The genetic control of herkogamy
Jacques-Joseph Boucher A B , Hilary S. Ireland C , Ruiling Wang C , Karine M. David B and Robert J. Schaffer
A
B
C
Abstract
Herkogamy is the spatial separation of anthers and stigmas within complete flowers, and is a key floral trait that promotes outcrossing in many angiosperms. The degree of separation between pollen-producing anthers and receptive stigmas has been shown to influence rates of self-pollination amongst plants, with a reduction in herkogamy increasing rates of successful selfing in self-compatible species. Self-pollination is becoming a critical issue in horticultural crops grown in environments where biotic pollinators are limited, absent, or difficult to utilise. In these cases, poor pollination results in reduced yield and misshapen fruit. Whilst there is a growing body of work elucidating the genetic basis of floral organ development, the genetic and environmental control points regulating herkogamy are poorly understood. A better understanding of the developmental and regulatory pathways involved in establishing varying degrees of herkogamy is needed to provide insights into the production of flowers more adept at selfing to produce consistent, high-quality fruit. This review presents our current understanding of herkogamy from a genetics and hormonal perspective.
Keywords: floral development, herkogamy, mating systems, phytohormones, pollination, stamen development, style development.
Overview
Herkogamy (from the Greek for fence – herko and marriage – gamos) is broadly defined as the spatial separation of anthers and stigmas within complete flowers. It is a key composite floral trait that introduces variation in plant mating systems and is central to promoting outcrossing as well as reducing interference between male and female functions (Webb and Lloyd 1986). As a functional trait, herkogamy has long been considered an important component of reproductive interactions in angiosperms and plays a significant role in outcrossing rates and autofertility. Floral structures define herkogamy, with the spatial positioning of the ‘female’ receptive stigma and ‘male’ pollen producing anthers separated either vertically, laterally or both. The height of the stigma depends on the shape and size of the gynoecium (female organ comprising the ovary, style and stigma). The position of the anthers is determined by the length and angle of the filament that supports the anther.
Herkogamy types were described in detail in a seminal review by Webb and Lloyd (1986) and have been grouped into several additional categories, summarised in Table 1. The three main classes of herkogamy are: (1) homomorphic herkogamy; (2) reciprocal herkogamy; and (3) interfloral herkogamy. Homomorphic herkogamy is found in populations where all of the flowers have a similar morphology. Within homomorphic herkogamy, flowers can be ordered or unordered. In unordered herkogamy, there is still physical separation of the anthers and stigma but the spatial separation between them may be so small that pollinator movement between reproductive organs is disorganised or haphazard (i.e. pollinators repeatedly alternate between organs). In ordered herkogamy, there is a distinct physical separation of anthers and stigmas facilitating the likelihood that pollinators encounter one organ before the other when entering or exiting the flower. Ordered types include approach herkogamy (stigmas protruding beyond the anthers so that pollinators are likely to contact stigmas first) and reverse herkogamy (stigmas positioned behind or below the anthers so that pollinators contact anthers first) (Fig. 1). Movement herkogamy occurs in floral morphs where either the stigma or anthers move relative to each other, changing the separation as the flower develops or responds to pollination signals. Situations where anthers and stigmas are in direct line with each other may be referred to as neutral herkogamy, whilst no (or zero) herkogamy would imply the organs being in direct contact. Lateral herkogamy, where styles are horizontally positioned on an angle away from the centre of the flower, is the least common but can be seen in Linum (Ruiz-Martín et al. 2018), Centaurium (Brys and Jacquemyn 2012) and Hylocereus undatus (Cho and Ding 2021). Some species, such as Lysimachia arvensis exhibit both lateral and vertical herkogamy (Jiménez-López et al. 2019).
Type | Class | Order | Description | |
---|---|---|---|---|
Homomorphic | All floral morphs of one form; complete flowers | |||
Unordered | Pollinators contact anthers and stigmas within a flower in no ordered sequence | |||
Ordered | Typically, pollinators make contact with pollen and stigmas in an ordered fashion | |||
Approach | Stigmas extend forward of the anthers so that they contact pollinators first | |||
Reverse | Anthers presented forward of the stigma so that pollinators may contact them first | |||
Movement | Stigmas and/or anthers moved into or out of presentation position | |||
Lateral | Style presented horizontally on an angle away from the centre of flower | |||
Reciprocal | Two or more floral morphs of complete/perfect flowers but differing in position of presentation of anthers or stigmas. Heterostyly, enantiomorphism | |||
Interfloral | Two or more different floral morphs, with anthers and stigmas presented either in part or in whole within separate morphs. Monoecism, gynomonoecism, andromonoecism, dioecy |
Modified from Webb and Lloyd (1986).
Floral structure and homomorphic types of herkogamy. (a) Flower structure showing four whorls of organs – sepals, petals, stamens and carpels. (b) Hypothetical flower with petals removed showing no herkogamy. (c) Lateral herkogamy. (d) Approach herkogamy. (e) Reverse herkogamy. ‘h’ represents the herkogamy distance.
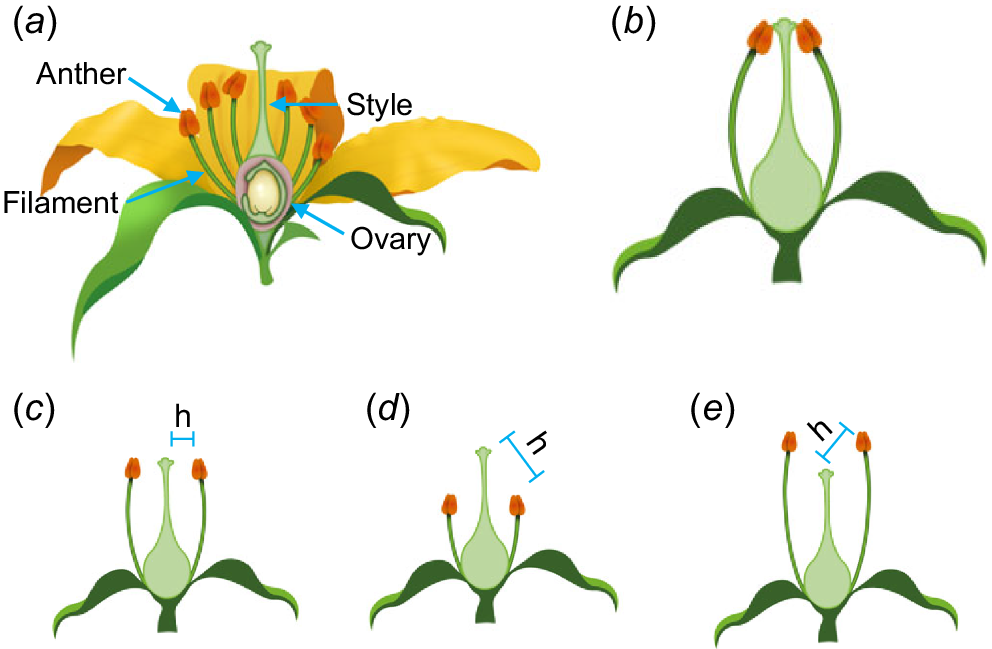
Reciprocal herkogamy is classed as either heterostyly or enantiostyly/enantiomorphy (Webb and Lloyd 1986; Almeida et al. 2013, 2018). Heterostyly (Fig. 2) refers to the system where herkogamy is vertical and presented reciprocally in two (distyly) or three (tristyly) floral morphs (Arunkumar et al. 2017). Effectively, distylous species have two different floral morphs (short-styled S-morphs, or thrums; and long-styled L-morphs, or pins) with one presenting approach herkogamy and the other reverse herkogamy. In tristylous species, a third floral morph is present that has an intermediate herkogamy profile relative to the other two morphs. In some reproductive systems, the flowers of a particular morph may only be compatible with those from different morphs – demonstrating organ separation through space working in concert with incompatibility systems (Barrett and Harder 2005; Arunkumar et al. 2017).
Variations in floral structures. (a) Distyly. (b) Tristyly (anther and stamen lengths displaying enantiomorphy). (c) Interfloral herkogamy: monoecism (two floral morphs within a plant). (d) Dioecy (plants have either only ‘male’ or ‘female’ flowers). Dotted red lines indicate pollen transfer between organs of different morphs.
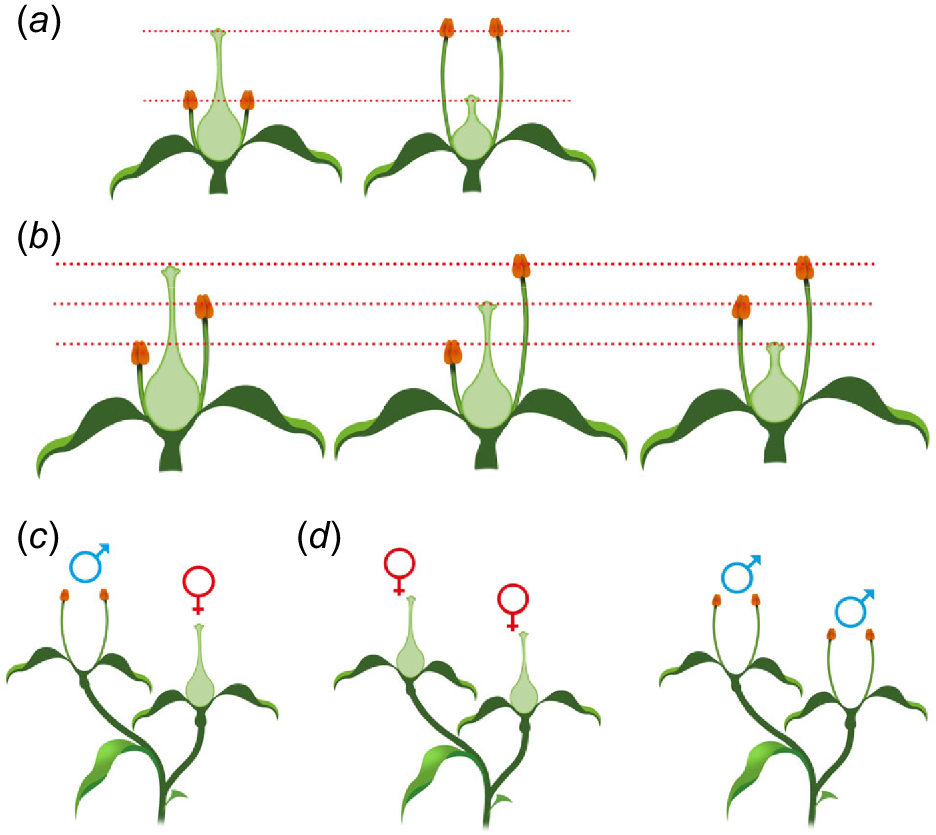
Interfloral herkogamy is when a plant contains two or more different floral morphologies, with anthers and stigmas presented either in part or in whole within separate morphs and includes monoecism (Fig. 2) (separate male and female flowers on an individual plant), gynomonoecism (female and complete flowers on the same individual plant) and andromonoecism (male and complete flowers on individual plants of a species).
Homomorphic, ordered, approach herkogamy is the most common amongst self-compatible species and tends to be associated with a limited number of pollinator species or functional groups (Opedal 2018). It can be found in the families Liliaceae, Amaryllidaceae, Boraginaceae, Convolvulaceae, Caprifoliaceae, Ericaceae, Papaveraceae and Gentianaceae (Kerner and Oliver 1902). The relationship between diverse pollinator communities, functional groups and plant reproductive success will not be covered in detail here but has been treated extensively by others (Fontaine et al. 2006; Albrecht et al. 2012; Ollerton 2017).
Function and evolution of herkogamy
Herkogamy has long been considered an important component of reproductive interactions in angiosperms and has been demonstrated to play a significant role in outcrossing rates and autofertility. While traditional interpretations of herkogamy posit its role mainly as a promoter of outcrossing (thus a mechanism to reduce selfing), its presence in species that are genetically self-incompatible, as well as the presence of other promoters of outcrossing such as dichogamy and dioecy, suggests it may also serve to prevent or reduce anther-stigma self-interference within a flower (Galen and Gregory 1989; Waser and Price 1991; Fetscher 2001). Self-interference may occur when self-sourced pollen saturates the stigmatic surface and clogs it so that outcrossed pollen is restricted from making contact and germinating, or when stamens remove pollen from stigmatic surfaces or block access to the stigma. Stigmas, depending on floral architecture, may also create a barrier to pollen access or redirect released pollen. However, in species that exhibit at least some degree of self-compatibility, it is logical to conclude that the spatial separation of anthers and stigmas will reduce the likelihood of self-fertilisation (Webb and Lloyd 1986).
Large-scale work on floral evolution within angiosperms have predicted early floral structures (Sauquet et al. 2017), but potential evolvability (capacity to adapt to changing conditions or pressures) of herkogamy, was calculated to be high and rapid in response to environmental changes and that the high degree of herkogamic variation often encountered within related species and populations could result from adaptive responses to the pollination environment (Opedal et al. 2017). It was found that the median evolvability of herkogamy was an order of magnitude greater than that of other floral measurements and was not strongly constrained by genetic covariance between pistil and stamen lengths. They concluded that herkogamy can rapidly evolve in response to environmental changes, suggesting that variation in herkogamy in related species and populations may result from variation in pollinator communities and other selective factors. More recent work (Opedal et al. 2022) suggests that the evolvability of certain floral traits varies throughout phenotypic space and that particular trait combinations may be subject to strong genetic constraints that stall evolution towards optimum self-pollination rates. This work on the potential genetic constraints of functionally interactive floral traits (Opedal et al. 2022) reinforces herkogamy’s high level of independent evolutionary potential.
Effect of herkogamy on pollination success
Numerous studies have demonstrated negative relationships between herkogamy and rates of autofertility (seed set in the absence of pollinators) and self-fertilisation (Moeller 2006; Herlihy and Eckert 2007; Eckert et al. 2009; Dart et al. 2012; Opedal et al. 2016), speaking to the functional importance of the trait. Additional studies have demonstrated positive relationships between pollinator abundance and herkogamy (Moeller 2006; Opedal et al. 2016).
The separation of pollen-producing anthers from receptive stigmas can influence rates of self-pollination amongst plants (Müller 1883). The degree of stigma exsertion (relative to anthers) has a major influence on the efficacy of self-pollination in many types of plants and is mainly determined by the relative lengths of anthers, styles and ovaries (Shang et al. 2021). Reducing herkogamy can lead to improved rates of successful self-pollination in species that are self-compatible (Luo and Widmer 2013; Toräng et al. 2017; Opedal 2018). Logically, reducing the space between anthers and stigmas may lead to increased or enhanced self-pollen transfer during both biotic and abiotic-induced floral movements. Approach and reverse herkogamy have been shown to have considerably different effects on pollen loads, outcrossing rates and seed set (Barrett 2002). Approach herkogamous flowers were shown to have captured less total pollen but exhibited much higher rates of outcrossing than reverse herkogamous flowers, supporting Webb and Lloyd’s (1986) assumption that approach herkogamy was superior in preventing self-pollination.
Predominately, studies that have investigated herkogamy-outcrossing or herkogamy-autofertility relationships have demonstrated that more herkogamous individuals, populations, and species tend to be more outcrossed (reviewed by Opedal 2018). The effect of greater anther-stigma separation on reducing individual selfing rates has been established for numerous taxa (Rick et al. 1978; Brunet and Eckert 1998; Motten and Stone 2000; Takebayashi et al. 2006). Takebayashi et al. (2006) demonstrated a strong positive association between herkogamy and outcrossing rates in Gilia achilleifolia, which supports previous findings. Certainly, within populations, flowers exhibiting greater herkogamy do often tend to be more outcrossed (Opedal 2018). This can result from reduced interference between anther and stigmas when they are highly separated. In self-incompatible species, reduced herkogamy could lead to pollinators being restricted from stigmas or lead to an increase in self-pollen deposition leading to clogged stigmas or increasing competition between self- and cross-pollen (Fægri and Van der Pijl 1979; Opedal 2018).
Variation of herkogamy within species
Herkogamy can exhibit significant variation among genotypes within a species and has been proposed to show local adaptation to the pollination environment (Opedal 2018). In tomato (Solanum lycopersicum), this variation is evident, particularly between self-incompatible wild relatives and autogamous domesticated varieties.
Among wild tomato relatives such as Solanum pennellii and Solanum peruvianum, exserted stigmas and allogamous breeding are prevalent traits (Rick and Lamm 1955; Rick 1982, Rick and Chetelat 1991). In contrast, commercially important domesticated varieties like S. lycopersicum exhibit reverse herkogamy, where stigmas are inserted within the anther cone, facilitating self-pollination (Rick and Lamm 1955; Rick et al. 1977; Karron et al. 1997; Motten and Stone 2000). The transition to inserted stigmas in domesticated tomatoes likely occurred alongside the development of self-compatibility during domestication (Georgiady and Lord 2002; Georgiady et al. 2002; Blanca et al. 2012).
Research by Liu et al. (2016) on Primula species demonstrated that reducing the distance between anthers and stigmas resulted in decreased outcrossed pollen deposition and increased self-pollen deposition. However, total pollen deposition remained relatively unaffected. Opedal (2018) further explored the qualitative relationships between herkogamy and mating systems, suggesting a negative association between herkogamy and autofertility, with potentially more pronounced declines in approach herkogamous species. However, the relationship between herkogamy and outcrossing rates remains challenging to elucidate due to complex interactions with pollinators (Opedal 2018).
Hormonal control of herkogamy
The growth and development of all tissues in plants are intricately regulated by the interplay of phytohormones. From the inception of floral primordia, auxin serves as a morphogenetic catalyst, facilitating the formation of primordia. Subsequently, it collaborates with other hormones to orchestrate the intricate processes of development, patterning and functioning of reproductive organs (Dresselhaus and Schneitz 2014; Zažímalová et al. 2014).
Cytokinin plays a crucial role in gynoecium patterning, particularly in early cell proliferation and inducing medial tissues in developing gynoecia (Marsch-Martínez et al. 2012). Cytokinin positively regulates reproductive meristems, which are relevant to inflorescence architecture. It has been proposed that high auxin levels are necessary for stigma and style development, low auxin and cytokinin induce ovary and ovule development, and high cytokinin levels regulate gynophore formation. Moreover, auxin signalling during gynoecium development has been associated with brassinosteroid signalling (Marsch-Martínez and de Folter 2016).
Stamen development in Arabidopsis thaliana is influenced by auxin, gibberellic acid (GA) and jasmonic acid (JA) coordinating and regulating various aspects of stamen development (Song et al. 2013). This includes stamen primordia formation, filament and anther development, pre-anthesis filament elongation, pollen maturation and anther dehiscence. Auxin transport from the tapetum through the middle layer and toward the filament is essential for coordinating anther maturation with filament growth (Cecchetti et al. 2017). Auxin is also involved in regulating stamen number and outgrowth (Heisler et al. 2005).
Defects in GA biosynthesis or perception result in shortened stamen filaments (Cheng et al. 2004; Tyler et al. 2004; Rieu et al. 2008a, 2008b). GA induces the synthesis of JA. However, the short stamen phenotype of GA-deficient plants cannot be rescued by exogenous JA, suggesting the involvement of other GA-dependent, JA-independent pathways in correct stamen filament elongation (Cheng et al. 2009).
Mutations in JA biosynthesis genes or components of the JA signalling pathway affect filament elongation (Xie et al. 1998; Stintzi and Browse 2000; Ishiguro et al. 2001; Park et al. 2002). Moreover, the development of stamens, including filament elongation, anther dehiscence and pollen maturation, is regulated by various environmental cues and endogenous hormone signals, including GA and JA (Ma 2005; Plackett et al. 2011; Song et al. 2013).
In species exhibiting distyly, local changes in genes associated with brassinosteroids have been attributed to the control of stamen and style length (detailed below). The application of brassinosteroids to primrose S-morphs caused an increase in style length, but not stamen length (Huu et al. 2016).
Genetic control of herkogamy
As herkogamy is determined by the positioning of anthers and stigmas in the flower, there is a need to understand the molecular control of growth and development of the gynoecium and stamens (filaments and anthers). The molecular regulation of growth and development of these floral structures have been extensively reviewed (Herrera-Ubaldo et al. 2018, 2023; Thomson and Wellmer 2019) so will not be covered in detail here. Key to the determination of the gynoecium and stamens are the floral homeotic B, C and E function genes. The C function gene AGAMOUS (AG) together with the E function genes SEPALLATA (SEP) determine gynoecium development, and AG togetherwith PISTILLATA (PI), APETALA3 (AP3) and SEP genes determine stamen development.
This review will focus on genes that have been reported to alter the differential length of these organs with respect to herkogamy. As may be expected, many of these genes are associated with the hormones detailed in the section above. It is well documented that hormones work together to control different aspects of development, and this seems to be the case in the control of gynoecium length and stamen length. As the genetic control of the gynoecium and stamen are independently controlled, for this review we will examine the molecular control of these organs separately.
Molecular control of gynoecium development
Variability in gynoecium length may be determined by ovary length, or, more commonly, style length. The gynoecium in the model plant Arabidopsis is predominantly ovary tissue with very little style tissue. However, there are a number of mutations associated with stylar tissue (Table 2, Fig. 3a). Genes associated with Arabidopsis ovary length appear to be key regulators of herkogamy with an E3 ubiquitin ligase gene, specifically ARM-Repeat Containing1 (ARC1), being shown to control ovary length among different Arabidopsis ecotypes and promote approach herkogamy in Arabidopsis flowers (Indriolo et al. 2014). In other model species, such as S. lycopersicum, the style comprises the majority of the gynoecium length.
Organism | Gene | Gene model number | Gene class | Function | References | |
---|---|---|---|---|---|---|
Brassica rapa | ARM Repeat Containing1 (ARC1) | LOC103865322 | U-box domain-containing protein | Controls ovary length; promotes approach herkogamy in A. thaliana | Indriolo et al. (2014) | |
Arabidopsis thaliana | STIGMA AND STYLE STYLIST (SSS) | AT1G56020 | Unknown gene; serine/arginine repetitive matrix-like protein | Suppresses style elongation | Li et al. (2020) | |
A. thaliana | NGATHA1 (NGA1) | AT2G46870 | AP2/B3-like transcriptional factor | Involved in stigma development and style elongation | Alvarez et al. (2009) and Fourquin and Ferrándiz (2014) | |
Solanum lycopersicum | Long styles (Lst) | Solyc12g027610.1 | Ethylene receptor EIN4-like | Inhibitor of style exsertion | Cheng et al. (2021) | |
S. lycopersicum | Style2.1 (Se2.1) | Style21 | Polypeptide bearing a helix-loop-helix; transcription factor | Promotes style growth | Chen et al. (2007) | |
S. lycopersicum | Stigma exsertion 3.1 (SE3.1) | Solyc03g098070 | C2H2 zinc finger transcription factor | Regulates anther and style length to control flush stigma development | Shang et al. (2021) | |
S. lycopersicum | Woolly (Wo) | Solyc02g080260 | HD ZIP (IV) | Regulates the expression of SE2.1 | Wu et al. (2024) | |
Oryza sativa subsp. japonica | GRAIN SIZE 3 (GS3) | OSNPB_030407400 | M-type_MADS | Regulates stigma length and exsertion | Takano-Kai et al. (2011) | |
Primula forbesii | CYP734A50 | AOA32958 | Cytochrome P450 | Inactivates brassinosteroid mediated growth activity | Huu et al. (2016) | |
Turnera subulata | TsBAHD | QDP16902 | Cytochrome P450 | Homologous to CYP73A50 similar to above | Shore et al. (2019) |
Genes and hormones shown to control (a) ovary and style length and (b) filament length. Ovals, genes; red, Arabidopsis; green, tomato; blue, rice; purple, Primula/Turnera; clouds, hormones; IAA, auxin; BR, brassinosteroids; CK, cytokinins; ET, ethylene; JA, jasmonic acid; GA, gibberellins; arrows, positive reported relationships; lines with bars, negative reported relationships.
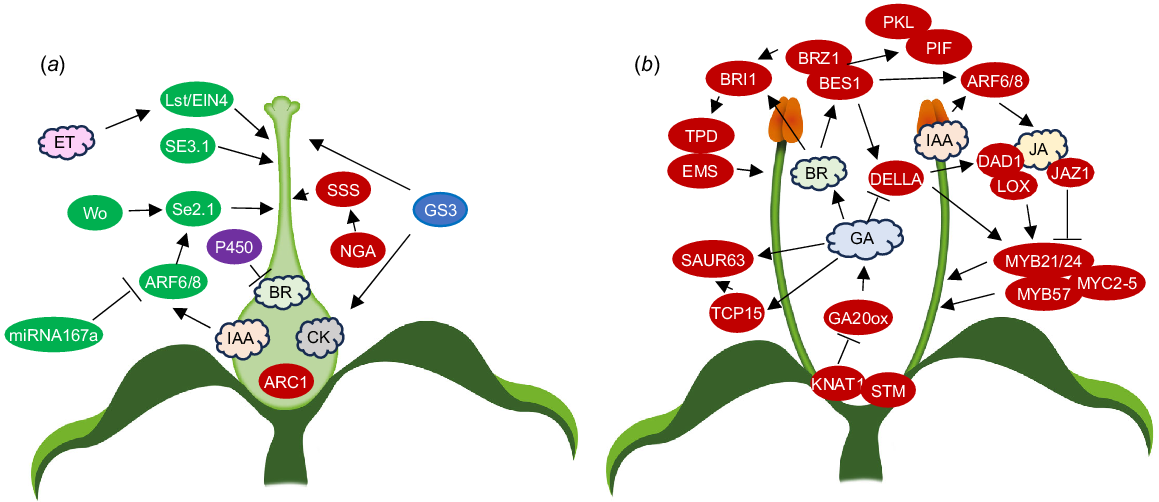
Style development appears to be associated with marginal tissue development, with the style positioned at the distal end of the gynoecium. Disruption of marginal tissue and organ fusion often leads to a loss of stylar tissue. The MADS-box transcription factor SHATTERPROOF (SHP) (Colombo et al. 2010) and other key transcription factors AUXIN RESPONSE FACTOR6/8 (ARF6/8), CRABSCLAW (CRC), AINTEGUMENTA (ANT), FILIMENTOUS FLOWER (FIL), SUESS (SEU), LUNIG (LUG) and SPATULA (SPT) have been characterised through mutation studies and shown to be associated with marginal tissue and style development. In cases where these genes are mutated individually or in combination, Arabidopsis gynoecia fail to fuse and often lack stylar and/or stigmatic tissue (Bowman et al. 1999; Liu et al. 2000; Alvarez and Smyth 2002; Groszmann et al. 2008).
The STYLISH1 (STY/SHI) gene family comprises zinc-finger transcription factors expressed in the gynoecium, ovule primordia and style. Their status as downstream genes has been established through genetic investigations, wherein lug mutations were found to be epistatic over sty mutations, and lug mutants exhibited reduced expression of STY1 (Kuusk et al. 2006). Ectopic expression of STY1 rescues style defects of lug, seu, crc, jag (JAGGED) and spt mutant gynoecia (Ståldal et al. 2008). Supporting the downstream status of STY1 from these genes, sty1 mutants result in only minor style development defects compared with mutations in the upstream genes (Sundberg and Ferrándiz 2018). The auxin biosynthesis gene YUCCA4 (YUC4) is among the downstream targets of STY1, indicating that SHI/STY family genes have a positive regulatory effect on local auxin biosynthesis (Sohlberg et al. 2006; Eklund et al. 2010). Supporting this, the application of exogenous auxin on the apical region of immature sty1 sty2 mutant gynoecia leads to a reversion to the wild-type style and stigma (Sundberg and Ferrándiz 2018).
Once patterning has been established, the control of style length has been studied using mutants in Arabidopsis and by gene mapping in other species. The Arabidopsis NGATHA (NGA) B3-domain transcription factors (Alvarez et al. 2009; Trigueros et al. 2009) serve as redundant regulators influencing the establishment of apical gynoecium and stylar length. Inactivation of individual or multiple NGA members leads to elongated and misshapen stylar tissue. Fourquin and Ferrándiz (2014) identified NGA orthologues in the longer-styled species Eschscholzia californica (Californian poppy) and Nicotiana benthamiana. Expression patterns of EcNGA and NbNGA genes closely resembled Arabidopsis NGA. Inactivation of these genes caused severe defects in style. Downstream of the NGA transcription factors are the STIGMA AND STYLE STYLIST (SSS) genes (Li et al. 2020), which encode a novel class of angiosperm-specific genes with unknown function. Arabidopsis possesses three partially redundant SSS genes with slightly different expression zones. Similar to nga mutants, suppression of SSS genes leads to elongated stylar tissue, whereas overexpression of SSS causes significant reduction in stylar length. Moreover, SSS overexpression effectively rescues the stylar defect in nga1nga3 double mutants, affirming the pivotal role of NGA transcription factors in regulating these genes.
Genetic investigations into stigma position in tomato have revealed a quantitative determination influenced by relatively few genes with high heritability and dominance effects (Rick and Dempsey 1969). One major quantitative trait locus (QTL) named style2.1 (se2.1) was identified on chromosome 2 (Chr2) using an interspecific mapping population derived from S. lycopersicum and Solanum habrochaites (Bernacchi and Tanksley 1997). The gene responsible for se2.1 was subsequently characterised and found to encode a polypeptide with a helix-loop-helix (HLH) motif, but lacking the typical basic region found in the broader class of basic-helix-loop-helix (bHLH) transcription factors (Chen et al. 2007). The SE2.1 protein promotes cell elongation in the style. The effect of the se2.1 allele can be attributed to a deletion in the promoter region and a resultant decrease in gene transcription in cultivated tomato, explaining part of the transition from long to short styles (Chen et al. 2007; Vosters et al. 2014). SE2.1 expression has been shown to be controlled by three HD-ZIP proteins, WO, HD7, HD7L. A dominant mutation of WO (woW106R) causes further style-length reduction to create reverse herkogamy (Wu et al. 2024). Additional QTLs for style exsertion have been identified through interspecific biparental populations. Three QTLs were reported on Chr4, Chr8 and Chr9 using a cross with Solanum pimpinellifolium (Georgiady et al. 2002), and one QTL was found on the long arm of Chr5 using a cross with S. habrochaites (Gorguet et al. 2008). Another locus, SE3.1, was identified through a genome-wide association study (GWAS) (Shang et al. 2021). This study identified a mutation in a C2H2 zinc finger transcription factor that plays a role in controlling stylar length. The long styles (Lst) phenotype in S. lycopersicum was suggested to be linked to an EIN4-like ethylene receptor (Solyc12g027610.1), implicating ethylene in conferring this style exsertion phenotype (Cheng et al. 2021).
Riccini et al. (2021) demonstrated a significant involvement of genes related to indole-3-acetic acid (IAA) function in exserted stigma genotype groups in tomato, supporting the accepted role of auxin in the apical-basal patterning development of the gynoecium in Arabidopsis (Ståldal and Sundberg 2009). In tomato, downregulation of ARF6 and ARF8 via miRNA167 overexpression resulted in shortened floral organs compared with control plants (Liu et al. 2014). This phenotype was accompanied by a decrease in SE2.1 expression, suggesting that ARF6 and ARF8 may function upstream of SE2.1, and the reduced expression of SE2.1 in MIR167a-plants contributes to reduced style growth (Liu et al. 2014).
In rice (Oryza sativa), GRAIN SIZE 3 (GS3), a MADS box gene known for regulating seed length, has been found also to influence style and (non-brush) stigma length resulting in stigma exsertion (Takano-Kai et al. 2011). Its basal mRNA expression is detected in young rice stigmas, and a nonsense mutation in its second exon leads to cell proliferation, resulting in elongated stigmas.
In distylous species, both the stamen and the style length are controlled by an ‘S-locus’ containing several genes recently reviewed (Kappel et al. 2017). In Primula (Huu et al. 2016, 2022) and Turnera (Shore et al. 2019; Matzke et al. 2021), cytochrome P450 genes that are homologous to Arabidopsis CYP72B1 (BAS1) have been shown to control style length. BAS1 modulates brassinosteroid associated growth (Neff et al. 1999) providing a possible mechanism for how this class of gene can regulate different stylar morphs (Henning et al. 2020).
In the mangrove species Lumnitzera littorea, Zhang et al. (2020) identified 12 MADS-box genes, including four MIKC-type and eight M-type transcription factors, associated with herkogamy. Some species exhibit stylar polymorphisms, leading to individuals with either approach or reverse herkogamy. Several major loci influencing such polymorphisms have been identified in genetic studies (Barrett et al. 2000; Ushijima et al. 2012; Nowak et al. 2015). Additionally, in other species, style length exhibits continuous variation, resulting in individual flower profiles ranging between approach and reverse herkogamy (Motten and Stone 2000; Takebayashi et al. 2006). The regulation of style length and stamen position often involves multiple loci with minor effects, and covariation between floral organs is not uncommon (Conner and Sterling 1996; Herrera 2001; Kulbaba and Worley 2008). Lande and Arnold (1983) suggest this continuous covariation could limit the evolvability of herkogamy, in contrast to earlier studies that proposed it to be highly evolvable (Opedal et al. 2017).
In an RNA-seq comparison of inserted- and exserted-stigma tomato genotypes (Riccini et al. 2021), the exserted genotypes showed an over-representation of genes involved in regulating cell wall structure and metabolism. Notably, two xyloglucan endotransglucosylase-hydrolases (XTH1 and XTH7), three expansins or expansin-precursor genes (EXP5, EXP12 and EXPA4), genes related to sucrose metabolism (STD1, SPS, SUSY), and the auxin efflux facilitator PIN-FORMED3 (PIN3) were identified among the cell wall-related genes in the exserted genotypes.
Molecular control of stamen development
Stamens, consisting of filaments and anthers and collectively known as the androecium, produce male gametophytes (D’Arcy 1996). The floral homeotic gene AG regulates stamen specification in part through JA genes (Bowman et al. 1991; Sieburth and Meyerowitz 1997). AG directly regulates DEFECTIVE IN ANTHER DEHISCENCE1 (DAD1), a JA catalytic enzyme, and controls stamen identity and differentiation through transcriptional cascades at different floral stages, including the regulation of SPOROCYTLESS/NOZZLE (SPL/NZZ) and JA biosynthesis (Ito et al. 2004, 2007).
For correct stamen development in Arabidopsis (Table 3, Fig. 3b), repression of Class I KNOX genes must occur during early flower development (Tabata et al. 2010; Rubio-Somoza and Weigel 2013). At late pre-anthesis, stamen growth typically increases rapidly before decreasing as the stamen reach the top of the pistil (Tashiro et al. 2009). In post-anthesis stages, it has been suggested that KNAT1 works redundantly with (SHOOTMERISTEMLESS) STM to limit stamen growth (Gastaldi et al. 2023). KNAT1 overexpression was shown to strongly reduce filament elongation. Defective GA synthesis causes reduced filament elongation (Rieu et al. 2008b). KNAT1 overexpression decreases transcription levels of the GA biosynthesis gene GA20OX1, indicating filament elongation may be repressed by KNAT1 via regulation of GA biosynthesis (Gastaldi et al. 2023). Late filament elongation is stimulated by members of the TCP transcription factor family and KNAT1 directly targets TCP15 to modulate filament elongation (Gastaldi et al. 2020, 2023). Class I TCPs are needed for the GA induction of SMALL AUXIN UP RNA 63 (SAUR63) subfamily genes. SAUR proteins promote cell expansion by activating plasma membrane H+-ATPases (Spartz et al. 2014) and overexpression of SAUR63 subfamily members stimulates stamen filament elongation (Chae et al. 2012). SAUR63 overexpression in plants expressing a fusion of TCP15 to a repressor domain (pTCP15::TCP15-EAR) rescued short stamens whilst TCP15 overexpression did the same in GA-deficient plants (Gastaldi et al. 2020).
Organism | Gene | Gene model number (Locus tag) | Gene class | Function/Phenotype | References | |
---|---|---|---|---|---|---|
Arabidopsis thaliana | BREVIPEDICELLUS/KNAT1 | AT4G08150 | Class 1 knotted-like homeobox gene | Represses TCP15 to limit filament growth | Gastaldi et al. (2020) | |
A. thaliana | SHOOT MERISTEMLESS (STM) | AT1G6230 | KNOX/ELK homeobox transcription factor | Works redundantly with KNAT1 to restrict stamen growth | Gastaldi et al. (2023) | |
A. thaliana | REPRESSOR OF GA/RGA-like2 (RGA)/(RGA2) | AT2G01570/AT3G03450 | GRAS family TF; DELLA protein | Inhibitors of stamen growth | Cheng et al. (2009) | |
A. thaliana | TCP15 | AT1G69690 | TCP family TF | Activates SAUR63 in filament elongation | Gastaldi et al. (2020, 2023) | |
A. thaliana | DAD1 | AT2G44810 | alpha/beta-Hydrolases superfamily protein | Anther dehiscence; catalyses the initial step of jasmonic acid biosynthesis. | Ishiguro et al. (2001) | |
A. thaliana | MYB21/MYB24 | AT3G27810/AT5G40350 | TF; MYB domain protein 21/24 | Differentiation and development of stamen. Induced by jasmonate. | Mandaokar et al. (2006) | |
A. thaliana | MYB57 | AT3G01530 | TF; R2R3 factor gene family | Specifies stamen identity and filament length | Cheng et al. (2009) | |
A. thaliana | BZR1/BES1 (BRASINOSTEROID-RELATED GENES) | AT1G75080 | Brassinosteroid signalling positive regulator (BZR1) family protein | Influence cell elongation and stamen size | Chen et al. (2019) | |
A. thaliana | SMALL AUXIN UP RNA 63 subfamily (SAUR63) | AT1G29440 | SAUR-like auxin-responsive protein family | Overexpression restores filament growth | Gastaldi et al. (2020) | |
A. thaliana | LIPOXYGENASE (LOX1) | AT1G55020 | Lipoxygenase 1; protein coding JA-related gene | Activates MYB TFs to mediate filament elongation | Cheng et al. (2009) | |
A. thaliana | Jasmonate zim domain protein 1 (JAZ1) | AT1G19180 | Jasmonate signalling nuclear localised protein | Represses MYB21 and MYB24 to inhibit stamen filament elongation | Huang et al. (2020) | |
A. thaliana | AUXIN RESPONSE FACTOR6/8 (ARF6/8) | AT1G30330 (ARF6) AT5G37020 (ARF8) | ARF transcription factors | Induce expression of JA biosynthesis in stamen development; ARF8.4 variant needed for filament elongation | Ghelli et al. (2023) | |
A. thaliana | EMS1/EXS (EXCESS MICROSPOROCYTES1) | AT5G07280 | Leucine-rich repeat transmembrane protein kinase | Regulates somatic and reproductive cell fates in anther development | Bai et al. (2023) | |
A. thaliana | BRASSINOSTEROID INSENSITIVE 1 (BRI1) | AT4G39400 | Leucine-rich receptor-like protein kinase family protein | Regulates filament elongation via the downstream activity of BZR1/BES1 | Bai et al. (2023) |
In Arabidopsis, DELLA proteins play a crucial role in regulating phytohormones for filament elongation (Huang et al. 2020). Cheng et al. (2009) demonstrated that DELLA proteins REPRESSOR OF GA (RGA) and RGA-like2 (RGL2) were principal players in inhibition of stamen development. GAs supress DELLA proteins and upregulate the JA-related genes DAD1 and LIPOXYGENASE (LOX1) to activate MYB21, MYB24 and MYB57 transcription factors to mediate filament elongation (Cheng et al. 2009). Huang et al. (2020) further demonstrated that DELLAs directly targeted MYB21 and MYB24 that govern GA-JA interaction in filament elongation and that co-expression of DELLA (RGL2) and JASMONATE ZIM-domain (JAZ1) proteins repressed MYB21 and MYB24 to inhibit filament elongation.
Mutations in genes associated with auxin, specifically AUXIN RESPONSE FACTOR genes ARF6 and ARF8, lead to deficiencies in stamen filament elongation and anther maturation (Nagpal et al. 2005; Cecchetti et al. 2008; Tashiro et al. 2009; Tabata et al. 2010; Reeves et al. 2012). Specifically, a splice variant of ARF8 (ARF8.4) is required for stamen filament elongation and correct expression of the auxin-inducible gene AUX/IAA19 (Ghelli et al. 2023). ARF6 and ARF8 have been reported to induce the expression of JA biosynthesis genes during late stages of stamen development, suggesting that auxin acts upstream of JA (Nagpal et al. 2005; Tabata et al. 2010; Song et al. 2011; Reeves et al. 2012). In arf6 arf8 double null mutants, plants produce short filaments and un-dehisced anthers whilst delayed filament elongation is observed in arf6 and arf8 single mutants homozygous for one mutation and heterozygous for the other (Nagpal et al. 2005; Wu et al. 2006; Tabata et al. 2010; Reeves et al. 2012). However, the fact that stamen filament elongation is not rescued by JA treatment in arf6 arf8 mutants (Nagpal et al. 2005) indicates the involvement of additional pathways. Among the genes downregulated in arf6 arf8 mutant flowers, several previously mentioned SAUR genes from the SAUR63 subfamily were identified (Nagpal et al. 2005). Therefore, the induction of SAUR genes by ARF6 and ARF8 may be required, in addition to JA biosynthesis, to stimulate filament elongation as JA synthesis induces expression of MYB21 and MYB24 (Reeves et al. 2012).
Qi et al. (2015) previously demonstrated that transcription factors MYC2–5 were JAZ-targeted proteins that worked redundantly to regulate filament elongation and formed a complex with MYB21 and MYB24 in filament elongation in Arabidopsis. In myb21 null mutants, filament elongation is prevented (Mandaokar et al. 2006) and anther opening delayed but pollen remains viable, whilst in myb21 myb24 double mutants filament elongation, anther dehiscence and pollen viability are all affected, suggesting MYB21 is essential for filament elongation and works redundantly with MYB24 for anther dehiscence and pollen viability (Mandaokar et al. 2006; Reeves et al. 2012; Acosta and Przybyl 2019).
The brassinosteroid-related gene BZR1 and its close homologue BES1 (BZR1/BES1) influence cell elongation to affect stamen length (Chen et al. 2019). DELLAs, PIFs, ARF6, and PKL directly interact with BZR1/BES1 to form a cell elongation regulatory network (Li et al. 2018). BZR1/BES1’s transcriptional activity can be constrained by the direct physical interaction of DELLAs leading to restrained cell elongation (Li et al. 2012). Also involved in influencing stamen development in Arabidopsis are EMS1/EXS. Bai et al. (2023) reported the transgenic expression of brassinosteroid receptor BRI1 rescued short filaments in ems1 mutants that were deficient in stamen elongation whilst co-expression of EMS1 and TPD1 rescued short filaments in bri1 mutants (Bai et al. 2023). Subsequently, EMS1 and BRI1 regulate filament elongation via the downstream activity of BZR1/BES1 (Bai et al. 2023).
Environmental effects on herkogamy
Herkogamy is subject to the influence of various environmental factors in different plant species. Environmental factors, such as water relations, temperature and light quality, have been shown to influence herkogamy by affecting floral development. In Arabidopsis, temperature and genotype have been suggested as determinants of herkogamy (Luo and Widmer 2013). Similarly, in tomato, style elongation has been linked to genotype dependence and sensitivity to relatively high temperatures (Peralta et al. 2005). Saeed et al. (2007) found that the length of the style of different tomato genotypes increased by 25–55% under high temperatures, whilst Sakata et al. (2010) demonstrated that auxin levels in tomato decreased under high temperature conditions leading to shorter filaments. The possibility of the opposite effect on pistils under high temperatures, resulting in stigma exsertion, has been proposed (Riccini 2019; Riccini et al. 2021). Additionally, it was observed that higher temperatures were needed to cause tomato stigma exsertion in the STlst1 mutation (Cheng et al. 2021).
In the high-altitude plant Aquilegia coerulea, Van Etten and Brunet (2013) observed phenotypic plasticity in herkogamy in response to changes in both water availability and temperature. Plants subjected to higher water treatments exhibited longer styles, while stamen lengths remained unchanged. In contrast, higher temperatures decreased the length of both styles and stamens, resulting in varying effects on herkogamy across different study populations in Colorado, Utah and Arizona with no change, a decrease, and an increase in herkogamy, respectively.
In a study on Arabidopsis and Brassica floral organs in response to light quality, Weinig (2002) found that wild-type Arabidopsis exposed to low red:far-red (R:FR) light had diminished petal and pistil lengths but longer filaments relative to petal size compared to those exposed to high R:FR light. Similar results were observed for Brassica. Arabidopsis mutants lacking phytochrome A exhibited smaller petals, pistils, and filaments, while those deficient in phytochrome B had longer filaments (Weinig 2002).
Concluding remarks
Herkogamy is an important floral trait that plays a crucial role in promoting cross-pollination and maintaining genetic diversity in plant populations. The genetic and hormonal control of herkogamy is a complex and dynamic process that involves multiple genetic pathways and environmental factors. Abiotic factors such as water availability, temperature, and light quality also play significant roles in modulating floral development and affecting herkogamy.
Recent advances in genomic and molecular techniques have greatly expanded our understanding of the genetic and hormonal regulation of herkogamy. These advances have helped to identify key genes and pathways that control herkogamy and have shed light on the molecular mechanisms underlying the development and maintenance of the trait.
However, there is still much to learn about the genetic and hormonal control of herkogamy, particularly in non-model plant species. Future research should focus on identifying additional genes and pathways involved in herkogamy regulation, as well as exploring the role of environmental factors and epigenetic modifications in herkogamy development.
Data availability
Data sharing is not applicable as no new data were generated or analysed during this study.
Declaration of funding
This work was funded by a grant from the New Zealand Ministry of Business, Innovation and Employment, SSIF grant C11X1702 to the New Zealand Institute for Plant and Food Research.
References
Acosta IF, Przybyl M (2019) Jasmonate signaling during Arabidopsis stamen maturation. Plant and Cell Physiology 60(12), 2648-2659.
| Crossref | Google Scholar | PubMed |
Albrecht M, Schmid B, Hautier Y, Müller CB (2012) Diverse pollinator communities enhance plant reproductive success. Proceedings of the Royal Society B: Biological Sciences 279(1748), 4845-4852.
| Crossref | Google Scholar |
Almeida NM, Castro CC, Leite AV, Novo RR, Machado IC (2013) Floral polymorphism in Chamaecrista flexuosa (Fabaceae-Caesalpinioideae): a possible case of atypical enantiostyly? Annals of Botany 112(6), 1117-1123.
| Crossref | Google Scholar | PubMed |
Almeida NM, Souza JT, Oliveira CRS, Bezerra TT, Novo RR, Siqueira Filho JA, Oliveira PE, Castro CC (2018) Functional dimorphic enantiostyly in monomorphic enantiostylous species of the subtribe Cassiinae (Fabaceae-Caesalpinioideae). Plant Biology 20(4), 797-801.
| Crossref | Google Scholar | PubMed |
Alvarez J, Smyth DR (2002) CRABS CLAW and SPATULA genes regulate growth and pattern formation during gynoecium development in Arabidopsis thaliana. International Journal of Plant Sciences 163(1), 17-41.
| Crossref | Google Scholar |
Alvarez JP, Goldshmidt A, Efroni I, Bowman JL, Eshed Y (2009) The NGATHA distal organ development genes are essential for style specification in Arabidopsis. The Plant Cell 21(5), 1373-1393.
| Crossref | Google Scholar | PubMed |
Arunkumar R, Wang W, Wright SI, Barrett SCH (2017) The genetic architecture of tristyly and its breakdown to self-fertilization. Molecular Ecology 26(3), 752-765.
| Crossref | Google Scholar | PubMed |
Bai Q, Wang L, Huang S, Ali K, Li G, Ren H, Zheng B (2023) The receptor-like kinase EMS1 and BRI1 coordinately regulate stamen elongation via the transcription factors BES1/BZR1 in Arabidopsis. Plant Science 331, 111673.
| Crossref | Google Scholar | PubMed |
Barrett SCH (2002) Sexual interference of the floral kind. Heredity 88(2), 154-159.
| Crossref | Google Scholar | PubMed |
Barrett SCH, Harder LD (2005) The evolution of polymorphic sexual systems in daffodils (Narcissus). New Phytologist 165(1), 45-53.
| Crossref | Google Scholar | PubMed |
Barrett SC, Jesson LK, Baker AM (2000) The evolution and function of stylar polymorphisms in flowering plants. Annals of Botany 85(suppl_1), 253-265.
| Crossref | Google Scholar |
Bernacchi D, Tanksley SD (1997) An interspecific backcross of Lycopersicon esculentum x L. hirsutum: linkage analysis and a QTL study of sexual compatibility factors and floral traits. Genetics 147(2), 861-877.
| Crossref | Google Scholar | PubMed |
Blanca J, Cañizares J, Cordero L, Pascual L, Diez MJ, Nuez F (2012) Variation revealed by SNP genotyping and morphology provides insight into the origin of the tomato. PLoS ONE 7(10), e48198.
| Crossref | Google Scholar | PubMed |
Bowman JL, Smyth DR, Meyerowitz EM (1991) Genetic interactions among floral homeotic genes of Arabidopsis. Development 112(1), 1-20.
| Crossref | Google Scholar | PubMed |
Bowman JL, Baum SF, Eshed Y, Putterill J, Alvarez J (1999) Molecular genetics of gynoecium development in Arabidopsis. Current Topics in Developmental Biology 45, 155-205.
| Crossref | Google Scholar | PubMed |
Brunet J, Eckert CG (1998) Effects of floral morphology and display on outcrossing in Blue Columbine, Aquilegia caerulea (Ranunculaceae). Functional Ecology 12, 596-606.
| Crossref | Google Scholar |
Brys R, Jacquemyn H (2012) Effects of human-mediated pollinator impoverishment on floral traits and mating patterns in a short-lived herb: an experimental approach. Functional Ecology 26(1), 189-197.
| Crossref | Google Scholar |
Cecchetti V, Altamura MM, Falasca G, Costantino P, Cardarelli M (2008) Auxin regulates Arabidopsis anther dehiscence, pollen maturation, and filament elongation. The Plant Cell 20(7), 1760-1774.
| Crossref | Google Scholar | PubMed |
Cecchetti V, Celebrin D, Napoli N, Ghelli R, Brunetti P, Costantino P, Cardarelli M (2017) An auxin maximum in the middle layer controls stamen development and pollen maturation in Arabidopsis. New Phytologist 213(3), 1194-1207.
| Crossref | Google Scholar | PubMed |
Chae K, Isaacs CG, Reeves PH, Maloney GS, Muday GK, Nagpal P, Reed JW (2012) Arabidopsis SMALL AUXIN UP RNA63 promotes hypocotyl and stamen filament elongation. The Plant Journal 71(4), 684-697.
| Crossref | Google Scholar | PubMed |
Chen K-Y, Cong B, Wing R, Vrebalov J, Tanksley SD (2007) Changes in regulation of a transcription factor lead to autogamy in cultivated tomatoes. Science 318(5850), 643-645.
| Crossref | Google Scholar | PubMed |
Chen W, Lv M, Wang Y, Wang P-A, Cui Y, Li M, Wang R, Gou X, Li J (2019) BES1 is activated by EMS1-TPD1-SERK1/2-mediated signaling to control tapetum development in Arabidopsis thaliana. Nature Communications 10(1), 4164.
| Crossref | Google Scholar | PubMed |
Cheng H, Qin L, Lee S, Fu X, Richards DE, Cao D, Luo D, Harberd NP, Peng J (2004) Gibberellin regulates Arabidopsis floral development via suppression of DELLA protein function. Development 131(5), 1055-1064.
| Crossref | Google Scholar | PubMed |
Cheng H, Song S, Xiao L, Soo HM, Cheng Z, Xie D, Peng J (2009) Gibberellin acts through jasmonate to control the expression of MYB21, MYB24, and MYB57 to promote stamen filament growth in Arabidopsis. PLoS Genetics 5(3), e1000440.
| Crossref | Google Scholar | PubMed |
Cheng M-Z, Gong C, Zhang B, Qu W, Qi H-N, Chen X-L, Wang X-Y, Zhang Y, Liu J-Y, Ding X-D, Qiu Y-W, Wang A-X (2021) Morphological and anatomical characteristics of exserted stigma sterility and the location and function of SlLst (Solanum lycopersicum Long styles) gene in tomato. Theoretical and Applied Genetics 134(2), 505-518.
| Crossref | Google Scholar | PubMed |
Cho JLY, Ding P (2021) Floral morphology and pollination process of red-fleshed dragon fruit (Hylocereus polyrhizus) grown in an open field. Horticultural Science and Technology 39(3), 277-293.
| Crossref | Google Scholar |
Colombo M, Brambilla V, Marcheselli R, Caporali E, Kater MM, Colombo L (2010) A new role for the SHATTERPROOF genes during Arabidopsis gynoecium development. Developmental Biology 337(2), 294-302.
| Crossref | Google Scholar | PubMed |
Conner JK, Sterling A (1996) Selection for independence of floral and vegetative traits: evidence from correlation patterns in five species. Canadian Journal of Botany 74(4), 642-644.
| Crossref | Google Scholar |
Dart SR, Samis KE, Austen E, Eckert CG (2012) Broad geographic covariation between floral traits and the mating system in Camissoniopsis cheiranthifolia (Onagraceae): multiple stable mixed mating systems across the species’ range? Annals of Botany 109(3), 599-611.
| Crossref | Google Scholar | PubMed |
Eckert CG, Ozimec B, Herlihy CR, Griffin CA, Routley MB (2009) Floral morphology mediates temporal variation in the mating system of a self-compatible plant. Ecology 90(6), 1540-1548.
| Crossref | Google Scholar | PubMed |
Eklund DM, Thelander M, Landberg K, Ståldal V, Nilsson A, Johansson M, Valsecchi I, Pederson ERA, Kowalczyk M, Ljung K, Ronne H, Sundberg E (2010) Homologues of the Arabidopsis thaliana SHI/STY/LRP1 genes control auxin biosynthesis and affect growth and development in the moss Physcomitrella patens. Development 137(8), 1275-1284.
| Crossref | Google Scholar | PubMed |
Fetscher AE (2001) Resolution of male-female conflict in an hermaphroditic flower. Proceedings of the Royal Society of London. Series B: Biological Sciences 268(1466), 525-529.
| Crossref | Google Scholar |
Fontaine C, Dajoz I, Meriguet J, Loreau M (2006) Functional diversity of plant–pollinator interaction webs enhances the persistence of plant communities. PLoS Biology 4(1), e1.
| Crossref | Google Scholar | PubMed |
Fourquin C, Ferrándiz C (2014) The essential role of NGATHA genes in style and stigma specification is widely conserved across eudicots. New Phytologist 202(3), 1001-1013.
| Crossref | Google Scholar | PubMed |
Galen C, Gregory T (1989) Interspecific pollen transfer as a mechanism of competition: consequences of foreign pollen contamination for seed set in the alpine wildflower, Polemonium viscosum. Oecologia 81, 120-123.
| Crossref | Google Scholar | PubMed |
Gastaldi V, Lucero LE, Ferrero LV, Ariel FD, Gonzalez DH (2020) Class-I TCP transcription factors activate the SAUR63 gene subfamily in gibberellin-dependent stamen filament elongation. Plant Physiology 182(4), 2096-2110.
| Crossref | Google Scholar | PubMed |
Gastaldi V, Alem AL, Mansilla N, Ariel FD, Viola IL, Lucero LE, Gonzalez DH (2023) BREVIPEDICELLUS/KNAT1 targets TCP15 to modulate filament elongation during Arabidopsis late stamen development. Plant Physiology 191(1), 29-34.
| Crossref | Google Scholar | PubMed |
Georgiady MS, Lord EM (2002) Evolution of the inbred flower form in the currant tomato, Lycopersicon pimpinellifolium. International Journal of Plant Sciences 163(4), 531-541.
| Crossref | Google Scholar |
Georgiady MS, Whitkus RW, Lord EM (2002) Genetic analysis of traits distinguishing outcrossing and self-pollinating forms of currant tomato, Lycopersicon pimpinellifolium (Jusl.) Mill. Genetics 161(1), 333-344.
| Crossref | Google Scholar | PubMed |
Ghelli R, Brunetti P, Marzi D, Cecchetti V, Costantini M, Lanzoni-Rossi M, Scaglia Linhares F, Costantino P, Cardarelli M (2023) The full-length Auxin Response Factor 8 isoform ARF8.1 controls pollen cell wall formation and directly regulates TDF1, AMS and MS188 expression. The Plant Journal 113(4), 851-865.
| Crossref | Google Scholar | PubMed |
Gorguet B, Eggink PM, Ocaña J, Tiwari A, Schipper D, Finkers R, Visser RGF, van Heusden AW (2008) Mapping and characterization of novel parthenocarpy QTLs in tomato. Theoretical and Applied Genetics 116, 755-767.
| Crossref | Google Scholar | PubMed |
Groszmann M, Paicu T, Smyth DR (2008) Functional domains of SPATULA, a bHLH transcription factor involved in carpel and fruit development in Arabidopsis. The Plant Journal 55(1), 40-52.
| Crossref | Google Scholar | PubMed |
Heisler MG, Ohno C, Das P, Sieber P, Reddy GV, Long JA, Meyerowitz EM (2005) Patterns of auxin transport and gene expression during primordium development revealed by live imaging of the Arabidopsis inflorescence meristem. Current Biology 15(21), 1899-1911.
| Crossref | Google Scholar | PubMed |
Henning PM, Shore JS, McCubbin AG (2020) Transcriptome and network analyses of heterostyly in Turnera subulata provide mechanistic insights: are S-loci a red-light for pistil elongation? Plants 9(6), 713.
| Crossref | Google Scholar | PubMed |
Herlihy CR, Eckert CG (2007) Evolutionary analysis of a key floral trait in Aquilegia canadensis (Ranunculaceae): genetic variation in herkogamy and its effect on the mating system. Evolution 61(7), 1661-1674.
| Crossref | Google Scholar | PubMed |
Herrera-Ubaldo H, Campos SE, Luna-García V, Zúñiga-Mayo VM, Armas-Caballero G, DeLuna A, Marsch-Martínez N, de Folter S (2018) An interaction map of transcription factors controlling gynoecium development in Arabidopsis. BioRxiv 500736.
| Crossref | Google Scholar |
Herrera-Ubaldo H, Campos SE, López-Gómez P, Luna-García V, Zúñiga-Mayo VM, Armas-Caballero GE, González-Aguilera KL, DeLuna A, Marsch-Martínez N, Espinosa-Soto C, de Folter S (2023) The protein–protein interaction landscape of transcription factors during gynoecium development in Arabidopsis. Molecular Plant 16, 260-278.
| Crossref | Google Scholar | PubMed |
Herrera CM (2001) Deconstructing a floral phenotype: do pollinators select for corolla integration in Lavandula latifolia? Journal of Evolutionary Biology 14(4), 574-584.
| Crossref | Google Scholar |
Huang H, Gong Y, Liu B, Wu D, Zhang M, Xie D, Song S (2020) The DELLA proteins interact with MYB21 and MYB24 to regulate filament elongation in Arabidopsis. BMC Plant Biology 20(1), 64.
| Crossref | Google Scholar | PubMed |
Huu CN, Kappel C, Keller B, Sicard A, Takebayashi Y, Breuninger H, Nowak MD, Bäurle I, Himmelbach A, Burkart M, Ebbing-Lohaus T, Sakakibara H, Altschmied L, Conti E, Lenhard M (2016) Presence versus absence of CYP734A50 underlies the style-length dimorphism in primroses. eLife 5, e17956.
| Crossref | Google Scholar | PubMed |
Huu CN, Plaschil S, Himmelbach A, Kappel C, Lenhard M (2022) Female self-incompatibility type in heterostylous Primula is determined by the brassinosteroid-inactivating cytochrome P450 CYP734A50. Current Biology 32(3), 671-676.e5.
| Crossref | Google Scholar | PubMed |
Indriolo E, Safavian D, Goring DR (2014) The ARC1 E3 ligase promotes two different self-pollen avoidance traits in Arabidopsis. The Plant Cell 26(4), 1525-1543.
| Crossref | Google Scholar | PubMed |
Ishiguro S, Kawai-Oda A, Ueda J, Nishida I, Okada K (2001) The DEFECTIVE IN ANTHER DEHISCENCE1 gene encodes a novel phospholipase A1 catalyzing the initial step of jasmonic acid biosynthesis, which synchronizes pollen maturation, anther dehiscence, and flower opening in Arabidopsis. The Plant Cell 13(10), 2191-2209.
| Crossref | Google Scholar | PubMed |
Ito T, Wellmer F, Yu H, Das P, Ito N, Alves-Ferreira M, Riechmann JL, Meyerowitz EM (2004) The homeotic protein AGAMOUS controls microsporogenesis by regulation of SPOROCYTELESS. Nature 430(6997), 356-360.
| Crossref | Google Scholar | PubMed |
Ito T, Ng K-H, Lim T-S, Yu H, Meyerowitz EM (2007) The homeotic protein AGAMOUS controls late stamen development by regulating a jasmonate biosynthetic gene in Arabidopsis. The Plant Cell 19(11), 3516-3529.
| Crossref | Google Scholar | PubMed |
Jiménez-López FJ, Arista M, Talavera M, Pannell JR, Ortiz PL (2019) Heritabilities of lateral and vertical herkogamy in Lysimachia arvensis. Plant Species Biology 34(1), 31-37.
| Crossref | Google Scholar |
Kappel C, Huu CN, Lenhard M (2017) A short story gets longer: recent insights into the molecular basis of heterostyly. Journal of Experimental Botany 68(21-22), 5719-5730.
| Crossref | Google Scholar | PubMed |
Karron JD, Jackson RT, Thumser NN, Schlicht SL (1997) Outcrossing rates of individual Mimulus ringens genets are correlated with anther-stigma separation. Heredity 79(4), 365-370.
| Crossref | Google Scholar |
Kulbaba MW, Worley AC (2008) Floral design in Polemonium brandegei (Polemoniaceae): genetic and phenotypic variation under hawkmoth and hummingbird pollination. International Journal of Plant Sciences 169(4), 509-522.
| Crossref | Google Scholar |
Kuusk S, Sohlberg JJ, Magnus Eklund D, Sundberg E (2006) Functionally redundant SHI family genes regulate Arabidopsis gynoecium development in a dose-dependent manner. The Plant Journal 47(1), 99-111.
| Crossref | Google Scholar | PubMed |
Lande R, Arnold SJ (1983) The measurement of selection on correlated characters. Evolution 37, 1210-1226.
| Crossref | Google Scholar | PubMed |
Li Q-F, Wang C, Jiang L, Li S, Sun SSM, He J-X (2012) An interaction between BZR1 and DELLAs mediates direct signaling crosstalk between brassinosteroids and gibberellins in Arabidopsis. Science Signaling 5(244), ra72.
| Crossref | Google Scholar |
Li Q-F, Lu J, Yu J-W, Zhang C-Q, He J-X, Liu Q-Q (2018) The brassinosteroid-regulated transcription factors BZR1/BES1 function as a coordinator in multisignal-regulated plant growth. Biochimica et Biophysica Acta (BBA) – Gene Regulatory Mechanisms 1861(6), 561-571.
| Crossref | Google Scholar | PubMed |
Li W, Huang X, Zou J, Wu J, Jiao H, Peng X, Sun M-X (2020) Three STIGMA AND STYLE STYLISTs pattern the fine architectures of apical gynoecium and are critical for male gametophyte-pistil interaction. Current Biology 30(23), 4780-4788.e5.
| Crossref | Google Scholar | PubMed |
Liu Z, Franks RG, Klink VP (2000) Regulation of gynoecium marginal tissue formation by LEUNIG and AINTEGUMENTA. The Plant Cell 12(10), 1879-1891.
| Crossref | Google Scholar | PubMed |
Liu N, Wu S, Van Houten J, Wang Y, Ding B, Fei Z, Clarke TH, Reed JW, Van Der Knaap E (2014) Down-regulation of AUXIN RESPONSE FACTORS 6 and 8 by microRNA 167 leads to floral development defects and female sterility in tomato. Journal of Experimental Botany 65(9), 2507-2520.
| Crossref | Google Scholar | PubMed |
Liu S-J, Wu L-Y, Huang S-Q (2016) Shortened anther–stigma distance reduces compatible pollination in two distylous Primula species. Journal of Plant Ecology 9(2), 224-232.
| Crossref | Google Scholar |
Luo Y, Widmer A (2013) Herkogamy and its effects on mating patterns in Arabidopsis thaliana. PLoS ONE 8(2), e57902.
| Crossref | Google Scholar | PubMed |
Ma H (2005) Molecular genetic analyses of microsporogenesis and microgametogenesis in flowering plants. Annual Review of Plant Biology 56, 393-434.
| Crossref | Google Scholar | PubMed |
Mandaokar A, Thines B, Shin B, Markus Lange B, Choi G, Koo YJ, Yoo YJ, Choi YD, Choi G, Browse J (2006) Transcriptional regulators of stamen development in Arabidopsis identified by transcriptional profiling. The Plant Journal 46(6), 984-1008.
| Crossref | Google Scholar | PubMed |
Marsch-Martínez N, de Folter S (2016) Hormonal control of the development of the gynoecium. Current Opinion in Plant Biology 29, 104-114.
| Crossref | Google Scholar | PubMed |
Marsch-Martínez N, Ramos-Cruz D, Irepan Reyes-Olalde J, Lozano-Sotomayor P, Zúñiga-Mayo VM, de Folter S (2012) The role of cytokinin during Arabidopsis gynoecia and fruit morphogenesis and patterning. The Plant Journal 72(2), 222-234.
| Crossref | Google Scholar | PubMed |
Matzke CM, Hamam HJ, Henning PM, Dougherty K, Shore JS, Neff MM, McCubbin AG (2021) Pistil mating type and morphology are mediated by the brassinosteroid inactivating activity of the S-locus gene BAHD in heterostylous Turnera species. International Journal of Molecular Sciences 22(19), 10603.
| Crossref | Google Scholar | PubMed |
Moeller DA (2006) Geographic structure of pollinator communities, reproductive assurance, and the evolution of self-pollination. Ecology 87(6), 1510-1522.
| Crossref | Google Scholar | PubMed |
Motten AF, Stone JL (2000) Heritability of stigma position and the effect of stigma-anther separation on outcrossing in a predominantly self-fertilizing weed, Datura stramonium (Solanaceae). American Journal of Botany 87(3), 339-347.
| Crossref | Google Scholar | PubMed |
Nagpal P, Ellis CM, Weber H, Ploense SE, Barkawi LS, Guilfoyle TJ, Hagen G, Alonso JM, Cohen JD, Farmer EE, Ecker JR, Reed JW (2005) Auxin response factors ARF6 and ARF8 promote jasmonic acid production and flower maturation. Development 132(18), 4107-4118.
| Crossref | Google Scholar | PubMed |
Neff MM, Nguyen SM, Malancharuvil EJ, Fujioka S, Noguchi T, Seto H, Tsubuki M, Honda T, Takatsuto S, Yoshida S, Chory J (1999) BAS1: a gene regulating brassinosteroid levels and light responsiveness in Arabidopsis. Proceedings of the National Academy of Sciences 96(26), 15316-15323.
| Crossref | Google Scholar |
Nowak MD, Russo G, Schlapbach R, Huu CN, Lenhard M, Conti E (2015) The draft genome of Primula veris yields insights into the molecular basis of heterostyly. Genome Biology 16(1), 12.
| Crossref | Google Scholar |
Ollerton J (2017) Pollinator diversity: distribution, ecological function, and conservation. Annual Review of Ecology, Evolution, and Systematics 48, 353-376.
| Crossref | Google Scholar |
Opedal ØH (2018) Herkogamy, a principal functional trait of plant reproductive biology. International Journal of Plant Sciences 179(9), 677-687.
| Crossref | Google Scholar |
Opedal ØH, Albertsen E, Armbruster WS, Pérez-Barrales R, Falahati-Anbaran M, Pélabon C (2016) Evolutionary consequences of ecological factors: pollinator reliability predicts mating-system traits of a perennial plant. Ecology Letters 19(12), 1486-1495.
| Crossref | Google Scholar | PubMed |
Opedal ØH, Bolstad GH, Hansen TF, Armbruster WS, Pélabon C (2017) The evolvability of herkogamy: quantifying the evolutionary potential of a composite trait. Evolution 71(6), 1572-1586.
| Crossref | Google Scholar | PubMed |
Opedal ØH, Hildesheim LS, Armbruster WS (2022) Evolvability and constraint in the evolution of three-dimensional flower morphology. American Journal of Botany 109(11), 1906-1917.
| Crossref | Google Scholar | PubMed |
Park J-H, Halitschke R, Kim HB, Baldwin IT, Feldmann KA, Feyereisen R (2002) A knock-out mutation in allene oxide synthase results in male sterility and defective wound signal transduction in Arabidopsis due to a block in jasmonic acid biosynthesis. The Plant Journal 31(1), 1-12.
| Crossref | Google Scholar | PubMed |
Peralta IE, Knapp S, Spooner DM (2005) New species of wild tomatoes (Solanum section Lycopersicon: Solanaceae) from Northern Peru. Systematic Botany 30(2), 424-434.
| Crossref | Google Scholar |
Plackett ARG, Thomas SG, Wilson ZA, Hedden P (2011) Gibberellin control of stamen development: a fertile field. Trends in Plant Science 16(10), 568-578.
| Crossref | Google Scholar | PubMed |
Qi T, Huang H, Song S, Xie D (2015) Regulation of jasmonate-mediated stamen development and seed production by a bHLH-MYB complex in Arabidopsis. The Plant Cell 27(6), 1620-1633.
| Crossref | Google Scholar | PubMed |
Reeves PH, Ellis CM, Ploense SE, Wu M-F, Yadav V, Tholl D, Chételat A, Haupt I, Kennerley BJ, Hodgens C, Farmer EE, Nagpal P, Reed JW (2012) A regulatory network for coordinated flower maturation. PLoS Genetics 8(2), e1002506.
| Crossref | Google Scholar | PubMed |
Riccini A, Picarella ME, De Angelis F, Mazzucato A (2021) Bulk RNA-Seq analysis to dissect the regulation of stigma position in tomato. Plant Molecular Biology 105, 263-285.
| Crossref | Google Scholar | PubMed |
Rick CM, Dempsey WH (1969) Position of the stigma in relation to fruit setting of the tomato. Botanical Gazette 130(3), 180-186.
| Crossref | Google Scholar |
Rick CM, Lamm R (1955) Biosystematic studies on the status of Lycopersicon chilense. American Journal of Botany 663-675.
| Crossref | Google Scholar |
Rick CM, Fobes JF, Holle M (1977) Genetic variation in Lycopersicon pimpinellifolium: evidence of evolutionary change in mating systems. Plant Systematics and Evolution 127, 139-170.
| Crossref | Google Scholar |
Rick CM, Holle M, Thorp RW (1978) Rates of cross-pollination in Lycopersicon pimpinellifolium: impact of genetic variation in floral characters. Plant Systematics and Evolution 129, 31-44.
| Crossref | Google Scholar |
Rieu I, Eriksson S, Powers SJ, Gong F, Griffiths J, Woolley L, Benlloch R, Nilsson O, Thomas SG, Hedden P, Phillips AL (2008a) Genetic analysis reveals that C19-GA 2-oxidation is a major gibberellin inactivation pathway in Arabidopsis. The Plant Cell 20(9), 2420-2436.
| Crossref | Google Scholar | PubMed |
Rieu I, Ruiz-Rivero O, Fernandez-Garcia N, Griffiths J, Powers SJ, Gong F, Linhartova T, Eriksson S, Nilsson O, Thomas SG, Phillips AL, Hedden P (2008b) The gibberellin biosynthetic genes AtGA20ox1 and AtGA20ox2 act, partially redundantly, to promote growth and development throughout the Arabidopsis life cycle. The Plant Journal 53(3), 488-504.
| Crossref | Google Scholar | PubMed |
Rubio-Somoza I, Weigel D (2013) Coordination of flower maturation by a regulatory circuit of three microRNAs. PLoS Genetics 9(3), e1003374.
| Crossref | Google Scholar | PubMed |
Ruiz-Martín J, Santos-Gally R, Escudero M, Midgley JJ, Pérez-Barrales R, Arroyo J (2018) Style polymorphism in Linum (Linaceae): a case of Mediterranean parallel evolution? Plant Biology 20, 100-111.
| Crossref | Google Scholar | PubMed |
Saeed A, Hayat K, Khan A, Iqbal S (2007) Heat tolerance studies in tomato (Lycopersicon esculentum Mill.). International Journal of Agriculture & Biology 9(4), 649-652.
| Google Scholar |
Sakata T, Oshino T, Miura S, Tomabechi M, Tsunaga Y, Higashitani N, Miyazawa Y, Takahashi H, Watanabe M, Higashitani A (2010) Auxins reverse plant male sterility caused by high temperatures. Proceedings of the National Academy of Sciences 107(19), 8569-8574.
| Crossref | Google Scholar |
Sauquet H, Von Balthazar M, Magallón S, Doyle JA, Endress PK, Bailes EJ, Barroso de Morais E, Bull-Hereñu K, Carrive L, Chartier M, et al. (2017) The ancestral flower of angiosperms and its early diversification. Nature Communications 8(1), 16047.
| Crossref | Google Scholar |
Shang L, Song J, Yu H, Wang X, Yu C, Wang Y, Li F, Lu Y, Wang T, Ouyang B, Zhang J, Larkin RM, Ye Z, Zhang Y (2021) A mutation in a C2H2-type zinc finger transcription factor contributed to the transition toward self-pollination in cultivated tomato. The Plant Cell 33(10), 3293-3308.
| Crossref | Google Scholar | PubMed |
Shore JS, Hamam HJ, Chafe PDJ, Labonne JDJ, Henning PM, McCubbin AG (2019) The long and short of the S-locus in Turnera (Passifloraceae). New Phytologist 224(3), 1316-1329.
| Crossref | Google Scholar | PubMed |
Sieburth LE, Meyerowitz EM (1997) Molecular dissection of the AGAMOUS control region shows that cis elements for spatial regulation are located intragenically. The Plant Cell 9(3), 355-365.
| Crossref | Google Scholar | PubMed |
Sohlberg JJ, Myrenås M, Kuusk S, Lagercrantz U, Kowalczyk M, Sandberg G, Sundberg E (2006) STY1 regulates auxin homeostasis and affects apical–basal patterning of the Arabidopsis gynoecium. The Plant Journal 47(1), 112-123.
| Crossref | Google Scholar | PubMed |
Song S, Qi T, Huang H, Ren Q, Wu D, Chang C, Peng W, Liu Y, Peng J, Xie D (2011) The jasmonate-ZIM domain proteins interact with the R2R3-MYB transcription factors MYB21 and MYB24 to affect jasmonate-regulated stamen development in Arabidopsis. The Plant Cell 23(3), 1000-1013.
| Crossref | Google Scholar | PubMed |
Song S, Qi T, Huang H, Xie D (2013) Regulation of stamen development by coordinated actions of jasmonate, auxin, and gibberellin in Arabidopsis. Molecular Plant 6(4), 1065-1073.
| Crossref | Google Scholar | PubMed |
Spartz AK, Ren H, Park MY, Grandt KN, Lee SH, Murphy AS, Sussman MR, Overvoorde PJ, Gray WM (2014) SAUR inhibition of PP2C-D phosphatases activates plasma membrane H+-ATPases to promote cell expansion in Arabidopsis. The Plant Cell 26(5), 2129-2142.
| Crossref | Google Scholar | PubMed |
Stintzi A, Browse J (2000) The Arabidopsis male-sterile mutant, opr3, lacks the 12-oxophytodienoic acid reductase required for jasmonate synthesis. Proceedings of the National Academy of Sciences 97(19), 10625-10630.
| Crossref | Google Scholar |
Ståldal V, Sundberg E (2009) The role of auxin in style development and apical-basal patterning of the Arabidopsis thaliana gynoecium. Plant Signaling & Behavior 4(2), 83-85.
| Crossref | Google Scholar | PubMed |
Ståldal V, Sohlberg JJ, Eklund DM, Ljung K, Sundberg E (2008) Auxin can act independently of CRC, LUG, SEU, SPT and STY1 in style development but not apical-basal patterning of the Arabidopsis gynoecium. New Phytologist 180(4), 798-808.
| Crossref | Google Scholar | PubMed |
Tabata R, Ikezaki M, Fujibe T, Aida M, Tian C-E, Ueno Y, Yamamoto KT, Machida Y, Nakamura K, Ishiguro S (2010) Arabidopsis AUXIN RESPONSE FACTOR6 and 8 regulate jasmonic acid biosynthesis and floral organ development via repression of class 1 KNOX genes. Plant and Cell Physiology 51(1), 164-175.
| Crossref | Google Scholar | PubMed |
Takano-Kai N, Doi K, Yoshimura A (2011) GS3 participates in stigma exsertion as well as seed length in rice. Breeding Science 61(3), 244-250.
| Crossref | Google Scholar |
Takebayashi N, Wolf DE, Delph LF (2006) Effect of variation in herkogamy on outcrossing within a population of Gilia achilleifolia. Heredity 96(2), 159-165.
| Crossref | Google Scholar | PubMed |
Tashiro S, Tian C-e, Watahiki MK, Yamamoto KT (2009) Changes in growth kinetics of stamen filaments cause inefficient pollination in massugu2, an auxin insensitive, dominant mutant of Arabidopsis thaliana. Physiologia Plantarum 137(2), 175-187.
| Crossref | Google Scholar | PubMed |
Thomson B, Wellmer F (2019) Molecular regulation of flower development. In ‘Current topics in developmental biology, Vol. 131’. (Ed. U Grossniklaus) pp. 185–210. (Academic Press) 10.1016/bs.ctdb.2018.11.007
Toräng P, Vikström L, Wunder J, Wötzel S, Coupland G, Ågren J (2017) Evolution of the selfing syndrome: anther orientation and herkogamy together determine reproductive assurance in a self-compatible plant. Evolution 71(9), 2206-2218.
| Crossref | Google Scholar | PubMed |
Trigueros M, Navarrete-Gómez M, Sato S, Christensen SK, Pelaz S, Weigel D, Yanofsky MF, Ferrándiz C (2009) The NGATHA genes direct style development in the Arabidopsis gynoecium. The Plant Cell 21(5), 1394-1409.
| Crossref | Google Scholar | PubMed |
Tyler L, Thomas SG, Hu J, Dill A, Alonso JM, Ecker JR, Sun T-P (2004) DELLA proteins and gibberellin-regulated seed germination and floral development in Arabidopsis. Plant Physiology 135(2), 1008-1019.
| Crossref | Google Scholar | PubMed |
Ushijima K, Nakano R, Bando M, Shigezane Y, Ikeda K, Namba Y, Kume S, Kitabata T, Mori H, Kubo Y (2012) Isolation of the floral morph-related genes in heterostylous flax (Linum grandiflorum): the genetic polymorphism and the transcriptional and post-transcriptional regulations of the S locus. The Plant Journal 69(2), 317-331.
| Crossref | Google Scholar | PubMed |
Van Etten ML, Brunet J (2013) The impact of global warming on floral traits that affect the selfing rate in a high-altitude plant. International Journal of Plant Sciences 174(8), 1099-1108.
| Crossref | Google Scholar |
Vosters SL, Jewell CP, Sherman NA, Einterz F, Blackman BK, Moyle LC (2014) The timing of molecular and morphological changes underlying reproductive transitions in wild tomatoes (Solanum sect. Lycopersicon). Molecular Ecology 23(8), 1965-1978.
| Crossref | Google Scholar | PubMed |
Waser NM, Price MV (1991) Reproductive costs of self-pollination in Ipomopsis aggregata (Polemoniaceae): are ovules usurped? American Journal of Botany 78(8), 1036-1043.
| Crossref | Google Scholar |
Webb CJ, Lloyd DG (1986) The avoidance of interference between the presentation of pollen and stigmas in angiosperms II. Herkogamy. New Zealand Journal of Botany 24(1), 163-178.
| Crossref | Google Scholar |
Weinig C (2002) Phytochrome photoreceptors mediate plasticity to light quality in flowers of the Brassicaceae. American Journal of Botany 89(2), 230-235.
| Crossref | Google Scholar | PubMed |
Wu M-F, Tian Q, Reed JW (2006) Arabidopsis microRNA167 controls patterns of ARF6 and ARF8 expression, and regulates both female and male reproduction. Development 133(21), 4211-4218.
| Crossref | Google Scholar | PubMed |
Wu M, Bian X, Huang B, Du Y, Hu S, Wang Y, Shen J, Wu S (2024) HD-Zip proteins modify floral structures for self-pollination in tomato. Science 384(6691), 124-130.
| Crossref | Google Scholar |
Xie D-X, Feys BF, James S, Nieto-Rostro M, Turner JG (1998) COI1: an Arabidopsis gene required for jasmonate-regulated defense and fertility. Science 280(5366), 1091-1094.
| Crossref | Google Scholar | PubMed |
Zhang Y, Chen Y, Zhou Y, Zhang J, Bai H, Zheng C (2020) Comparative transcriptome reveals the genes’ adaption to herkogamy of Lumnitzera littorea (Jack) Voigt. Frontiers in Genetics 11, 584817.
| Crossref | Google Scholar |