Comprehensive analysis of potato (Solanum tuberosum) PYL genes highlights their role in stress responses
Shareef Gul A # , Hameed Gul A # , Muhammad Shahzad B , Ikram Ullah C , Ali Shahzad

A
B
C
D
E
F
Handling Editor: Inzamam Haq
Abstract
Abscisic acid (ABA) regulates plant development, seed germination, and stress responses. The PYR1-like (PYL) proteins are essential for ABA signalling. However, the evolution and expression of PYL genes in potato (Solanum tuberosum) remain poorly understood. Here, we analysed and identified 17 PYL genes in the potato genome, which were categorised into three groups based on phylogenetic analysis. These genes are distributed across nine chromosomes with predicted proteins subcellar localisation primarily in the cytoplasm. These StPYLs revealed conserved exon structures and domains among the groups. Promoter region analysis indicated hormone and stress-related elements in all StPYLs. Protein–protein interactions and microRNA networks predicted that the interactions of StPYLs are crucial components of ABA signalling, underlining their pivotal role in stress management and growth regulation in potato. Expression profiling across different tissues and under various stresses revealed their varied expression pattern. Further, we validated the expression pattern of selected StPYLs through reverse transcription quantitative PCR under drought, salt, and Phytophthora infestans stresses. This revealed consistent upregulation of StPYL6 in these stresses, while StPYL11 exhibited significant downregulation over time. Other genes showed downregulation under drought and salt stresses while upregulation under P. infestans. Overall, our results suggested the potential role of PYL genes in abiotic and biotic stresses.
Keywords: ABA signalling, biotic and abiotic stresses, conserved domains, different tissues, expression profile, genome-wide analysis, PYL gene family, Solanum tuberosum L.
Introduction
Potato (Solanum tuberosum) is an important agricultural and annual Solanaceae crop that is cultivated worldwide (Lee et al. 2016; ain-Ali et al. 2021; del Mar Martínez-Prada et al. 2021). Potato ranks as the fourth-most widely grown crop for food, following maize (Zea mays), rice (Oryza sativa), and wheat (Triticum aestivum) (Zhang et al. 2017; Bonthala and Stich 2024), and also the top horticultural crops (Jennings et al. 2020). Potatoes are not only utilised for vegetative propagation but also serve as a valuable source of carbohydrates, protein, antioxidants, and vitamins (Denham et al. 2020). Given their nutritional and agricultural importance, the continued production of potatoes is of great importance. However, due to biotic and abiotic factors, potato production is under severe danger, and these stresses are increasing due to sudden climatic changes (Shahzad et al. 2021; Zaidi et al. 2022; Shahzad et al. 2023a; Jing et al. 2024). These stresses restrict potato growth and formation of tubers (Zhang et al. 2022a; Siano et al. 2024). Ultimately, both biotic and abiotic stresses have a substantial impact on potato farming and can cause poorer yields and crop failure (Tiwari et al. 2022).
Plants have evolved a range of complex signalling mechanisms in response to stress (Singh et al. 2019). Abscisic acid (ABA) signalling is one of these mechanisms, and it is essential for many aspects of plant growth and development, including cell elongation and division, seed desiccation tolerance and dormancy, root growth, leaf senescence, fruit ripening, and adaptation to biotic and abiotic stresses (Di et al. 2018; Chen et al. 2020a; Wang et al. 2022). As a major stress hormone, ABA controls several stress reactions in plants. To improve osmotic adjustment under salinity stress, it stimulates that the accumulation of compatible solutes, modulates stomatal closure, and triggers the expression of stress-responsive genes (Muhammad Aslam et al. 2022). Additionally, ABA coordinates intricate stress responses and fine-tunes plant adaption methods by interacting with other signalling molecules like ethylene and jasmonic acid (JA) (Lei et al. 2021; Zhang et al. 2022b). The development of stress-tolerant crops is made possible by understanding the complex signalling networks involving ABA and its interactions with other hormones (Di et al. 2018; Singh et al. 2019; Lamers et al. 2020; Wang et al. 2022; Huang et al. 2024). Along with other plant hormones, ABA control how plants develop and respond to environmental cues (Mohammadi et al. 2021). Key players in this process are PYL (pyrabactin resistance 1-like) proteins, which belong to the START (star-related lipid-transfer) superfamily. These proteins function as ABA receptors by directly interacting with ABA in hydrophobic ligand cavities (Clark 2020; Zhang et al. 2021; Rani et al. 2024). PYLs together with PYR1 (pyrabactin resistance 1) are regulatory components of ABA receptors (RCARs) that have the START domain and play a significant role in ABA signalling pathways (Park et al. 2009; Dittrich et al. 2019). This regulatory network is essential for the plant’s ability to adapt to varying environmental conditions.
In recent years, advances in genome assembly of various plant species have significantly facilitated the identification of gene families and their structural characterisations. Numerous studies have now identified the PYL gene family in various plant species, including Arabidopsis thaliana, T. aestivum, O. sativa, Z. mays, Solanum lycopersicum, Camellia sinensis, Prunus avium, Hordeum vulgare, Helianthus annuus, Punica granatum, and Acer palmatum (Kim et al. 2014; Soma et al. 2017; Mega et al. 2019; Wang et al. 2022, 2024; An et al. 2023; Shahzad et al. 2023b; Zhou et al. 2023; Yin et al. 2024; Zhu et al. 2024). A total of 14 PYL proteins from Arabidopsis have been discovered and divided into three subfamilies based on their highly conserved START domains (Park et al. 2009; Lei et al. 2021). While certain AtPYLs form ABA-dependent homodimers to interact with phosphatase 2C (PP2C), others engage with PP2C independently (Hao et al. 2011; Zhang et al. 2021). Orthologous genes of PYLs have also been identified in other crops, including grape (Vitis vinifera), cotton (Gossypium hirsutum), tomato (Solanum lycopersicum), rice, and maize (Zhang et al. 2022b). Various studies revealed the role of AtPYLs in plant growth and various abiotic stresses. For instance, AtPYL8 modulates lateral root growth during stress recovery, and also is essential for leaf senescence, while AtPYL4 greatly enhances drought tolerance (Zhao et al. 2016; Dittrich et al. 2019; Zhang et al. 2022b). Various downstream developmental and stress response processes are regulated by the interaction between AtPYL6 and MYC2, an essential transcription factor in the jasmonic acid response (Ghorbel et al. 2021). Additionally, AtPYL11 and AtPYL12 have been shown to control ABA-mediated seed germination (Zhao et al. 2020). The resistance to drought, salt, and cold in rice is increased by overexpression of OsPYL5 and OsPYL10, while the overexpression of ZmPYL8, ZmPYL9, and ZmPYL12 improves maize’s tolerance to low temperature (Kim et al. 2014; Lei et al. 2021). Overall, the expression of PYLs is induced by a number of abiotic stressors, including cold, drought, and salinity (Cui et al. 2020; Narsing Rao et al. 2022), rather than just serving as ABA receptors, which highlights the crucial roles played by the PYL gene family in plant development and stress responses (Singh et al. 2019). In this perspective, identification and examination the roles of PYL genes in plants is crucial for enhancing crop stress tolerance and advancing our understanding of ABA signal transduction. However, still no comprehensive study conducted to identify and characterise the PYL genes in potato genome.
In this study, we used the genome-search approach and identified the 17 StPYLs in potato. We conducted comprehensive analysis of 17 StPYLs, and investigated their phylogenetic relationships, chromosome distribution, gene structure, sequence characteristics, and gene duplications. Moreover, we assessed the expression pattern of StPYLs in various tissues and under various biotic and abiotic stress conditions. Finally, we validated the expression level of selected StPYLs under drought, salt, and Phytophthora infestans stresses. Overall, our study provides an in-depth examination of the potato PYL gene family, offering valuable data and a novel perspective for molecular breeding research.
Materials and methods
Genome-wide identification of PYL gene family in potato
We obtained the protein sequences of 14 AtPYLs from The Arabidopsis Information Resource (TAIR) website (https://www.arabidopsis.org). We used the Spud DB Potato Genomics Resources website (http://spuddb.uga.edu) to obtain the genomic and protein datasets of potato (Solanum tuberosum L.). For the four other plant species (Oryza sativa L., Capsicum annum L., Solanum lycopersicum L., and Zea mays L.), we obtained the data from the ensemble plants website (http://plants.ensembl.org/index.html). Finally, to search the PYLs in protein datasets, 14 AtPYL proteins were used as query with local BLASTP search (Altschul et al. 1997) using threshold e-value 1e−5 with and 50% minimal alignment coverage (Shahzad et al. 2023b). Moreover, in order to verify the presence of Polyketide_cyc2 domain in the obtained candidate proteins, firstly we used the Pfamscan website (https://www.ebi.ac.uk/Tools/pfa/pfamscan/; see Supplementary Table S1) then both the START and NCBI CD-search tools used to further verify the domain presence (Letunic et al. 2021; Wang et al. 2023).
Prediction of physicochemical characteristics and subcellular localisation
To examine the physicochemical characteristics of StPYL proteins, we used the ExPasy server (https://web.expasy.org/protparam/). We estimated the number of amino acids, protein’s molecular weights, theoretical isoelectric points, protein instability index, and the overall hydropathicity (GRAVY). Additionally, we used the Plant-mPLoc website (http://www.csbio.sjtu.edu.cn/bioinf/plant-multi/) to predict the subcellular localisation of the StPYL proteins (Chou and Shen 2010).
Phylogenetic analysis, gene structure, and conserved motif analysis
The protein sequences of PYLs from Arabidopsis thaliana L., S. tubersoum, S. lycopersicum, C. annuum, O. sativa, and Z. mays were used for phylogenetic analysis to elaborate the evolutionary relationship of potato’s PYL gene with other species. To construct the phylogenetic tree, we used the MEGA X software (Kumar et al. 2018). Initially, the protein sequences were aligned using ClusterW with default parameters and then phylogenetic tree was created by using neighbour-joining (NJ) method (Shahzad et al. 2023b) with the following parameters: bootstrap values with 1000 replicates, using the Jones-Taylor-Thornton (JTT) + invariant sites (I) + Gamma (G) substitution model (Kumar et al. 2018). Finally, the resulted tree was visualised using the online tool Interaction Tree of Life (iTOL) (Letunic and Bork 2021).
Gene structure of StPYLs were examined by the Gene Structure Display Server 2.0 (GSDS 2.0; https://gsds.gao-lab.org/). The exons and introns of all StPYLs were identified and then visualised by the TBtools program. We used the Multiple Expectation Maximisation for Motif Elucidation (MEME, ver. 5.5.3) online tool with default settings to find conserved motifs in the StPYLs.
Chromosomal location and synteny analysis
The chromosomal locations of all StPYLs were determined using the positional information from the annotation of the potato genome sequence. Using the TBtools mapping of the PYL genes to the relevant chromosomes, we were able to see where the PYL genes were located within the genome. We investigated the gene duplication within the PYL gene family using TBtools with default parameters. They were located based on the precise positions of the tandemly duplicated genes on each chromosome. To assess the level of selective pressure on the dataset, we computed the synonymous (Ks) and non-synonymous (Ka) replacement rates as well as the Ka/Ks ratio.
To provide a full understanding of the synteny relationship between orthologous PYL genes, we constructed syntenic analysis maps using the default parameters of TBtools Multiple Synteny Plotter. This study identified conserved genomic regions and the degree of synteny among the PYL genes. The homologous genetic linkages of the PYL genes were visualised using the TBtools Amazing Super Circos program.
Evaluation of cis-acting elements in StPYL promoters
To identify the cis-acting elements, up to 2000 bp upstream of the initiation codon (ATG) of 17 StPYLs were extracted using TBtools. Then all of the 2000 bp sequences were uploaded to the PlantCARE website (http://bioinformatics.psb.ugent.be/webtools/plantcare/html/) for the investigation of plant cis-regulatory components of all StPYLs (Conesa et al. 2005).
Protein–protein interaction prediction analysis and miRNA target prediction
Interaction networks of StPYL proteins were predicted using the STRING database (http://string-db.org/cgi) and displayed through Cytoscape software (ver. 3.8.2). The targeted relationship between microRNA (miRNA) and PYL genes in potato was predicted with psRNATarget (https://www.zhaolab.org/psRNATarget/) and then visualised by Cytoscape (ver. 3.8.2).
Expression profile of StPYLs in different tissues and under various stresses
To examine the expression patterns of 17 StPYL genes, we used the Potato Genome Sequencing Consortium’s website (http://spuddb.uga.edu/pgsc_download.shtml) for data of FPKM (fragments per kilobase of transcript per million mapped reads) extraction. Comprehensive expression data for representative transcripts from the 40 DM and 16 RH libraries were provided in this dataset.
Fourteen different potato tissues were studied for StPYL expression, including the callus, roots, shoots, leaves, petioles, stolons, tubers, stamens, sepals, carpels, petals, full flowers, immature fruits, and mature fruits. This dataset also included the expression of gene under various biotic (P. infestans, β-aminobutyric acid (BABA), benzothiadiazole (BTH) treatments under stress conditions) and abiotic (salinity, drought, heat) stresses. To display the expression patterns, heatmaps of StPYLs expressions were made using the TBtools (Chen et al. 2020a). Heatmaps were created to display the variation in StPYL gene expression levels across different tissues and developmental stages, and under different stresses using the normalised log2(FPKM) values.
Plant material and stress treatments
The tetraploid potato cultivar Desiree was utilised as the experimental subject in this investigation. Aseptic explants were generated from 4-week-old sterile seedlings of Desiree cultivated in culture vessels. These explants were subsequently sub-cultured on 30 mL of Murashige and Skoog (MS) medium for a duration of 30 days. Following this, potato seedlings were transplanted into pots and placed in greenhouses under a photoperiod of 16 h of light and 8 h of darkness at a temperature of 22°C. To analyse the expression of the PYL genes, abiotic stresses including 150 mM NaCl (salinity) and 260 μM mannitol (dehydration) were applied to whole plants for durations of 0, 5, and 10 h. Biotic stress treatments involved the imposition of P. infestans on the leaves for 10 h. Samples were collected at 0, 5, and 10 h post-treatment.
Total RNA extraction and reverse transcription quantitative PCR (RT-qPCR)
Total RNA was extracted from various samples using the SteadyPure Plant RNA Extraction Kit (Accurate Biology, Changsha, China). The extracted RNA was subjected to a purity assay using a NanoDrop 2000c spectrophotometer (Thermo, USA), where the OD260/OD280 ratio was employed to evaluate the level of protein contamination, with readings within the range of 1.8–2.1 indicating high-quality RNA. RNA integrity was further assessed by loading samples onto a 1.0% agarose gel with 1× TAE running buffer for electrophoresis, ensuring that the RNA was intact before proceeding to complementary DNA (cDNA) synthesis. Subsequently, cDNA was synthesised using the PrimeScript RT Reagent Kit (TakaRa, Kusatsu, Japan) following the manufacturer’s instructions. RT-qPCR was conducted with the following program: (1) preincubation at 95°C for 2 min; (2) denaturation at 95°C for 15 s; and (3) annealing/extension at 60°C for 20 s, repeated for 40 cycles. The remaining steps were performed according to the default settings of the instrument. The EF1a gene served as the reference gene. Three independent biological replicates were analysed, and gene expression levels were quantified using the 2−ΔΔCt method. The primer sequences for RT-qPCR are provided in Table S2. Finally, the data visualised using GraphPad Prism 8.0.2 (GraphPad Software, San Diego, CA, USA). Data were presented as mean ± s.e. Statistical significance was assessed using Student’s t-tests (*P < 0.05; **P < 0.01).
Results
Identification and features of StPYLs
We used 14 AtPYL protein sequences as references to identify the PYL protein in potato genome and identified the 17 StPYL proteins that were named according to their physical locations on the corresponding chromosomes (Table 1). Further, we investigated the physiochemical properties of StPYLs. The amino acid length varied among StPYLs ranging from 154 (StPYL6) to 231 (StPYL11). The relative molecular weight ranged from 17,274.54 Da (StPYL6) to 25,285.95 Da (StPYL11) (Table 1). Based on the isoelectric points (pI), 11 StPYLs proteins were acidic nature with pIs ≤ 6.5, five were neutral 6.5 < pI < 7.5, and only one was alkaline with with pIs > 7.5. (Table 1). Meanwhile, the instability index indicated about the stable and unstable StPYL proteins while the GRAVY values indicating their hydrophilic nature. The subcellular localisation analysis predicted that most StPYL proteins were primarily localised in cytoplasm with few exceptions to mitochondrion, nucleus, and chloroplast.
Name | Chromosome location | Gene ID | Transcript ID | Protein ID | Protein length | MW (Da) | pI | Instability index | GRAVY | Subcellular location | |
---|---|---|---|---|---|---|---|---|---|---|---|
StPYL1 | 1:73262565:73265101 | PGSC0003DMG400000215 | PGSC0003DMT400000606 | PGSC0003DMP400000459 | 186 | 20,793.66 | 6.30 | 47.53 | −0.290 | Chloroplast | |
StPYL2 | 2:32093246:32093821 | PGSC0003DMG400010238 | PGSC0003DMT400026521 | PGSC0003DMP400018101 | 191 | 21,587.47 | 4.97 | 49.16 | −0.232 | Cytoplasm, nucleus | |
StPYL3 | 3:35845286:35846191 | PGSC0003DMG400020606 | PGSC0003DMT400053119 | PGSC0003DMP400035814 | 201 | 22,158.08 | 5.95 | 34.63 | −0.074 | Cytoplasm | |
StPYL4 | 3:35848465:35849370 | PGSC0003DMG400020599 | PGSC0003DMT400053102 | PGSC0003DMP400035800 | 201 | 22,158.08 | 5.95 | 34.63 | −0.074 | Cytoplasm | |
StPYL5 | 3:35855161:35856066 | PGSC0003DMG400020607 | PGSC0003DMT400053120 | PGSC0003DMP400035815 | 201 | 22,158.08 | 5.95 | 34.63 | −0.074 | Cytoplasm | |
StPYL6 | 3:48808147:48810927 | PGSC0003DMG400009108 | PGSC0003DMT400023511 | PGSC0003DMP400016033 | 185 | 20,958.80 | 5.61 | 38.20 | −0.311 | Chloroplast | |
PGSC0003DMT400023512 | PGSC0003DMP400016034 | 154 | 17,274.54 | 4.77 | 43.35 | −0.172 | Nucleus | ||||
StPYL7 | 5:47710525:47711337 | PGSC0003DMG400027118 | PGSC0003DMT400069734 | PGSC0003DMP400047101 | 208 | 22,885.80 | 7.15 | 35.59 | −0.283 | Cytoplasm | |
StPYL8 | 6:30365180:30366374 | PGSC0003DMG400023949 | PGSC0003DMT400061535 | PGSC0003DMP400041401 | 218 | 23,996.03 | 6.40 | 45.18 | −0.235 | Cytoplasm | |
PGSC0003DMT400061536 | PGSC0003DMP400041402 | 218 | 23,996.03 | 6.40 | 45.18 | −0.235 | Cytoplasm | ||||
StPYL9 | 6:44736847:44737898 | PGSC0003DMG400002100 | PGSC0003DMT400005378 | PGSC0003DMP400003768 | 213 | 23,716.28 | 4.98 | 32.97 | −0.395 | Cytoplasm, nucleus | |
StPYL10 | 8:36289145:36290327 | PGSC0003DMG400029952 | PGSC0003DMT400077008 | PGSC0003DMP400052190 | 188 | 21,082.03 | 6.65 | 38.65 | −0.322 | Cytoplasm | |
StPYL11 | 8:49657262:49658162 | PGSC0003DMG400017514 | PGSC0003DMT400045156 | PGSC0003DMP400030588 | 231 | 25,285.95 | 5.12 | 36.29 | −0.401 | Cytoplasm, nucleus | |
StPYL12 | 8:55782145:55786603 | PGSC0003DMG400012155 | PGSC0003DMT400031686 | PGSC0003DMP400021479 | 189 | 21,572.67 | 6.75 | 36.59 | −0.368 | Chloroplast, mitochondrion, nucleus | |
StPYL13 | 9:10575747:10576691 | PGSC0003DMG400029194 | PGSC0003DMT400075056 | PGSC0003DMP400050836 | 214 | 23,368.31 | 8.60 | 42.11 | −0.286 | Cytoplasm | |
StPYL14 | 10:49647857:49648738 | PGSC0003DMG400015897 | PGSC0003DMT400041080 | PGSC0003DMP400027854 | 207 | 22,815.81 | 7.10 | 48.50 | −0.303 | Cytoplasm | |
StPYL15 | 10:53771445:53772594 | PGSC0003DMG400011033 | PGSC0003DMT400028658 | PGSC0003DMP400019485 | 213 | 23,496.32 | 6.79 | 57.45 | −0.302 | Cytoplasm | |
StPYL16 | 12:53685700:53690893 | PGSC0003DMG400005016 | PGSC0003DMT400012887 | PGSC0003DMP400008947 | 177 | 20,186.94 | 5.97 | 43.88 | −0.337 | Chloroplast, mitochondrion, nucleus | |
PGSC0003DMT400012888 | PGSC0003DMP400008948 | 177 | 20,186.94 | 5.97 | 43.88 | −0.337 | Chloroplast, mitochondrion, nucleus | ||||
StPYL17 | 12:57787723:57789097 | PGSC0003DMG400029300 | PGSC0003DMT400075340 | PGSC0003DMP400051018 | 190 | 21,242.03 | 5.12 | 34.26 | −0.275 | Cytoplasm |
Mapping of the PYL gene loci onto the potato chromosomes revealed that the 17 StPYLs distributed unevenly across nine chromosomes (Fig. 1). Notably, StPYL3/4/5/6 were clustered on chromosome 3 (Chr 3), while StPYL10/11/12 were located on Chr 8. Furthermore, single PYL genes were identified on Chr 1, Chr 2, Chr 5, and Chr 9 (StPYL1, StPYL2, StPYL7, and StPYL13, respectively). Dispersion of StPYL genes across multiple chromosomes could suggest functional divergence or redundancy within the gene family, allowing for fine-tuning of ABA signalling in various tissues or developmental stages.
Phylogenetic and molecular evolution analysis of StPYLs
To examine the evolutionary relationship of potato PYL proteins, we created a phylogenetic tree by using the protein sequence alignment of 17 StPYLs along with 14 AtPYLs, 12 OsPYLs, 11 CaPYLs, 14 SlPYLs, and 14 ZmPYLs (Fig. 2). These proteins were divided into three subfamilies: (1) Subfamily I with 31 PYLs; (2) Subfamily II with 20 PYLs; and (3) Subfamily III with 31 PYLs (Fig. 2). Representatives from both eudicots and monocots in all three groups indicate that PYLs originated before their divergence, suggesting long-term conservation of PYL genes evolution across plant lineages.
Phylogenetic analysis of PYL gene family from Solanum tubersoum, Arabidopsis thaliana, Solanum lycopersicum, Capsicum annuum, Oryza sativa, and Zea mays. Phylogenetic tree was constructed by MEGA X using neighbour-joining method with 1000 bootstrap values. PYL proteins are divided into three subfamilies: (1) I, green; (2) II, turquoise; and (3) III, pink.
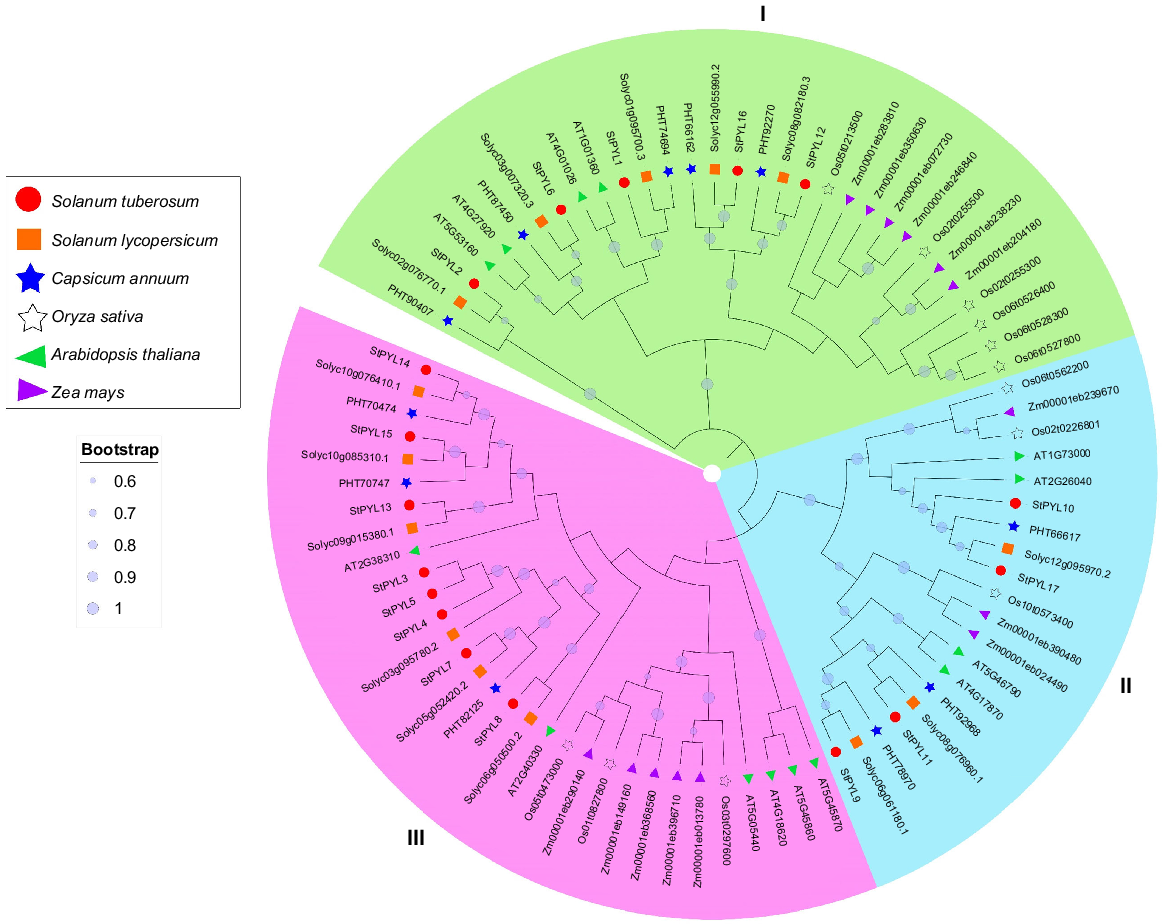
Gene structure and conserved motifs of StPYLs
To elucidate the organisation of StPYLs, we thoroughly examined the exon-intron architecture of these genes and further analysed the conserved motifs in their protein sequences (Fig. 3). Gene structure results revelled that the three genes from Subfamily I contain the two introns, one contains three introns while one gene contain no introns. All the genes from Subfamily I contain two exons except StPYL2 that has only one exon. In Subfamily II two genes (StPYL17, StPYL10) contain two introns and two exons, while other two genes have only single exon. Subfamily III members contain a single exon and lack introns.
PYL gene structure and conserved motifs analysis. (a) Phylogenetic tree of StPYLs. Subfamily I, green boxes; Subfamily II, turquoise boxes; Subfamily III, pink boxes. (b) Conserved motifs were detected using MEME and are represented by boxes of different colours. (c) Gene structures were generated using the Gene Structure Display Server (GSDS 2.0), exons (CDS) and introns are indicated with green ellipses and black lines, respectively, and yellow ellipses indicate untranslated regions (UTRs).
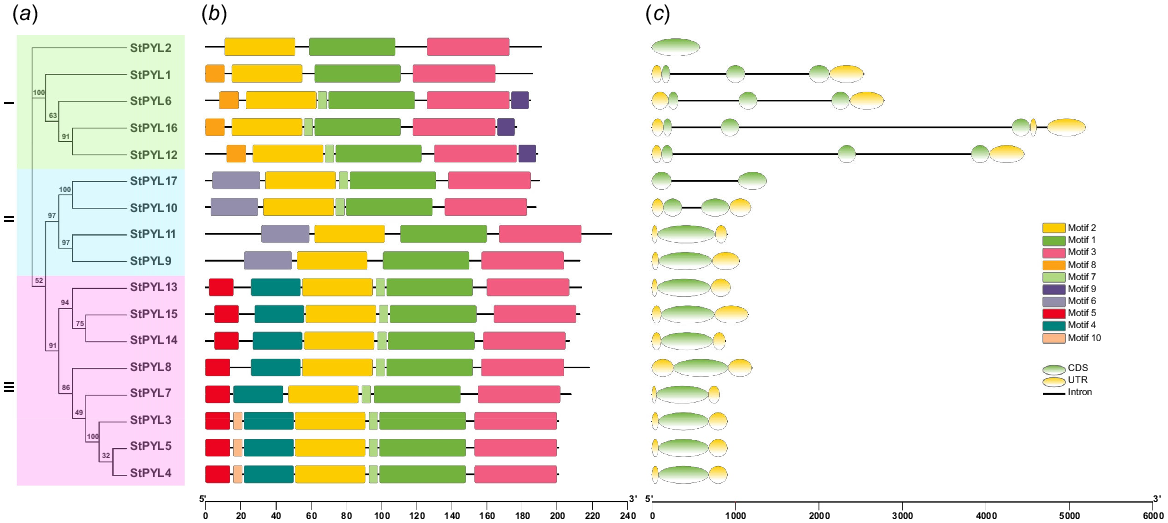
Using the MEME website, we discovered 10 conserved motifs in StPYLs (Fig. 3b, Table S3). Among these, three motifs (Motif 1, Motif 2, and Motif 3) were found to have known descriptions: ‘Pathogenesis-related protein Bet v 1 family’, ‘Polyketide cyclase/dehydrase and lipid transport’, and ‘BDHCT (NUC031) domain’, and these three motifs were present in all members of StPYLs, which provides insight into the functional roles of the PYL proteins. While some motifs were specific to the subfamilies. Subfamily I members contained Motif 8 and Motif 9 with some exceptions such as StPYL2 did not contain these motifs. The members of Subfamily II contained Motif 6 while Subfamily III members contained Motif 4, Motif 5, and Motif 7. The remaining seven motifs, although not having explicit descriptions, likely play essential roles in the structural integrity and function of PYL proteins. These conserved motifs may be crucial for the proper folding, stability, and interaction of PYL proteins with other components of the ABA signalling pathway. Understanding the precise roles of motifs requires further investigation, possibly through structural and functional analyses. These findings suggest that proteins within the same subfamily possess motifs with similar properties, indicating potential shared biological roles. In contrast, proteins with distinct motifs may perform specialised or unique functions.
Gene duplication and synteny analysis of StPYLs
Gene family formation and evolution are significantly influenced by tandem, segmental, and whole-genome duplication. Our findings revealed the gene duplications events. We found the 10 gene duplication events in potato PYL gene family through segmental duplication (SD). These duplicated genes were distributed across Chr 1, Chr 3, Chr 5, Chr 6, Chr 8, Chr 9, Chr 10, and Chr 12 (Fig. 4a). Further, we analysed the substitution rates of synonymous (Ka) and non-synonymous (Ks) mutations to determine the selection pressure present during duplication events. The Ka/Ks values of duplicated StPYLs ranged from 0.0560 to 0.1750, indicating that purifying selection has predominantly driven their evolution (Table 2). To explore evolutionary relationships and gene arrangement similarities across species, we conducted a synteny analysis (Fig. 4b, Table S4). We compared the synteny of StPYLs in potatoes with orthologous genes in five other plant species: two monocots (rice and maize) and three dicots (Arabidopsis, pepper, and tomato). The synteny between potato PYLs and dicots was generally greater than that with monocots. There were 13, 13, 15, seven, and five StPYLs that had collinearity with the PYLs of A. thaliana, C. annuum, S. lycopersicum, O. sativa, and Z. mays, respectively.
Gene duplication and synteny analysis. (a) Duplicate gene pairs in Solanum tuberosum. (b) Synteny between StPYLs and PYLs from five other species (Arabidopsis thaliana, Solanum lycopersicum, Capsicum annuum, Oryza sativa, Zea mays). The grey lines represent background which indicated the collinear blocks within the genomes of S. tuberosum and other species, PYL pairs were marked by red lines.
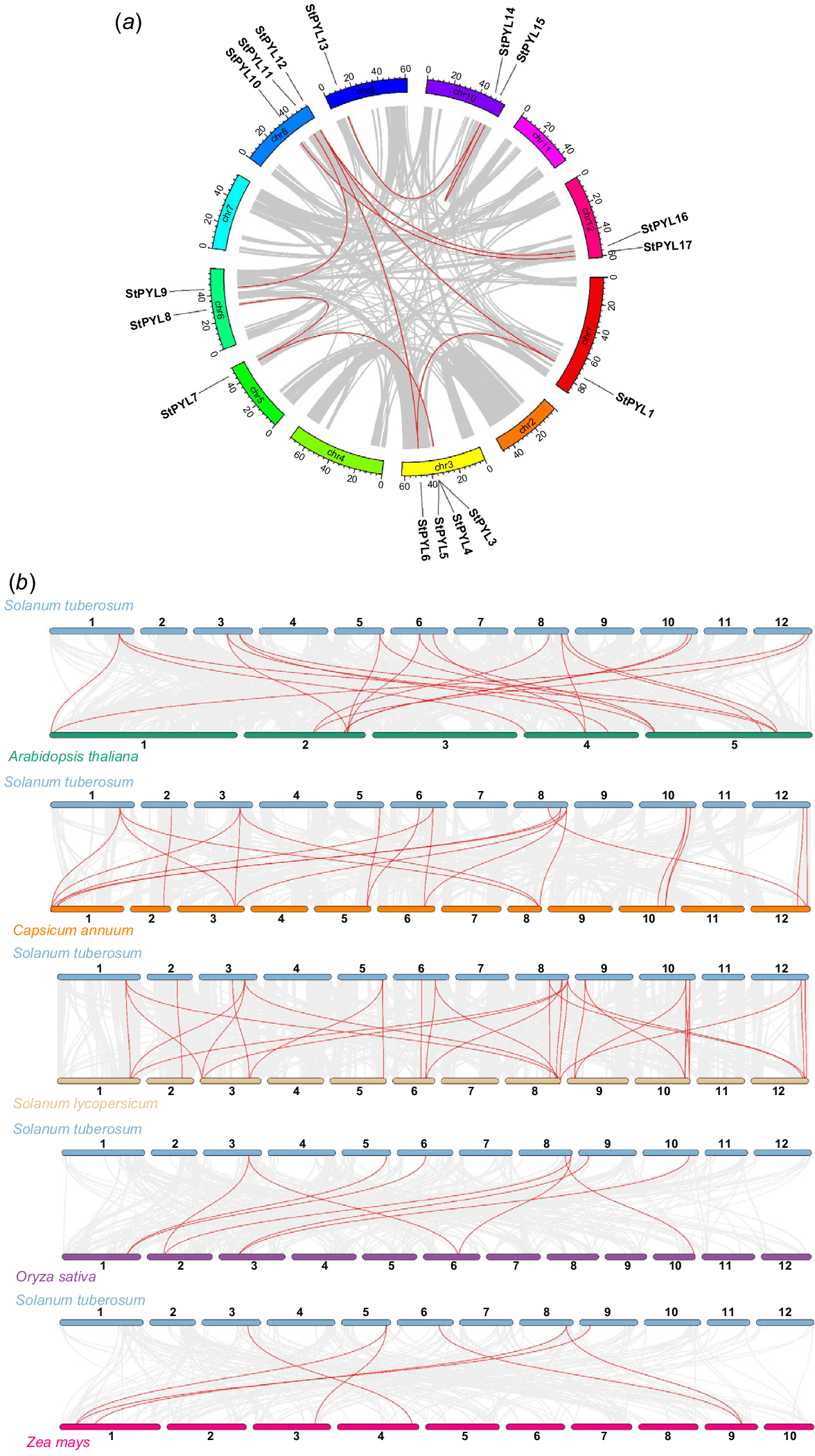
Seq 1 | Seq 2 | Type of gene duplication | Ka | Ks | Ka_Ks | Divergence time (MYA) | |
---|---|---|---|---|---|---|---|
StPYL1 | StPYL6 | SD | 0.15 | 1.94 | 0.08 | 64.62 | |
StPYL1 | StPYL12 | SD | 0.17 | 1.59 | 0.11 | 52.90 | |
StPYL3 | StPYL7 | SD | 0.15 | 0.92 | 0.17 | 30.70 | |
StPYL6 | StPYL12 | SD | 0.14 | 1.88 | 0.07 | 62.93 | |
StPYL7 | StPYL8 | SD | 0.14 | 1.33 | 0.10 | 44.44 | |
StPYL9 | StPYL11 | SD | 0.15 | 0.88 | 0.17 | 29.48 | |
StPYL17 | StPYL10 | SD | 0.09 | 1.69 | 0.06 | 56.38 | |
StPYL16 | StPYL12 | SD | 0.05 | 0.73 | 0.07 | 24.45 | |
StPYL14 | StPYL13 | SD | 0.17 | 1.03 | 0.17 | 34.41 | |
StPYL14 | StPYL15 | SD | 0.14 | 1.49 | 0.09 | 49.76 |
MYA, million years ago.
Promoter analysis of StPYLs
We examined the cis-acting regulatory elements (CREs) in the promoter regions of StPYLs to understand gene regulation. We analysed the 2000 bp upstream sequences using PlantCARE. The StPYL promoters were found to contain numerous regulatory elements, with a significant emphasis on hormone and stress-related CREs (Fig. 5). Key stress-responsive elements identified included MBS, LTR (low temperature responsive), TC-rich repeats, WUN-motif, and W box, which were present in the promoter regions of 14 StPYLs (Table S5). Notably, LTR elements were the most prevalent. Hormone-responsive elements were also abundant, with ABA response elements (ABRE) and ethylene responsive element (ERE) being the most prominent. Other hormone-related elements including P-box, TGACG-motif, CGTCA-motif, TCA-element, TATC-box, GARE-motif, and TGA-element, indicating responsiveness to various hormones such as ABA, ethylene, auxin, and gibberellins. The presence of multiple auxin-responsive and gibberellin-responsive elements in the promoters suggests a potential interaction between phytohormones in regulating ABA receptor development. StPYL1 exhibited the highest number of cis-acting elements, followed by StPYL2, StPYL2, StPYL6, and StPYL8. Except StPYL3/5/4, all other StPYLs contained LTR and TC-rich repeats within their promoter regions. While this analysis provides a comprehensive overview of CREs, further experimental validation in necessary to confirm their functional relevance. Previous studies have shown that similar CREs play crucial roles in gene regulation under stress conditions and hormone treatments, which support our findings (Billah et al. 2022; Liu et al. 2022; Marand et al. 2023).
Genomic distribution of cis-regulatory elements in StPYL promoter regions. Different cis-regulatory elements are indicated with different colours. The cis-regulatory elements in promoter regions were predicted using PlantCARE (http://bioinformatics.psb.ugent.be/webtools/plantcare/html/) and drawn by TBtools.
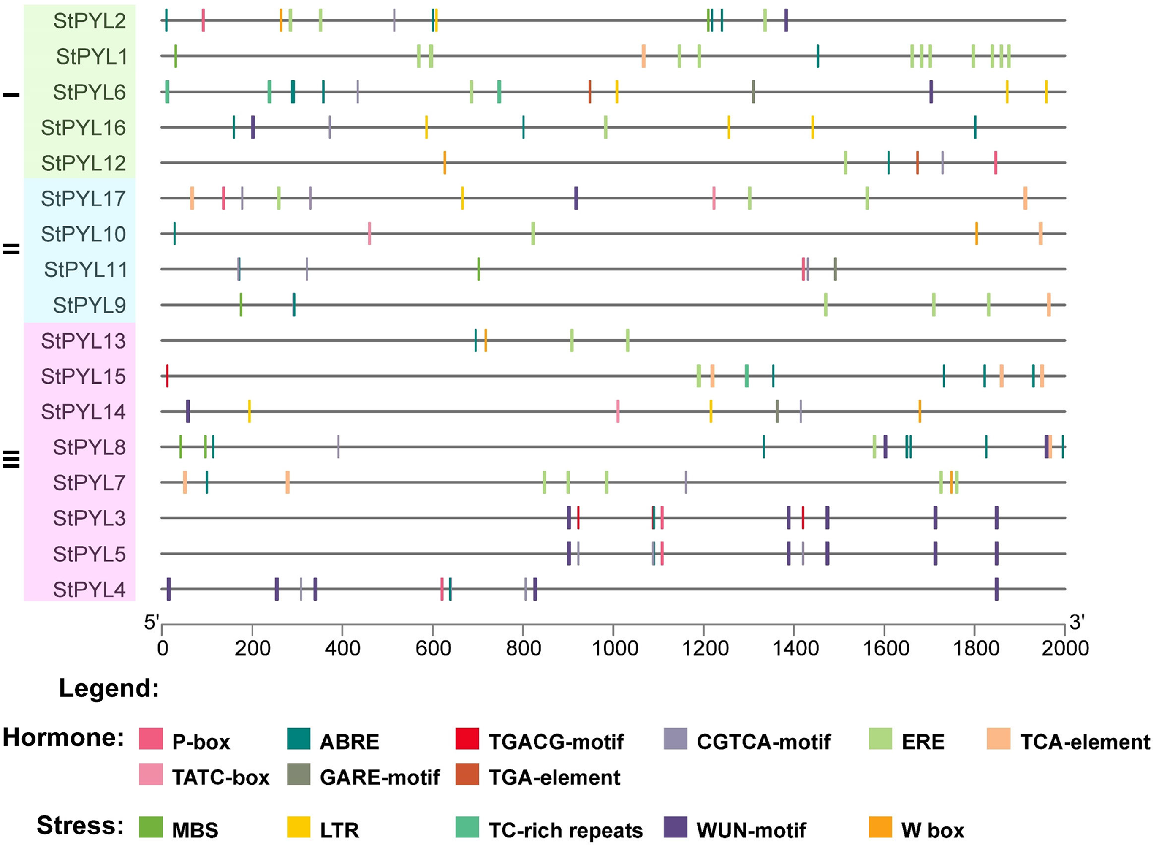
Protein interaction networks analysis of StPYLs and miRNA targets
The STRING database was used to predict the protein–protein interactions (PPI) of the StPYLs, which enhanced our understanding of the biological function and regulatory network associated with them. The results showed the 14 StPYLs interacted with 21 functional proteins.
Due to PYL protein involvement in ABA signalling their interacting proteins were expected to be involved in ABA signalling complex. As anticipated, the majority of the proteins were predicted to interact were crucial and functionally verified constituents of the ABA signalling complex, such as clade A protein phosphatase 2C (PP2C) and SNF1-related protein kinase 2 (SnRK2), as shown in Fig. 6. Moreover, we observed that PYL proteins may have interactions with polyketide synthases (PKSs). According to functional annotations, these interacting proteins of StPYLs can be divided into three groups: (1) five phosphatase 2C family proteins; (2) seven serine/threonine-protein kinases; and (3) three protein phosphatase 2C-like proteins. Additionally, one MYB27 protein showed an indirect interaction with StPYL proteins (Fig. 6). Although our analysis offers predictions, experimental validation will be necessary in future studies to thoroughly explore the relevance of these findings.
Predicted protein–protein interaction networks of StPYL proteins with other potato proteins using STRING tool. The green circles represent potato PYL proteins, and the circles on the inside represent proteins that interact with StPYLs. The two circles connected by the grey line represent the interaction between the proteins.
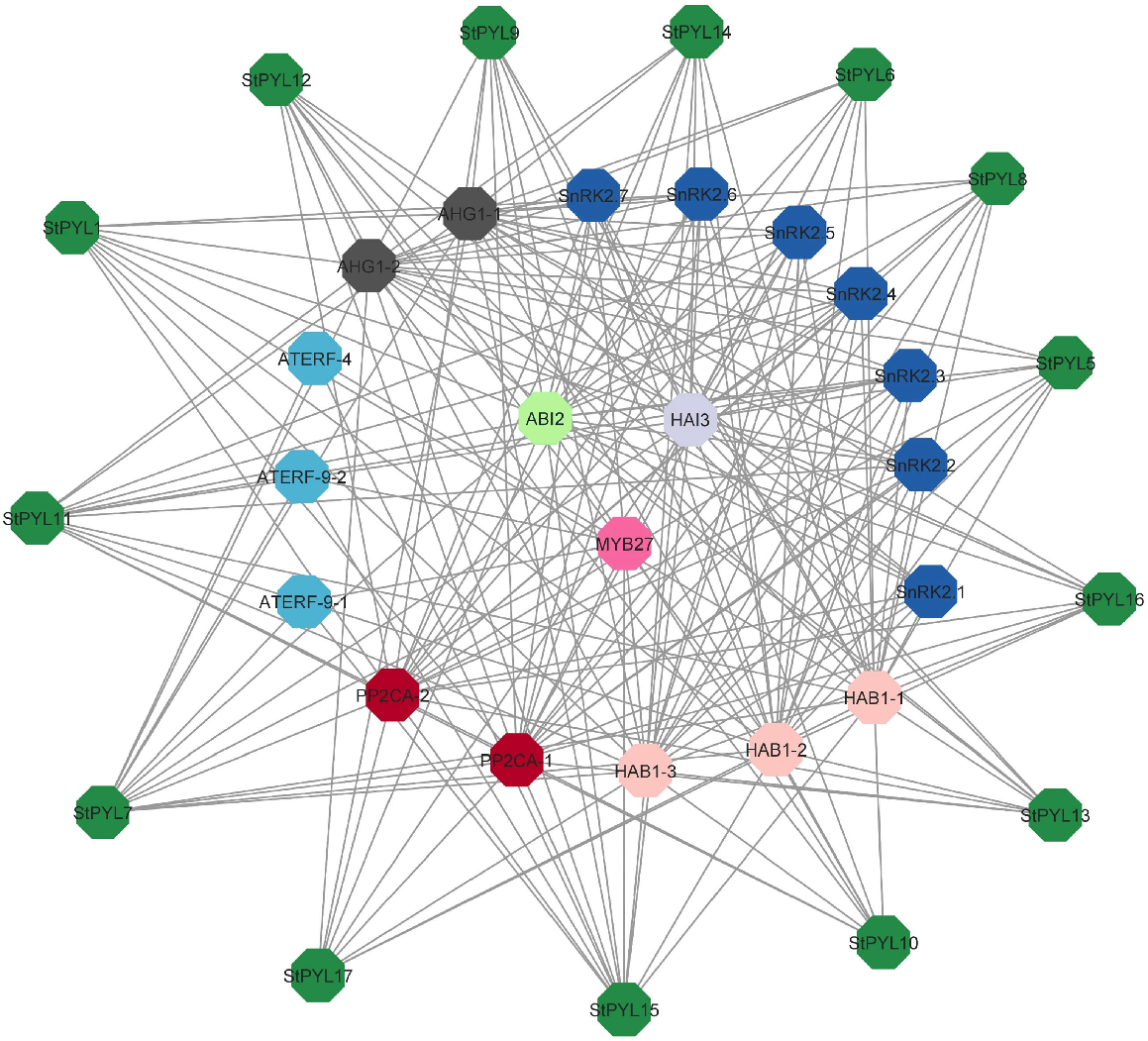
Moreover, the relationships between the miRNAs and StPYLs were predicted using psRNATarget database. We identified that all 17 StPYLs were targeted by several miRNAs. StPYL15 were targeted by 20 miRNAs, which is the most targeted gene. StPYL6 and 16 were targeted by six miRNAs, while StPYL12 were targeted by five miRNAs, respectively. StPYL1, StPYL8, and StPYL10 were targeted by three miRNAs while StPYL13 were targeted by only one miRNA. Each of the other 10 StPYLs was targeted by two miRNAs (Fig. 7).
Expression pattern of StPYLs among different potato tissues
To investigate the biological functions of StPYL gene, we examined the publicly available transcriptome data set 14 different tissues, including callus, leaves, roots, shoots, tubers, sepals, petals, stamens, mature flowers, petioles, carpels, stolons, mature fruit, and immature fruit, and we found the tissue-specific expression patters of StPYLs. For example, StYPL5/4/9/13/14/15 exhibited relatively higher expression levels in stolons, indicating their potential importance in potato seed development (Fig. 8). Additionally, StYPL3/4/5/7/8 showed notable expression levels in callus and tubers, suggesting their significance in these tissues. StPYL10 and StPYL17 displayed substantial expression levels in shoots. StPYL11 exhibited exclusive and high-level expression in petioles, while StPYL2, StPYL11, and StPYL12 showed relatively lower expression levels across various tissues (Fig. 8). However, StPYL2 exhibited high expression level in mature fruits than the other tissues. These variations in the expressions of StPYLs across different tissues indicate their involvements in diverse stages of potato growth and development, potentially contributing to geographical differences in potato phenotypes.
Expression profile of StPYLs in 14 tissues based on transcriptomic data. The heatmap was generated using the log2 expression levels (FPKM). The colour bar to the right represents the log2 expression value, with red representing upregulated, and blue representing downregulated expression level. PYL Subfamily I, green; Subfamily II, turquoise; and Subfamily III, pink.
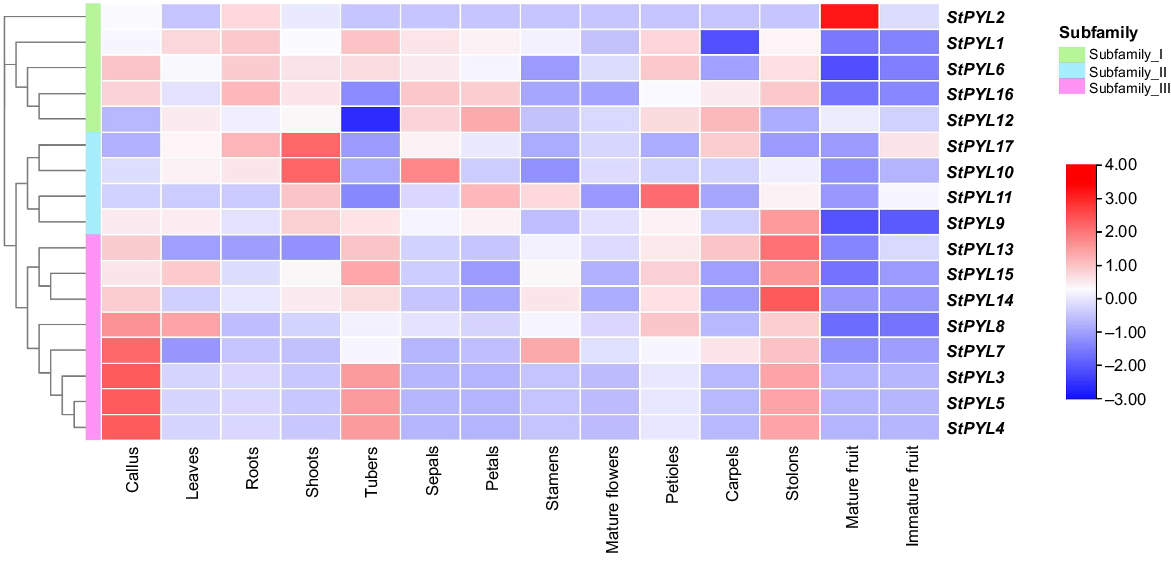
Response of StPYLs expression to various stresses
We examined the expression patterns of the 17 StPYLs under various abiotic and biotic stresses (Figs 8 and 9). Under abiotic stresses, we observed the diverse expression pattern such as StPYL6 showed the higher expression level under drought and salt stress, while StPYL1 showed higher expression level under all observed abiotic stress. StPYL11/13/14/15 showed the lower expression pattern under drought and salt stresses, while StPYL13/14 showed the upregulation under heat stress (Fig. 8). For the biotic stress the results showed that among StPYLs, five members of StPYL3/5/4/8/13, exhibited high expression exclusively in response to P. infestans, indicating their potential roles in this specific pathogen-induced stress. In contrast, five other members StPYL6/12/15/14/8 showed relatively lower expression levels in response to BTH and BABA treatments, suggesting their involvements in different aspects of biotic stress response pathways (Fig. 9). Notably, StPYL2 and StPYL13 demonstrated high expression specifically in response to BABA treatment, indicating their unique role in this particular stress condition. By examining the expression of StPYLs under diverse biotic stress conditions, we gain insights into their potential roles in specific signalling pathways activated during biotic stress responses.
StPYL genes expression levels in response to various biotic and abiotic stresses. The heatmap was generated using the log2 expression levels (FPKM). The colour bar to the right represents the log2 expression value, with red representing upregulated and blue representing downregulated expression level. PYL Subfamily I, green; Subfamily II, turquoise; and Subfamily III, pink. Yellow bars, abiotic stresses; green bars, biotic stresses.
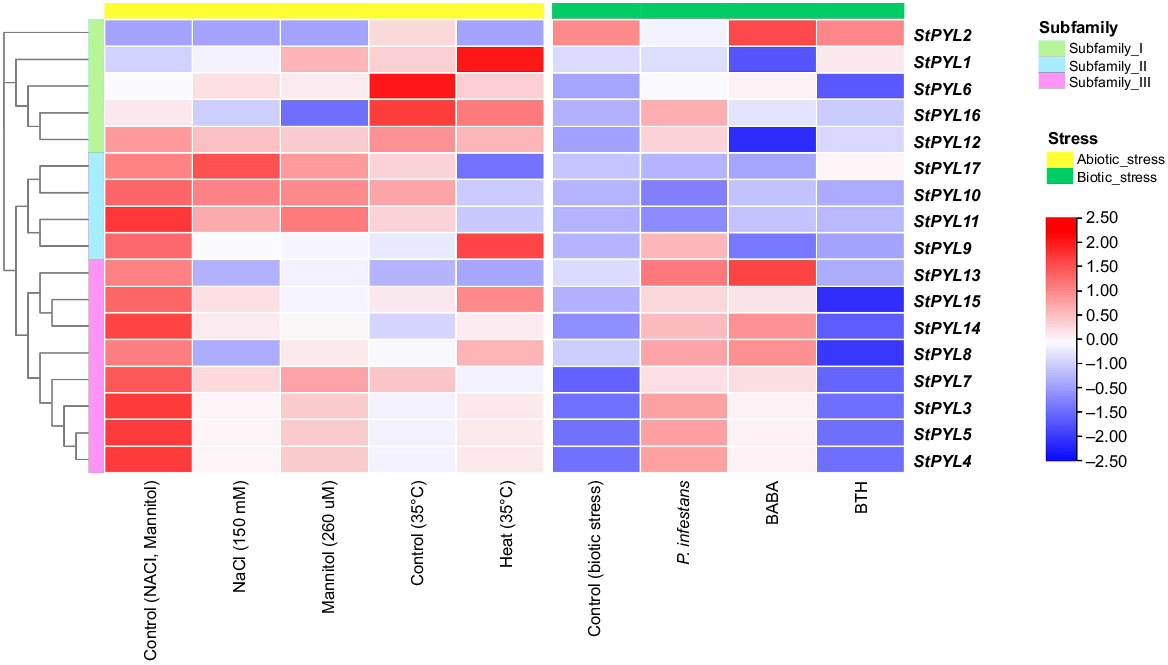
Expression validation of selected StPYLs under various stress treatments
Further to validate the expression pattern of StPYLs under the abiotic and biotic stresses, we selected the eight StPYL genes and performed RT-qPCR to access their expression pattern under drought, salt (abiotic stresses), and P. infestans (biotic stress) stresses. We accessed the expression level at three time intervals (0, 5, and 10 h) and we observed the significant variation (Fig. 10). Specifically, StPYL6 showed the upregulation under all the treatments and the expression level increased with time interval in drought and P. infestans stresses while it decreases in salt stress. All the observed genes showed the upregulation in their expression level under P. infestans stress except StPYL11, which showed downregulation and this downregulation increased with the time interval. However, the observed genes showed the downregulation of their transcript level under the drought and salt stresses and their downregulation varied with the time interval in some genes.
Discussion
ABA is a crucial regulator of various essential agronomical traits, including seed maturation, germination, transition from vegetative to reproductive growth, and responses to abiotic and biotic stresses (Guo et al. 2017; Yadav et al. 2020). The PYL gene family is essential for the growth and development of plants in the ABA signal transduction pathway and as the ABA receptor (Ali et al. 2022). It is crucial to comprehend the identification and functional validation of PYLs in the ABA metabolic process and signal transduction pathway in order to breed improved crops with superior agronomic traits and stress tolerance, addressing the challenges posed by anthropogenic climate change (Lei et al. 2021; Zhang et al. 2022a). Previously, PYL genes were identified and characterised among several plant species, including Arabidopsis (Ma et al. 2009), cucumber (Cucumis sativus) (Zhang et al. 2022a), rice (Kim et al. 2014), cotton (Liu et al. 2023), rubber tree (Hevea brasiliensis) (Guo et al. 2017), rapeseed (Brassica napus) (Di et al. 2018), maize (He et al. 2018), barley (Hordeum vulgare) (Shahzad et al. 2023b), and sunflower (Helianthus annuus) (Wang et al. 2024). However, the majority of PYL gene family research initiatives have primarily focused on functional diversification of PYLs in Arabidopsis (Wang et al. 2022). There has been little search on the genome-wide functions of PYL gene family and its evolutionary link to other important crops. Our study sought to fill this gap in knowledge by thoroughly examining the functions of PYL genes in development and their responses to abiotic stimuli using both bioinformatic and experimental methods according to Yadav et al. (2020). In particular, we discovered 17 StPYLs in potatoes by using 14 AtPYLs (Park et al. 2009). With the aid of chromosome mapping, we learned that the PYL genes in potatoes were spread throughout the genome, with Chr 3 being the high number of StPYLs (Fig. 1). In contrast, the partial clustering seen in rapeseed and rice (Di et al. 2018; Yadav et al. 2020) suggests distinct evolutionary paths among different plant species. Our comprehensive study provides valuable information on the biological roles, chromosomal distribution, and evolutionary relationships of the PYL gene family, specifically in potatoes. It also expands our understanding of ABA-mediated processes and provides a starting point for further study of the PYL gene family in other important crops. The similarity between the PYL genes from potatoes and A. thaliana suggests potential functional conservation in specific biological processes (Pieczynski et al. 2018).
The gene families has expanded as a result of a number of mechanisms, including segmental, tandem, and whole-genome duplication events (Yanai 2022). In current study, we found that StPYL genes showed the segmental duplication. Further, we computed the Ka/Ks values that indicated the purifying selection (Maqsood et al. 2022). The PYL genes from potatoes and five other plant species (A. thaliana, C. annuum, S. lycopersicum, O. sativa, and Z. mays) were divided into three different groups by the phylogenetic tree analysis (Fig. 2). This grouping was similar to those discovered in rubber trees (Guo et al. 2017), cotton (Zhang et al. 2017), rice (Yadav et al. 2020), rapeseed (Di et al. 2018), and barley (Shahzad et al. 2023b), but it was different from the groups discovered in apples (Malus domestica) (Hou et al. 2020). These clusters in the evolutionary tree were supported by the exon-intron structure (Fig. 3). The variety of species being sequenced could be the cause of the observed variances. Furthermore, the close clustering of potato PYLs with dicotyledonous plants rather than monocotyledonous plants was consistent with the relationships that these species had developed over evolutionary time. Members of diverse groups shared structural similarities, and closely related PYL genes, especially those in Subfamilies I and II, showed comparable exon lengths, indicating a high amount of evolutionary conservation. The significance of gene structure as a crucial determinant for establishing the evolutionary links among members of a gene family is highlighted by our findings, which are in line with other study (Di et al. 2018; Zhang et al. 2021). Moreover, our subcellular localisation analysis predicted the majority of StPYL proteins were found to be located in the cytoplasm, while few in nucleus, chloroplast, and mitochondrion. This suggests their potential involvement in crucial processes such as photosynthesis, respiration, and cell growth and development (Denham et al. 2020). However, in future the confirmation of subcellular localisation of these proteins can offer valuable insights into their functional roles.
In this study, analyses of phylogeny, gene structure, and conserved domains supported the relatively conserved structures of StPYL proteins. In this investigation, conserved motifs identified in StPYLs and these motifs were highly conserved across PYL gene families (Subfamilies I, II, and III), indicating their importance. We further identified these motifs as part of the polyketide cyclase/dehydrase enzyme family, known for its involvement in polyketide production. Similar findings in pear and rice studies confirmed that these novel motifs are distinct from existing motifs (Yadav et al. 2020), suggesting the presence of unique motifs in PYLs. The motifs identified in StPYLs suggest diverse and significant roles in plant stress responses. The presence of the Bet v 1 family motifs, known for their involvement in pathogen resistance, indicates that StPYLs might enhance defense mechanisms against biotic stress by inhibiting pathogen growth or neutralising toxins (Sinha et al. 2014). The identification of polyketide cyclase/dehydrase and lipid transport motifs suggests that StPYLs may participate in the biosynthesis of polyketides and lipid signalling pathways, which are crucial for producing secondary metabolites with antibiotic and antifungal properties and for regulating membrane biogenesis and metabolism (Shen 2003; Satheesh et al. 2014; Shin et al. 2022). Additionally, the BDHCT domain, often associated with nucleic acid binding and chromatin structure modulation, implies that StPYLs could play a regulatory role at the transcriptional level, modulating the expression of stress-responsive genes. These diverse motifs highlight the multifaceted functions of StPYLs, contributing to both metabolic regulation and enhanced plant defense under various stress conditions. In current study, the importance of PYLs in potato development, growth, and response to biotic and abiotic stimuli is highlighted by the inclusion of stress-related components such as LTR elements and defense/stress responsiveness (TC-rich repeats) in the promoters regions (Liu et al. 2021).
We predicted the interaction network of PYL proteins with other proteins. Our findings revealed that potato PYL proteins interacted with PP2C and SnRK2s, key components of the ABA signalling cascade. PP2CA negatively regulates the ABA metabolic pathway, crucial for seed germination and cold acclimatisation. Studies on the SnRK2 gene family in Arabidopsis, including SnRK2.2, SnRK2.3, and SnRK2.6, have demonstrated their positive regulation with exogenous ABA application, as well as their response to osmotic stress (Park et al. 2009). The interactions between several barley PYL proteins and PP2CA and SnRK2s also predicted, suggests their involvement in mediating biological processes (Shahzad et al. 2023b). Small RNAs (sRNAs), typically ranging from 21 to 24 nucleotides in length, have garnered significant attention due to their pivotal roles in plant growth, development, and responses to both biotic and abiotic stresses (Zuo et al. 2021). Among these, miRNAs, which are a subset of sRNAs, regulate gene expression by binding to target mRNA. Biotic and abiotic stresses impose constraints on crop growth and yield by altering metabolic activity and biological functions (Zhang et al. 2022c). Notably, miRNAs have emerged as key players in the response to biotic stress in potato. In our investigation, we identified 17 miRNA targets of StPYL genes, shedding light on their potential involvement in stress responses (Deng et al. 2021). Furthermore, the miR156 family was recognised as a primary regulator in response to high-temperature stress during G. hirsutum anther development (Chen et al. 2020b). These identified miRNA targets represent valuable candidates for further experimental exploration of the stress response mechanisms associated with StPYL genes.
PYL genes exhibited varying degrees of tissue-specific expression, consistent with the expression patterns observed in different plant tissues. Previously, have identified that some specific tissue types preferentially express certain genes in plants such as in rubber, rice, and rapeseed (Guo et al. 2017; Di et al. 2018; Yadav et al. 2020). Studies have revealed that PYL gene expression is tissue-specific such as seeds, roots, leaves, flowers, and fruits (Zhang et al. 2022a). We found that StPYL12 and StPYL9 had showed the expression levels in tubers, ripe fruit, and immature fruit. The expression levels of StPYL4/5/3/7/8 were higher in callus and tubers, while those of StPYL10 and StPY17 were higher in shoots. However, other tissues showed lower expression levels for these genes. Previously, a potential involvement for CsPYL2 in fruit development, ripening, and ABA signal transduction in cucumber seedlings under drought stress was reported (Li et al. 2020). While according to (Bai et al. 2019), the majority of PYLs in soybean (Glycine max) are expressed at considerably higher levels in seeds than in other tissues. PYL transcripts are frequently also found in the latex of rubber trees (Guo et al. 2017). Our investigation revealed that StPYLs had noticeably higher expression levels in callus, tubers, and stolons, which provides the evidence for tissue specific involvement of these genes.
Biotic and abiotic factors including salt, extreme heat, and drought have a substantial impact on potato yield and quality (Chourasia et al. 2021; Nasir and Toth 2022). ABA has been known as a key factor in reducing abiotic stressors. PYLs serve as essential building blocks in the ABA signal transduction pathway (Fernando and Schroeder 2016). The specific PYL receptor mediated the ABA response to abiotic or biotic stresses in potatoes has not yet been identified. However, the expression level of most StPYLs in potatoes align with the previous findings (Mehari et al. 2021; Wang et al. 2022; Shahzad et al. 2023b; Wu et al. 2023; Wang et al. 2024). Overexpression of the cotton GhPYL26, has been shown to enhance drought tolerance. Similarly, overexpressing AtPYL9 in A. thaliana improves its drought resistance (Mehari et al. 2021). We found that expression levels of StPYL17 and StPYL12 decrease during drought stress, with corresponding effects on StPYL1 and StPYL9. Further, we observed the responses of 17 StPYLs in potato to biotic stresses such as P. infestans, and under BABA and BTH treatments (Fig. 9). Our resulted indicated that StPYL6/12/15/14/8/12 genes had significantly low expression levels under BTH and BABA treatments. Conversely, StPYL13/3/5/4/8 exhibited high expression levels exclusively in response to P. infestans stress. This is an interesting finding because ABA involvement in abiotic stresses has extensively reported but for biotic stresses little known. Further, we conducted the RT-qPCR analysis to confirm the expression variations of selected StPYLs under biotic and abiotic stresses. Here, we also found that the expression levels of selected StPYLs significantly varied among control and biotic and abiotic treatments. Our results indicated the involvement of PYL genes in biotic stress management potentially through ABA signalling pathways. Similarly, Wu et al. (2023), found the involvement of PYL genes in biotic stress responses. They found that in the presence of biotic stress induced by scale insects and whiteflies, Liriodendron spp. hybrid leaves exhibited distinctive disease symptoms characterised by dark green centres and yellow margins. RT-qPCR analysis revealed a significant upregulation in the expression levels of PYL genes, specifically Lchi13641 and Lchi11622 by 1.76 and 3.75 times, respectively, compared to normal leaves. Moreover, recently, ABA has also been regarded as an element of the biotic stress response; however, its role is still highly unclear. Many studies connect the susceptibility to various diseases with increased concentration of this phytohormone. The involvement of ABA in biotic stress responses suggests a complex interplay between phytohormones and immune signalling pathways in plants (García-Andrade et al. 2020; Gietler et al. 2020; Wu et al. 2023). Understanding its precise role may provide insights into developing strategies for enhancing plant resilience against pathogens.
Conclusions
This study offers valuable insights into StPYL gene family, highlighting their relevance in biotic and abiotic stress responses. By employing a comprehensive approach, including gene characterisation, evolutionary analysis, gene structure, conserved motifs, synteny, promoter activity, tissue-specific expression, and protein interactions, we enhanced the understanding of the StPYLs in potato. We identified and characterised 17 StPYL genes, revealing their properties, localisation, and evolutionary origins. The classification of these genes into three subfamilies suggest an ancient and conserved lineage across plants. Exon-intron structure and conserved motifs analysis provided clues to the potential functions and evolutionary relationships of StPYLs. Synteny analysis emphasised segmental duplications contributions to the expansion of this family under purifying selection. Promoter analysis revealed hormone-responsive elements, indicating role in hormonal regulation and stress responses. Expression profiling demonstrated varied role of StPYLs in potato development and stress responses. Protein interaction network analysis identified potential ABA signalling interactors, enhancing our understanding of StPYL-mediated environmental responses. While these findings pave the way for future research into the physiological and stress response functions of StPYL gene family in potatoes, further functional validation is necessary to confirm the predicted roles and explore their potential applications in crop improvement.
Data availability
All the sequence data used in this study were downloaded from The Arabidopsis Information Resource (TAIR; https://www.arabidopsis.org), Spud DB Potato Genomics Resources (Spud DB; http://spuddb.uga.edu) and Ensembl plants database (http://plants.ensembl.org/index.html). The publicly available transcriptomic dataset of all the representative transcripts across 40 DM and 16 RH libraries: DM_RH_RNA Seq_FPKM_expression_matrix_for_DM_v4.03_13dec2013_desc.xlsx (http://spuddb.uga.edu/pgsc_download.shtml) was used to examine expression patterns of StPYLs.
Declaration of funding
The authors declare that no funds, grants, or other support were received during the preparation of this manuscript.
Author contributions
Conceptualisation: A.S., S.U.K., and H.G. Software: A.S., H.G., and S.G. Experimental validation: S.G., H.G., and I.U. Formal analysis: M.S., S.G., and H.G. Writing – original draft preparation: S.G. and H.G. Writing – review and editing: S.U.K, M.S., and A.S. All authors have read and agreed to the published version of the manuscript.
References
ain-Ali Q-U, Mushtaq N, Amir R, Gul A, Tahir M, Munir F (2021) Genome-wide promoter analysis, homology modeling and protein interaction network of Dehydration Responsive Element Binding (DREB) gene family in Solanum tuberosum. PLoS ONE 16(12), e0261215.
| Crossref | Google Scholar | PubMed |
Ali F, Qanmber G, Li F, Wang Z (2022) Updated role of ABA in seed maturation, dormancy, and germination. Journal of Advanced Research 35, 199-214.
| Crossref | Google Scholar | PubMed |
Altschul SF, Madden TL, Schäffer AA, Zhang J, Zhang Z, Miller W, Lipman DJ (1997) Gapped BLAST and PSI-BLAST: a new generation of protein database search programs. Nucleic Acids Research 25(17), 3389-3402.
| Crossref | Google Scholar | PubMed |
An Y, Mi X, Xia X, Qiao D, Yu S, Zheng H, Jing T, Zhang F (2023) Genome-wide identification of the PYL gene family of tea plants (Camellia sinensis) revealed its expression profiles under different stress and tissues. BMC Genomics 24(1), 362.
| Crossref | Google Scholar | PubMed |
Bai G, Xie H, Yao H, Li F, Chen X, Zhang Y, Xiao B, Yang J, Li Y, Yang D-H (2019) Genome-wide identification and characterization of ABA receptor PYL/RCAR gene family reveals evolution and roles in drought stress in Nicotiana tabacum. BMC Genomics 20(1), 575.
| Crossref | Google Scholar |
Billah SA, Khan NZ, Ali W, Aasim M, Usman M, Alezzawi MA, Ullah H (2022) Genome-wide in silico identification and characterization of the stress associated protein (SAP) gene family encoding A20/AN1 zinc-finger proteins in potato (Solanum tuberosum L.). PLoS ONE 17(8), e0273416.
| Crossref | Google Scholar | PubMed |
Bonthala VS, Stich B (2024) StCoExpNet: a global co-expression network analysis facilitates identifying genes underlying agronomic traits in potatoes. Plant Cell Reports 43(5), 117.
| Crossref | Google Scholar |
Chen K, Li G-J, Bressan RA, Song C-P, Zhu J-K, Zhao Y (2020a) Abscisic acid dynamics, signaling, and functions in plants. Journal of Integrative Plant Biology 62(1), 25-54.
| Crossref | Google Scholar | PubMed |
Chen J, Pan A, He S, Su P, Yuan X, Zhu S, Liu Z (2020b) Different microRNA families involved in regulating high temperature stress response during cotton (Gossypium hirsutum L.) anther development. International Journal of Molecular Sciences 21(4), 1280.
| Crossref | Google Scholar | PubMed |
Chou K-C, Shen H-B (2010) Plant-mPLoc: a top-down strategy to augment the power for predicting plant protein subcellular localization. PLoS ONE 5(6), e11335.
| Crossref | Google Scholar | PubMed |
Chourasia KN, Lal MK, Tiwari RK, Dev D, Kardile HB, Patil VU, Kumar A, Vanishree G, Kumar D, Bhardwaj V, Meena JK, Mangal V, Shelake RM, Kim J-Y, Pramanik D (2021) Salinity stress in potato: understanding physiological, biochemical and molecular responses. Life 11(6), 545.
| Crossref | Google Scholar | PubMed |
Clark BJ (2020) The START-domain proteins in intracellular lipid transport and beyond. Molecular and Cellular Endocrinology 504, 110704.
| Crossref | Google Scholar | PubMed |
Conesa A, Götz S, García-Gómez JM, Terol J, Talón M, Robles M (2005) Blast2GO: a universal tool for annotation, visualization and analysis in functional genomics research. Bioinformatics 21(18), 3674-3676.
| Crossref | Google Scholar | PubMed |
Cui Y-X, Xu Z-C, Chen X-L, Nie L-P, Wu L-W, Wang Y, Song J-Y, Yao H (2020) Genome-wide identification of abscisic acid (ABA) receptor pyrabactin resistance 1-like protein (PYL) family members and expression analysis of PYL genes in response to different concentrations of ABA stress in Glycyrrhiza uralensis. Chinese Journal of Natural Medicines 18(8), 606-611.
| Crossref | Google Scholar |
del Mar Martínez-Prada M, Curtin SJ, Gutiérrez-González JJ (2021) Potato improvement through genetic engineering. GM Crops & Food 12(1), 479-496.
| Crossref | Google Scholar | PubMed |
Deng K, Yin H, Xiong F, Feng L, Dong P, Ren M (2021) Genome-wide miRNA expression profiling in potato (Solanum tuberosum L.) reveals TOR-dependent post-transcriptional gene regulatory networks in diverse metabolic pathway. PeerJ 9, e10704.
| Crossref | Google Scholar | PubMed |
Denham T, Barton H, Castillo C, Crowther A, Dotte-Sarout E, Florin SA, Pritchard J, Barron A, Zhang Y, Fuller DQ (2020) The domestication syndrome in vegetatively propagated field crops. Annals of Botany 125(4), 581-597.
| Crossref | Google Scholar | PubMed |
Di F, Jian H, Wang T, Chen X, Ding Y, Du H, Lu K, Li J, Liu L (2018) Genome-wide analysis of the PYL gene family and identification of PYL genes that respond to abiotic stress in Brassica napus. Genes 9(3), 156.
| Crossref | Google Scholar | PubMed |
Dittrich M, Mueller HM, Bauer H, Peirats-Llobet M, Rodriguez PL, Geilfus C-M, Carpentier SC, Al Rasheid KAS, Kollist H, Merilo E, Herrmann J, Müller T, Ache P, Hetherington AM, Hedrich R (2019) The role of Arabidopsis ABA receptors from the PYR/PYL/RCAR family in stomatal acclimation and closure signal integration. Nature Plants 5(9), 1002-1011.
| Crossref | Google Scholar | PubMed |
Fernando VD, Schroeder DF (2016) Role of ABA in Arabidopsis salt, drought, and desiccation tolerance. In ‘Abiotic and biotic stress in plants-recent advances and future perspectives’. (Eds AK Shanker, C Shanker). (IntechOpen). doi:10.5772/61957
García-Andrade J, González B, Gonzalez-Guzman M, Rodriguez PL, Vera P (2020) The role of ABA in plant immunity is mediated through the PYR1 receptor. International Journal of Molecular Sciences 21, 5852.
| Crossref | Google Scholar | PubMed |
Ghorbel M, Brini F, Sharma A, Landi M (2021) Role of jasmonic acid in plants: the molecular point of view. Plant Cell Reports 40, 1471-1494.
| Crossref | Google Scholar | PubMed |
Gietler M, Fidler J, Labudda M, Nykiel M (2020) Abscisic acid—enemy or savior in the response of cereals to abiotic and biotic stresses? International Journal of Molecular Sciences 21, 4607.
| Crossref | Google Scholar | PubMed |
Guo D, Zhou Y, Li H-L, Zhu J-H, Wang Y, Chen X-T, Peng S-Q (2017) Identification and characterization of the abscisic acid (ABA) receptor gene family and its expression in response to hormones in the rubber tree. Scientific Reports 7(1), 45157.
| Crossref | Google Scholar |
Hao Q, Yin P, Li W, Wang L, Yan C, Lin Z, Wu JZ, Wang J, Yan SF, Yan N (2011) The molecular basis of ABA-independent inhibition of PP2Cs by a subclass of PYL proteins. Molecular Cell 42(5), 662-672.
| Crossref | Google Scholar | PubMed |
He Z, Zhong J, Sun X, Wang B, Terzaghi W, Dai M (2018) The maize ABA receptors ZmPYL8, 9, and 12 facilitate plant drought resistance. Frontiers in Plant Science 9, 422.
| Crossref | Google Scholar | PubMed |
Hou H, Lv L, Huo H, Dai H, Zhang Y (2020) Genome-wide identification of the ABA receptors genes and their response to abiotic stress in apple. Plants 9(8), 1028.
| Crossref | Google Scholar | PubMed |
Huang S, Wang C, Ding Z, Zhao Y, Dai J, Li J, Huang H, Wang T, Zhu M, Feng M, Ji Y, Zhang Z, Tao X (2024) A plant NLR receptor employs ABA central regulator PP2C-SnRK2 to activate antiviral immunity. Nature Communications 15(1), 3205.
| Crossref | Google Scholar |
Jennings SA, Koehler A-K, Nicklin KJ, Deva C, Sait SM, Challinor AJ (2020) Global potato yields increase under climate change with adaptation and CO2 fertilisation. Frontiers in Sustainable Food Systems 4, 519324.
| Crossref | Google Scholar |
Jing Z, Liu N, Zhang Z, Hou X (2024) Research progress on plant responses to stress combinations in the context of climate change. Plants 13(4), 469.
| Crossref | Google Scholar | PubMed |
Kim H, Lee K, Hwang H, Bhatnagar N, Kim D-Y, Yoon IS, Byun M-O, Kim ST, Jung K-H, Kim B-G (2014) Overexpression of PYL5 in rice enhances drought tolerance, inhibits growth, and modulates gene expression. Journal of Experimental Botany 65(2), 453-464.
| Crossref | Google Scholar | PubMed |
Kumar S, Stecher G, Li M, Knyaz C, Tamura K (2018) MEGA X: molecular evolutionary genetics analysis across computing platforms. Molecular Biology and Evolution 35(6), 1547-1549.
| Crossref | Google Scholar | PubMed |
Lamers J, van Der Meer T, Testerink C (2020) How plants sense and respond to stressful environments. Plant Physiology 182(4), 1624-1635.
| Crossref | Google Scholar | PubMed |
Lee SH, Oh SH, Hwang IG, Kim HY, Woo KS, Woo SH, Kim HS, Lee J, Jeong HS (2016) Antioxidant contents and antioxidant activities of white and colored potatoes (Solanum tuberosum L.). Preventive Nutrition and Food Science 21(2), 110-116.
| Crossref | Google Scholar | PubMed |
Lei P, Wei X, Gao R, Huo F, Nie X, Tong W, Song W (2021) Genome-wide identification of PYL gene family in wheat: evolution, expression and 3D structure analysis. Genomics 113(2), 854-866.
| Crossref | Google Scholar | PubMed |
Letunic I, Bork P (2021) Interactive Tree Of Life (iTOL) v5: an online tool for phylogenetic tree display and annotation. Nucleic Acids Research 49(W1), W293-W296.
| Crossref | Google Scholar | PubMed |
Letunic I, Khedkar S, Bork P (2021) SMART: recent updates, new developments and status in 2020. Nucleic Acids Research 49(D1), D458-D460.
| Crossref | Google Scholar | PubMed |
Li Y, Wang Y, Tan S, Li Z, Yuan Z, Glanc M, Domjan D, Wang K, Xuan W, Guo Y, Gong Z, Friml J, Zhang J (2020) Root growth adaptation is mediated by PYLs ABA receptor-PP2A protein phosphatase complex. Advanced Science 7(3), 1901455.
| Crossref | Google Scholar | PubMed |
Liu Z, Li Y, Zhu J, Ma W, Li Z, Bi Z, Sun C, Bai J, Zhang J, Liu Y (2021) Genome-wide identification and analysis of the NF-Y gene family in potato (Solanum tuberosum L.). Frontiers in Genetics 12, 739989.
| Crossref | Google Scholar | PubMed |
Liu S, Lu C, Jiang G, Zhou R, Chang Y, Wang S, Wang D, Niu J, Wang Z (2022) Comprehensive functional analysis of the PYL-PP2C-SnRK2s family in Bletilla striata reveals that BsPP2C22 and BsPP2C38 interact with BsPYLs and BsSnRK2s in response to multiple abiotic stresses. Frontiers in Plant Science 13, 963069.
| Crossref | Google Scholar | PubMed |
Liu Z, Zhang M, Wang L, Sun W, Li M, Feng C, Yang X (2023) Genome-wide identification and expression analysis of PYL family genes and functional characterization of GhPYL8D2 under drought stress in Gossypium hirsutum. Plant Physiology and Biochemistry 203, 108072.
| Crossref | Google Scholar | PubMed |
Ma Y, Szostkiewicz I, Korte A, Moes D, Yang Y, Christmann A, Grill E (2009) Regulators of PP2C phosphatase activity function as abscisic acid sensors. Science 324(5930), 1064-1068.
| Crossref | Google Scholar | PubMed |
Maqsood H, Munir F, Amir R, Gul A (2022) Genome-wide identification, comprehensive characterization of transcription factors, cis-regulatory elements, protein homology, and protein interaction network of DREB gene family in Solanum lycopersicum. Frontiers in Plant Science 13, 1031679.
| Crossref | Google Scholar | PubMed |
Marand AP, Eveland AL, Kaufmann K, Springer NM (2023) cis-Regulatory elements in plant development, adaptation, and evolution. Annual Review of Plant Biology 74, 111-137.
| Crossref | Google Scholar | PubMed |
Mega R, Abe F, Kim J-S, Tsuboi Y, Tanaka K, Kobayashi H, Sakata Y, Hanada K, Tsujimoto H, Kikuchi J, Cutler SR, Okamoto M (2019) Tuning water-use efficiency and drought tolerance in wheat using abscisic acid receptors. Nature Plants 5(2), 153-159.
| Crossref | Google Scholar | PubMed |
Mehari TG, Xu Y, Umer MJ, Shiraku ML, Hou Y, Wang Y, Yu S, Zhang X, Wang K, Cai X, Zhou Z, Liu F (2021) Multi-omics-based identification and functional characterization of Gh_A06G1257 proves its potential role in drought stress tolerance in Gossypium hirsutum. Frontiers in Plant Science 12, 746771.
| Crossref | Google Scholar | PubMed |
Mohammadi F, Naghavi MR, Peighambari SA, Khosravi Dehaghi N, Khaldari I, Bravi E, Marconi O, Perretti G (2021) Abscisic acid crosstalk with auxin and ethylene in biosynthesis and degradation of inulin-type fructans in chicory. Plant Biology 23(4), 636-642.
| Crossref | Google Scholar | PubMed |
Muhammad Aslam M, Waseem M, Jakada BH, Okal EJ, Lei Z, Saqib HSA, Yuan W, Xu W, Zhang Q (2022) Mechanisms of abscisic acid-mediated drought stress responses in plants. International Journal of Molecular Sciences 23(3), 1084.
| Crossref | Google Scholar |
Narsing Rao MP, Lohmaneeratana K, Bunyoo C, Thamchaipenet A (2022) Actinobacteria–plant interactions in alleviating abiotic stress. Plants 11(21), 2976.
| Crossref | Google Scholar | PubMed |
Nasir MW, Toth Z (2022) Effect of drought stress on potato production: a review. Agronomy 12(3), 635.
| Crossref | Google Scholar |
Park S-Y, Fung P, Nishimura N, Jensen DR, Fujii H, Zhao Y, Lumba S, Santiago J, Rodrigues A, Chow T-FF, Alfred SE, Bonetta D, Finkelstein R, Provart NJ, Desveaux D, Rodriguez PL, McCourt P, Zhu J-K, Schroeder JI, Volkman BF, Cutler SR (2009) Abscisic acid inhibits type 2C protein phosphatases via the PYR/PYL family of START proteins. Science 324(5930), 1068-1071.
| Crossref | Google Scholar | PubMed |
Pieczynski M, Wyrzykowska A, Milanowska K, Boguszewska-Mankowska D, Zagdanska B, Karlowski W, Jarmolowski A, Szweykowska-Kulinska Z (2018) Genomewide identification of genes involved in the potato response to drought indicates functional evolutionary conservation with Arabidopsis plants. Plant Biotechnology Journal 16(2), 603-614.
| Crossref | Google Scholar |
Rani V, Singh VK, Joshi DC, Singh R, Yadav D (2024) Molecular docking insights into nuclear factor Y (NF-Y) transcription factor and pyrabactin resistance 1 (PYL) receptor proteins reveal abiotic stress regulation in finger millet. Crop Design 3(1), 100051.
| Crossref | Google Scholar |
Satheesh V, Chidambaranathan P, Jagannadham PT, Kohli D, Jain PK, Bhat SR, Srinivasan R (2014) A polyketide cyclase/dehydrase and lipid transport superfamily gene of Arabidopsis and its orthologue of chickpea exhibit rapid response to wounding. Indian Journal of Genetics and Plant Breeding 74, 463-470.
| Crossref | Google Scholar |
Shahzad A, Qian M, Sun B, Mahmood U, Li S, Fan Y, Chang W, Dai L, Zhu H, Li J, Qu C, Lu K (2021) Genome-wide association study identifies novel loci and candidate genes for drought stress tolerance in rapeseed. Oil Crop Science 6(1), 12-22.
| Crossref | Google Scholar |
Shahzad A, Gul H, Ahsan M, Wang D, Fahad S (2023a) Comparative genetic evaluation of maize inbred lines at seedling and maturity stages under drought stress. Journal of Plant Growth Regulation 42(2), 989-1005.
| Crossref | Google Scholar |
Shahzad A, Shahzad M, Imran M, Gul H, Gul S (2023b) Genome wide identification and expression profiling of PYL genes in barley. Plant Gene 36, 100434.
| Crossref | Google Scholar |
Shen B (2003) Polyketide biosynthesis beyond the type I, II and III polyketide synthase paradigms. Current Opinion in Chemical Biology 7(2), 285-295.
| Crossref | Google Scholar | PubMed |
Siano AB, Roskruge N, Kerckhoffs H, Sofkova-Bobcheva S (2024) Effects of abiotic stress associated with climate change on potato yield and tuber quality under a multi-environment trial in New Zealand. Potato Research 6, 1-22.
| Google Scholar |
Singh B, Salaria N, Thakur K, Kukreja S, Gautam S, Goutam U (2019) Functional genomic approaches to improve crop plant heat stress tolerance. F1000Research 8, 1721.
| Google Scholar |
Sinha M, Singh RP, Kushwaha GS, Iqbal N, Singh A, Kaushik S, Kaur P, Sharma S, Singh TP (2014) Current overview of allergens of plant pathogenesis related protein families. The Scientific World Journal 2014, 543195.
| Crossref | Google Scholar | PubMed |
Soma F, Mogami J, Yoshida T, Abekura M, Takahashi F, Kidokoro S, Mizoi J, Shinozaki K, Yamaguchi-Shinozaki K (2017) ABA-unresponsive SnRK2 protein kinases regulate mRNA decay under osmotic stress in plants. Nature Plants 3(1), 16204.
| Crossref | Google Scholar |
Tiwari JK, Buckseth T, Zinta R, Bhatia N, Dalamu D, Naik S, Poonia AK, Kardile HB, Challam C, Singh RK, Luthra SK, Kumar V, Kumar M (2022) Germplasm, breeding, and genomics in potato improvement of biotic and abiotic stresses tolerance. Frontiers in Plant Science 13, 805671.
| Crossref | Google Scholar | PubMed |
Wang G, Qi K, Gao X, Guo L, Cao P, Li Q, Qiao X, Gu C, Zhang S (2022) Genome-wide identification and comparative analysis of the PYL gene family in eight Rosaceae species and expression analysis of seeds germination in pear. BMC Genomics 23(1), 233.
| Crossref | Google Scholar | PubMed |
Wang J, Chitsaz F, Derbyshire MK, Gonzales NR, Gwadz M, Lu S, Marchler GH, Song JS, Thank N, Yamashita RA, Yang M, Zhang D, Zheng C, Lanczycki CJ, Marchler-Bauer A (2023) The conserved domain database in 2023. Nucleic Acids Research 51(D1), D384-D388.
| Crossref | Google Scholar | PubMed |
Wang Z, Zhou J, Zou J, Yang J, Chen W (2024) Characterization of PYL gene family and identification of HaPYL genes response to drought and salt stress in sunflower. PeerJ 12, e16831.
| Crossref | Google Scholar | PubMed |
Wu X, Zhu J, Chen X, Zhang J, Lu L, Hao Z, Shi J, Chen J (2023) PYL family genes from Liriodendron chinense positively respond to multiple stresses. Plants 12, 2609.
| Crossref | Google Scholar | PubMed |
Yadav SK, Santosh Kumar VV, Verma RK, Yadav P, Saroha A, Wankhede DP, Chaudhary B, Chinnusamy V (2020) Genome-wide identification and characterization of ABA receptor PYL gene family in rice. BMC Genomics 21, 676.
| Crossref | Google Scholar |
Yanai I (2022) Quantifying gene duplication. Nature Reviews Genetics 23(4), 196-197.
| Crossref | Google Scholar | PubMed |
Yin K, Cheng F, Ren H, Huang J, Zhao X, Yuan Z (2024) Insights into the PYR/PYL/RCAR gene family in pomegranates (Punica granatum L.): a genome-wide study on identification, evolution, and expression analysis. Horticulturae 10(5), 502.
| Crossref | Google Scholar |
Zaidi F, Shahzad A, Ahsan M, Gul H, Shahzad M, Gul S, Mohamed S (2022) Evaluation of genetic variation among maize inbred lines for salinity stress at seedling stage through salt-stress-responsive traits. Acta Universitatis Sapientiae, Agriculture and Environment 14(1), 62-84.
| Crossref | Google Scholar |
Zhang H, Fen X, Yu W, Hu H-H, Dai X-F (2017) Progress of potato staple food research and industry development in China. Journal of Integrative Agriculture 16(12), 2924-2932.
| Crossref | Google Scholar |
Zhang G, Tang R, Niu S, Si H, Yang Q, Rajora OP, Li X-Q (2021) Heat-stress-induced sprouting and differential gene expression in growing potato tubers: comparative transcriptomics with that induced by postharvest sprouting. Horticulture Research 8, 226.
| Crossref | Google Scholar |
Zhang Z, Luo S, Liu Z, Wan Z, Gao X, Qiao Y, Yu J, Zhang G (2022a) Genome-wide identification and expression analysis of the cucumber PYL gene family. PeerJ 10, e12786.
| Crossref | Google Scholar | PubMed |
Zhang H, Zhu J, Gong Z, Zhu J-K (2022b) Abiotic stress responses in plants. Nature Reviews Genetics 23(2), 104-119.
| Crossref | Google Scholar | PubMed |
Zhang F, Yang J, Zhang N, Wu J, Si H (2022c) Roles of microRNAs in abiotic stress response and characteristics regulation of plant. Frontiers in Plant Science 13, 919243.
| Crossref | Google Scholar | PubMed |
Zhao Y, Chan Z, Gao J, Xing L, Cao M, Yu C, Hu Y, You J, Shi H, Zhu Y, Gong Y, Mu Z, Wang H, Deng X, Wang P, Bressan RA, Zhu J-K (2016) ABA receptor PYL9 promotes drought resistance and leaf senescence. Proceedings of the National Academy of Sciences 113(7), 1949-1954.
| Crossref | Google Scholar |
Zhao H, Nie K, Zhou H, Yan X, Zhan Q, Zheng Y, Song C-P (2020) ABI5 modulates seed germination via feedback regulation of the expression of the PYR/PYL/RCAR ABA receptor genes. New Phytologist 228(2), 596-608.
| Crossref | Google Scholar | PubMed |
Zhou J, An F, Sun Y, Guo R, Pan L, Wan T, Hao Y, Cai Y (2023) Genome-wide identification of the ABA receptor PYL gene family and expression analysis in Prunus avium L. Scientia Horticulturae 313, 111919.
| Crossref | Google Scholar |
Zhu L, Yan K, Ren J, Chen Z, Ma Q, Du Y, Wang Y, Li S, Li Q (2024) Genome-wide investigation of the PYL genes in Acer palmatum and their role in freezing tolerance. Industrial Crops and Products 210, 118107.
| Crossref | Google Scholar |
Zuo Z-F, He W, Li J, Mo B, Liu L (2021) Small RNAs: the essential regulators in plant thermotolerance. Frontiers in Plant Science 12, 726762.
| Crossref | Google Scholar | PubMed |