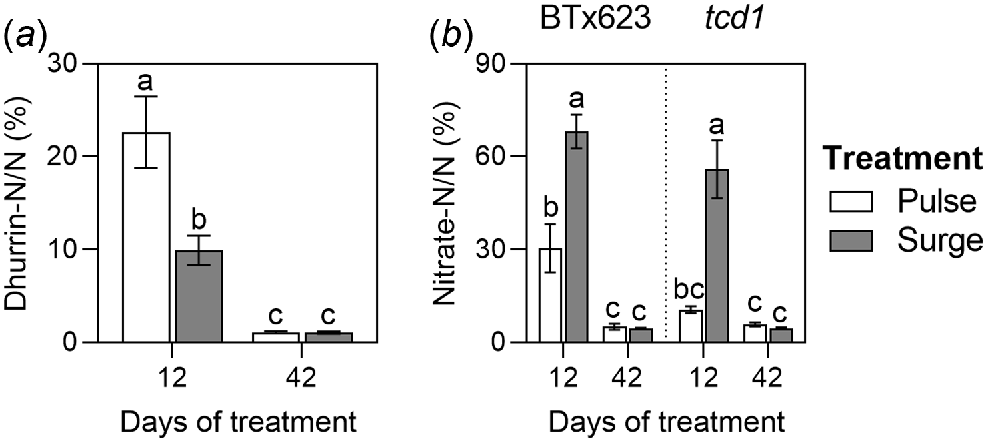
Within timepoints, mean proportion of nitrogen allocated to nitrate was much higher than that to dhurrin, ranging from 10.55% in tcd1 pulse plants to 68.04% in BTx623 surge plants after 12 days of treatment, and from 4.46% in BTx623 surge plants to 5.78% in tcd1 pulse plants after 42 days of treatment. Proportions of nitrogen allocated to nitrate were significantly higher after 12 days of treatment than after 42 days (Table S3 in supplementary information). Within the 42-day timepoint, there were no significant differences among genotypes or treatments (Fig. 5). Within the 12-day timepoint, surge plants had significantly higher nitrogen allocation to nitrate than pulse plants, with no significant differences between the genotypes (Fig. 5b).
Nitrogen productivity quantifies growth rate per gram of nitrogen in plants (Ingestad 1979), therefore assessing the efficiency with which a plant uses nitrogen for growth. A significant treatment effect on nitrogen productivity was observed, with pulse plants having higher nitrogen productivity than surge plants overall. Within pulse plants, no significant differences were found among genotypes or timepoints. No significant effect of genotype on nitrogen productivity effect was detected (Table 1).
Gene expression
CYP79A1 is involved in the rate-determining first step of the dhurrin biosynthesis pathway. In both expanding and expanded leaves, expression of CYP79A1 was significantly higher at the 24-h timepoint than at the 0-h and 48-h timepoints in both genotypes. No significant changes were observed in pulse plants (Fig. 6a, b; Fig. S1a, b). NIT4B2 is involved in the dhurrin recycling pathway that enables the recovery of nitrogen without releasing HCN. There were no significant changes in expression of NIT4B2 in expanded leaf tissue. In expanding leaves of surge plants, there was a significant treatment effect on expression: NIT4B2 expression was significantly lower at the 24-h timepoint than in both the 0-h and 48-h timepoints. No significant changes were observed in pulse plants (Fig. 6c, d; Fig. S1c, d). GS2 is involved in primary assimilation of nitrogen through the glutamine-glutamate (GS-GOGAT) cycle. GS2 expression in surge plants of both genotypes was significantly higher at the 24-h timepoint than at the 0-h and 48-h timepoints, in both expanding and expanded leaves. No significant changes were observed in pulse plants (Fig. 6e, f; Fig. S1e, f).
Expression levels of CYP79A1, nitrilase 4B2 (NIT4B2) and glutamine synthetase 2 (GS2) in (a, c, e) the youngest fully expanded leaf and (b, d, f) the expanding leaf of two S. bicolor genotypes (BTx623 and tcd1). Plants were grown under two nitrogen treatments: low dose, high frequency (pulse) and high dose, low frequency (surge). Samples were taken 0, 24 and 48 h after the application of nutrient solution on day 40 of the treatment period. Transcripts were normalised relative to ubiquitin expression. Values are means ± s.e.m. (n = 3). Within graphs, columns marked with different letters are significantly different, analysed by three-way ANOVA and Tukey’s test (P < 0.05).
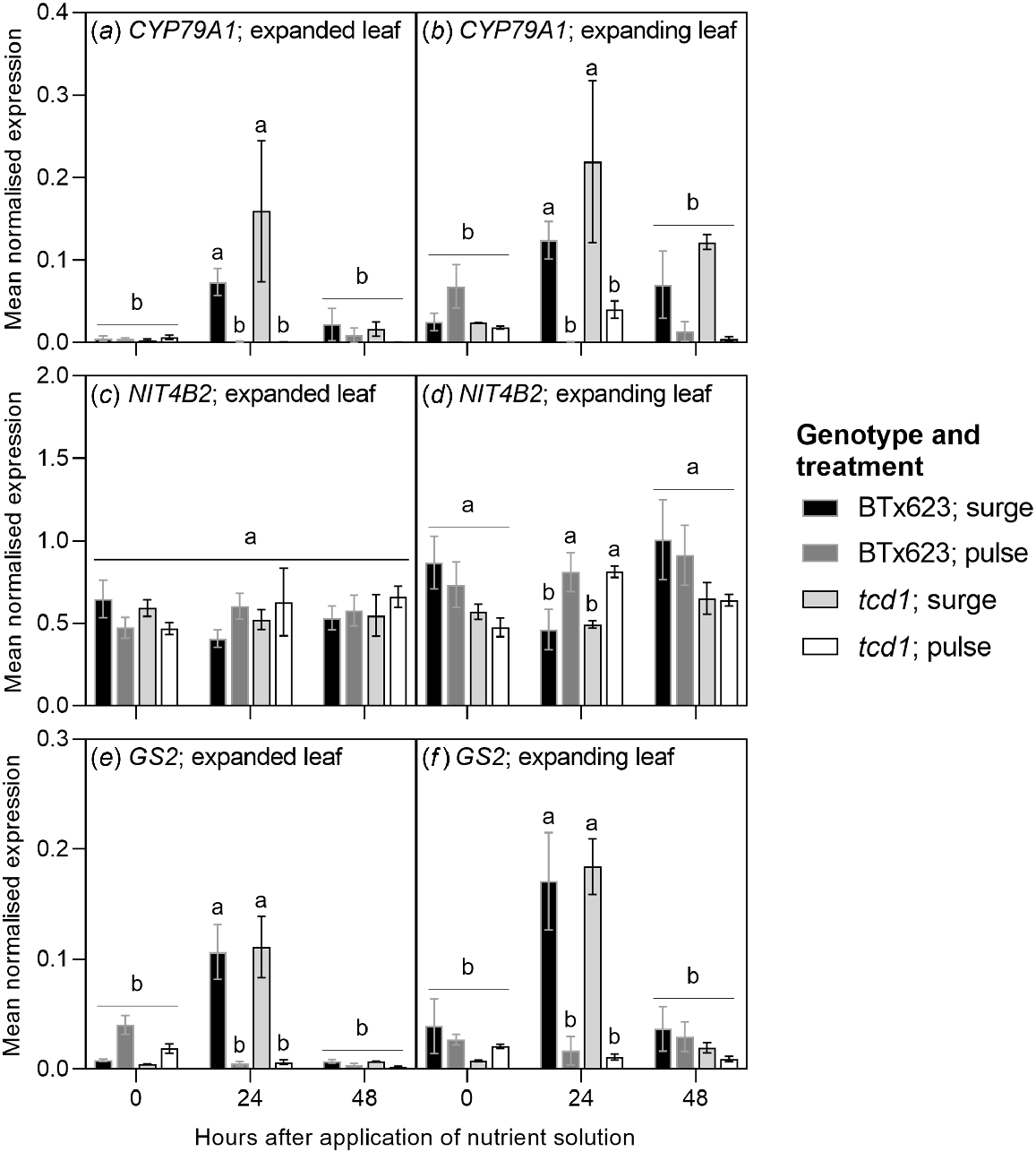
Discussion
Nitrogen was a limiting resource for study plants
To induce a growth/defence trade-off in this study, nitrogen had to be a limiting resource in terms of total nitrogen application over the duration of the experiment. Therefore, we applied nitrogen at a rate previously found to be limiting (Blomstedt et al. 2018; Myrans et al. 2021). Mean leaf nitrogen concentration ranged from 2.5 to 3.5% after 12 days of treatment (~5 leaves per plant) and from 1.6 to 2.1% after 42 days of treatment (~9 leaves per plant), across treatments and genotypes. In comparison, Blomstedt et al. (2018) found that, under nitrogen-limiting conditions, the same lines had mean leaf nitrogen concentration ranging from 4.0 to 4.2% at the 5-leaf stage and from 1.3 to 1.4% at the 10-leaf stage. When provided with sufficient nitrogen, Blomstedt et al. (2018) found that the same lines had higher mean leaf nitrogen concentrations of 4.6% at the 5-leaf stage and 3.0–3.1% at the 10-leaf stage. These findings suggest that nitrogen was indeed a limiting resource for our study plants.
Dhurrin did not significantly impact growth rate
Our first hypothesis was that, if dhurrin is produced for defence despite nitrogen being limiting, cyanogenic BTx623 would grow more slowly than acyanogenic tcd1 under the pulse treatment. However, within the pulse treatment, whole plant biomass, nitrogen productivity, Npool in any organ and total Npool did not differ significantly between genotypes at any timepoint, suggesting that dhurrin is not a form of reserve storage in sorghum (Chapin et al. 1990). This occurred even in young plants, in which 22.6% of BTx623 pulse plants’ nitrogen was stored in dhurrin (12 days of treatment).
Previous studies have produced mixed results on whether dhurrin production reduces growth. Gruss et al. (2022) found that an acyanogenic sorghum mutant (SbEMS2447 cyp79a1) had a larger green plant area than the wildtype in the absence of herbivory, whereas Blomstedt et al. (2012) and Sohail et al. (2020) observed slower growth in tcd1 seedlings than in BTx623. We observed no such genotype differences in terms of growth, nitrogen acquisition or nitrogen productivity, suggesting that, in our study, dhurrin did not result in a growth advantage or disadvantage. This is likely because any nitrogen that was diverted to dhurrin, away from growth, was recycled when necessary (Chapin et al. 1990). Our study differed from that of Gruss et al. (2022) in making nitrogen a limiting resource; acyanogenic lines possibly only experience a growth advantage when sufficient nitrogen is supplied. Further studies are needed to fully understand the complex relationship between cyanogenic glucosides and growth.
Dhurrin can be recycled to boost growth when necessary
The ability for sorghum to recycle dhurrin to release nitrogen was exemplified when comparing surge and pulse BTx623 plants. Day 12 of treatment (29 days post-sowing) was the only timepoint at which HCN potential significantly differed between these two groups and the only timepoint at which biomass did not significantly differ between these. Dhurrin concentrations typically peak in sorghum in the first two weeks post-sowing and gradually decrease over time (Miller et al. 2014; Emendack et al. 2017; Cowan et al. 2021), as observed in our BTx623 pulse plants. However, in BTx623 surge plants, leaf and sheath HCN potential decreased much more rapidly than in pulse plants during the first treatment cycle but later increased. This unusual HCN potential trend suggests that, to maintain a similar growth rate to pulse plants during their greatest nitrogen deficit (days 0–9 of treatment), surge plants sacrificed defence by recycling dhurrin. On average, surge plants had 12.7% less of their total nitrogen pool allocated to dhurrin than pulse plants after 12 days of treatment, suggesting that in the preceding days, dhurrin had represented a sizeable back-up source of nitrogen, allowing surge plants to grow as quickly as pulse plants despite having a smaller total Npool.
The importance of dhurrin’s contribution appears to diminish with ontogeny, in terms of both defence and nitrogen storage. Between days 12 and 42 of treatment, BTx623 pulse plants dropped the proportion of nitrogen allocated to dhurrin from 22.6 to 1.1%. Myrans et al. (2021) found similarly low proportions of nitrogen allocated to dhurrin in mature BTx623 (0.9% in nitrogen-deficient plants). Despite the low allocation of nitrogen to dhurrin on day 42, mean leaf HCN potentials remained high enough to offer effective defence against small herbivores (0.4 mg g−1). The allocation costs of synthesising and storing dhurrin appear to be much lower than in seedlings by this stage but this also means that the amount of nitrogen released by recycling dhurrin might be too low to noticeably impact growth. Gruss et al. (2023) found that an acyanogenic mutant of sorghum line Tx642 did not have inferior post-anthesis drought tolerance compared to the wildtype, contrary to hypotheses (Burke et al. 2013; Emendack et al. 2017), supporting the possibility that dhurrin may not play a significant role in nitrogen storage and supplementation in the later growth stages of sorghum.
Nitrate and amino acids were important for fast nitrogen accumulation
Our second hypothesis was that, if BTx623 can accumulate influxes of nitrogen in dhurrin and recycle this when needed, BTx623 surge plants could manage nitrogen more efficiently and grow faster than tcd1 surge plants. However, within the surge treatment, neither biomass nor nitrogen productivity significantly differed between genotypes at any timepoint and BTx623’s HCN potential was never significantly higher in surge plants than pulse plants. This result was supported by Blomstedt et al. (2018), who found no significant differences in BTx623’s HCN potential between plants given limiting, sufficient or excessive nitrogen supply. Our results suggest that BTx623 did not extensively use dhurrin to accumulate nitrogen provided in surge applications.
Instead of accumulating large quantities of dhurrin after surge applications of nitrogen, both genotypes appear to have accumulated nitrate and amino acids. Concentrations of amino acids in leaves and sheaths, and nitrate in sheaths and roots, increased more rapidly and by proportionally higher quantities than HCN potential in BTx623 plants following the first two surge applications of nitrogen. These accumulation spikes were unique to surge-treated plants. Furthermore, BTx623 surge plants allocated 6.9 and 4.3 times more nitrogen to nitrate than dhurrin on days 12 and 42 of treatment respectively, while BTx623 pulse plants allocated 1.3 and 4.7 times more nitrogen to nitrate than dhurrin on these days respectively. Sorghum is known to accumulate nitrate when this is provided in excess (Blomstedt et al. 2018; Sowiński and Głąb 2018), and many taxa accumulate and store nitrate because this can be quickly reduced for assimilation and aids in the maintenance of turgor pressure (Mott and Steward 1972; Burns et al. 2010). Collectively, our results suggest that other forms of nitrogen storage, such as nitrate and amino acids, are likely to have contributed more to overall nitrogen metabolism than dhurrin. The need to accumulate and store the nitrogen provided in surges may have contributed to surge plants’ lower nitrogen productivity than in the pulse counterparts in both genotypes.
Dhurrin biosynthesis was upregulated in surge plants
Our third hypothesis was that, following nitrogen application, surge plants of both genotypes would upregulate nitrogen assimilation and dhurrin biosynthesis genes more than the pulse counterparts. Pulse plants showed no significant changes in expression of the measured genes during the 48 h following treatment. In contrast, at the 24-h timepoint, surge plants had upregulated GS2 and CYP79A1, and downregulated NIT4B2. Higher GS2 expression indicates more rapid nitrogen assimilation, resulting from the high nitrogen dose provided 24 h earlier. The upregulation of CYP79A1 and concurrent downregulation of NIT4B2 indicate increased biosynthesis and decreased recycling of dhurrin (Bjarnholt et al. 2018), supporting our hypothesis. An antagonistic relationship between these two genes was also observed by Blomstedt et al. (2018), whereby CYP79A1 expression was higher and NIT4B2 expression lower in the sorghum line acdc1 than in BTx623, resulting in faster dhurrin recycling. Despite the product of CYP79A1 in tcd1 plants being non-functional, upregulation of this gene still occurred, as was also documented by Blomstedt et al. (2018).
Although our hypothesis was supported by the gene expression results, there was no rise in HCN potential in surge BTx623 plants between days 32 and 42 of treatment to correspond with the upregulation of CYP79A1 and downregulation of NIT4B2. One explanation for this is that small fluctuations in HCN potential were not captured due to this trait only being measured once every 10 days. Expression of sorghum’s dhurrin biosynthesis and recycling genes has been documented to fluctuate frequently, affected by diverse stimuli such as circadian rhythms (Gleadow et al. 2021), wounding (Sohail et al. 2023), drought (Ananda et al. 2022) and, in line with our results, nitrogen application (Rosati et al. 2024). The changing gene expression we observed brings dhurrin’s complex and enduring role in sorghum’s broader nitrogen metabolism to attention, even if this is not the most important nitrogen storage molecule during later developmental stages.
Applications and future directions
In this study, tcd1 was found to have no growth disadvantage compared to BTx623, signifying suitability for further development as a crop. tcd1 also did not store higher concentrations of nitrate than BTx623. However, aboveground nitrate concentrations in both genotypes frequently exceeded the recommended limit of 2 mg g−1 for ruminant forage (Sunaga et al. 2008; Stuart 2012), especially in sheaths and surge plants, raising concerns of nitrate poisoning. Cattle are recommended to not graze on forage sorghum directly after the application of fertiliser, especially in the lower part of the stem and leaf sheaths, to mitigate the risk of nitrate poisoning (Stuart 2012). Each of our harvests occurred only 2 days after a surge application of nitrogen, when nitrate concentrations were expected to peak.
The surge treatment also contributed to lowered nitrogen productivity in both genotypes, suggesting that frequent, low doses of fertiliser are more resource-efficient for producing biomass than infrequent, high doses. Notably, while nitrogen productivity is a useful estimate of the efficiency with which plants use nitrogen to grow, this is a static measurement and relies on assumptions that no nitrogen has been lost through means such as leaf senescence and root exudates. As an alternative, measurement of nitrogen use efficiency would yield more accurate data in future studies.
To deepen our understanding of dhurrin production and recycling in sorghum, further research into the mechanisms controlling HCN potential would be beneficial. Further research into the gene expression of the dhurrin biosynthesis and recycling pathways would help to uncover how nitrogen-deficient plants rapidly decrease dhurrin concentration to recover nitrogen (Gleadow et al. 2021). A study with tissue harvested at many timepoints during the first four weeks post-germination may be the most insightful, as this is the period when dhurrin fluctuations appear to be largest and most important. A comparison between plants provided with an excessive or sufficient nitrogen supply versus a limiting supply would provide further insight into whether nitrogen influxes can stimulate dhurrin accumulation when nitrogen is not limiting. Lastly, monitoring HCN potential in plants given an early, high dose of nitrogen, followed by a period with no nitrogen provision may be useful. This would test the extent to which dhurrin recycling can buffer the need to use up other nitrogen stores and find the point at which BTx623 begins to receive a growth advantage over tcd1 as a result.
Conclusion
Overall, we did not find evidence of BTx623 accumulating surge applications of nitrogen in dhurrin. Instead, dhurrin concentration appeared to be high in seedlings for defence and surge plants recycled dhurrin for additional nitrogen as required. At later growth stages, the proportion of nitrogen allocated to dhurrin was much lower, even though HCN potential was maintained at levels high enough to be useful in defence. At this stage, the quantity of nitrogen stored in dhurrin may be too low to noticeably impact growth, reducing the usefulness of recycling dhurrin. In tcd1, dhurrin was not available to buffer against fluctuations in nitrogen supply but growth was not slower than in BTx623, possibly due to nitrogen being stored in other metabolites. We also found evidence of the nitrogen application regime affecting both BTx623 and tcd1, including more rapid growth and higher nitrogen productivity when nitrogen was applied at a high frequency and low dose (pulse treatment). Our results deepen our understanding of dhurrin’s changing role in nitrogen metabolism as sorghum develops and demonstrate tcd1’s potential to be a safe, fast-growing forage crop.
Data availability
The data that support this study will be shared upon reasonable request to the corresponding author.
Declaration of funding
This research was funded by the Australian Research Council DP210103010. Alicia A. Quinn was supported by an Australian Government Research Training Program (RTP) Scholarship and an AW Howard Memorial Trust Inc. Research Fellowship.
Acknowledgements
The authors thank Cecilia Blomstedt, Nawar Shamaya, Alyssa Jefferyes, Jess Heares and Aspen Roberts for technical assistance and the Monash Analytical Platform for measuring total elemental nitrogen.
References
Ananda GKS, Myrans H, Norton SL, Gleadow R, Furtado A, Henry RJ (2020) Wild sorghum as a promising resource for crop improvement. Frontiers in Plant Science 11, 1108.
| Crossref | Google Scholar |
Ananda GKS, Norton SL, Blomstedt C, Furtado A, Møller BL, Gleadow R, Henry RJ (2022) Transcript profiles of wild and domesticated sorghum under water-stressed conditions and the differential impact on dhurrin metabolism. Planta 255(2), 51.
| Crossref | Google Scholar | PubMed |
Bjarnholt N, Neilson EHJ, Crocoll C, Jørgensen K, Motawia MS, Olsen CE, Dixon DP, Edwards R, Møller BL (2018) Glutathione transferases catalyze recycling of auto-toxic cyanogenic glucosides in sorghum. The Plant Journal 94(6), 1109-1125.
| Crossref | Google Scholar | PubMed |
Blomstedt CK, Gleadow RM, O’Donnell N, Naur P, Jensen K, Laursen T, Olsen CE, Stuart P, Hamill JD, Møller BL, Neale AD (2012) A combined biochemical screen and TILLING approach identifies mutations in Sorghum bicolor L. Moench resulting in acyanogenic forage production. Plant Biotechnology Journal 10(1), 54-66.
| Crossref | Google Scholar | PubMed |
Blomstedt CK, Rosati VC, Møller BL, Gleadow R (2018) Counting the costs: nitrogen partitioning in Sorghum mutants. Functional Plant Biology 45(7), 705-718.
| Crossref | Google Scholar | PubMed |
Boyd FT, Aamodt OS, Bohstedt G, Truog E (1938) Sudan grass management for control of cyanide poisoning. Journal of the American Society of Agronomy 30, 569-582.
| Crossref | Google Scholar |
Burke JJ, Chen J, Burow G, Mechref Y, Rosenow D, Payton P, Xin Z, Hayes CM (2013) Leaf dhurrin content is a quantitative measure of the level of pre- and postflowering drought tolerance in sorghum. Crop Science 53(3), 1056-1065.
| Crossref | Google Scholar |
Burns IG, Zhang K, Turner MK, Edmondson R (2010) Iso-osmotic regulation of nitrate accumulation in lettuce. Journal of Plant Nutrition 34(2), 283-313.
| Crossref | Google Scholar |
Busk PK, Møller BL (2002) Dhurrin synthesis in sorghum is regulated at the transcriptional level and induced by nitrogen fertilization in older plants. Plant Physiology 129(3), 1222-1231.
| Crossref | Google Scholar | PubMed |
Chapin FS, Schulze E, Mooney HA (1990) The ecology and economics of storage in plants. Annual Review of Ecology and Systematics 21(1), 423-447.
| Crossref | Google Scholar |
Clark RB, Pier PA, Knudsen D, Maranville JW (1981) Effect of trace element deficiencies and excesses on mineral nutrients in sorghum. Journal of Plant Nutrition 3(1–4), 357-374.
| Crossref | Google Scholar |
Cowan MF, Blomstedt CK, Møller BL, Henry RJ, Gleadow RM (2021) Variation in production of cyanogenic glucosides during early plant development: a comparison of wild and domesticated sorghum. Phytochemistry 184, 112645.
| Crossref | Google Scholar | PubMed |
Emendack YY, Hayes CM, Chopra R, Sanchez J, Burow G, Xin Z, Burke JJ (2017) Early seedling growth characteristics relate to the staygreen trait and dhurrin levels in sorghum. Crop Science 57(1), 404-415.
| Crossref | Google Scholar |
Getachew G, Putnam DH, De Ben CM, De Peters EJ (2016) Potential of sorghum as an alternative to corn forage. American Journal of Plant Sciences 7(7), 1106-1121.
| Crossref | Google Scholar |
Gleadow RM, Møller BL (2014) Cyanogenic glycosides: synthesis, physiology, and phenotypic plasticity. Annual Review of Plant Biology 65, 155-185.
| Crossref | Google Scholar | PubMed |
Gleadow RM, Møldrup ME, O’Donnell NH, Stuart PN (2012) Drying and processing protocols affect the quantification of cyanogenic glucosides in forage sorghum. Journal of the Science of Food and Agriculture 92(11), 2234-2238.
| Crossref | Google Scholar | PubMed |
Gleadow RM, McKinley BA, Blomstedt CK, Lamb AC, Møller BL, Mullet JE (2021) Regulation of dhurrin pathway gene expression during Sorghum bicolor development. Planta 254(6), 119.
| Crossref | Google Scholar | PubMed |
Gruss SM, Ghaste M, Widhalm JR, Tuinstra MR (2022) Seedling growth and fall armyworm feeding preference influenced by dhurrin production in sorghum. Theoretical and Applied Genetics 135(3), 1037-1047.
| Crossref | Google Scholar | PubMed |
Gruss SM, Souza A, Yang Y, Dahlberg J, Tuinstra MR (2023) Expression of stay-green drought tolerance in dhurrin-free sorghum. Crop Science 63(3), 1270-1283.
| Crossref | Google Scholar |
Herms DA, Mattson WJ (1992) The dilemma of plants: to grow or defend. The Quarterly Review of Biology 67(3), 283-335.
| Crossref | Google Scholar |
Hunt BJ, Taylor AO (1976) Hydrogen cyanide production by field-grown sorghums. New Zealand Journal of Experimental Agriculture 4(2), 191-194.
| Crossref | Google Scholar |
Ingestad T (1979) Nitrogen stress in birch seedlings: II. N, K, P, Ca, and Mg nutrition. Physiologia Plantarum 45(1), 149-157.
| Crossref | Google Scholar |
Jones DL, Owen AG, Farrar JF (2002) Simple method to enable the high resolution determination of total free amino acids in soil solutions and soil extracts. Soil Biology and Biochemistry 34(12), 1893-1902.
| Crossref | Google Scholar |
Kumari A, Goyal M, Kumar R, Sohu R (2021) Morphophysiological and biochemical attributes influence intra-genotypic preference of shoot fly [Atherigona soccata (Rondani)] among sorghum genotypes. Protoplasma 258, 87-102.
| Crossref | Google Scholar | PubMed |
McCall AC, Fordyce JA (2010) Can optimal defence theory be used to predict the distribution of plant chemical defences? Journal of Ecology 98(5), 985-992.
| Crossref | Google Scholar |
McKey D (1974) Adaptive patterns in alkaloid physiology. The American Naturalist 108(961), 305-320.
| Crossref | Google Scholar |
Miller RE, Gleadow RM, Cavagnaro TR (2014) Age versus stage: does ontogeny modify the effect of phosphorus and arbuscular mycorrhizas on above- and below-ground defence in forage sorghum? Plant, Cell & Environment 37(4), 929-942.
| Crossref | Google Scholar | PubMed |
Moore S, Stein WH (1954) A modified ninhydrin reagent for the photometric determination of amino acids and related compounds. Journal of Biological Chemistry 211, 907-913.
| Crossref | Google Scholar |
Mott RL, Steward FC (1972) Solute accumulation in plant cells V. An aspect of nutrition and development. Annals of Botany 36(5), 915-937.
| Crossref | Google Scholar |
Mwadalu R, Mwangi M (2013) The potential role of sorghum in enhancing food security in semi-arid eastern Kenya: a review. Journal of Applied Biosciences 71, 5786-5799.
| Crossref | Google Scholar |
Myrans H, Gleadow RM (2022) Regulation of cyanogenic glucosides in wild and domesticated Eusorghum taxa. Plant Biology 24(6), 1084-1088.
| Crossref | Google Scholar | PubMed |
Myrans H, Vandegeer RK, Henry RJ, Gleadow RM (2021) Nitrogen availability and allocation in sorghum and its wild relatives: divergent roles for cyanogenic glucosides. Journal of Plant Physiology 258–259, 153393.
| Crossref | Google Scholar | PubMed |
Møller BL (2010) Functional diversifications of cyanogenic glucosides. Current Opinion in Plant Biology 13(3), 337-346.
| Crossref | Google Scholar |
Neilson EH, Goodger JQD, Woodrow IE, Møller BL (2013) Plant chemical defense: at what cost? Trends in Plant Science 18(5), 250-258.
| Crossref | Google Scholar | PubMed |
Nelson C (1953) Hydrocyanic acid content of certain sorghums under irrigation as affected by nitrogen fertilizer and soil moisture stress. Agronomy Journal 45(12), 615-617.
| Crossref | Google Scholar |
O’Donnell NH, Møller BL, Neale AD, Hamill JD, Blomstedt CK, Gleadow RM (2013) Effects of PEG-induced osmotic stress on growth and dhurrin levels of forage sorghum. Plant Physiology and Biochemistry 73, 83-92.
| Crossref | Google Scholar | PubMed |
Pičmanová M, Neilson EH, Motawia MS, Olsen CE, Agerbirk N, Gray CJ, Flitsch S, Meier S, Silvestro D, Jørgensen K, Sánchez-Pérez R, Møller BL, Bjarnholt N (2015) A recycling pathway for cyanogenic glycosides evidenced by the comparative metabolic profiling in three cyanogenic plant species. Biochemical Journal 469(3), 375-389.
| Crossref | Google Scholar | PubMed |
Quinn AA, Myrans H, Gleadow RM (2022) Cyanide content of cassava food products available in Australia. Foods 11(10), 1384.
| Crossref | Google Scholar | PubMed |
Ramatoulaye F, Mady C, Fallou S, Amadou K, Cyril D, Massamba D (2016) Production and use sorghum: a literature review. Journal of Nutritional Health & Food Science 4(1), 1-4.
| Crossref | Google Scholar |
Rosati VC, Quinn AA, Gleadow RM, Blomstedt CK (2024) The putative GATA transcription factor SbGATA22 as a novel regulator of Dhurrin biosynthesis. Life 14(4), 470.
| Crossref | Google Scholar | PubMed |
Siegień I, Fiłoc M, Staszak MA, Ciereszko I (2021) Cyanogenic glycosides can function as nitrogen reservoir for flax plants cultured under N-deficient conditions. Plant, Soil and Environment 67(4), 245-253.
| Crossref | Google Scholar |
Simon P (2003) Q-Gene: Processing quantitative real-time RT-PCR data. Bioinformatics 19(11), 1439-1440.
| Crossref | Google Scholar | PubMed |
Sohail MN, Blomstedt CK, Gleadow RM (2020) Allocation of resources to cyanogenic glucosides does not incur a growth sacrifice in Sorghum bicolor (L.) Moench. Plants 9(12), 1791.
| Crossref | Google Scholar | PubMed |
Sohail MN, O’Donnell NH, Kaiser BN, Blomstedt CK, Gleadow RM (2023) Wounding and methyl jasmonate increase cyanogenic glucoside concentrations in Sorghum bicolor via upregulation of biosynthesis. Plant Biology 25(4), 498-508.
| Crossref | Google Scholar | PubMed |
Sowiński J, Głąb L (2018) The effect of nitrogen fertilization management on yield and nitrate contents in sorghum biomass and bagasse. Field Crops Research 227, 132-143.
| Crossref | Google Scholar |
Sunaga Y, Harada H, Kawachi T, Hatanaka T (2008) Management of nitrogen fertilizer application rates based on soil nitrogen fertility with the goal of lowering nitrate nitrogen concentrations in Sudangrass (Sorghum sudanense (Piper) Stapf). Soil Science and Plant Nutrition 54(4), 543-554.
| Crossref | Google Scholar |
Wang B, Xiong W, Guo Y (2024) Dhurrin in sorghum: Biosynthesis, regulation, biological function and challenges for animal production. Plants 13(16), 2291.
| Crossref | Google Scholar | PubMed |
Woodrow IE, Slocum DJ, Gleadow RM (2002) Influence of water stress on cyanogenic capacity in Eucalyptus cladocalyx. Functional Plant Biology 29(1), 103-110.
| Crossref | Google Scholar | PubMed |