Enhancing the productivity and resilience of rice (Oryza sativa) under environmental stress conditions using clustered regularly interspaced short palindromic repeats (CRISPR) technology
Aamir Riaz

A
B
C
D
# These authors contributed equally to this work
Handling Editor: Muhammad Nadeem
Abstract
Rice (Oryza sativa) is a crucial staple crop worldwide, providing nutrition to more than half of the global population. Nonetheless, the sustainability of grain production is increasingly jeopardized by both biotic and abiotic stressors exacerbated by climate change, which increases the crop’s rvulnerability to pests and diseases. Genome-editing by clustered regularly interspaced short palindromic repeats and CRISPR-associated Protein 9 (CRISPR-Cas9) presents a potential solution for enhancing rice productivity and resilience under climatic stress. This technology can alter a plant’s genetic components without the introduction of foreign DNA or genes. It has become one of the most extensively used approaches for discovering new gene functions and creating novel varieties that exhibit a higher tolerance to both abiotic and biotic stresses, herbicide resistance, and improved yield production. This study examines numerous CRISPR-Cas9-based genome-editing techniques for gene knockout, gene knock-in, multiplexing for simultaneous disruption of multiple genes, base-editing, and prime-editing. This review elucidates the application of genome-editing technologies to enhance rice production by directly targeting yield-related genes or indirectly modulating numerous abiotic and biotic stress-responsive genes. We highlight the need to integrate genetic advancements with conventional and advanced agricultural methods to create rice varieties that are resilient to stresses, thereby safeguarding food security and promoting agricultural sustainability amid climatic concerns.
Keywords: biotic, drought, genome editing, heat, heavy metals, mutation, rice, stresses, yield.
Introduction
Cereals constitute an essential part of the diets of the world’s growing population. Rice (Oryza sativa), maize (Zea mays), and wheat (Triticum aestivum) account for roughly half of the global calorie consumption for humans (Falcon et al. 2022). Rice is the most significant food crop for Asian countries, particularly in south-east Asia where it is cultivated on millions of hectares of land (Giller et al. 2021; Husain and Sharma 2022). It is the world’s second-most planted crop (Rahaman and Shehab 2019; Singh et al. 2021). Rice was cultivated 10,000 years ago in the river basins of south and south-east Asia and China (Zeng 2020), and is today, it is also grown Latin America, Europe, certain parts of Africa, and the United States (Samal et al. 2021).
There are various obstacles that need to be overcome in order to continue increasing rice production to meet the food needs of the world’s expanding population (Boyd et al. 2020; Islam et al. 2024). The migration of workers from rural areas to urban areas is limiting the labor supply available to work on farms, which places rice production at risk due to the crop’s high eliance on water and labor. The widespread use of high-yielding rice varieties and hybrids, combined with excessive fertilizer application, particularly nitrogen, has been the primary driving force behind the notable increase in rice production over the past 40 years (Yu et al. 2020; Tseng et al. 2021). Overuse of nitrogen fertilizer has resulted in environmental issues such as eutrophication of surface and groundwater, ozone layer depletion, and greenhouse warming. These issues have sparked widespread concern around the world (Chandini et al. 2019; Ashitha et al. 2021).
Overcoming diseases caused by bacteria, fungi, and viruses is another obstacle that is critical to maximizing yield potential (Pérez-Méndez et al. 2022; Raza et al. 2022; Chaitanya et al. 2023). The dynamics of rice diseases have changed through time as a consequence of changes in farming techniques, a reduction in varietal range that has led to a restricted genetic basis, and changes in climate (Heredia et al. 2022; Khoury et al. 2022). Certain diseases have become more prevalent and threatening, having spread to more locations. A great number of diseases that were previously regarded as relatively unimportant have recently emerged as major economic threats in certain areas. Because new and more dangerous viral strains have emerged, managing diseases has become much more difficult due to a faster breakdown of disease resistance. Therefore, new technologies are needed to keep pace with these changes. This review discusses the role of clustered regularly interspaced short palindromic repeats and CRISPR-associated Protein 9 (CRISPR/Cas9) system, a genome-editing tool, to develop rice varieties that are more resistant to range of abiotic and biotic stresses caused a changing climate (Fig. 1).
Responses to abiotic stresses in rice
Abiotic stressors that adversely agricultural production are often inter-related with one another, and often manifest in plants as osmotic stress, a failure in ion transportation, or a disruption in the metabolism of plant cells (Madani et al. 2019). These responses are induced by a collection of genes that alter how they are expressed, which has an impact on the rate of growth and ultimately, profitability. Therefore, the identification of genes that are sensitive to abiotic challenges is required to comprehend the processes that agricultural plants use to react when they are subjected to abiotic stresses (Bueno and Lopes 2020). Plants are are constantly adapting to improve their defense systems against various abiotic pressures .Here, we discuss some of these abiotic pressures.
Cold
Cold stress reduces the productivity of crops (Gull et al. 2019; Kul et al. 2019; Teklić et al. 2021) by affecting crop quality and fruit production, especially in temperate climates where chilling and freezing can detrimentally affect plant development and growth (Selvaraj et al. 2022). Plants can undergo acclimatization where they build up resistance to potentially damaging cold shocks to adapt to cold or freezing conditions (Sakina et al. 2019). Despite this, a significant number of key crops are not yet capable of acclimatization to cold conditions. The abiotic stress that is generated by low temperatures affects every element of the cellular functioning of plants. There are many different signal transduction pathways, such as elements of reactive oxygen species (ROS), protein phosphate, protein kinase, abscisic acid (ABA), and Ca2, which transduce the effects of cold stressors. Among these, ABA has been shown to be the most effective (Sakina et al. 2019).
Salt
Soil salinity is a worldwide threat to agriculture across the globe by lowering the agricultural production (Singh 2022). Salt stress has adverse effects on plant growth and production in two main ways: (1) osmotic load; and (2) oxidative stress, which is the imbalance in the production and neutralisation of reactive oxygen species (ROS) (Hussain et al. 2019; Adil et al. 2023). Due to the presence of excess salts, the osmotic pressure in the soil solution is higher than that in the plant cells during salt stress. As a result, the plant’s ability to absorb essential minerals and water, such as calcium and potassium is hampered, which induce downstream effect such as diminished cell function and growth, impaired cytosolic efficiency, and impaired assimilate synthesis (Singhal et al. 2021; El-Badri et al. 2022).
Drought
Average global temperatures and atmospheric carbon dioxide levels are both rising at a steady rate, which has resulted in widespread alterations to the planet’s climate (Isson et al. 2020). The shift in weather has resulted in an irregular rainfall distribution that contributes to drought in areas that once had regular precipitation (Wang et al. 2022a). Because of extreme drought conditions, the quantity of soil water that is available to plants has been reducing, which has led to the untimely mortality of plants (Frei et al. 2022). Plants stop growing in drought conditions as metabolic energy required for growth decreases (Frei et al. 2022). Under drought stress, plants produce protective chemicals by mobilizing compounds that are necessary for osmoregulation (Jacques et al. 2021).
Heat
A rise in temperature around the world has become a major cause for concern and affects plant development and yield (Hinojosa et al. 2019; Morales et al. 2020). When plants are subjected to heat stress, seed germination and photosynethetic effectiveness decrease. When a plant experiences heat shock during the productive growth stage, heat damages the tapetal cell’s functioning, and another cell becomes dysplastic (Younis et al. 2020).
Heavy metals
Increased use of synthetic fertilizers, irrigation with sewage wastewater, and rapid urbanization have resulted in toxic metals being introduced into agricultural soils, which has had a negative impact on the soil–plant environment (Gull et al. 2019; Lin et al. 2022). Heavy metals like chromium (Cr), cadmium (Cd), lead (Pb), mercury (Hg), arsenic (As), and thallium (Tl) are harmful living organisms, and the contamination of rice grains with Cd and As is a worldwide problem (Yu et al. 2016; Sharma et al. 2022). Toxic levels of zinc (Zn) and Cd uptake can impact on iron (Fe) absorption in moderate soil dryness and cause detrimental effects on the photosynthesis process and yield (Table 1) (Slamet-Loedin et al. 2015; Özyiğit et al. 2021). The accumulation of heavy metals in rice can result in biomagnification within the food chain, posing a potential hazard to human and animal health.
Heavy metal | Reported yield loss (%) | References | |
---|---|---|---|
Hg | 51–70 | Hillary and Ceasar (2022) | |
Cr | 30–47 | AbdElgawad et al. (2023) | |
Al | 25–60 | AbdElgawad et al. (2023) | |
Ni | 53–69 | Aziz et al. (2015) | |
Zn | 20–40 | Panhwar et al. (2015) | |
Cu | 10–90 | Xu et al. (2006) | |
Fe | 16–78 | Audebert and Fofana (2009) | |
Cd | 34–63 | Kanu et al. (2017) | |
As | 30–40 | Muehe et al. (2019) | |
Pb | 46–69 | Ashraf et al. (2017) |
Biotic stresses and crops
Plants are exposed to a diverse range of biotic stressors, such as fungal infections, viral diseases, bacterial infections, insects, and nematodes that cause infections, and crop damage, ultimately affecting crop yield (Panth et al. 2020). Weed imposes significant impact on plant growth through the competition of resources, sunlight, and space. There are several pathogenic plants that have the ability to enter the host plant and infect both the roots and the aboveground parts of the host. Cuscuta sp., Arceuthobium sp., Orobanche sp., and Phelipanche aegyptiaca are parasitic plants that infest host plants, significantly damaging the agro-economic production (Ghosh and Dey 2022). Bacterial blight, caused by Xanthomonas oryzae pv. oryzae (Xoo) (Oliva et al. 2019), is one of the most significant rice bacterial pathogens. Magnaporthe oryzae, the pathogen responsible for rice blast disease, has resulted in significant economic losses in rice production (Wei et al. 2024). Viruses are obligate parasites with a strong capacity to infect plants, resulting in a significant decrease in crop output (Das et al. 2019). The CRISPR-Cas technique provides plants with resistance against both DNA and RNA viruses by directly targeting and cleaving the viral genome (Mushtaq et al. 2020). The fungal infection can readily infiltrate the host plant due to its genetic adaptability. Fungi are responsible for causing various diseases, such as mildew, smut, and rot, which ultimately lead to significant reductions in crop yield (Dong and Ronald 2019).
Genome-editing technologies
Due to a new era of genome engineering and genome-editing technologies, plant genomes may now be altered rapidly, precisely, and efficiently. In the 1990s, mega nucleases (MNs) and zinc-finger nucleases (ZFNs) established the foundation for genome-editing and sparked the advancement of this science (Bibikova et al. 2002; Carroll 2021). MNs were a newly discovered type of nucleases that were commonly employed in the editing of plant genomes. MNs are differentiated from other endonucleases by their substantial recognition site, often consisting of 12–40 base pairs (bps). This characteristic makes MNs the most effective method for delivering all types of vectors, including plant RNA viruses (Mishra and Zhao 2018). The most frequent MNs are homing endonucleases. MNs pose challenges for modification when used alongside other genome-targeting methods due to the frequent fusion of their DNA-binding and catalytic domains, which cannot be easily separated (Zhang et al. 2010). Prior research in several crops shown the potential utility of modified MNs for genetic editing in plants. Nevertheless, due to the challenging nature of managing MNs, further investigation is required to enhance this approach. Consequently, scientists have focused their efforts on developing gene engineering techniques that are more efficient, uncomplicated, and precise. These include ZFNs, transcription activator-like effector nuclease (TALEN), and clustered regularly interspaced short palindromic repeats and CRISPR-associated Protein 9 (CRISPR/Cas9).
ZFNs revolutionized genome modification by being the pioneering protein reagents with the ability to precisely target specific locations. ZFNs act as DNA-binding domains that precisely identify and bind to a specific target spot by perfectly recognizing three base pairs (Rai et al. 2019). They are a highly effective and influential tools for genome-editing due to their ability to target double-strand breaks (DSBs) specifically (Durai et al. 2005). ZFNs consist of two essential domains: (1) the DNA-binding domain; and (2) the DNA cleavage domain. These domains are necessary for ZFNs to carry out its function (Papworth et al. 2006). According to (Carlson et al. 2012), the DNA-binding domain consists of 300–600 zinc-finger repeats, each that can recognise and interpret nine to 18 bps of DNA sequence. The DNA cleavage domain is an extensively acknowledged element of the type II restriction endonuclease Fok1, serving as a non-selective cleavage domain. According to (Carroll et al. 2006), this identical domain also functions as the DNA cleavage domain in ZFNs. ZFNs have been extensively created and tested across multiple species. In rice, low starch levels, dwarf morphologies, and no SSIVa mRNA expression were found in transgenic plants expressing premature stop codons and substitution events, which were created by ZFN-mediated targeted gene disruption in the SSIVa rice gene’s coding sequence (Jung et al. 2018). The occurrence of unintended consequences decreased as technology grew more precise and efficient.
Similar to ZFNs, TALENs are site-directed mutagenesis enzymes found in the rice plant pathogenic bacteria X. oryzae. In 2010, TALENs were created by fusing a transcription activator-like effector (TALE) with FokI’s catalytic domain. Genome-editing became accessible to scientists because they were easier to utilize (Christian et al. 2010). The DNA binding domain of TALENs consists of a single TALE protein that binds to a single base pair of DNA. Researchers were able to decode the recognition preferences of 400 distinct RVDs in 2014, which established TALENs as a significant tool for studying gene function and gene therapy (Yang et al. 2014). TALEs are distinguished by their 34-amino-acid repetitive sequences, which enable precise editing of a single base pair (Zhang et al. 2019a). Non-adjacent repetitive sequences can impact TALE proteins as well. TALE proteins continuously repair the nucleotides of the DNA sequence at the thymidine base located at the 5′ end. Without a 50 T, the functions of TALE transcription factors (TALE-TFs) and TALE recombinase (TALE-R) are diminished (Lamb et al. 2013). TALENs surpass ZFNs in terms of mouldability and exhibit a reduced off-target rate. Nevertheless, TALENs and ZFNs have challenges in protein synthesis, validation, and design, which serve as obstacles to their widespread implementation for standardized purposes.
CRISPR/Cas is an adaptive immune system found in bacteria and archaea that defends against viruses and plasmids (Barrangou et al. 2007). The CRISPR/Cas system comprises two primary components: (1) the CRISPR array; and (2) the Cas proteins (Westra et al. 2014; van Beljouw et al. 2023). The CRISPR array is a segment of the bacterial genome that consists of brief recurring sequences interspersed with distinct spacer sequences obtained from the genetic material of prior intruders, such as viruses or plasmids. Its purpose is to retain a record of past infections. The Cas proteins, also known as CRISPR-associated proteins, play a crucial role in identifying and cutting foreign genetic material. They operate by utilising the data stored in the CRISPR array to detect and selectively target particular regions in the foreign DNA or RNA (Atia et al. 2024). The CRISPR/Cas immunity pathway comprises multiple sequential stages. The CRISPR/Cas9 system was created in 2012 as a tool for editing genomes and has since become the most commonly employed technology for this purpose (Mali et al. 2013). The CRISPR/Cas9 system consists of two primary components: (1) Cas9, an RNA-guided DNA endonuclease; and (2) a single guide RNA (sgRNA). The CRISPR-Cas9 system identifies specific DNA sequences by means of DNA–RNA interaction. The Cas9 protein requires guidance to the target location provided by the sgRNA, which consists of 20 nucleotides that are complementary to the target sequences (Jiang et al. 2013a; Shan et al. 2013).
CRISP/Cas differs from ZFN and TALEN genome-editing methods as it identifies target DNA sequences through DNA-protein interaction. The CRISPR/Cas9 method necessitates the creation of a sgRNA, which may be adjusted for various genomic targets through the simple alteration of the complementary sequence (Jiang et al. 2013a). Cas9 cleaves the DNA strand at the spot where the sgRNA binds to the protospacer-adjacent motif (PAM) sequence, which is a short nucleotide sequence located near the cutting site at the 3′ end of the target region.
As previously mentioned, the CRISPR/Cas9-mediated targeted DSB can be repaired using either error-prone non-homologous end joining (NHEJ) or precise-mutation homology-directed repair (HDR). For HDR, accurate genetic changes can be made by using DNA repair donors that have homology arms. Nevertheless, achieving high-fidelity HDR while minimising the occurrence of NHEJ remains a significant obstacle (Miyaoka et al. 2016). The CRISPR-Cas9 system has undergone additional optimisation since its initial development, resulting in improved specificity and the ability to do precise genome-editing while minimising any impact on the genome (Jaganathan et al. 2018). Therefore, enhancements to CRISPR/Cas9 that reduce off-target effects and/or eliminate the protospacer adjacent motif (PAM) constraints can broaden its range of uses. Specifically, several methods have been demonstrated to effectively reduce unintended consequences, such as modifying Cas9 and sgRNA, conducting bioinformatics analysis, and optimising delivery methods (Gohil et al. 2021). However, various strategies for adapting have been developed through research to counteract the negative effects of biotic stressors. One method to mitigate the consequences of these pressures on crops is to investigate the genetics of the agents responsible for biotic and abiotic stressors in plants. Using genome-editing to develop resistant varieties of agricultural plants has proven to be an effective strategy for reducing the damaging effects of both biotic and abiotic pressures on plant (Knorre and Vlasov 1985; ul Haq et al. 2019).
According to Lu et al. (2018), the use of CRISPR/Cas9 technology for genome-editing in rice crop is a promising alternative to conventional breeding methods (Fig. 2) (Lu et al. 2018). Genome-editing is a scientific technique for modifying or regulating specific DNA sequences within a genome. CRISPR/Cas9 is a molecular tool that can be precisely engaged to make desirable modifications in targeted locations within complex genomes. According to Lu et al. (2018), the use of CRISPR/Cas9 technology for genome-editing in rice offers a promising alternative to conventional breeding methods (Fig. 2) (Hsu et al. 2014). In response to insect attacks on rice grains, researchers were able to produce CRISPR-based genetically modified (GM) rice in 2018 by suppressing the synthesis of serotonin, a neurotransmitter found in mammals (Lu et al. 2018) to develop rice gains that are resistant to planthoppers and stem borers (Chen et al. 2011; Iftikhar et al. 2023).
CRISPR systems for rice crop improvements
Enhancing plant resistance to biotic and abiotic stresses while increasing yield is the main goal of agricultural research (Delorge et al. 2014; Rehman et al. 2022; Rauf et al. 2024). Recently, more accurate methods for editing the genome have been created, replacing older techniques like random mutagenesis, naturally occurring mutations, and plant classical breeding. These conventional methods are time-consuming and inefficient in producing individuals with the desired characteristics. Emerging gene methodologies have become crucial instruments in the field of plant science and plant molecular breeding (Nazir et al. 2022). The progression of genome-editing technology has gone through multiple phases, including the use of ZFNs, TALENs, and the more recent development of the CRISPR/Cas system (Nazir et al. 2022; Dong 2024). CRISPR/Cas system is known for its versatility, simplicity, and cost-effectiveness, and has been the dominant method for genetic manipulation in the field of genome-editing since its discovery. Genome-editing technology has been extensively utilized for gene functional study and crop enhancement. The initial reports of rice and other plant genome-editing using CRISPR/Cas technology date back to 2013 (Jiang et al. 2013b; Miao et al. 2013; Xie and Yang 2013). Shan et al. (2013) created two sgRNAs to target the rice phytoene desaturase gene OsPDS and achieved a 15% mutagenesis efficiency in transformed rice (Nipponbare) protoplasts. In a study conducted by Jiang et al. (2013a), single guide RNAs (sgRNAs) were employed to manipulate the genes OsSWEET14 and OsSWEET11. These genes play a crucial role in the immune response to bacterial blight induced by X. oryzae. Following protoplast transformation, the presence of the desired modifications was verified using PCR and sequencing. Following the validation of CRISPR/Cas genome-editing in rice with the publishing of these results, numerous studies have been conducted to implement it for various applications (Fig. 3).
Visual presentation shows the CRISPR systems used for improvement in rice plants. The road map and timeline show the CRISPR systems along with targeted genes improved by these tools: CRISPR/SpCas9 (Shan et al. 2013); CRISPR/SpCas9-VQR (Hu et al. 2016); CRISPR/SpCas9-VRER (Hu et al. 2016); CRISPR/SaCas9 (Kaya et al. 2016)); CRISPR/FnCpf-1 (Endo et al. 2016); CRISPR/LbCpf-1 (Wang et al. 2017); CRISPR/AsCpf-1 (Tang et al. 2017a); CRISPR/LbCpf-1-RR (Li et al. 2018); CRISPR/LbCpf-1-RV and CRISPR/FnCpf-1-RR; and CRISPR/FnCpf-1-RVR (Zhong et al. 2018).
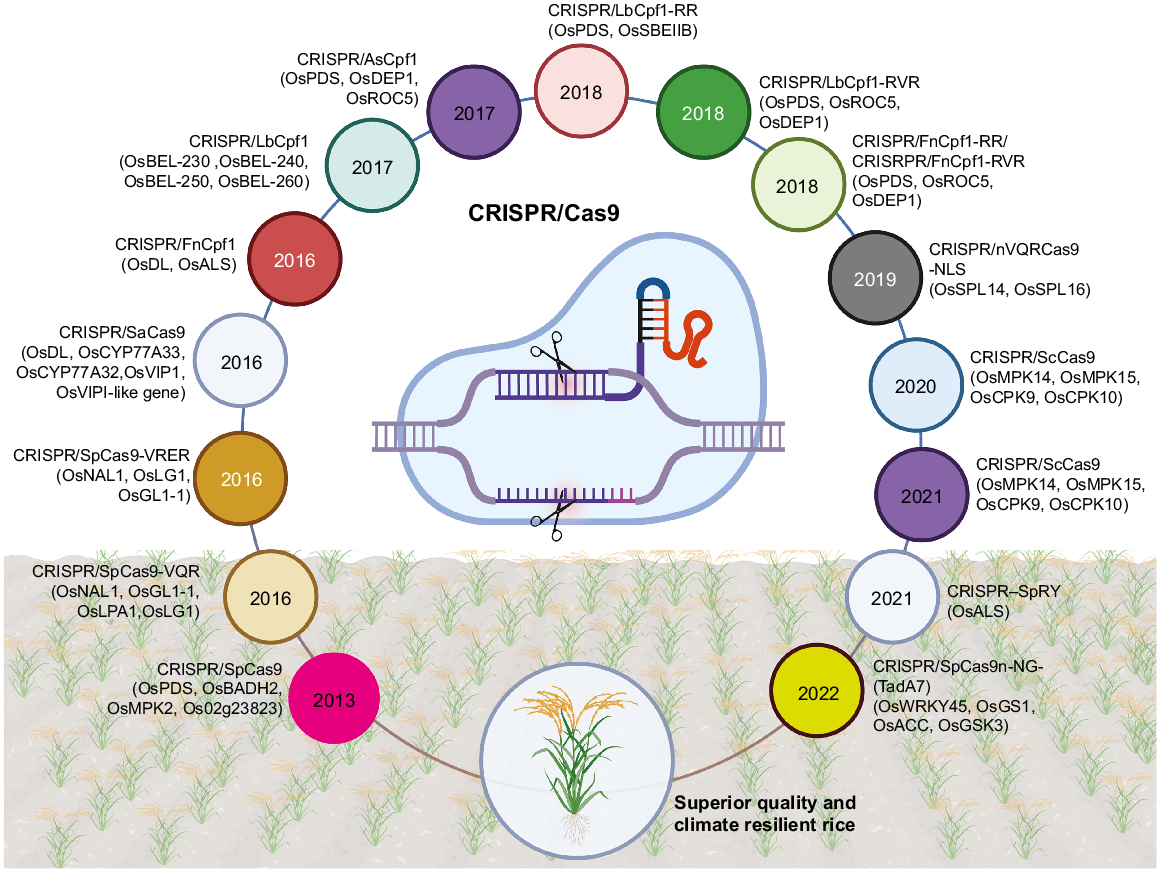
Applications of CRISPR/Cas in rice
This method has several interesting applications, one of which is the development of a genome-wide mutant library developmet (Fig. 4). This library may be utilized for determining the roles of genes and for improving genetics. Meng et al. (2017) combed through the rice genome in search of every possible sgRNA. They did this while keeping in mind that the seed region (12 bps immediately next to PAM) must only correlate once somewhere in the genome. In the exon regions, they discovered 1,535,852 target locations in 52,916 genes of rice. sgRNAs that are placed in exons that are close to the start of ORFs are chosen, and two sgRNAs from each candidate gene are picked. This is done so that loss-of-function mutations may be enriched. This process results in the production of 12,802 genes and 25,604 sgRNAs. Through the process of sequencing, the plasmid library’s quality, sgRNA accuracy, and gene coverage are evaluated. The Agrobacterium is then used for rice (cv. Zhonghai 11), resulting in the creation of over 14,000 distinct T0 lines. 182 T0 plants are arbitrarily sequenced to assess the accuracy and comprehensiveness of the mutations, with 139 of them having a single precise sgRNA. A total of 139 plants were found to have a single accurate sgRNA, with 136 of them being unique, implying that each sgRNA was well represented. Furthermore, potential off-target effects for specific sgRNAs were investigated, but no off-target modifications were discovered (Meng et al. 2017). Lu et al. (2017) employed a similar technique to the one described earlier to create a sgRNA library with 88,541 members that targeted 34,234 genes. The rice cultivar MSU7 has an average of 2.59 sgRNAs per gene (Lu et al. 2017). A sequencing process is used to validate the library’s creation. As a result, over 90% of the plasmid was confirmed and 99% of the genome was examined. Following that, the plasmid is transferred into Agrobacterium for rice (MSU7, ‘Rice Genome Annotation Project’ MSU version 7) modification. The mutation rate is 83.9%, resulting in the creation of 84,384 transgenic plants. A high-throughput genotyping procedure is used (Bell et al. 2014). This method was used on 9216 plants, of which 7004 were successfully identified and 86.4% had a sgRNA. This resulted in the identification of 5541 plants and the analysis of 2326 loci (Lu et al. 2017).
Different breeding and genome-editing methods and their timeframes required to produce improved rice varieties.
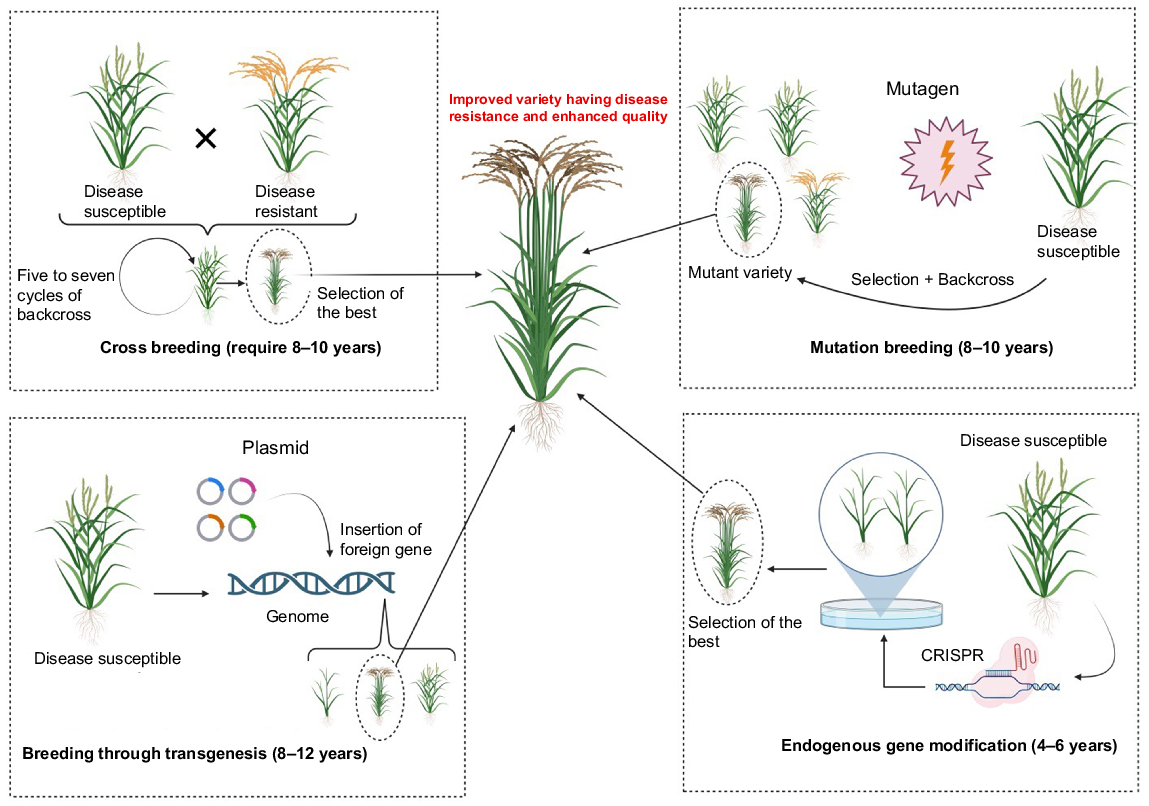
CRISPR/Cas technology is an influential tool for developing mutant libraries in rice, which can significantly speed up the breeding progression. Furthermore, it can help with the functional description of unidentified genes in rice and other crop plants. A notable and exciting application of the CRISPR/Cas system is the accurate removal of marker genes in genetically modified plants (Khan et al. 2021).
Biosafety is a critical factor in determining whether or not selection marker genes code for herbicide resistance and/or antibiotic resistance. Selection markers can also make the insertion of additional genes into transgenic lines more problematic. Consequently, eliminating and inserting selection markers should be prioritized. Two short guide RNAs were generated to target opposing ends of the GUS gene in the B1 transgenic rice (cv. Nipponbare) line in order to achieve this objective (Nandy and Srivastava 2012; Srivastava 2021). Using this technique, only five of 113 transgenic events showed evidence of the expected complete gene deletion. Excisions occur on both alleles in two of these events. When the sequence data for these events was examined, it was discovered that the excision was quite precise, as no mutations were found in either of the two cleavage sites (Srivastava et al. 2017). This newly discovered use of CRISPR/Cas has the potential to be utilized for the excision of selectable marker genes in rice and other plant species, thus enhancing breeding strategies (Fig. 4).
Strategies for high-yielding environmental stress resistant rice crop through CRISPR technology
To improve germplasm traits such as yield, the conventional breeding process includes selecting parental lines, crossbreeding, and multiple selection cycles, which can take up to seven to eight growing seasons to release a new variety. In contrast, CRISPR/Cas9 technology can accomplish this in less than 1 year (Hussain et al. 2018), and has been used to modify several negative regulator genes, resulting in enhanced yield and other significant agronomic characteristics. Being the foremost grain crop, rice has emerged as a prominent central focus for the CRISPR-based genome engineering technology, with the aim of enhancing its inherent characteristics. This method enables the mutation of several genes and quantitative trait loci (QTLs) in rice plants, with the aim of enhancing grain yield and other essential traits (Zihe et al. 2019; Riaz et al. 2021). Tables 2–5 show the genes of rice plants, that have been edited by CRISPR/Cas9.
Improved gene | Traits improved | Chromosomal number | MSU ID | Reference | |
---|---|---|---|---|---|
SD2 | Tiller number | – | – | Cui et al. (2020) | |
SCM1 | Stem cross-sectional area, Tiller number | 1 | – | Cui et al. (2020) | |
SCM2 | Stem cross-sectional area, Tiller number | 6 | LOC_Os06g45460.1 | Cui et al. (2020) | |
OsGA20ox2 | Reduced gibberellins, Reduced flag leaf length, Grain yield | 3 | LOC_Os03g63970.1 | Han et al. (2019) | |
SD1 | Resistance to lodging, grain yield | 1 | LOC_Os01g66100.1 | Hu et al. (2019) | |
OsPYL9 | Grain yield under limited water accessibility | 6 | LOC_Os06g33690.1 | Usman et al. (2020) | |
PYL1 | Grain yield, grain number | 10 | LOC_Os10g42280.1 | Miao et al. (2018) | |
PYL4 | Grain yield, grain number | 1 | LOC_Os01g61210.1 | Miao et al. (2018) | |
PYL6 | Grain yield, grain number | 3 | LOC_Os03g18600.1 | Miao et al. (2018) | |
OsBADH2 | Grain size, grain yield, aroma | 8 | LOC_Os08g32870.1 | Nawaz et al. (2020) | |
OsFWL | Number of tillers, grain size, number of cells in flag leaf grain length, flag leaf area, grain yield | 2 | LOC_Os02g52550.1 | Gao et al. (2020) | |
DEP1 | Grain size, grain number, grain yield, panicle architecture | 9 | LOC_Os09g26999.1 | Ma et al. (2015) | |
GS3 | Grain size, grain number, grain yield, panicle architecture | 12 | LOC_Os12g34380.1 | Ma et al. (2015) | |
Gn1a | Grain size, grain number, grain yield, panicle architecture | 1 | LOC_Os01g10110.1 | Ma et al. (2015) | |
OsSPL16 | Grain size, grain yield | 8 | LOC_Os08g41940.1 | Usman et al. (2021) | |
GW2 | Grain weight, grain yield | 2 | LOC_Os02g14720.1 | Xu et al. (2016) | |
GW5 | Grain weight, grain yield | 5 | LOC_Os05g09520.1 | Xu et al. (2016) | |
GW6 | Grain weight, grain yield | 6 | LOC_Os06g15620.1 | Xu et al. (2016) | |
OsPIN5b | Grain size, length of pinnacle | 8 | LOC_Os08g41720.1 | Zeng et al. (2020) | |
IPA1 | Number of tillers, grain yield, plant architecture, grain size, dense erect panicles, grain number | 8 | LOC_Os08g39890.1 | Schausberger (2018) | |
SBE1 | Starch metabolism | 6 | LOC_Os06g51084.1 | Romero and Gatica-Arias (2019) |
Disease/pathogen | Improved gene | Pathogen resistance/disease | Chromosome No. | MSU ID | References | |
---|---|---|---|---|---|---|
Fungi | OsERF922 | Magnaporthe oryzae/Rice blast | 1 | LOC_Os01g54890.1 | Schausberger (2018) | |
OsALB1 | Magnaporthe oryzae/Rice blast | 10 | LOC_Os10g38950.1 | Foster et al. (2018) | ||
OsUSTA | Ustilaginiodea virens/False smut | 10 | LOC_Os10g38950.1 | Liang et al. (2018) | ||
OsUvSLT2 | Ustilaginiodea virens/False smut | 10 | LOC_Os10g38950.1 | Liang et al. (2018) | ||
OsPi21 | Magnaporthe oryzae/Rice blast | 4 | LOC_Os04g32850.3 LOC_Os04g32850.1 LOC_Os04g32850.2 | Nawaz et al. (2020) | ||
OsRSY1 | Magnaporthe oryzae/Rice blast | 10 | LOC_Os10g38950.1 | Foster et al. (2018) | ||
Bacteria | OsSWEET11 | Xanthomonas oryzae pv. oryzae/Bacterial leaf blight | 8 | LOC_Os08g42350.1 | Kim et al. (2019) | |
OsSWEET13 | Xanthomonas oryzae pv. oryzae/Bacterial leaf blight | 12 | LOC_Os12g29220.1 | Oliva et al. (2019) | ||
OsSWEET14 | Xanthomonas oryzae pv. oryzae/Bacterial leaf blight | 11 | LOC_Os11g31190.1 | Xu et al. (2019) | ||
Os8N3 | Xanthomonas oryzae pv. oryzae/Bacterial leaf blight | 8 | LOC_Os08g42350.1 | Kim et al. (2019) | ||
OsXa13 | Xanthomonas oryzae pv. oryzae/Bacterial leaf blight | 8 | LOC_Os08g42350.1 | MacNeil et al. (2020) | ||
Virus | eIF4G | Rice tongro spherical virus | 7 | LOC_Os07g36940.1 | Macovei et al. (2018) | |
General disease resistance | OsMPK1 | Infection resistant signaling pathway | 6 | LOC_Os06g06090.1 | Minkenberg et al. (2017) | |
OsMPK2 | Infection resistant signaling pathway | – | – | Minkenberg et al. (2017) | ||
OsMPK5 | Infection resistant signaling pathway | 3 | LOC_Os03g17700.1 | Xie and Yang (2013) | ||
OsMPK6 | Infection resistant signaling pathway | 10 | LOC_Os10g38950.1 | Minkenberg et al. (2017) | ||
Herbicide resistance | OsEPSPS | Glyphosate | – | – | Li et al. (2016) | |
OsALS | Bispyrabic-sodium, Imazamox, Imazethapyr-IMT and Imazapic-IMP, Nicosulphuron, Pyroxasulam, Flucarbazonesodium | 2 | LOC_Os02g30630.1 | Malhotra et al. (2016), Shimatani et al. (2017), Kuang et al. (2020) and Zhang and Gao (2020) | ||
OsFTIP1 | Imazamox | 6 | LOC_Os06g41090.1 | Shimatani et al. (2017) |
Stress | Improved gene | Chromosome number | MSU ID | Reference | |
---|---|---|---|---|---|
Drought | OsSAPK2 | 7 | LOC_Os07g42940.1 | Lou et al. (2017) | |
OsSRL1 | 7 | LOC_Os07g01240.1 | Liao et al. (2019) | ||
OsSRL2 | 3 | LOC_Os03g19520.1 | Liao et al. (2019) | ||
OsPYL9 | 6 | LOC_Os06g33690.1 | Usman et al. (2020) | ||
OsERA1 | 1 | LOC_Os01g53600.1 | Ogata et al. (2020) | ||
DST | 3 | LOC_Os03g57240.1 | Santosh Kumar et al. (2020) | ||
OsmiR535 | – | – | Yue et al. (2020) | ||
Salinity | OsSAPK2 | 7 | LOC_Os07g42940.1 | Lou et al. (2017) | |
OsRR22 | 6 | LOC_Os06g08440.1 | Zhang et al. (2019a) | ||
DST | 3 | LOC_Os03g57240.1 | Santosh Kumar et al. (2020) | ||
OsmiR535 | – | – | Yue et al. (2020) | ||
Cold stress | OsAnn3 | 5 | LOC_Os05g31750.1 | Shen et al. (2017) | |
OsMYB30 | 2 | LOC_Os02g41510.1 | Zeng et al. (2020) |
MSU ID and chromosome numbers are derived from (https://shigen.nig.ac.jp/rice/oryzabase/quicksearch/list).
Heavy metal | Targeted gene | Chromosome number | MSU ID | Reference | |
---|---|---|---|---|---|
Cs | OsHAK1 | 4 | LOC_Os04g32920.1 | Nieves-Cordones et al. (2017) | |
Mn, Cd, Fe, Pb | OsNramp5 | 7 | LOC_Os07g15370.1 | Tang et al. (2017b) | |
Cd | OsLCT1 | 6 | LOC_Os06g38120.1 | Songmei et al. (2019) | |
Cd | OsLCT2 | 6 | – | Tang et al. (2022) | |
Cd, As | OsNRAMP1 | 7 | LOC_Os07g15460.1 | Chang et al. (2020) | |
Cd | OsABCG36 | 1 | LOC_Os01g42380.1 | Fu et al. (2019) | |
Si, As | OsLsi | 6 | LOC_Os06g12310.1 | Hillary and Ceasar (2022) |
Biotic stress tolerance in rice by CRISPR
It is estimated that biotic stressors, which include infections caused by bacteria, viruses, and fungi are responsible for 20–40% of the total losses in agricultural production across the globe (Gimenez et al. 2018; Moustafa-Farag et al. 2020; Bhoi et al. 2022). Repetitive stress exposures have caused significant yield loss in plants over time, which has had a severe impact on the food secuity worldwide (Richard et al. 2022). Scientists have used CRISPR technology to develop rice crops that are resistant to a variety of pathogenic microorganisms (Chen et al. 2019; Karmakar et al. 2022) (Table 3).
Fungal infections, particularly powdery mildew, frequently impede crop productivity and can be disastrous. Another fungal disease that can have a significant impact on agricultural productivity is rice blast. The OsSEC3A and OsERF922 genes were targeted by researchers using the CRISPR/Cas9 technology in order to significantly boost resistance to rice blast infection (Wang et al. 2016a; Ma et al. 2018). The OsERF922 mutant did not exhibit any alterations in its agronomic traits. However, researchers observed an increase in SA content in OsSEC3A, which led to a reduction in its size (Ma et al. 2018). The partial resistance to rice blast can also be induced by CRISPR/Cas9-mediated mutations of rice Bsr-d1 and Pi21 genes. However, the magnitude of this effect is comparatively weaker than that of OsERF922 (Nawaz et al. 2020; Zhou et al. 2022).
According to Schloss and Handelsman (2004), numerous bacterial species have the potential to induce disease in plants, with several of them manifesting various disease symptoms (Schloss and Handelsman 2004). The management of plant pathogenic bacteria is complicated due to difficulties in early disease detection and insufficient pesticides. However, the application of the CRISPR/Cas9 approach in plant genome modification has been shown to enhance crop resistance to bacterial diseases. Susceptibility is linked to the OsSWEET13 gene, which encodes a sucrose transporter and plays a significant character in plant–pathogen interaction. X. oryzae produces an effector protein called PthXo2 in affected plants, which triggers the expression of OsSWEET13 in the host, leading to susceptibility in rice. Resistance against bacterial blight was increased in rice plants by suppressing the OsSWEET13 promoter (Zhou et al. 2015).
Abiotic stress tolerance in rice by CRISPR
Salinity, drought, heavy metals, and extreme temperature exposure are all examples of abiotic stresses that have an important impact on plant development and growth (Table 4). These factors have the potential to reduce crop production by up to 50% (Liu et al. 2022; Nawaz et al. 2023; Noman and Azhar 2023). According to research, salinization has affected 7% of the world’s land and 20% of its arable land, and there are signs that the problem will worsen (Al Murad et al. 2020). The activation of osmotic stress, ion stress, and secondary stress pathways comes about as a consequence of plants being subjected to salt stress (Yang and Guo 2018). This occurrence has been observed to have a negative influence on crop yield and quality (Siddiqui et al. 2017; Ali et al. 2024). It has been found that using CRISPR/Cas9 technology to cause mutation in the OsRR22 gene increases salt tolerance in rice (Zhang et al. 2019b; Han et al. 2022), while other agronomic traits remained unchanged. Using CRISPR/Cas9 technology, Liu et al. (2020) were able to effectively alter the OsRAV2 gene (Liu et al. 2020). Researchers successfully mutated the OsRAV2 gene using CRISPR/Cas9 technology, resulting in a mutant rice crop with increased survival rates when subjected to salt stress. Furthermore, using CRISPR/Cas9 technology to disrupt genes like OsDST, OsNAC041, and OsmiR535 has been shown to significantly improve rice’s ability to tolerate salt-induced stress. Ogata et al. (2020) discovered that using CRISPR/Cas9-mediated mutagenesis on OsERA1 led to an important enhancement in drought stress tolerance in rice (Wang et al. 2016b; Ogata et al. 2020; Santosh Kumar et al. 2020; Yue et al. 2020). CRISPR-based genome-editing technology has been used to improve the rice plant’s ability to withstand extreme temperatures, both cold and heat. Specifically, using this technology, the OsAnn3 gene in rice was mutated to confer tolerance to cold stress in the plant (Kumar et al. 2022).
Resistance to heavy metal contamination in rice by CRISPR
The negative consequences of heavy metals on plant growth and yield pose a significant barrier to agricultural sustainability. Stresses such as oxidative stress, osmotic ion imbalance, membrane tissue instability, cell death, and disrupted metabolic equilibrium detrimentally impact on plant physiology and biochemistry (Hoque et al. 2021). The ingestion of plants that have accumulated heavy metals can result in significant harm to human and animal health (Kaur et al. 2021). A previous study found that using CRISPR/Cas9 technology to knock down the OsLCT1 and OsNramp5 genes reduced Cd accumulation in rice (Table 5). Another study found that disrupting OsNRAMP1 with CRISPR/Cas9 reduced the levels Cd and Pb in rice grains (Chang et al. 2020; Chu et al. 2022). Simultaneously, it was discovered that OsNRAMP5 and OsNRAMP1 have different abilities to reduce Cd accumulation. The CRISPR/Cas9 method was used to prevent arsenic absorption and transportation in rice by disrupting the R2R3 MYB transcription factor OsARM1. Furthermore, Nieves-Cordones et al. (2017) used the CRISPR/Cas9 technique to inactivate the K+ transporter OsHAK1 to produce rice plants with lower cesium (Cs) levels (Nieves-Cordones et al. 2017; Chang et al. 2020). Researchers employed CRISPR/Cas9 technology to create oslcd single mutants derived from both indica and japonica rice crops. In addition, we created single mutants of osnramp5 and double mutants of oslcd, and osnramp5 in the indica background. When cultivated in paddy soils contaminated with Cd, all single mutants of oslcd acquired a lower amount of Cd compared to the wild-types (Chen et al. 2023). Li et al. (2022) findings suggest that CRISPR/Cas9 mutated OsPMEI12 plays a role in controlling the process of methyl esterification during growth, impacting the composition of the cell wall and several agronomic features. Additionally, it is likely to have a significant function in responding to phytohormones and Cd stress. The application of CRISPR/Cas9 resulted in a higher accumulation of Cd in rice straws, seeds, and brown rice due to mutation. Conversely, the overexpression lines exhibited comparatively lower quantities of Cd. The findings indicate that the metal chaperone gene OsHIPP56 can suppress the harmful effects of cadmium by decreasing its accumulation in crops. In summary, our work contributes to the comprehension of the functional significance of OsHIPP56 in rice’s detoxification of Cd (Zhao et al. 2022).
Limitations
CRISPR/Cas9 has multiple applications in plant breeding, although it does have specific constraints. The primary obstacle is in the limited availability of a narrow gene pool regulating vital phenotype. Therefore, the accessibility of genes is vital for this tool. CRISPR/Cas9 still has relatively few restrictions (Fig. 5), even though it has numerous benefits and a wide range of applications. However, there are a few imminent challenges that might impede the effectiveness of the CRISPR/Cas9 system in the creation of disease resistance. These challenges are in Fig. 5.
SpCas9 necessitates a 50-NGG-30PAM in close proximity to the 20 nt DNA target sequence because to its exclusive recognition of NGG PAM sites. This constraint restricts its efficacy. While this restriction is crucial, the objective is to deactivate the gene using selected mutagenesis in any circumstance. However, PAM restricts the editing of resistance genes. Hence, it is necessary to select sequences that are located near PAM (Mohamed et al. 2024). It is possible that the direct manipulation of S genes would result in a loss of fitness owing to the S genes’ connection with other desired genes, in particular, the genes that govern plant development and growth. In addition, the mutation in the targeted gene might cause the route of the product to become disrupted, which would eventually have a negative impact on a large number of other products that use the same pathway. This cost to fitness is not as devastating as the previous one, but it still has the potential to result in a lack of key micronutrients and phenotypic abnormalities. Nevertheless, the cost of viability may vary due to the targeting of specific gene/s, and it is also dependent on various other parameters, like disease susceptibility facilitators, defense silencers, genes, or post relevant aspects in the reporter gene (van Schie and Takken 2014). Research on naturally occurring or synthetically produced pathogen inactivating promoters or transcription factors has been the primary focus of scientists during the past two decades (Rushton et al. 2002). By employing regulatory elements, it is possible to develop pathogen-inducible CRISPR systems. These systems may transiently knock off a S gene that corresponds to a particular micronutrient or a sugar promoter, so avoiding the fitness repercussions that would otherwise occur. Editing may be used to create desirable S gene mutants in almost all plants that are of interest for breeding, bypassing species boundaries in the process. It is anticipated that new S genes will be identified, which will result in the availability of additional sites for genome-editing.
‘Off-target mutations’ is major factor that cause hindrance (Hahn and Nekrasov 2019). Off-target genome-editing refers to genetic changes occurring at unplanned and non-specific regions. This type of editing can occur due to erroneous gRNA or in a gRNA-independent manner (Jin et al. 2019). CRISPR/Cas can cause random off-target variations in the plant DNA. The lack of ability to effectively extract Cas proteins and generate transgene-free crops has impeded the commercialization of CRISPR-edited crops (Wang et al. 2022b). The majority of the work that has been done to solve the issue of off-target editing can be broken down into two categories: (1) development of a mechanism for off-target detection; and (2) engineering of the CRISPR mechanism for high-quality modification. To date, a variety of bioinformatics tools have been created to address this problem. Some examples of these tools are CasOFFinder and CCTop (Koo et al. 2015), as well as DISCOVER (Wienert et al. 2019). Because each analytical instrument has its own set of benefits and drawbacks, researchers may need to choose an analytical method that is appropriate for the task. In contrast, great progress has been made in reducing the off-target activity of CRISPR/Cas9, which is an encouraging development. Cas9 proteins with enhanced target selectivity have to be created first. These Cas9 proteins include eSpCas9 (Slaymaker et al. 2016), Sniper Cas9 (Lee et al. 2018), HF-Cas9 (Kleinstiver et al. 2016), and HypaCas9 (Chen et al. 2017). Sniper Cas9 was selected from a collection of SpCas9 mutants that displayed improved specificity, while HypaCas9, HiFiCas9, and eSpCas9 were produced by rational design for structural alterations to boost specificity. The majority of the modified Cas proteins exhibited a striking drop in off-target levels while maintaining their activity on the intended target. In addition, improvements in specificity have been made through the creation of gRNA (Moon et al. 2019). Recent studies have demonstrated the occurrence of off-target mutations in rice as a result of cytosine base-editing. Jin et al. (2019), but not adenine-based editors. This finding demonstrates that the new tools also need some kind of modification.
Both the safety of people and other living species, as well as questions about commercialization, are closely tied to these concerns. Because the subsequent transgenic does not contain any foreign nuclei, the crops evolved through transgene-free mechanisms would not be labeled as transgenic, and it would be easier to adopt them for commercial cultivation. This is because the TKC and VIGE systems do not involve the use of any foreign material. However, the widespread use of genome-edited crops presents a difficult ethical conundrum. Concerning this issue, certain states have openly adopted genome-edited crops and a significant number of nations are presently engaged in debates surrounding this topic. A European court has ruled that the crop with changed genome is considered genetically modified. Despite the fact that the decision has certain technical limitations, which may be reviewed by member states, there is still a significant opportunity for the creation of new breeds and varieties quickly and effectively (Abbott 2018). It is anticipated that the use of this technology would unquestionably go a long way toward creating conditions that would facilitate the acceptance of genetically modified crops in the majority of nations in a manner that is comparatively straightforward.
In the past, the CRISPR/Cas9 system has been used to alter RNA and DNA based viruses and it has assisted researchers in the production of plant material that is resistant to viruses. However, the efficacy of this mechanism has been called into doubt since it has been shown to be ineffective against the viral immunity found in eukaryotes. Based on the results of Mehta et al. (2019), we may hypothesize that CRISPR/Cas9 has significant limits not just against DNA viruses but also RNA viruses, and these restrictions are caused by the viruses’ ability to escape and rapidly replicate. As a result, there is an immediate need for the creation of a version of CRISPR that is efficient, effective, and socially acceptable, such as CRISPR/Cas13a. This will allow for the resolution of problems caused by viruses. CRISPR/Cas13a has tremendous ability as just a precise, robust, and expandable RNA-targeting channel for RNA manipulation techniques and RNA virus targeting, according to recent findings (Aman et al. 2018). These results advocate that among the three Cas13 protein families (Cas13a, Cas13b, and Cas13c), CRISPR/Cas13a holds the most promise as an RNA-targeting platform. Cas13a enzymes have the potential to be repurposed as tools for a variety of applications, including the detection of nucleic acids, the suppression of RNA in mammalian and plant cells, and the monitoring of transcripts (Abudayyeh et al. 2017; Rehman et al. 2022). RNA interference with Cas13a may occur even without the biological protospacer-f-linking sequence (PFS). This finding is interesting as in the not-too-distant future, Cas13 could prove to be a superior option to CRISPR/Cas9 when it comes to targeting viral RNA. CRISPR-based-editing holds the capacity to enhance crops by increasing their ability to withstand abiotic and biotic stress and improving their nutritional composition. The CRISPR-based genome-editing technique is widely recognized as a highly potent technology for enhancing agriculture to sustain the constantly expanding population.
Future perspective
CRISPR technology has revolutionized agriculture by addressing specific crop problems and providing significant solutions to important agricultural issues. CRISPR/Cas9 technology is being employed to improve the quality, yield, and resilience of both monocots and dicots by precisely editing crop genomes. This approach enables the modification of specific genes, enhancing the plant’s ability to withstand biotic and abiotic stresses, ultimately leading to more robust and productive crop varieties. Rice is one of the world’s most important food crops, playing an imperative role in global food security. In light of increasing challenges posed by climate change and population growth, significant efforts are focused on developing new rice varieties that exhibit enhanced agronomic traits, particularly resilience to various biotic and abiotic stresses. The CRISPR/Cas9 system has emerged as a groundbreaking tool in this endeavor, offering unparalleled efficiency, simplicity, and versatility to precisely improve various crop traits. CRISPR/Cas technology has rapidly advanced, leading to DNA-free genome-editing systems, Cas9 variants, multi-gene targeting, and enhanced Homology-Directed Repair. Recent computational techniques have amplified its effectiveness, driving its growing adoption in real world applications.
CRISPR/Cas9 technology enables targeted rice genome modifications, enhancing adaptability and productivity, contributing to global food security in a rapidly changing climatic era. The regulatory frameworks around genetically modified organisms (GMOs) continue to be a major issue, irrespective of the considerable potential of CRISPR/Cas9 in rice enhancement. Although some regulatory concerns may be addressed by the capacity to modify genes without introducing foreign DNA, discussions over the implications of gene-editing in agriculture will continue to determine the technology’s potential uses. Communicating CRISPR/Cas-mediated genetic modification to the public is crucial for agriculture’s future. Establishing legal distinctions between gene-edited plants with foreign genes and those without is essential. Exemptions from regulations can facilitate easier integration. Clear regulations are crucial for the marketing approval of transgene-free, genome-edited crops, especially in regions where such practices are not yet prevalent. Advanced genome-editing techniques, like CRISPR/Cas9, hold significant promise for agricultural innovation; however, the lack of well-defined regulatory frameworks is widening the gap between laboratory advancements and practical field applications. To facilitate the safe and effective deployment of these technologies, comprehensive risk assessments, regulatory oversight, and precautionary measures are essential. Furthermore, optimizing transformation procedures is vital for the successful integration of CRISPR/Cas genome-editing into crop improvement initiatives. Community and private sector facilities can bridge the gap between scientific breakthroughs and practical agriculture by supplying advanced genome-editing tools, streamlining crop development processes, and fostering public acceptance of genome-edited crops through the implementation of science-based regulations. Our review will be useful in understanding the latest technical advancements, knowledge gaps, and challenges with technical adaptations that are necessary for the optimal usage of genome-editing techniques for crop development.
Compliance with ethical standards
This manuscript does not contain any studies with humans/animals performed by any authors.
Declaration of funding
This work is supported by the Ministry of National Food Security and Research of Pakistan released for the PSDP-project ‘Sino-Pak Agricultural Breeding Innovations Project for Rapid Yield Enhancement’ (857).
Author contributions
AAR and MRK designed the study. MU, AR, SI, BB, SJ, RI, and AAR data collection, data analysis, and writing of the original draft. MU and MRK revision of the article. MRK supervised the research. All authors review and edit the manuscript.
Acknowledgements
The authors acknowledge the research facilities provided by the Functional Genomics and Bioinformatics Group, National Institute for Genomics and Advanced Biotechnology (NIGAB), Pakistan.
References
Abbott A (2018) European court suggests relaxed gene-editing rules. Nature 19,.
| Crossref | Google Scholar |
AbdElgawad H, Mohammed AE, van Dijk JR, Beemster GTS, Alotaibi MO, Saleh AM (2023) The impact of chromium toxicity on the yield and quality of rice grains produced under ambient and elevated levels of CO2. Frontiers in Plant Science 14, 1019859.
| Crossref | Google Scholar |
Abudayyeh OO, Gootenberg JS, Essletzbichler P, Han S, Joung J, Belanto JJ, Verdine V, Cox DBT, Kellner MJ, Regev A, et al. (2017) RNA targeting with CRISPR–Cas13. Nature 550, 280-284.
| Crossref | Google Scholar | PubMed |
Adil M, Shah AN, Khan AN, Younas T, Mehmood MS, Mahmood A, Asghar RMA, Javed MS (2023) Amelioration of harmful effects of soil salinity in plants through silicon application: a review. Pakistan Journal of Botany 55, 9-18.
| Crossref | Google Scholar |
Ali L, Khan MB, Ahmed F, Nisar I, Fatima A, Khadim MU, Hafeez HT, Tariq S, Hanif S, Zahra M, et al. (2024) Influence of abiotic stresses on the production and sustainability of rice crop. Agrobiological Records 16, 99-108.
| Crossref | Google Scholar |
Al Murad M, Khan AL, Muneer S (2020) Silicon in horticultural crops: cross-talk, signaling, and tolerance mechanism under salinity stress. Plants 9, 460.
| Crossref | Google Scholar | PubMed |
Aman R, Ali Z, Butt H, Mahas A, Aljedaani F, Khan MZ, Ding S, Mahfouz M (2018) RNA virus interference via CRISPR/Cas13a system in plants. Genome Biology 19, 1.
| Crossref | Google Scholar |
Ashraf U, Kanu AS, Deng Q, Mo Z, Pan S, Tian H, Tang X (2017) Lead (Pb) toxicity; physio-biochemical mechanisms, grain yield, quality, and Pb distribution proportions in scented rice. Frontiers in Plant Science 8, 259.
| Crossref | Google Scholar | PubMed |
Atia M, Jiang W, Sedeek K, Butt H, Mahfouz M (2024) Crop bioengineering via gene editing: reshaping the future of agriculture. Plant Cell Reports 43, 98.
| Crossref | Google Scholar | PubMed |
Audebert A, Fofana M (2009) Rice yield gap due to iron toxicity in West Africa. Journal of Agronomy and Crop Science 195, 66-76.
| Crossref | Google Scholar |
Aziz H, Sabir M, Ahmad HR, Aziz T, Zia-ur-Rehman M, Hakeem KR, Ozturk M (2015) Alleviating effect of calcium on nickel toxicity in rice. CLEAN–Soil, Air, Water 43, 901-909.
| Crossref | Google Scholar |
Barrangou R, Fremaux C, Deveau H, Richards M, Boyaval P, Moineau S, Romero DA, Horvath P (2007) CRISPR provides acquired resistance against viruses in prokaryotes. Science 315, 1709-1712.
| Crossref | Google Scholar | PubMed |
Bell CC, Magor GW, Gillinder KR, Perkins AC (2014) A high-throughput screening strategy for detecting CRISPR-Cas9 induced mutations using next-generation sequencing. BMC Genomics 15, 1002.
| Crossref | Google Scholar |
Bhoi A, Yadu B, Chandra J, Keshavkant S (2022) Mutagenesis: a coherent technique to develop biotic stress resistant plants. Plant Stress 3, 100053.
| Crossref | Google Scholar |
Bibikova M, Golic M, Golic KG, Carroll D (2002) Targeted chromosomal cleavage and mutagenesis in Drosophila using zinc-finger nucleases. Genetics 161, 1169-1175.
| Crossref | Google Scholar | PubMed |
Boyd CE, D’Abramo LR, Glencross BD, Huyben DC, Juarez LM, Lockwood GS, McNevin AA, Tacon AGJ, Teletchea F, Tomasso JR, Jr, et al. (2020) Achieving sustainable aquaculture: historical and current perspectives and future needs and challenges. Journal of the World Aquaculture Society 51, 578-633.
| Crossref | Google Scholar |
Bueno PCP, Lopes NP (2020) Metabolomics to characterize adaptive and signaling responses in legume crops under abiotic stresses. ACS Omega 5, 1752-1763.
| Crossref | Google Scholar | PubMed |
Carlson DF, Fahrenkrug SC, Hackett PB (2012) Targeting DNA with fingers and TALENs. Molecular Therapy-Nucleic Acids 1, e3.
| Crossref | Google Scholar |
Carroll D (2021) A short, idiosyncratic history of genome editing. Gene and Genome Editing 1, 100002.
| Crossref | Google Scholar |
Carroll D, Morton JJ, Beumer KJ, Segal DJ (2006) Design, construction and in vitro testing of zinc finger nucleases. Nature Protocols 1, 1329-1341.
| Crossref | Google Scholar | PubMed |
Chang J-D, Huang S, Yamaji N, Zhang W, Ma JF, Zhao F-J (2020) OsNRAMP1 transporter contributes to cadmium and manganese uptake in rice. Plant, Cell & Environment 43, 2476-2491.
| Crossref | Google Scholar | PubMed |
Chen M, Shelton A, Ye G-Y (2011) Insect-resistant genetically modified rice in China: from research to commercialization. Annual Review of Entomology 56, 81-101.
| Crossref | Google Scholar | PubMed |
Chen JS, Dagdas YS, Kleinstiver BP, Welch MM, Sousa AA, Harrington LB, Sternberg SH, Joung JK, Yildiz A, Doudna JA (2017) Enhanced proofreading governs CRISPR–Cas9 targeting accuracy. Nature 550, 407-410.
| Crossref | Google Scholar | PubMed |
Chen K, Wang Y, Zhang R, Zhang H, Gao C (2019) CRISPR/Cas genome editing and precision plant breeding in agriculture. Annual Review of Plant Biology 70, 667-697.
| Crossref | Google Scholar | PubMed |
Chen H, Ye R, Liang Y, Zhang S, Liu X, Sun C, Li F, Yi J (2023) Generation of low-cadmium rice germplasms via knockout of OsLCD using CRISPR/Cas9. Journal of Environmental Sciences 126, 138-152.
| Crossref | Google Scholar |
Christian M, Cermak T, Doyle EL, Schmidt C, Zhang F, Hummel A, Bogdanove AJ, Voytas DF (2010) Targeting DNA double-strand breaks with TAL effector nucleases. Genetics 186, 757-761.
| Crossref | Google Scholar | PubMed |
Chu C, Huang R, Liu L, Tang G, Xiao J, Yoo H, Yuan M (2022) The rice heavy-metal transporter OsNRAMP1 regulates disease resistance by modulating ROS homoeostasis. Plant, Cell & Environment 45, 1109-1126.
| Crossref | Google Scholar | PubMed |
Cui Y, Hu X, Liang G, Feng A, Wang F, Ruan S, Dong G, Shen L, Zhang B, Chen D, et al. (2020) Production of novel beneficial alleles of a rice yield-related QTL by CRISPR/Cas9. Plant Biotechnology Journal 18, 1987.
| Crossref | Google Scholar | PubMed |
Das A, Sharma N, Prasad M (2019) CRISPR/Cas9: a novel weapon in the arsenal to combat plant diseases. Frontiers in Plant Science 9, 2008.
| Crossref | Google Scholar | PubMed |
Delorge I, Janiak M, Carpentier S, Van Dijck P (2014) Fine tuning of trehalose biosynthesis and hydrolysis as novel tools for the generation of abiotic stress tolerant plants. Frontiers in Plant Science 5, 147.
| Crossref | Google Scholar |
Dong H (2024) Application of genome editing techniques to regulate gene expression in crops. BMC Plant Biology 24, 100.
| Crossref | Google Scholar | PubMed |
Dong OX, Ronald PC (2019) Genetic engineering for disease resistance in plants: recent progress and future perspectives. Plant Physiology 180, 26-38.
| Crossref | Google Scholar | PubMed |
Durai S, Mani M, Kandavelou K, Wu J, Porteus MH, Chandrasegaran S (2005) Zinc finger nucleases: custom-designed molecular scissors for genome engineering of plant and mammalian cells. Nucleic Acids Research 33, 5978-5990.
| Crossref | Google Scholar | PubMed |
El-Badri AM, Hashem AM, Batool M, Sherif A, Nishawy E, Ayaad M, Hassan HM, Elrewainy IM, Wang J, Kuai J, et al. (2022) Comparative efficacy of bio-selenium nanoparticles and sodium selenite on morpho-physiochemical attributes under normal and salt stress conditions, besides selenium detoxification pathways in Brassica napus L. Journal of Nanobiotechnology 20, 163.
| Crossref | Google Scholar |
Endo A, Masafumi M, Kaya H, Toki S (2016) Efficient targeted mutagenesis of rice and tobacco genomes using Cpf1 from Francisella novicida. Scientific Reports 6, 38169.
| Crossref | Google Scholar | PubMed |
Falcon WP, Naylor RL, Shankar ND (2022) Rethinking global food demand for 2050. Population and Development Review 48, 921-957.
| Crossref | Google Scholar |
Foster AJ, Martin-Urdiroz M, Yan X, Wright HS, Soanes DM, Talbot NJ (2018) CRISPR-Cas9 ribonucleoprotein-mediated co-editing and counterselection in the rice blast fungus. Scientific Reports 8, 14355.
| Crossref | Google Scholar |
Frei ER, Gossner MM, Vitasse Y, Queloz V, Dubach V, Gessler A, Ginzler C, Hagedorn F, Meusburger K, Moor M, et al. (2022) European beech dieback after premature leaf senescence during the 2018 drought in northern Switzerland. Plant Biology 24, 1132-1145.
| Crossref | Google Scholar | PubMed |
Fu S, Lu Y, Zhang X, Yang G, Chao D, Wang Z, Shi M, Chen J, Chao D-Y, Li R, et al. (2019) The ABC transporter ABCG36 is required for cadmium tolerance in rice. Journal of Experimental Botany 70, 5909-5918.
| Crossref | Google Scholar | PubMed |
Gao Q, Li G, Sun H, Xu M, Wang H, Ji J, Wang D, Yuan C, Zhao X (2020) Targeted mutagenesis of the rice FW 2.2-like gene family using the CRISPR/Cas9 system reveals OsFWL4 as a regulator of tiller number and plant yield in rice. International Journal of Molecular Sciences 21, 809.
| Crossref | Google Scholar | PubMed |
Ghosh S, Dey G (2022) Biotic and abiotic stress tolerance through CRISPR-Cas mediated genome editing. Journal of Plant Biochemistry and Biotechnology 31, 227-238.
| Crossref | Google Scholar |
Giller KE, Delaune T, Silva JV, Descheemaeker K, van de Ven G, Schut AGT, van Wijk M, Hammond J, Hochman Z, Taulya G, et al. (2021) The future of farming: who will produce our food? Food Security 13, 1073-1099.
| Crossref | Google Scholar |
Gimenez E, Salinas M, Manzano-Agugliaro F (2018) Worldwide research on plant defense against biotic stresses as improvement for sustainable agriculture. Sustainability 10, 391.
| Crossref | Google Scholar |
Gohil N, Bhattacharjee G, Lam NL, Perli SD, Singh V (2021) CRISPR-Cas systems: challenges and future prospects. Progress in Molecular Biology and Translational Science 180, 141-151.
| Crossref | Google Scholar | PubMed |
Hahn F, Nekrasov V (2019) CRISPR/Cas precision: do we need to worry about off-targeting in plants? Plant Cell Reports 38, 437-441.
| Crossref | Google Scholar | PubMed |
Han Y, Teng K, Nawaz G, Feng X, Usman B, Wang X, Luo L, Zhao N, Liu Y, Li R (2019) Generation of semi-dwarf rice (Oryza sativa L.) lines by CRISPR/Cas9-directed mutagenesis of OsGA20ox2 and proteomic analysis of unveiled changes caused by mutations. 3 Biotech 9, 387.
| Crossref | Google Scholar |
Han X, Chen Z, Li P, Xu H, Liu K, Zha W, Li S, Chen J, Yang G, Huang J, et al. (2022) Development of novel rice germplasm for salt-tolerance at seedling stage using CRISPR-Cas9. Sustainability 14, 2621.
| Crossref | Google Scholar |
Heredia MC, Kant J, Prodhan MA, Dixit S, Wissuwa M (2022) Breeding rice for a changing climate by improving adaptations to water saving technologies. Theoretical and Applied Genetics 135, 17-33.
| Crossref | Google Scholar | PubMed |
Hillary VE, Ceasar SA (2022) Prime editing in plants and mammalian cells: mechanism, achievements, limitations, and future prospects. BioEssays 44, 2200032.
| Crossref | Google Scholar |
Hinojosa L, Matanguihan JB, Murphy KM (2019) Effect of high temperature on pollen morphology, plant growth and seed yield in quinoa (Chenopodium quinoa Willd.). Journal of Agronomy and Crop Science 205, 33-45.
| Crossref | Google Scholar |
Hoque MN, Tahjib-Ul-Arif M, Hannan A, Sultana N, Akhter S, Hasanuzzaman M, Akter F, Hossain MS, Sayed MA, Hasan MT, et al. (2021) Melatonin modulates plant tolerance to heavy metal stress: morphological responses to molecular mechanisms. International Journal of Molecular Sciences 22, 11445.
| Crossref | Google Scholar | PubMed |
Hsu PD, Lander ES, Zhang F (2014) Development and applications of CRISPR-Cas9 for genome engineering. Cell 157, 1262-1278.
| Crossref | Google Scholar | PubMed |
Hu X, Wang C, Fu Y, Liu Q, Jiao X, Wang K (2016) Expanding the range of CRISPR/Cas9 genome editing in rice. Molecular Plant 9, 943-945.
| Crossref | Google Scholar | PubMed |
Hu X, Cui Y, Dong G, Feng A, Wang D, Zhao C, Zhang Y, Hu J, Zeng D, Guo L, et al. (2019) Using CRISPR-Cas9 to generate semi-dwarf rice lines in elite landraces. Scientific Reports 9, 19096.
| Crossref | Google Scholar |
Hussain B, Lucas SJ, Budak H (2018) CRISPR/Cas9 in plants: at play in the genome and at work for crop improvement. Briefings in Functional Genomics 17, 319-328.
| Crossref | Google Scholar | PubMed |
Iftikhar R, Hafeez S, Masood S, Shaheen A, Manzoor M (2023) Biological control of rice insect pest: a critical review. Agrobiological Records 12, 68-75.
| Crossref | Google Scholar |
Islam M, Hasan M, Akter T, Islam S (2024) Agronomic performance and grain quality assessment of small-grain aromatic rice advanced breeding lines in Bangladesh. Agrobiological Records 17, 30-41.
| Crossref | Google Scholar |
Isson TT, Planavsky NJ, Coogan LA, Stewart EM, Ague JJ, Bolton EW, Zhang S, McKenzie NR, Kump LR (2020) Evolution of the global carbon cycle and climate regulation on earth. Global Biogeochemical Cycles 34, e2018GB006061.
| Crossref | Google Scholar |
Jacques C, Salon C, Barnard RL, Vernoud V, Prudent M (2021) Drought stress memory at the plant cycle level: a review. Plants 10, 1873.
| Crossref | Google Scholar | PubMed |
Jaganathan D, Ramasamy K, Sellamuthu G, Jayabalan S, Venkataraman G (2018) CRISPR for crop improvement: an update review. Frontiers in Plant Science 9, 985.
| Crossref | Google Scholar | PubMed |
Jiang W, Zhou H, Bi H, Fromm M, Yang B, Weeks DP (2013a) Demonstration of CRISPR/Cas9/sgRNA-mediated targeted gene modification in Arabidopsis, tobacco, sorghum and rice. Nucleic Acids Research 41, e188.
| Crossref | Google Scholar | PubMed |
Jiang W, Bikard D, Cox D, Zhang F, Marraffini LA (2013b) RNA-guided editing of bacterial genomes using CRISPR-Cas systems. Nature Biotechnology 31, 233-239.
| Crossref | Google Scholar | PubMed |
Jin S, Zong Y, Gao Q, Zhu Z, Wang Y, Qin P, Liang C, Wang D, Qiu J-L, Zhang F, et al. (2019) Cytosine, but not adenine, base editors induce genome-wide off-target mutations in rice. Science 364, 292-295.
| Crossref | Google Scholar | PubMed |
Jung Y-J, Nogoy FM, Lee S-K, Cho Y-G, Kang K-K (2018) Application of ZFN for site directed mutagenesis of rice SSIVa gene. Biotechnology and Bioprocess Engineering 23, 108-115.
| Crossref | Google Scholar |
Kanu AS, Ashraf U, Mo Z, Fuseini I, Mansaray LR, Duan M, Pan S, Tang X (2017) Cadmium uptake and distribution in fragrant rice genotypes and related consequences on yield and grain quality traits. Journal of Chemistry 2017, 1405878.
| Crossref | Google Scholar |
Karmakar A, Taufiqa S, Baig MJ, Molla KA (2022) Increasing disease resistance in host plants through genome editing. Proceedings of the Indian National Science Academy 88, 417-429.
| Crossref | Google Scholar |
Kaur R, Das S, Bansal S, Singh G, Sardar S, Dhar H, Ram H (2021) Heavy metal stress in rice: uptake, transport, signaling, and tolerance mechanisms. Physiologia Plantarum 173, 430-448.
| Crossref | Google Scholar | PubMed |
Kaya H, Mikami M, Endo A, Endo M, Toki S (2016) Highly specific targeted mutagenesis in plants using Staphylococcus aureus Cas9. Scientific Reports 6, 26871.
| Crossref | Google Scholar | PubMed |
Khan I, Khan S, Zhang Y, Zhou J, Akhoundian M, Jan SA (2021) CRISPR-Cas technology based genome editing for modification of salinity stress tolerance responses in rice (Oryza sativa L.). Molecular Biology Reports 48, 3605-3615.
| Crossref | Google Scholar | PubMed |
Khoury CK, Brush S, Costich DE, Curry HA, de Haan S, Engels JMM, Guarino L, Hoban S, Mercer KL, Miller AJ, et al. (2022) Crop genetic erosion: understanding and responding to loss of crop diversity. New Phytologist 233, 84-118.
| Crossref | Google Scholar | PubMed |
Kim Y-A, Moon H, Park C-J (2019) CRISPR/Cas9-targeted mutagenesis of Os8N3 in rice to confer resistance to Xanthomonas oryzae pv. oryzae. Rice 12, 67.
| Crossref | Google Scholar |
Kleinstiver BP, Pattanayak V, Prew MS, Tsai SQ, Nguyen NT, Zheng Z, Joung JK (2016) High-fidelity CRISPR–Cas9 nucleases with no detectable genome-wide off-target effects. Nature 529, 490-495.
| Crossref | Google Scholar | PubMed |
Knorre DG, Vlasov VV (1985) Reactive derivatives of nucleic acids and their components as affinity reagents. Russian Chemical Reviews 54, 836.
| Crossref | Google Scholar |
Koo T, Lee J, Kim J-S (2015) Measuring and reducing off-target activities of programmable nucleases including CRISPR-Cas9. Molecules and Cells 38, 475-481.
| Crossref | Google Scholar | PubMed |
Kuang Y, Li S, Ren B, Yan F, Spetz C, Li X, Zhou X, Zhou H (2020) Base-editing-mediated artificial evolution of OsALS1 in planta to develop novel herbicide-tolerant rice germplasms. Molecular Plant 13, 565-572.
| Crossref | Google Scholar | PubMed |
Kumar M, Prusty MR, Pandey MK, Singh PK, Guo B, Varshney RK (2023) Application of CRISPR-Cas9-mediated gene editing for abiotic stress management in crop plants. Frontiers in Plant Science 14, 1157678.
| Crossref | Google Scholar |
Lamb BM, Mercer AC, Barbas CF, III (2013) Directed evolution of the TALE N-terminal domain for recognition of all 5′ bases. Nucleic Acids Research 41, 9779-9785.
| Crossref | Google Scholar | PubMed |
Lee JK, Jeong E, Lee J, Jung M, Shin E, Kim Y-H, Lee K, Jung I, Kim D, Kim S, et al. (2018) Directed evolution of CRISPR-Cas9 to increase its specificity. Nature Communications 9, 3048.
| Crossref | Google Scholar |
Li J, Meng X, Zong Y, Chen K, Zhang H, Liu J, Li J, Gao C (2016) Gene replacements and insertions in rice by intron targeting using CRISPR–Cas9. Nature Plants 2, 16139.
| Crossref | Google Scholar |
Li S, Zhang X, Wang W, Guo X, Wu Z, Du W, Zhao Y, Xia L (2018) Expanding the scope of CRISPR/Cpf1-mediated genome editing in rice. Molecular Plant 11, 995-998.
| Crossref | Google Scholar | PubMed |
Li Z, Rao MJ, Li J, Wang Y, Chen P, Yu H, Ma C, Wang L (2022) CRISPR/Cas9 mutant rice Ospmei12 involved in growth, cell wall development, and response to phytohormone and heavy metal stress. International Journal of Molecular Sciences 23, 16082.
| Crossref | Google Scholar |
Liang Y, Han Y, Wang C, Jiang C, Xu J-R (2018) Targeted deletion of the USTA and UvSLT2 genes efficiently in Ustilaginoidea virens with the CRISPR-Cas9 system. Frontiers in Plant Science 9, 699.
| Crossref | Google Scholar |
Liao S, Qin X, Luo L, Han Y, Wang X, Usman B, Nawaz G, Zhao N, Liu Y, Li R (2019) CRISPR/Cas9-induced mutagenesis of Semi-Rolled Leaf1,2 confers curled leaf phenotype and drought tolerance by influencing protein expression patterns and ROS scavenging in rice (Oryza sativa L.). Agronomy 9, 728.
| Crossref | Google Scholar |
Lin H, Wang Z, Liu C, Dong Y (2022) Technologies for removing heavy metal from contaminated soils on farmland: a review. Chemosphere 305, 135457.
| Crossref | Google Scholar |
Liu X, Wu D, Shan T, Xu S, Qin R, Li H, Negm M, Wu D, Li J (2020) The trihelix transcription factor OsGTγ-2 is involved adaption to salt stress in rice. Plant Molecular Biology 103, 545-560.
| Crossref | Google Scholar | PubMed |
Liu Z, Ma C, Hou L, Wu X, Wang D, Zhang L, Liu P (2022) Exogenous SA affects rice seed germination under salt stress by regulating Na+/K+ balance and endogenous GAs and ABA homeostasis. International Journal of Molecular Sciences 23, 3293.
| Crossref | Google Scholar | PubMed |
Lou D, Wang H, Liang G, Yu D (2017) OsSAPK2 confers abscisic acid sensitivity and tolerance to drought stress in rice. Frontiers in Plant Science 8, 993.
| Crossref | Google Scholar |
Lu Y, Ye X, Guo R, Huang J, Wang W, Tang J, Tan L, Zhu J-K, Chu C, Qian Y (2017) Genome-wide targeted mutagenesis in rice using the CRISPR/Cas9 system. Molecular Plant 10, 1242-1245.
| Crossref | Google Scholar | PubMed |
Lu H-P, Luo T, Fu H-W, Wang L, Tan Y-Y, Huang J-Z, Wang Q, Ye G-Y, Gatehouse AMR, Lou Y-G, et al. (2018) Resistance of rice to insect pests mediated by suppression of serotonin biosynthesis. Nature Plants 4, 338-344.
| Crossref | Google Scholar | PubMed |
Ma X, Zhang Q, Zhu Q, Liu W, Chen Y, Qiu R, Wang B, Yang Z, Li H, Lin Y, et al. (2015) A robust CRISPR/Cas9 system for convenient, high-efficiency multiplex genome editing in monocot and dicot plants. Molecular Plant 8, 1274-1284.
| Crossref | Google Scholar | PubMed |
Ma J, Chen J, Wang M, Ren Y, Wang S, Lei C, Cheng Z, Sodmergen (2018) Disruption of OsSEC3A increases the content of salicylic acid and induces plant defense responses in rice. Journal of Experimental Botany 69, 1051-1064.
| Crossref | Google Scholar | PubMed |
MacNeil A, Glaziou P, Sismanidis C, Date A, Maloney S, Floyd K (2020) Global epidemiology of tuberculosis and progress toward meeting global targets—worldwide, 2018. MMWR. Morbidity and Mortality Weekly Report 69, 281-285.
| Crossref | Google Scholar | PubMed |
Macovei A, Sevilla NR, Cantos C, Jonson GB, Slamet-Loedin I, Čermák T, Voytas DF, Choi I-R, Chadha-Mohanty P (2018) Novel alleles of rice eIF4G generated by CRISPR/Cas9-targeted mutagenesis confer resistance to Rice tungro spherical virus. Plant Biotechnology Journal 16, 1918-1927.
| Crossref | Google Scholar | PubMed |
Malhotra K, Subramaniyan M, Rawat K, Kalamuddin M, Qureshi MI, Malhotra P, Mohmmed A, Cornish K, Daniell H, Kumar S (2016) Compartmentalized metabolic engineering for artemisinin biosynthesis and effective malaria treatment by oral delivery of plant cells. Molecular Plant 9, 1464-1477.
| Crossref | Google Scholar | PubMed |
Mali P, Yang L, Esvelt KM, Aach J, Guell M, DiCarlo JE, Norville JE, Church GM (2013) RNA-guided human genome engineering via Cas9. Science 339, 823-826.
| Crossref | Google Scholar | PubMed |
Mehta D, Stürchler A, Anjanappa RB, Zaidi SS-e-A, Hirsch-Hoffmann M, Gruissem W, Vanderschuren H (2019) Linking CRISPR-Cas9 interference in cassava to the evolution of editing-resistant geminiviruses. Genome Biology 20, 80.
| Crossref | Google Scholar |
Meng X, Yu H, Zhang Y, Zhuang F, Song X, Gao S, Gao C, Li J (2017) Construction of a genome-wide mutant library in rice using CRISPR/Cas9. Molecular Plant 10, 1238-1241.
| Crossref | Google Scholar | PubMed |
Miao J, Guo D, Zhang J, Huang Q, Qin G, Zhang X, Wan J, Gu H, Qu L-J (2013) Targeted mutagenesis in rice using CRISPR-Cas system. Cell Research 23, 1233-1236.
| Crossref | Google Scholar | PubMed |
Miao C, Xiao L, Hua K, Zou C, Zhao Y, Bressan RA, Zhu J-K (2018) Mutations in a subfamily of abscisic acid receptor genes promote rice growth and productivity. Proceedings of the National Academy of Sciences 115, 6058-6063.
| Crossref | Google Scholar |
Minkenberg B, Xie K, Yang Y (2017) Discovery of rice essential genes by characterizing a CRISPR-edited mutation of closely related rice MAP kinase genes. The Plant Journal 89, 636-648.
| Crossref | Google Scholar | PubMed |
Mishra R, Zhao K (2018) Genome editing technologies and their applications in crop improvement. Plant Biotechnology Reports 12, 57-68.
| Crossref | Google Scholar |
Miyaoka Y, Berman JR, Cooper SB, Mayerl SJ, Chan AH, Zhang B, Karlin-Neumann GA, Conklin BR (2016) Systematic quantification of HDR and NHEJ reveals effects of locus, nuclease, and cell type on genome-editing. Scientific Reports 6, 23549.
| Crossref | Google Scholar | PubMed |
Mohamed HI, Khan A, Basit A (2024) CRISPR-Cas9 system mediated genome editing technology: an ultimate tool to enhance abiotic stress in crop plants. Journal of Soil Science and Plant Nutrition 24, 1799-1822.
| Crossref | Google Scholar |
Moon SB, Kim DY, Ko J-H, Kim J-S, Kim Y-S (2019) Improving CRISPR genome editing by engineering guide RNAs. Trends in Biotechnology 37, 870-881.
| Crossref | Google Scholar | PubMed |
Morales F, Ancín M, Fakhet D, González-Torralba J, Gámez AL, Seminario A, Soba D, Ben Mariem S, Garriga M, Aranjuelo I (2020) Photosynthetic metabolism under stressful growth conditions as a bases for crop breeding and yield improvement. Plants 9, 88.
| Crossref | Google Scholar | PubMed |
Moustafa-Farag M, Almoneafy A, Mahmoud A, Elkelish A, Arnao MB, Li L, Ai S (2020) Melatonin and its protective role against biotic stress impacts on plants. Biomolecules 10, 54.
| Crossref | Google Scholar | PubMed |
Muehe EM, Wang T, Kerl CF, Planer-Friedrich B, Fendorf S (2019) Rice production threatened by coupled stresses of climate and soil arsenic. Nature Communications 10, 4985.
| Crossref | Google Scholar | PubMed |
Mushtaq M, Mukhtar S, Sakina A, Dar AA, Bhat R, Deshmukh R, Molla K, Kundoo AA, Dar MS (2020) Tweaking genome-editing approaches for virus interference in crop plants. Plant Physiology and Biochemistry 147, 242-250.
| Crossref | Google Scholar | PubMed |
Nandy S, Srivastava V (2012) Marker-free site-specific gene integration in rice based on the use of two recombination systems. Plant Biotechnology Journal 10, 904-912.
| Crossref | Google Scholar | PubMed |
Nawaz G, Usman B, Peng H, Zhao N, Yuan R, Liu Y, Li R (2020) Knockout of Pi21 by CRISPR/Cas9 and iTRAQ-based proteomic analysis of mutants revealed new insights into M. oryzae resistance in elite rice line. Genes 11, 735.
| Crossref | Google Scholar | PubMed |
Nawaz MS, Sami SA, Bano M, Khan MRQ, Anwar Z, Ijaz A, Ahmed T (2023) Impact of salt stress on cotton. International Journal of Agriculture and Biosciences 12, 98-103.
| Crossref | Google Scholar |
Nazir R, Mandal S, Mitra S, Ghorai M, Das N, Jha NK, Majumder M, Pandey DK, Dey A (2022) Clustered regularly interspaced short palindromic repeats (CRISPR)/CRISPR-associated genome-editing toolkit to enhance salt stress tolerance in rice and wheat. Physiologia Plantarum 174, e13642.
| Crossref | Google Scholar | PubMed |
Nieves-Cordones M, Mohamed S, Tanoi K, Kobayashi NI, Takagi K, Vernet A, Guiderdoni E, Périn C, Sentenac H, Véry A-A (2017) Production of low-Cs+ rice plants by inactivation of the K+ transporter OsHAK1 with the CRISPR-Cas system. The Plant Journal 92, 43-56.
| Crossref | Google Scholar | PubMed |
Noman MU, Azhar S (2023) Metabolomics, a potential way to improve abiotic stresses tolerance in cereal crops. International Journal of Agriculture and Biosciences 12, 47-55.
| Crossref | Google Scholar |
Ogata T, Ishizaki T, Fujita M, Fujita Y (2020) CRISPR/Cas9-targeted mutagenesis of OsERA1 confers enhanced responses to abscisic acid and drought stress and increased primary root growth under nonstressed conditions in rice. PLoS ONE 15, e0243376.
| Crossref | Google Scholar | PubMed |
Oliva R, Ji C, Atienza-Grande G, Huguet-Tapia JC, Perez-Quintero A, Li T, Eom J-S, Li C, Nguyen H, Liu B, et al. (2019) Broad-spectrum resistance to bacterial blight in rice using genome editing. Nature Biotechnology 37, 1344-1350.
| Crossref | Google Scholar | PubMed |
Özyiğit İİ, Abakirova A, Hocaoğlu-Özyiğit A, Kurmanbekova G, Chekirov K, Yalcin B, Yalçin İE (2021) Cadmium stress in barley seedlings: accumulation, growth, anatomy and physiology. International Journal of Life Sciences and Biotechnology 4, 204-223.
| Crossref | Google Scholar |
Panhwar QA, Naher UA, Radziah O, Shamshuddin J, Razi IM (2015) Eliminating aluminum toxicity in an acid sulfate soil for rice cultivation using plant growth promoting bacteria. Molecules 20, 3628-3646.
| Crossref | Google Scholar | PubMed |
Panth M, Hassler SC, Baysal-Gurel F (2020) Methods for management of soilborne diseases in crop production. Agriculture 10, 16.
| Crossref | Google Scholar |
Papworth M, Kolasinska P, Minczuk M (2006) Designer zinc-finger proteins and their applications. Gene 366, 27-38.
| Crossref | Google Scholar | PubMed |
Pérez-Méndez N, Miguel-Rojas C, Jimenez-Berni JA, Gomez-Candon D, Pérez-de-Luque A, Fereres E, Catala-Forner M, Villegas D, Sillero JC (2022) Plant breeding and management strategies to minimize the impact of water scarcity and biotic stress in cereal crops under Mediterranean conditions. Agronomy 12, 75.
| Crossref | Google Scholar |
Rahaman MM, Shehab MK (2019) Water consumption, land use and production patterns of rice, wheat and potato in South Asia during 1988–2012. Sustainable Water Resources Management 5, 1677-1694.
| Crossref | Google Scholar |
Rauf S, Shahid A, Faizan M, Khalid MN, Amjad I (2024) CRISPER/CAS: a potential tool for genomes editing. Agrobiological Records 15, 13-23.
| Crossref | Google Scholar |
Raza A, Hairs M, Riaz A, Rafiq R, Basharat M, Shaheen M, Goshi S, Asghar S, Khan A, Rehman ZU (2022) Trending killers of plants; an overview of the most prevailing pathogenic fungi. The Journal of Microbiology and Molecular Genetics 3, 97-147.
| Crossref | Google Scholar |
Rehman I, Shaukat F, Anwar Z, Ijaz A, Nadeem R (2022) Applications of Crispr/Cas system in plants. International Journal of Agriculture and Biosciences 11, 231-237.
| Crossref | Google Scholar |
Riaz A, Huimin W, Zhenhua Z, Zegeye WA, Yanhui L, Hong W, Pao X, Zequn P, Xihong S, Shihua C, et al. (2021) Development of chromosome segment substitution lines and genetic dissection of grain size related locus in rice. Rice Science 28, 322-324.
| Crossref | Google Scholar |
Richard B, Qi A, Fitt BDL (2022) Control of crop diseases through Integrated Crop Management to deliver climate-smart farming systems for low- and high-input crop production. Plant Pathology 71, 187-206.
| Crossref | Google Scholar |
Romero FM, Gatica-Arias A (2019) CRISPR/Cas9: development and application in rice breeding. Rice Science 26, 265-281.
| Crossref | Google Scholar |
Rushton PJ, Reinstädler A, Lipka V, Lippok B, Somssich IE (2002) Synthetic plant promoters containing defined regulatory elements provide novel insights into pathogen- and wound-induced signaling. The Plant Cell 14, 749-762.
| Crossref | Google Scholar | PubMed |
Samal P, Babu SC, Mondal B (2021) The global rice scenario towards 2050: results for six continents. In ‘2021 Conference’, 17–31 August 2021, Virtual 315085, International Association of Agricultural Economists. 10.22004/ag.econ.315085
Santosh Kumar VV, Verma RK, Yadav SK, Yadav P, Watts A, Rao MV, Chinnusamy V (2020) CRISPR-Cas9 mediated genome editing of drought and salt tolerance (OsDST) gene in indica mega rice cultivar MTU1010. Physiology and Molecular Biology of Plants 26, 1099-1110.
| Crossref | Google Scholar | PubMed |
Schausberger P (2018) Herbivore-associated bacteria as potential mediators and modifiers of induced plant defense against spider mites and thrips. Frontiers in Plant Science 9, 1107.
| Crossref | Google Scholar | PubMed |
Schloss PD, Handelsman J (2004) Status of the microbial census. Microbiology and Molecular Biology Reviews 68, 686-691.
| Crossref | Google Scholar |
Selvaraj GK, Wilson JJ, Kanagaraj N, Subashini E, Thangavel S (2022) Enhanced antifungal activity of Piper betle against candidiasis infection causing Candida Albicans and In silico analysis with its virulent protein. Biomedical and Biotechnology Research Journal (BBRJ) 6, 73-80.
| Crossref | Google Scholar |
Shan Q, Wang Y, Li J, Zhang Y, Chen K, Liang Z, Zhang K, Liu J, Xi JJ, Qiu J-L, et al. (2013) Targeted genome modification of crop plants using a CRISPR-Cas system. Nature Biotechnology 31, 686-688.
| Crossref | Google Scholar | PubMed |
Sharma LK, McCray JM, Morgan KJE (2022) Plant essential nutrients and their role: SS-AGR-463/AG462, 5/2022. EDIS 2022,.
| Crossref | Google Scholar |
Shen C, Que Z, Xia Y, Tang N, Li D, He R, Cao M (2017) Knock out of the annexin gene OsAnn3 via CRISPR/Cas9-mediated genome editing decreased cold tolerance in rice. Journal of Plant Biology 60, 539-547.
| Crossref | Google Scholar |
Shimatani Z, Kashojiya S, Takayama M, Terada R, Arazoe T, Ishii H, Teramura H, Yamamoto T, Komatsu H, Miura K, et al. (2017) Targeted base editing in rice and tomato using a CRISPR-Cas9 cytidine deaminase fusion. Nature Biotechnology 35, 441-443.
| Crossref | Google Scholar | PubMed |
Siddiqui MN, Mostofa MG, Akter MM, Srivastava AK, Sayed MA, Hasan MS, Tran L-SP (2017) Impact of salt-induced toxicity on growth and yield-potential of local wheat cultivars: oxidative stress and ion toxicity are among the major determinants of salt-tolerant capacity. Chemosphere 187, 385-394.
| Crossref | Google Scholar | PubMed |
Singh A (2022) Soil salinity: a global threat to sustainable development. Soil Use and Management 38, 39-67.
| Crossref | Google Scholar |
Singh G, Gupta MK, Chaurasiya S, Sharma VS, Pimenov DY (2021) Rice straw burning: a review on its global prevalence and the sustainable alternatives for its effective mitigation. Environmental Science and Pollution Research 28, 32125-32155.
| Crossref | Google Scholar |
Singhal RK, Jatav HS, Aftab T, Pandey S, Mishra UN, Chauhan J, Chand S, Indu , Saha D, Dadarwal BK, et al. (2021) Roles of nitric oxide in conferring multiple abiotic stress tolerance in plants and crosstalk with other plant growth regulators. Journal of Plant Growth Regulation 40, 2303-2328.
| Crossref | Google Scholar |
Slamet-Loedin IH, Johnson-Beebout SE, Impa S, Tsakirpaloglou N (2015) Enriching rice with Zn and Fe while minimizing Cd risk. Frontiers in Plant Science 6, 121.
| Crossref | Google Scholar | PubMed |
Slaymaker IM, Gao L, Zetsche B, Scott DA, Yan WX, Zhang F (2016) Rationally engineered Cas9 nucleases with improved specificity. Science 351, 84-88.
| Crossref | Google Scholar | PubMed |
Songmei L, Jie J, Yang L, Jun M, Shouling X, Yuanyuan T, Youfa L, Qingyao S, Jianzhong H (2019) Characterization and evaluation of OsLCT1 and OsNramp5 mutants generated through CRISPR/Cas9-mediated mutagenesis for breeding low Cd rice. Rice Science 26, 88-97.
| Crossref | Google Scholar |
Srivastava V, Underwood JL, Zhao S (2017) Dual-targeting by CRISPR/Cas9 for precise excision of transgenes from rice genome. Plant Cell, Tissue and Organ Culture (PCTOC) 129, 153-160.
| Crossref | Google Scholar |
Tang X, Lowder LG, Zhang T, Malzahn AA, Zheng X, Voytas DF, Zhong Z, Chen Y, Ren Q, Li Q, et al. (2017a) A CRISPR–Cpf1 system for efficient genome editing and transcriptional repression in plants. Nature Plants 3, 17018.
| Crossref | Google Scholar |
Tang L, Mao B, Li Y, Lv Q, Zhang L, Chen C, He H, Wang W, Zeng X, Shao Y, et al. (2017b) Knockout of OsNramp5 using the CRISPR/Cas9 system produces low Cd-accumulating indica rice without compromising yield. Scientific Reports 7, 14438.
| Crossref | Google Scholar |
Tang L, Dong J, Qu M, Lv Q, Zhang L, Peng C, Hu Y, Li Y, Ji Z, Mao B, et al. (2022) Knockout of OsNRAMP5 enhances rice tolerance to cadmium toxicity in response to varying external cadmium concentrations via distinct mechanisms. Science of The Total Environment 832, 155006.
| Crossref | Google Scholar | PubMed |
Teklić T, Parađiković N, Špoljarević M, Zeljković S, Lončarić Z, Lisjak M (2021) Linking abiotic stress, plant metabolites, biostimulants and functional food. Annals of Applied Biology 178, 169-191.
| Crossref | Google Scholar |
Tseng M-C, Roel Á, Macedo I, Marella M, Terra J, Zorrilla G, Pittelkow CM (2021) Field-level factors for closing yield gaps in high-yielding rice systems of Uruguay. Field Crops Research 264, 108097.
| Crossref | Google Scholar |
ul Haq S, Khan A, Ali M, Khattak AM, Gai W-X, Zhang H-X, Wei A-M, Gong Z-H (2019) Heat shock proteins: dynamic biomolecules to counter plant biotic and abiotic stresses. International Journal of Molecular Sciences 20, 5321.
| Crossref | Google Scholar |
Usman B, Nawaz G, Zhao N, Liao S, Liu Y, Li R (2020) Precise editing of the OsPYL9 gene by RNA-guided Cas9 nuclease confers enhanced drought tolerance and grain yield in rice (Oryza sativa L.) by regulating circadian rhythm and abiotic stress responsive proteins. International Journal of Molecular Sciences 21, 7854.
| Crossref | Google Scholar | PubMed |
Usman B, Nawaz G, Zhao N, Liao S, Qin B, Liu F, Liu Y, Li R (2021) Programmed editing of rice (Oryza sativa L.) OsSPL16 gene using CRISPR/Cas9 improves grain yield by modulating the expression of pyruvate enzymes and cell cycle proteins. International Journal of Molecular Sciences 22, 249.
| Crossref | Google Scholar | PubMed |
van Beljouw SPB, Sanders J, Rodríguez-Molina A, Brouns SJJ (2023) RNA-targeting CRISPR–Cas systems. Nature Reviews Microbiology 21, 21-34.
| Crossref | Google Scholar | PubMed |
van Schie CCN, Takken FLW (2014) Susceptibility genes 101: how to be a good host. Annual Review of Phytopathology 52, 551-581.
| Crossref | Google Scholar | PubMed |
Wang F, Wang C, Liu P, Lei C, Hao W, Gao Y, Liu Y-G, Zhao K (2016a) Enhanced rice blast resistance by CRISPR/Cas9-targeted mutagenesis of the ERF transcription factor gene OsERF922. PLoS ONE 11, e0154027.
| Crossref | Google Scholar | PubMed |
Wang H, La Russa M, Qi LS (2016b) CRISPR/Cas9 in genome editing and beyond. Annual Review of Biochemistry 85, 227-264.
| Crossref | Google Scholar | PubMed |
Wang M, Mao Y, Lu Y, Tao X, Zhu J-K (2017) Multiplex gene editing in rice using the CRISPR-Cpf1 system. Molecular Plant 10, 1011-1013.
| Crossref | Google Scholar | PubMed |
Wang X-B, Azarbad H, Leclerc L, Dozois J, Mukula E, Yergeau É (2022a) A drying-Rewetting cycle imposes more important shifts on soil microbial communities than does reduced precipitation. mSystems 7, e00247–22.
| Crossref | Google Scholar | PubMed |
Wang Y, Zafar N, Ali Q, Manghwar H, Wang G, Yu L, Ding X, Ding F, Hong N, Wang G, et al. (2022b) CRISPR/Cas genome editing technologies for plant improvement against biotic and abiotic stresses: advances, limitations, and future perspectives. Cells 11, 3928.
| Crossref | Google Scholar | PubMed |
Wei J, Liu X, Li C, Yang Y, Song C, Chen Y, Ciren Q, Jiang C, Li Q (2024) Identification and characterization of Hibiscus mutabilis varieties resistant to Bemisia tabaci and their resistance mechanisms. Insects 15, 454.
| Crossref | Google Scholar | PubMed |
Westra ER, Buckling A, Fineran PC (2014) CRISPR–Cas systems: beyond adaptive immunity. Nature Reviews Microbiology 12, 317-326.
| Crossref | Google Scholar | PubMed |
Wienert B, Wyman SK, Richardson CD, Yeh CD, Akcakaya P, Porritt MJ, Morlock M, Vu JT, Kazane KR, Watry HL, et al. (2019) Unbiased detection of CRISPR off-targets in vivo using DISCOVER-Seq. Science 364, 286-289.
| Crossref | Google Scholar | PubMed |
Xie K, Yang Y (2013) RNA-guided genome editing in plants using a CRISPR–Cas system. Molecular Plant 6, 1975-1983.
| Crossref | Google Scholar | PubMed |
Xu J, Yang L, Wang Z, Dong G, Huang J, Wang Y (2006) Toxicity of copper on rice growth and accumulation of copper in rice grain in copper contaminated soil. Chemosphere 62, 602-607.
| Crossref | Google Scholar | PubMed |
Xu R, Yang Y, Qin R, Li H, Qiu C, Li L, Wei P, Yang J (2016) Rapid improvement of grain weight via highly efficient CRISPR/Cas9-mediated multiplex genome editing in rice. Journal of Genetics and Genomics= Yi chuan xue bao 43, 529-532.
| Crossref | Google Scholar | PubMed |
Xu Z, Xu X, Gong Q, Li Z, Li Y, Wang S, Yang Y, Ma W, Liu L, Zhu B, et al. (2019) Engineering broad-spectrum bacterial blight resistance by simultaneously disrupting variable TALE-binding elements of multiple susceptibility genes in rice. Molecular Plant 12, 1434-1446.
| Crossref | Google Scholar | PubMed |
Yang Y, Guo Y (2018) Elucidating the molecular mechanisms mediating plant salt-stress responses. New Phytologist 217, 523-539.
| Crossref | Google Scholar | PubMed |
Yang J, Zhang Y, Yuan P, Zhou Y, Cai C, Ren Q, Wen D, Chu C, Qi H, Wei W (2014) Complete decoding of TAL effectors for DNA recognition. Cell Research 24, 628-631.
| Crossref | Google Scholar | PubMed |
Younis A, Ramzan F, Ramzan Y, Zulfiqar F, Ahsan M, Lim KB (2020) Molecular markers improve abiotic stress tolerance in crops: a review. Plants 9, 1374.
| Crossref | Google Scholar | PubMed |
Yu H-Y, Liu C, Zhu J, Li F, Deng D-M, Wang Q, Liu C (2016) Cadmium availability in rice paddy fields from a mining area: the effects of soil properties highlighting iron fractions and pH value. Environmental Pollution 209, 38-45.
| Crossref | Google Scholar | PubMed |
Yu S, Ali J, Zhang C, Li Z, Zhang Q (2020) Genomic breeding of green super rice varieties and their deployment in Asia and Africa. Theoretical and Applied Genetics 133, 1427-1442.
| Crossref | Google Scholar | PubMed |
Yue E, Cao H, Liu B (2020) OsmiR535, a potential genetic editing target for drought and salinity stress tolerance in Oryza sativa. Plants 9, 1337.
| Crossref | Google Scholar | PubMed |
Zeng Y, Wen J, Zhao W, Wang Q, Huang W (2020) Rational improvement of rice yield and cold tolerance by editing the three genes OsPIN5b, GS3, and OsMYB30 with the CRISPR–Cas9 system. Frontiers in Plant Science 10, 1663.
| Crossref | Google Scholar | PubMed |
Zhang F, Maeder ML, Unger-Wallace E, Hoshaw JP, Reyon D, Christian M, Li X, Pierick CJ, Dobbs D, Peterson T, et al. (2010) High frequency targeted mutagenesis in Arabidopsis thaliana using zinc finger nucleases. Proceedings of the National Academy of Sciences 107, 12028-12033.
| Crossref | Google Scholar |
Zhang H-X, Zhang Y, Yin H (2019a) Genome editing with mRNA encoding ZFN, TALEN, and Cas9. Molecular Therapy 27, 735-746.
| Crossref | Google Scholar | PubMed |
Zhang A, Liu Y, Wang F, Li T, Chen Z, Kong D, Bi J, Zhang F, Luo X, Wang J, et al. (2019b) Enhanced rice salinity tolerance via CRISPR/Cas9-targeted mutagenesis of the OsRR22 gene. Molecular Breeding 39, 47.
| Crossref | Google Scholar |
Zhang R, Chen S, Meng X, Chai Z, Wang D, Yuan Y, Chen K, Jiang L, Li J, Gao C (2021) Generating broad-spectrum tolerance to ALS-inhibiting herbicides in rice by base editing. Science China Life Sciences 64, 1624-1633.
| Crossref | Google Scholar |
Zhao YN, Wang MQ, Li C, Cao HW, Rono JK, Yang ZM (2022) The metallochaperone OsHIPP56 gene is required for cadmium detoxification in rice crops. Environmental and Experimental Botany 193, 104680.
| Crossref | Google Scholar |
Zhong Z, Zhang Y, You Q, Tang X, Ren Q, Liu S, Yang L, Wang Y, Liu X, Liu B, et al. (2018) Plant genome editing using FnCpf1 and LbCpf1 nucleases at redefined and altered PAM sites. Molecular Plant 11, 999-1002.
| Crossref | Google Scholar | PubMed |
Zhou J, Peng Z, Long J, Sosso D, Liu B, Eom J-S, Huang S, Liu S, Vera Cruz C, Frommer WB, et al. (2015) Gene targeting by the TAL effector PthXo2 reveals cryptic resistance gene for bacterial blight of rice. The Plant Journal 82, 632-643.
| Crossref | Google Scholar | PubMed |
Zhou Y, Xu S, Jiang N, Zhao X, Bai Z, Liu J, Yao W, Tang Q, Xiao G, Lv C, et al. (2022) Engineering of rice varieties with enhanced resistances to both blast and bacterial blight diseases via CRISPR/Cas9. Plant Biotechnology Journal 20, 876-885.
| Crossref | Google Scholar | PubMed |
Zihe LI, Riaz A, Yingxin Z, Anis GB, Aike Z, Liyong C, Shihua C (2019) Quantitative trait loci mapping for rice yield-related traits using chromosomal segment substitution lines. Rice Science 26, 261-264.
| Crossref | Google Scholar |