Genomic blueprints of soybean (Glycine max) pathogen resistance: revealing the key genes for sustainable agriculture
Aiman Hina



A
B
C
D
E
F
G
H
I
J
K
Abstract
Soybean (Glycine max) is an important oilseed, protein and biodiesel crop. It faces significant threats from bacterial, fungal and viral pathogens, which cause economic losses and jeopardises global food security. In this article, we explore the relationship between soybeans and these pathogens, focusing on the molecular responses that are crucial for soybeans defence mechanisms. Molecular responses involve small RNAs and specific genes, including resistance (R) genes that are pivotal in triggering immune responses. Functional genomics, which makes use of cutting-edge technologies, such as CRISPR Cas9 gene editing, allows us to identify genes that provide insights into the defence mechanisms of soybeans with the focus on using genomics to understand the mechanisms involved in host pathogen interactions and ultimately improve the resilience of soybeans. Genes like GmKR3 and GmVQ58 have demonstrated resistance against soybean mosaic virus and common cutworm, respectively. Genetic studies have identified quantitative trait loci (QTLs) including those linked with soybean cyst nematode, root-knot nematode and Phytophthora root and stem rot resistance. Additionally, resistance against Asian soybean rust and soybean cyst nematode involves specific genes and their variations in terms of different copy numbers. To address the challenges posed by evolving pathogens and meet the demands of a growing population, accelerated soybean breeding efforts leveraging functional genomics are imperative. Targeted breeding strategies based on a deeper understanding of soybean gene function and regulation will enhance disease resistance, ensuring sustainable agriculture and global food security. Collaborative research and continued technological advancements are crucial for securing a resilient and productive agricultural future.
Keywords: CRISPR Cas9, food security, pathogen resistance genes, plant defence, R genes, soybean cyst nematode, soybean genomics, small RNA.
Introduction
Soybean (Glycine max) has emerged as a crucially significant oilseed, protein source and biodiesel crop, providing extensive oil and protein for human food along with animal feed (Tao 2007; Rani et al. 2023a). Numerous pathogens such as viruses, nematodes, fungi and bacteria can cause devastating diseases in soybean, resulting in considerable economic losses for farmers worldwide. Plant–insect interactions are influenced by pathogens, altering plant characteristics that serve as indicators for herbivorous insects. These alterations can include changes in colour, volatile compounds, size and growth patterns (Roth et al. 2020; Ansari et al. 2023). The effects of pathogens on plant–insect interactions are often predictable, and understanding plant responses to different pathogen species is crucial to establish a relationship between pathogen life cycles, plant reactions and the resulting impact on plant fitness (Wrather and Koenning 2006). In response to pathogenic attacks, plants deploy an intricate network of signalling and response mechanisms to defend against biotic stress. These defence mechanisms involve physiological responses, such as modification of stomatal guard cell movement, alterations in plant cell walls, modulation of water transport and induction of programmed cell death. Additionally, plants mount molecular responses, involving the activation of specific genes and small RNAs, to combat pathogens effectively (Razzaq et al. 2023a). To effectively enhance the ability of plants to withstand threats, it is vital to understand the dynamics between plants and pathogens at the molecular level. State of the art genomic and functional genomics methods have become increasingly important in our efforts to combat these threats and boost soybean resistance. Functional genomics, which includes transcriptomics, proteomics, metabolomics and genome editing plays a role in unravelling the mechanisms behind interactions, between soybeans and pathogens (Du et al. 2023). By providing pathways for viruses to enter the body or by serving as vectors, insects can function as a secondary source of disease transmission by moving pathogens from one host to another (Che et al. 2023). Crop yields are significantly reduced when soybeans are exposed to several diseases and pests. For example, the United States had a significant decline in soybean production from 2010 to 2014, with an average loss of almost 11.5 million tons (Allen et al. 2017). The four main domains of notable soybean pathogens are: (1) oomycetes; (2) fungus; (3) bacteria; and (4) nematodes. However, the potential threats to soybean production may differ based on the region of cultivation (Allen et al. 2017). These encompass the charcoal rot, seedling diseases and soybean cyst nematode. However, important soybean diseases such as sudden death syndrome (SDS), Phytophthora stem and root rot, and Sclerotinia stem rot are mostly influenced by environmental factors. The occurrence of disease in a crop necessitates the presence of three components in the disease triangle: (1) a susceptible host; (2) a virulent pathogen; and (3) a favourable environment (Roth et al. 2020).
Through the understanding of the intricate molecular mechanisms underpinning host–pathogen interactions, functional genomics plays a critical role in improving soybean resistance against infections (Razzaq et al. 2023a) (Table 1). Despite attempts to improve cultivars of soybeans, diseases persist in adapting and evading plant defence systems. Therefore, to create comprehensive and long-lasting resistance to harmful infections, it is essential to discover novel resistance genes and defensive signalling networks. The identification and modification of genes implicated in plant defence and disease resistance responses has been made easier by recent developments in functional genomics, such as RNA interference (RNAi) and virus-induced gene silencing (VIGS) (Akbar et al. 2022). Understanding soybean gene activity and regulation has greatly benefited from functional genomics, which has made it possible to develop focused breeding techniques for improved disease resistance and whole crop protection (Liu et al. 2023). By using cutting-edge genomic and molecular methods, scientists may pinpoint and define important genes linked to soybean disease resistance. Knowing how these genes express themselves and work during pathogen attacks provides important information on how different diseases affect soybeans (Zubaidah et al. 2023). In addition, functional genomics makes it easier to identify putative target genes for genome editing or genetic engineering, which can strengthen soybean resistance (Razzaq et al. 2023a). Functional genomics sheds light on the intricate genetic relationships between pathogens and soybeans, paving the way for the creation of creative solutions aimed at reducing the negative effects of infections on soybean crops and, eventually, promoting food security and sustainable agriculture. In this review article, we examine the physiological and molecular features of plant responses to infections and discuss the potential use of functional genomics in the fight against diseases that affect soybeans. We also examine the possibility of modifying plant defence systems in the future to improve soybean immunity.
Pathogen resistance mechanism | Description | Examples | References | |
---|---|---|---|---|
Physical traits | Thickened cell walls inhibit pathogen penetration. | Cell wall fortification due to deposition of lignin and callose. | Jones and Dangl (2006) | |
Presence of trichomes physically deter pathogens. | Trichome density and morphology affecting pathogen attachment and movement. | Li et al. (2017) | ||
Biochemical pathways | Activation of defense-related enzymes such as chitinases and glucanases degrade pathogen cell walls. | Increased activity of chitinase and glucanase enzymes upon pathogen attack. | van Loon et al. (2006) | |
Production of antimicrobial compounds like phytoalexins inhibits pathogen growth. | Synthesis of phytoalexins such as glyceollins and isoflavonoids upon pathogen perception. | Dixon et al. (2002) | ||
Genetic factors | Presence of resistance (R) genes encoding proteins that recognise and trigger defence responses against specific pathogen avirulence (Avr) genes. | Rpg1-b gene conferring resistance against soybean rust (Phakopsora pachyrhizi). | Flor (1971) | |
Activation of systemic acquired resistance (SAR) through gene expression changes upon pathogen recognition. | Induction of SAR-related genes such as PR1 and NPR1 following pathogen infection. | Durrant and Dong (2004) | ||
Expression of pathogenesis-related (PR) proteins involved in defence signalling pathways. | Upregulation of PR proteins like PR10 and PR5 involved in defence responses. | Van Loon and Van Strien (1999) | ||
Regulation of transcription factors controlling defence gene expression. | Activation of transcription factors such as WRKY and ERF families in response to pathogen attack. | Rushton et al. (2010) | ||
Epigenetic modifications influencing gene expression in response to pathogen stress. | DNA methylation and histone modifications altering gene expression patterns during pathogen infection. | Dowen et al. (2012) |
Plant reactions against pathogens
Various signalling and response networks within plants contribute to their resilience against stress, offering an intricate mechanism to prevent pathogen attacks (Leibman-Markus et al. 2023) (Table 1). Biotic stress has the ability to cause epigenetic modifications to DNA and histone levels, which can impact resistance and signal modifications via mechanisms such DNA methylation, histone modification and short non-coding RNAs (sncRNAs) (Garcion et al. 2014). Among the many defence strategies plants use to fend off infections is the generation of reactive oxygen species (ROS) (Dumanović et al. 2021), H2O2 accumulation (Feng et al. 2022), cell wall lignification and suberisation at infected areas (Garcion et al. 2014), and the expression of pathogenesis-related (PR) protein genes (Alizadeh et al. 2021). WRKY proteins, chitinases (PR-3, PR-4, PR-8 and PR-11), β −13-glucanase (PR-2), proteinase inhibitors (PR-6), ribonuclease-like (PR-10), NBS-LRR protein, catalases, thaumatin (PR-5), glycoproteins, defensin (PR-12), peroxidase (PR-9), lipid-transfer protein (PR-14) are among the defense-related proteins that sugarcane displays in response to biotic stress (Souza et al. 2017). Plants possess natural immune system and an adaptive immune response towards multiple molecular patterns associated with pathogen/microbe (PAMPs/MAMPs), activating pattern-triggered immunity (PTI), effector-triggered immunity (ETI) via nucleotide-binding domain leucine-rich repeat-containing receptors (NLRs) (Saur et al. 2021; Benjamin et al. 2022; Yang et al. 2022). Plant responses to pathogenic attack can be divided into physiological and molecular responses as follows.
Plant physiological responses to pathogens
Plants exhibit a diverse array of physiological responses when faced with pathogen attacks. For instance, these processes involve movement of stomatal guard cells, plant cell walls modification, water transport modulation, translocation of photo assimilates, programmed cell death induction, and regulation of metal homeostasis, hypertrophy and hyperplasia (Fig. 1).
Overview of general physiological response of plants to pathogens. These responses collectively contribute to the plant’s ability to resist and defend against pathogen attacks.
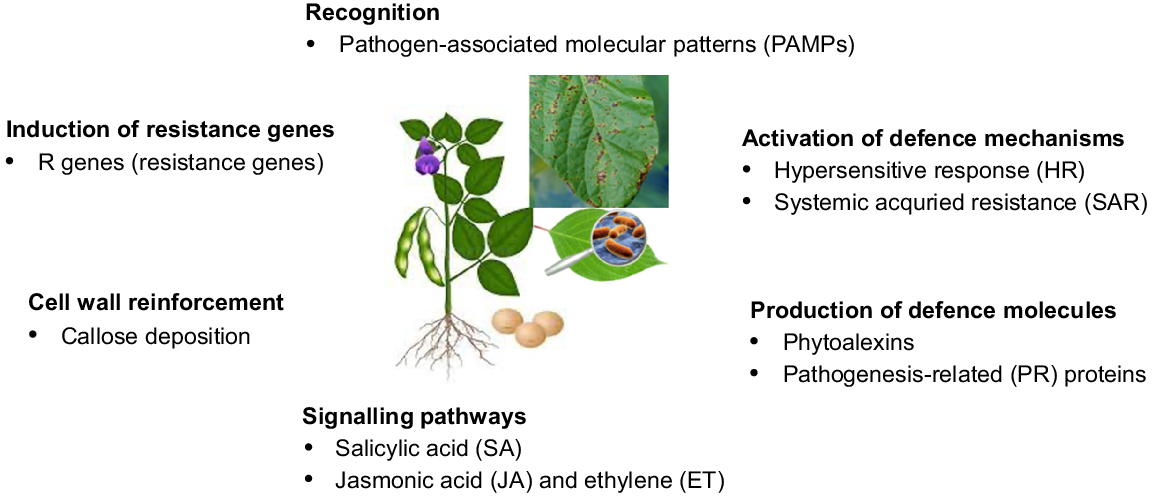
The movement of stomatal guard cells is a crucial aspect of plant defence mechanisms. For instance, stomatal opening provides a significant entry point for bacterial infiltration into a host plant by ensuring frequent water and gaseous exchange. Plants have developed a mechanism to mitigate bacterial penetration by inducing pathogen-induced molecular pattern (PAMP)-triggered stomatal closure. However, plant pathogens have evolved strategies to counter this defence mechanism by targeting various regulators and processes. For instance, they interfere with salicylic acid (SA) signalling, the mitogen-activated protein kinase 3 (MPK3) pathway, OST1 (open stomata 1) kinase and abscisic acid (ABA) signalling, proteasome-mediated protein degradation, proton pump, K+ accumulation, and the breakdown of starch in guard cells (Guimarães and Stotz 2004; Melotto et al. 2006; Gudesblat et al. 2009a, 2009b; Geng et al. 2014; Lozano-Durán et al. 2014). These actions either prevent stomatal closure or induce their reopening. In particular, Pseudomonas syringae can stimulate stomatal opening during the entry phase of infection followed by stomatal closure after entering the internal host for water conservation (Goel et al. 2008; Freeman and Beattie 2009).
The plant cell wall (PCW) functions both as an obstacle against microbes and as a sensing top crucial for perceiving pathogens. Recognition of pathogens initiates a range of host plant responses, enhancing the antipathogenic properties of PCWs (Underwood 2012). However, certain reactions related to the PCW might render plants more susceptible to biotic stressors, representing Stressful Reactions (SRs). Phytopathogens typically need for structural modification and characteristics of the PCW to successfully infect the host plant. Given the significant variability in the polysaccharides forming the bulk of this compartment, this modification process is highly intricate. While the fundamental types of PCW polysaccharides are relatively few (approximately 10), the fine structure of a specific polysaccharide and functional traits can be tailored to specific physiological roles, such as developmental stage or cell type (Albersheim et al. 2010).
Wilting, a common symptom of plant infectious diseases, was traditionally attributed to the plant’s vascular system impediment by exopolysaccharides (i.e. microbial metabolites and cells). Hence, it has been observed that in diseased plants, the quantity of bacterial biomass is typically not substantial enough to significantly impede the conductive elements, resulting in majority of xylem vessels free from bacteria (Sun et al. 2013). Recent research has revealed that the obstruction in the vascular system and drooping in infectious plants could be one of the plant’s responses, particularly the tyloses and gels formation (Ellendorff et al. 2009; Klosterman et al. 2009; Beattie 2011; Sun et al. 2013). The extensions of parenchyma cells known as tyloses are generally extend through pit membranes inside the vascular system, resulting in their blockage. Both, tyloses and gels act to hinder the pathogens production through the vascular system, thereby serving as defence structures. Nevertheless, it is important to note that the effectiveness of tyloses could vary in susceptible and resistant plants (Sun et al. 2013; Yadeta and Thomma 2013; Wang et al. 2015). Further, maximum prevalence of tyloses have been reported in susceptible plants compared to resistant varieties (Sun et al. 2013). Also, pathogens can induce water transport-related stress responses in the outer tissues of the host plant in addition to vascular system. For instance, the Pseudomonas and Xanthomonas species prompt host plants for maximum water absorption from leaf top (Xin et al. 2016; Aung et al. 2018). Increased water assimilation by leaves is believed to be caused by a pathogen-driven rise in the plasma membrane water permeability and hygroscopicity of PCWs.
Plant molecular responses to pathogens
Biotic threats to food security encompass a range of microorganisms (bacteria, fungi, oomycetes, nematodes, insect pests and viruses) (Bebber and Gurr 2015). Globally, these pathogens collectively contribute to approximately a 30% reduction in crop yield both before and after harvesting of crop (Liu et al. 2017). Plant pathogens persistently challenge the host defence mechanisms (Fig. 2) (Liu et al. 2017). To effectively combat various infection types, plants have developed a diverse set of immune responses by modulating extensive genetic diversity (Liu et al. 2017; Noman et al. 2017; Zaynab et al. 2017). Following occurrences of pathogen attacks, plants detect host pathogen-associated molecular patterns (a sequence of PAMPs) (Santoni et al. 2015; Dana et al. 2017; Liston and Masters 2017). To recognises specific molecular patterns associated with pathogens, plants have specific receptors. When a plant detects these patterns, it triggers an initial response (McLachlan et al. 2014). Majority of the receptors situated in the plasma membrane identify PAMPs, initiating a phosphorylation cascade upon binding, ultimately triggering the onset of early basal resistance (Grennan 2006). Majority of the pattern-recognition receptors (PRRs) located on the cell surface (EF-Tu, FLS2, CERK1 and LYK5) recognise PAMPs or DAMPs triggered by pathogens like bacteria and fungi (Park et al. 2012; Newman et al. 2013; Li et al. 2014; Trdá et al. 2015). These DAMPs and PAMPs initiate PAMP-triggered immunity (PTI), leading to the activation of pathogenesis-related (PR) gene expression, callose accumulation, the reactive oxygen species (ROS) generation, and salicylic acid deposition (Jwa and Hwang 2017; Withers and Dong 2017). However, throughout evolution, majority of pathogens have developed functional proteins to suppress PTI, ultimately resulting in ETS (de Wit 2016; Schuebel et al. 2016; Gouveia et al. 2017).
Summary of plant molecular responses to pathogens. These molecular responses collectively form a sophisticated defence system that helps plants recognise and combat pathogens. The specific response can vary depending on the type of pathogen, the plant species and the specific signalling pathways involved.
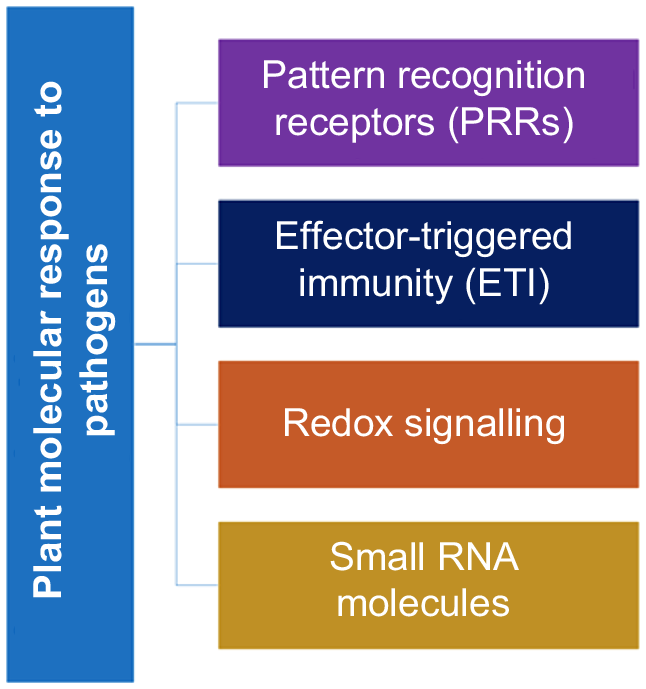
Various phytopathogens (bacteria, fungi, mycoplasma, nematodes, viruses, viroids and parasites) cause significant damage to crop production, resulting in substantial economic losses (Zaynab et al. 2017). Different categories of plant small RNAs (sRNAs) contribute to plant defence mechanisms in resistance to disease causing agents (Fig. 2). These sRNAs (miRNA and siRNA) regulate plant immunity by addressing PAMPs and other functional proteins (Martinez and Köhler 2017). The up- or downregulation of sRNAs in response to pathogen attack leads to the target site expression suppression (Huang et al. 2016). However, there is still a need for extensive research on the regulation of several sRNAs. Multiple proteins participate in sRNA pathways to initiate a successful immunity response against harmful microorganism via synthesis and role of sRNA. Such proteins comprise endoribonuclease (DCL), argonaute (AGOs) and RNA-dependent RNA polymerase (RDRs) are involved in sRNA production, sRNA-directed gene suppression and the genesis of double-stranded RNA (dsRNA) precursors, respectively (Islam et al. 2017). The Arabidopsis thaliana genome encodes 04 proteins, with DCL1 being a vital player in miRNA genesis. Numerous miRNAs are associated with ETI and PTI against bacterial and fungal diseases. Two mutants of Arabidopsis, dcl1-9 (Zhang et al. 2016) and dcl1-7 (Weiberg et al. 2013), were considered susceptible to pathogens, highlighting the role of miRNAs in defence mechanism regulation. Other proteins (DCL4) are responsible for generation of siRNA and are crucial for defence against pathogens like bacteria, fungi and viruses (Nicaise 2014). AGOs also play a significant role in regulating the immunity responses. In Arabidopsis, for instance, bacterial infection leads to AGO2 generation. Few mutants such as ago2-1 in tomato (Solanum lycopersicum), embedding miRNA393, show considerable susceptibility to various strains of P. syringae (Zhang et al. 2011). Interestingly, miRNA393 operates through AGO1 to suppress auxin receptors, activating anti-bacterial immunity (Zvereva and Pooggin 2012). Likewise, AGO1-25 and AGO1-27 demonstrate enhanced immunity in response to bacterial and fungal infection (Peláez and Sanchez 2013). The Arabidopsis genome holds 6 RDRs, among which RDR6 generates secondary siRNA (ta-siRNA), this rdr6 mutant is increasingly vulnerable to fungi (Ellendorff et al. 2009) but resistant to Pseudomonas strains (Katiyar-Agarwal et al. 2006). Moreover, mutations at the interface of RDR6 and antiviral proteins (SGS3) heighten infections susceptibility of Verticillium (Ellendorff et al. 2009). Thus, it is evident that sRNA pathways play a crucial role in anti-fungal defence mechanisms of plants.
Revolutionising soybean pathogen defence through functional genomics approaches
Recently, proteomics and genome-wide transcriptomics have been used to discover differentially expressed genes (DEGs) and proteins involved in plant defence mechanisms by downregulating gene expression using post-transcriptional gene silencing (PTGS) technologies such as RNAi and VIGS, allowing for hypothesis-based experiments and gene function association with plant phenotype (Senthil-Kumar and Mysore 2011; Kandoth et al. 2013). One of the valuable applications of gene-silencing technology is silencing many copies of a gene at once, especially for plants with multiple ploidy levels (polyploid). Generally, VIGS is widely used for massive-scale screening, which makes it one of the potent and flexible methods for soybean functional genomic analysis. In soybean, VIGS has made use of several viral systems, including bean pod mottle virus (BPMV) (Zhang et al. 2010) and Apple latent spherical virus (ALSV) (Zhang et al. 2009). This review reflects on recent developments in functional genomics of soybean defence and disease resistance mechanism (Tables 1 and 2) coupled with responses and the potential for future manipulation of soybean immunity.
Determining genes of resistance against the soybean mosaic virus (SMV)
The plant virus Soybean mosaic virus (SMV) belongs to the Potyvirus genus in the Potyviridae family. Approximately 30% of known plant viruses worldwide are members of the Potyviridae family, which includes viruses that are important to the economy and impact cash crops (Tolin 1999; Gunduz et al. 2004; King et al. 2012; Yang et al. 2021). SMV is transmitted by aphids during the growing season, substantially impacting crop productivity in many Asian soybean-producing countries, such as China, Korea and Indonesia (Wrather et al. 2001a; Hwang et al. 2006).
So far, four distinct loci (Rsv1, Rsv3, Rsv4 and Rsv5) are linked to SMV resistance (Kiihl and Hartwig 1979; Buzzell and Tu 1989; Buss et al. 1997; Klepadlo et al. 2017b). While most resistant soybean germplasm either has one dominant gene or more (Zheng et al. 2005; Li et al. 2010), may possess two resistance genes (Liao et al. 2002; Ma et al. 2002; Zheng et al. 2006). An exception is the landrace ‘8101’ from South Korea, which carries three SMV R-genes (Liao et al. 2011) (Table 2).
Understanding the signalling pathways responsible for SMV resistance in soybeans is not fully elucidated. One study employed microarray technology to observe changes in gene expression in the SMV-susceptible Williams 82 (W82) genome infiltrated by strain of SMV-G2 (Babu et al. 2008). Moreover, a research investigation employed analyses of small RNAs, degradome, and transcriptome sequencing to detect variations in gene expression and microRNAs in W82 infected by three distinct SMV isolates. Additionally, PI 96983, which is resistant or necrotic to SMV (Rsv1), was examined when infected by SMV-G7 strain (Chen et al. 2016). Recent molecular analyses indicate diverse mechanisms leading to resistance breakdown for multiple genes, requiring frequent genetic shifts in multi-viral proteins facilitating SMV to establish virulence against various genes carrying resistance (Chowda-Reddy et al. 2011a, 2011b). The emergence of a more potent SMV variant capable of overcoming all identified resistance genes is deemed highly dubious. Therefore, pyramiding resistance genes (R-genes), as demonstrated by the stacking of three R-genes using marker-assisted selection (MAS), has become a viable option in breeding programs (Saghai Maroof et al. 2008; Cervantes-Martinez et al. 2015; Klepadlo et al. 2016).
Determining genes that resist the southern root-knot nematode (RKN)
The root-knot nematode (RKN; Meloidogyne spp.) is ubiquitous and destructive plant parasitic nematode (PPN) (Jones et al. 2013; Machado 2014). RKNs exhibit a broad host range, impacting various agricultural crops and wild plants (Dutta et al. 2015; Abd-Elgawad 2022). Meloidogyne species have been found to attack over 3000 plant species, causing annual losses amounting to billions of dollars (Gowda et al. 2007; Forghani and Hajihassani 2020). Transcriptomic analysis has played a crucial role in advancing our insights into the molecular pathways underlying the parasitism of PPN (Jacob and Mitreva 2011). By investigating the transcriptome, we have gained insights into individual resistance genes associated with various stages of the defence system. This approach provides valuable information about metabolic processes and gene expression that undergo differential regulation during plant–pathogen dynamics (Samac et al. 2011; Barilli et al. 2014). Utilising a homology database, researchers compared transcriptome data from Coffea arabica, G. max, Oryza glaberrima and Arachis stenosperma; all possessing resistant RKNs when infected with three different Meloidogyne species (Beneventi et al. 2013; Petitot et al. 2017).
RNA interference has manifested as a promising method for managing PPNs. The top-notch approach for promoting nematode resistant trait in plants involves targeting nematode genes and engages both nematode RNAi machinery and plant, referred host-mediated RNAi (Joshi et al. 2022). RKNs are known to secrete protein effectors to manipulate host reactions and facilitate successful infections. RNAi gene silencing, achieved by exposing nematodes to dsRNA, hampers the specific nematode gene modification, leading to the suppression of parasitic success. RNAi of PPN genes was demonstrated by applying this strategy successfully to cyst nematodes (Globodera pallida and Heterodera glycines) (Urwin et al. 2002). In a groundbreaking study, RNAi was used on Meloidogyne incognita J2s to silence two genes (Mi-pg1 and Mi-crt) (Rosso et al. 2005). Likewise, by targeting four different genes with RNAi constructs, their ability to diminish galls in soybean roots induced by M. incognita was evaluated. Remarkably, two constructs designed for mitochondrial stress-70 protein precursor (MSP) genes and tyrosine phosphatase (TP) were reported to effectively impeded M. incognita gall formation. These results highlight the potential to enhance soybean plant resistance against root-knot nematodes through the silencing of TP and MSP genes (Ibrahim et al. 2011).
Quantitative trait loci (QTL) introgression into improved varieties, particularly through biparental populations, allows for the analysis of genetic factors influencing resistance efficacy without compromising other important crop traits. In soybean, dissecting QTL associated with RKN resistance, three distinct QTL were identified; the primary QTL was precisely mapped on chromosome 10 to a specific region of 29.7-kilobase (Xu et al. 2013). A GmVQ58 gene encoding VQ motif-containing protein, was identified to boost resistance against the common cutworm in soybean (Li et al. 2020) (Table 2).
Determining genes of resistance against Phytophthora
Phytophthora disease, induced by Phytophthora sojae that causes root and stem rot poses a significant threat and causing extensive damage to soybean. There are two main processes that control soybean resistance to P. sojae; either: (1) complete; or (2) partial resistance (Dorrance et al. 2003; Sugimoto et al. 2012). With complete resistance, there is just one dominant resistant gene at play. P. sojae interacts with Rps genes gene-for-gene to stop disease from spreading (Niu et al. 2017; Zhong et al. 2018a, 2018b, 2019). In contrast, partial resistance entails the contribution of multiple genes, working together to limit plant damage (Schmitthenner 2000; Dorrance et al. 2003). In soybean, the identification and mapping of the dominant RpsWY (Phytophthora root rot resistance gene) were achieved using a high-density genetic map (Cheng et al. 2017). In another study, increased expression of GmERF5, responsible for ethylene response factor 5, or GmMYB29A2, a glyceollin transcription factor, resulted in a significant enhancement of resistance against P. sojae. Additionally, upregulation of certain microRNAs, including miR393, demonstrated the potential to bolster soybean defence mechanisms against P. sojae (Dong et al. 2015; Jahan et al. 2020; Jiang et al. 2022).
Identifying R-genes revealing targets for durable pathogen resistance
In the past 15 years, researchers in the field of soybeans have employed diverse genetic and genomic strategies to comprehend how soybeans react to various pathogens. The unveiling of the W82 reference genome sequence linked with the genetic map, made it easy for researchers hundreds of sequences for R-gene loci (Schmutz et al. 2010). An examination of the W82 genome revealed 319 nucleotide binding site-leucine rich repeat (NBS-LRR) genes (Kang et al. 2012), representing the prevalent type of plant R-genes, which functions in triggering targeted resistance responses that prove effective against a wide array of pathogens (Razzaq et al. 2022). It is reported that in soybean, almost half of the NBS-LRR genes are located on 6/20 chromosomes, and a significant number of disease resistance QTLs are found within genetic intervals possessing NBS-LRR genes. Despite the availability of the soybean genome sequence, the cloning of R-genes poses challenges. Being a paleopolyploid, soybean demonstrate approximately 75% of its genes in duplicates (Schmutz et al. 2010). Additionally, the analysis of R-gene loci in resistant germplasm indicates substantial divergence in copy number among soybean lines showing resistance and susceptibility, overlooked by the reference genome (Meyer et al. 2009; Cook et al. 2012; McHale et al. 2012).
In soybean genome, a total of 319 NBS-LRR R-genes were estimated (Kang et al. 2012). For providing immunity to Phakopsora pachyrhizi, the GmRpp1 (ULP1-NBS-LRR) gene was identified (Pedley et al. 2019). Additionally, the overexpression of the gene GmKR3 (TIRNBS–LRR R) was found to enhance soybean resistance against various SMV strains, a prevalent viral disease, potentially leading to significant reductions in yield losses (Xun et al. 2019). The importance of specific genes in pathogen resistance was underscored by the study on GmNDR1 homologues, where their silencing was shown to be essential for effective resistance against pathogens (Selote et al. 2014). Notably, plants with silenced GmMPK4 and GmMPK6 exhibited robust phenotypes, including elevated levels of salicylic acid and the induction of PR gene expression (Liu et al. 2011, 2014). Furthermore, the manipulation of pathogen avirulence effector gene expression through genome editing was found to impact the compatibility of plant disease, offering insights for enhancing disease resistance in crop (Ochola et al. 2020). In another study, four prominent loci associated with SMV resistance (Rsv1, Rsv3, Rsv4 and Rsv5) were identified (Liu and Murray 2016; Klepadlo et al. 2017b)
Identifying resistance genes against Asian soybean rust (ASR)
P. pachyrhizi, the causative agent of Asian soybean rust (ASR), is non-necrotrophic fungus capable of infecting a multiple leguminous host. Initially identified in the early 1900s in the eastern hemisphere, this pathogen has since extended its presence to every primary soybean exporting country worldwide. The onset of the disease has the potential to cause significant yield losses, with estimates reaching as high as 80% (Ogle et al. 1979; Patil et al. 1997). Studies reported six primary and dominant loci conferring resistance to ASR: Rpp1, Rpp2, Rpp3, Rpp4, Rpp5 and Rpp6 (Cheng and Chan 1968; Singh 1977; Hidayat and Somaatmadja 1979; Bromfield and Hartwig 1980; McLean and Byth 1980; Hartwig and Bromfield 1983; Hartwig 1986; Monteros et al. 2007; Garcia et al. 2008; Li et al. 2012; Pedley et al. 2019) (Table 2). Prior to the release of the reference genome, Meyer et al. (2009) utilised the Rpp4 mapping markers to construct and genetic sequencing on a bacterial artificial chromosome assembly from W82 (susceptible to ASR) (Silva et al. 2008). In the case of Rpp2 and Rpp4, the compatible and incompatible interactions were attained by infecting both susceptible and resistant genotypes with identical P. pachyrhizi variants (van de Mortel et al. 2007; Morales et al. 2013). Regarding Rpp3, the identical soybean genotype carrying Rpp3 underwent inoculation with either virulent or avirulent P. pachyrhizi strains. High-throughput proteomics has been employed to explore how susceptible and resistant soybean plants respond to P. pachyrhizi. This approach not only directly identifies protein accumulation but also reveals the location within the cell and any posttranslational modifications. One study reported the analysis of near-isogenic lines (NILs), derived from W82 carrying either the Rpp1 resistance or susceptible allele (Cooper et al. 2011). The analysis revealed 260 proteins showing differential accumulation in the Rpp1 plant nuclei at 24 h post-infection by P. pachyrhizi. Among these proteins, 111 showed elevation, while 149 were found at lower levels in the Rpp1 plant nuclei when compared to isogenic Rpp1 plants. These findings highlight significant and specific changes in the nuclear proteome triggered by Rpp1 upon recognition of P. pachyrhizi. Further, soybean rust (SBR), caused by the two fungal pathogens Phakopsora meibomiae and P. pachyrhizi, has been recognised as a prominent disease in Asia and the United States (Wrather et al. 2001b).
Identifying resistance genes against soybean cyst nematode (SCN)
H. glycines, recognised by its common name, soybean cyst nematode (SCN), represents a highly disruptive plant pathogen known for its significant adverse effects on soybean crops, leading to a drastic reduction in the overall yield of harvested soybeans (Arjoune et al. 2022), affecting soybean crops on a global scale (Wrather and Koenning 2006). The SCNProDB database curated the proteins potentially linked to SCN (Natarajan et al. 2014). Among the key contributors to SCN resistance are Rhg1 and Rhg4, identified as major QTLs/genes (McHale et al. 2012; Mitchum 2016). Another SCN gene, Rhg4, encoding a serine hydroxymethyl transferase (SHMT), plays a crucial role in conferring a novel plant defence response against pathogens (Liu et al. 2012). In the Rhg1 locus, the copy number variations of three genes have been linked with resistance to SCN (Cook et al. 2012, 2014; Lee et al. 2016).
The resistance to SCN involves the activation of salicylic acid, with studies showing that the overexpression studies of a salicylic acid methyltransferase gene can confer SCN resistance (Lin et al. 2016). Among root pieces infected by SCN, both the concentration of 1-aminocyclopropane-1-carboxylic acid (ACC) and the ACC synthase expression were found to be at peak compared to other root parts (Tucker et al. 2010). Additionally, various genes, including CLE, t-SNAREs, GmAFS and MIR396 have been identified to respond to SCN colonisation (Guo et al. 2015; Noon et al. 2019; Dong et al. 2020). Particularly, alongside SCN colony, alterations in DNA methylation in specific loci have been linked to genetic shifts (Rambani et al. 2020). Subsequent investigations revealed that the duplicated gene copies responsible to code an atypical alpha-soluble N-ethylmaleimide-sensitive factor (NSF) attachment protein (alpha-SNAP) were identified as the primary candidates for carrying SCN resistance (Patil et al. 2017). Within the germplasm possessing Rhg1(+), an examination of a unique NSF allele demonstrated that NSF showed enhanced in vitro binding with Rhg1 resistance-type alpha-SNAPs. This observation suggests an unusual interdependent evolution of the soybean SNARE recycling mechanism, which acts to balance the integration of disrupting protein, ultimately acquired disease resistance (Bayless et al. 2018).
However, there is an urgent need to accelerate soybean breeding efforts to align with the demands of a growing global population (Hina et al. 2020; Rani et al. 2023b; Razzaq et al. 2023b). This acceleration is vital not only to advance sustainable agricultural practices but also to effectively address approaching environmental changes. The reference soybean genome (Schmutz et al. 2010) marked a substantial milestone, greatly streamlining progress in soybean functional genomics. Functional genomics has significantly advanced soybean improvement by identifying key genes and mechanisms critical for enhancing traits such as disease resistance. This knowledge has informed targeted breeding strategies, paving the way for enhanced soybean resistance and crop protection. Soybean functional genomics tools encompass a range of techniques including microarrays, RNA-Seq, CRISPR-Cas9 gene editing, proteomics, metabolomics and various sequencing approaches like ChIP-Seq and ATAC-Seq, all contributing to a comprehensive understanding of soybean gene function and regulation in response to pathogens and stressors (Mubarik et al. 2021; Razzaq et al. 2021; Sharmin et al. 2021).
Identifying resistance genes against bacterial pathogens
The predominant bacterial diseases effecting soybeans are bacterial pustule, caused by Xanthomonas axonopodis pv. glycines and bacterial blight, which is caused by P. syringae pv. glycinea (Psg) (Hartman and Hill 2010). The Psg–soybean pathosystem stands as a pivotal model for delving into the genetic and molecular mechanisms of pathogen recognition in plants. Pioneering molecular studies on gene-for-gene disease resistance have utilised Psg and soybean. Remarkably, researchers derived the first avirulence gene cloned from Psg, emphasising its crucial role in advancing our comprehension of plant–pathogen interactions (Staskawicz et al. 1984). Five resistance genes or alleles, labelled Rpg1-b, Rpg1-r, Rpg2, Rpg3 and Rpg4, have been identified and confer protection against distinct Psg avirulence factors (AvrB, AvrRpm1, AvrA, AvrC and AvrD) (Farhatullah et al. 2011; Whitham et al. 2016). Initially positioned on MLG F (A), the Rpg1-b and Rpg1-r genes were later cloned. The Rpg2 shows a loose linkage to Rpg1, while Rpg3 is genetically tied to Rpg4 within a range of 40.5 ± 3.2 recombination units (Keen and Buzzell 1991; Ashfield et al. 2014).
Bacterial pustule, a prevalent affliction in areas with warm and humid climates, affects soybean. It is triggered by X. axonopodis pv. glycines, which induces minor and light green spots featuring raised pustules within lesion centres. These pustules can develop into extensive necrotic lesions, resulting in premature defoliation of the plants (Narvel et al. 2001; Matsuo et al. 2017). The discovery of the first resistance gene, rxp from CNS cultivar, placed it between Satt014 and Satt372 markers on MLG D2 during the mapping process (Narvel et al. 2001). Subsequent investigations led to the refinement of the rxp locus, pinpointing it to a 33 kb genomic segment situated between SNUSSR17_9 and SNUSNP17_12 markers and identified two potential candidate genes (Kim et al. 2010). Additionally, a separate recessive resistance gene was discovered originating from PI 96188, situated on MLG O, having a close linkage with Sat_108 marker (Kim et al. 2011). Previous studies have also outlined the presence of QTLs underlying resistance against bacterial pustule. Specifically, studies have reported four QTLs distributed across chr09, chr14, chr17 and chr20, accounting for 2.7–20.9% of observed trait variances (Seo et al. 2009; Chang et al. 2016).
Conclusions and future perspectives
As a major crop in the world, soybeans are threatened by a number of pathogenic organisms, such as bacteria, viruses, nematodes and fungus. These infections have the capacity to seriously reduce yields and harm the economy. Traditionally, tillage and crop rotation are two effective management techniques that have been used to lessen some of these problems. Pathogens, however, are always evolving, which highlights the necessity of continuing study to find resistance genes and defensive signalling networks in order to create novel and long-lasting infection-fighting tactics. Functional genomics, enabled by advanced technologies, has significantly contributed to identifying key genes and mechanisms critical for enhancing traits like disease resistance in soybeans.
Soybean functional genomics currently encounter technical trials, particularly the absence of a steady and effective transgenic approach, which prolongs functional studies compared to model plants. Phenotyping is also a challenge due to soybean’s sensitivity to photoperiod, causing significant variations in line phenotypes across different environments. However, advancements in technologies like target base editing and transient expression systems are expected to streamline soybean functional studies. Additionally, the recent development of a graph-based soybean pan-genome is poised to revitalise omics and transform the soybean genomics with functional and evolutionary perspective. It is expected that the continued research and collaboration within the scientific community are essential to address the hurdles posed by evolving pathogens and to secure a resilient and thriving agricultural prospects.
Data availability
Data sharing is not applicable as no new data were generated or analysed during this study.
Author contributions
A.H., M.K.R. and A.A conceived and designed the project. A.H., M.K.R., A.A., M.B.S., K.A. and G.R. contributed to writing the original draft, data verification, and proofreading. F.H., N.A., A.H., A.A., M.A., T.S., H.M.A. and N.R.A. revised the paper. All authors have read and agreed to the final version of the manuscript.
References
Abd-Elgawad MMM (2022) Understanding molecular plant–nematode interactions to develop alternative approaches for nematode control. Plants 11, 2141.
| Crossref | Google Scholar | PubMed |
Akbar S, Wei Y, Zhang M-Q (2022) RNA interference: promising approach to combat plant viruses. International Journal of Molecular Sciences 23, 5312.
| Crossref | Google Scholar | PubMed |
Alizadeh M, Vasebi Y, Chauhan M, Rani A (2021) Biopriming: a prospective techniques for crop improvement. Innovations 66, 1035-1058.
| Google Scholar |
Allen TW, Bradley CA, Sisson AJ, Byamukama E, Chilvers MI, Coker CM, Collins AA, Damicone JP, Dorrance AE, Dufault NS, et al. (2017) Soybean yield loss estimates due to diseases in the United States and Ontario, Canada, from 2010 to 2014. Plant Health Progress 18, 19-27.
| Crossref | Google Scholar |
Ansari M, Ahmed S, Khan MT, Hamad NA, Ali HM, Abbasi A, Mubeen I, Intisar A, Hasan ME, Jasim IK (2023) Evaluation of in vitro and in vivo antifungal activity of green synthesized silver nanoparticles against early blight in tomato. Horticulturae 9, 369.
| Crossref | Google Scholar |
Arjoune Y, Sugunaraj N, Peri S, Nair SV, Skurdal A, Ranganathan P, Johnson B (2022) Soybean cyst nematode detection and management: a review. Plant Methods 18, 110.
| Crossref | Google Scholar |
Ashfield T, Redditt T, Russell A, Kessens R, Rodibaugh N, Galloway L, Kang Q, Podicheti R, Innes RW (2014) Evolutionary relationship of disease resistance genes in soybean and Arabidopsis specific for the Pseudomonas syringae effectors AvrB and AvrRpm1. Plant Physiology 166, 235-251.
| Crossref | Google Scholar | PubMed |
Aung K, Jiang Y, He SY (2018) The role of water in plant–microbe interactions. The Plant Journal 93, 771-780.
| Crossref | Google Scholar | PubMed |
Babu M, Gagarinova AG, Brandle JE, Wang A (2008) Association of the transcriptional response of soybean plants with soybean mosaic virus systemic infection. Journal of General Virology 89, 1069-1080.
| Crossref | Google Scholar | PubMed |
Barilli E, Rubiales D, Gjetting T, Lyngkjaer MF (2014) Differential gene transcript accumulation in peas in response to powdery mildew (Erysiphe pisi) attack. Euphytica 198, 13-28.
| Crossref | Google Scholar |
Bayless AM, Zapotocny RW, Grunwald DJ, Amundson KK, Diers BW, Bent AF (2018) An atypical N-ethylmaleimide sensitive factor enables the viability of nematode-resistant Rhg1 soybeans. Proceedings of the National Academy of Sciences 115, E4512-E4521.
| Crossref | Google Scholar |
Beattie GA (2011) Water relations in the interaction of foliar bacterial pathogens with plants. Annual Review of Phytopathology 49, 533-555.
| Crossref | Google Scholar | PubMed |
Bebber DP, Gurr SJ (2015) Crop-destroying fungal and oomycete pathogens challenge food security. Fungal Genetics and Biology 74, 62-64.
| Crossref | Google Scholar | PubMed |
Beneventi MA, da Silva OB, de Sá MEL, Firmino AAP, de Amorim RMS, Albuquerque ÉVS, da Silva MCM, da Silva JP, Campos MdA, Lopes MJC, Togawa RC, Pappas GJ, Grossi–de–Sa MF (2013) Transcription profile of soybean-root-knot nematode interaction reveals a key role of phythormones in the resistance reaction. BMC Genomics 14, 322.
| Crossref | Google Scholar |
Benjamin G, Pandharikar G, Frendo P (2022) Salicylic acid in plant symbioses: beyond plant pathogen interactions. Biology 11, 861.
| Crossref | Google Scholar | PubMed |
Bromfield KR, Hartwig EE (1980) Resistance to soybean rust and mode of inheritance. Crop Science 20, 254-255.
| Crossref | Google Scholar |
Buss GR, Ma G, Chen P, Tolin SA (1997) Registration of V94-5152 soybean germplasm resistant to soybean mosaic potyvirus. Crop Science 37, 1987-1988.
| Crossref | Google Scholar |
Buzzell RI, Tu JC (1989) Inheritance of a soybean stem-tip necrosis reaction to soybean mosaic virus. Journal of Heredity 80, 400-401.
| Crossref | Google Scholar |
Cervantes-Martinez I, Chen P, Orazaly M, Klepadlo M (2015) Identification of a new allele at the Rsv3 locus for resistance to Soybean mosaic virus in PI 61944 soybean accession. Crop Science 55, 999-1005.
| Crossref | Google Scholar |
Chang H-X, Lipka AE, Domier LL, Hartman GL (2016) Characterization of disease resistance loci in the USDA soybean germplasm collection using genome-wide association studies. Phytopathology 106, 1139-1151.
| Crossref | Google Scholar | PubMed |
Che Z, Zhang S, Pu Y, Yang Y, Liu H, Yang H, Wang L, Zhang Y, Liu B, Zhang H, et al. (2023) A novel soybean malectin-like receptor kinase-encoding gene, GmMLRK1, provides resistance to soybean mosaic virus. Journal of Experimental Botany 74, 2692-2706.
| Crossref | Google Scholar | PubMed |
Chen P, Buss GR, Roane CW, Tolin SA (1991) Allelism among genes for resistance to soybean mosaic virus in strain-differential soybean cultivars. Crop Science 31, 305-309.
| Crossref | Google Scholar |
Chen P, Buss GR, Tolin SA (1993) Resistance to soybean mosaic virus conferred by two independent dominant genes in PI 486355. Journal of Heredity 84, 25-28.
| Crossref | Google Scholar |
Chen P, Ma G, Buss GR, Gunduz I, Roane CW, Tolin SA (2001) Inheritance and allelism tests of Raiden soybean for resistance to soybean mosaic virus. Journal of Heredity 92, 51-55.
| Crossref | Google Scholar | PubMed |
Chen P, Buss GR, Tolin SA, Gunduz I, Cicek M (2002) A valuable gene in Suweon 97 soybean for resistance to soybean mosaic virus. Crop Science 42, 333-337.
| Crossref | Google Scholar |
Chen H, Arsovski AA, Yu K, Wang A (2016) Genome-wide investigation using sRNA-seq, degradome-seq and transcriptome-seq reveals regulatory networks of microRNAs and their target genes in soybean during soybean mosaic virus infection. PLoS ONE 11, e0150582.
| Crossref | Google Scholar | PubMed |
Cheng YW, Chan KL (1968) The breeding of rust resistance soybean ‘Tainung 3’. Journal of Taiwan Agricultural Research 17, 30-34.
| Google Scholar |
Cheng Y, Ma Q, Ren H, Xia Q, Song E, Tan Z, Li S, Zhang G, Nian H (2017) Fine mapping of a Phytophthora-resistance gene RpsWY in soybean (Glycine max L.) by high-throughput genome-wide sequencing. Theoretical and Applied Genetics 130, 1041-1051.
| Crossref | Google Scholar | PubMed |
Chowda-Reddy RV, Sun H, Chen H, Poysa V, Ling H, Gijzen M, Wang A (2011a) Mutations in the P3 protein of Soybean mosaic virus G2 isolates determine virulence on Rsv4-genotype soybean. Molecular Plant-Microbe Interactions 24, 37-43.
| Crossref | Google Scholar | PubMed |
Chowda-Reddy RV, Sun H, Hill JH, Poysa V, Wang A (2011b) Simultaneous mutations in multi-viral proteins are required for Soybean mosaic virus to gain virulence on soybean genotypes carrying different R genes. PLoS ONE 6, e28342.
| Crossref | Google Scholar | PubMed |
Cook DE, Lee TG, Guo X, Melito S, Wang K, Bayless AM, Wang J, Hughes TJ, Willis DK, Clemente TE, et al. (2012) Copy number variation of multiple genes at Rhg1 mediates nematode resistance in soybean. Science 338, 1206-1209.
| Crossref | Google Scholar | PubMed |
Cook DE, Bayless AM, Wang K, Guo X, Song Q, Jiang J, Bent AF (2014) Distinct copy number, coding sequence, and locus methylation patterns underlie Rhg1-mediated soybean resistance to soybean cyst nematode. Plant Physiology 165, 630-647.
| Crossref | Google Scholar | PubMed |
Cooper B, Campbell KB, Feng J, Garrett WM, Frederick R (2011) Nuclear proteomic changes linked to soybean rust resistance. Molecular BioSystems 7, 773-783.
| Crossref | Google Scholar | PubMed |
Dana H, Chalbatani GM, Mahmoodzadeh H, Karimloo R, Rezaiean O, Moradzadeh A, Mehmandoost N, Moazzen F, Mazraeh A, Marmari V, et al. (2017) Molecular mechanisms and biological functions of siRNA. International Journal of Biomedical Science 13, 48-57.
| Crossref | Google Scholar | PubMed |
de Wit PJGM (2016) Cladosporium fulvum effectors: weapons in the arms race with tomato. Annual Review of Phytopathology 54, 1-23.
| Crossref | Google Scholar | PubMed |
Dixon RA, Achnine L, Kota P, Liu C-J, Reddy MSS, Wang L (2002) The phenylpropanoid pathway and plant defence – a genomics perspective. Molecular Plant Pathology 3, 371-390.
| Crossref | Google Scholar | PubMed |
Dong L, Cheng Y, Wu J, Cheng Q, Li W, Fan S, Jiang L, Xu Z, Kong F, Zhang D, Xu P, Zhang S (2015) Overexpression of GmERF5, a new member of the soybean EAR motif-containing ERF transcription factor, enhances resistance to Phytophthora sojae in soybean. Journal of Experimental Botany 66, 2635-2647.
| Crossref | Google Scholar | PubMed |
Dong J, Zielinski RE, Hudson ME (2020) t-SNAREs bind the Rhg1 α-SNAP and mediate soybean cyst nematode resistance. The Plant Journal 104, 318-331.
| Crossref | Google Scholar | PubMed |
Dorrance AE, McClure SA, St. Martin SK (2003) Effect of partial resistance on Phytophthora stem rot incidence and yield of soybean in Ohio. Plant Disease 87, 308-312.
| Crossref | Google Scholar | PubMed |
Dowen RH, Pelizzola M, Schmitz RJ, Lister R, Dowen JM, Nery JR, Dixon JE, Ecker JR (2012) Widespread dynamic DNA methylation in response to biotic stress. Proceedings of the National Academy of Sciences 109, E2183-E2191.
| Crossref | Google Scholar |
Du H, Fang C, Li Y, Kong F, Liu B (2023) Understandings and future challenges in soybean functional genomics and molecular breeding. Journal of Integrative Plant Biology 65, 468-495.
| Crossref | Google Scholar | PubMed |
Dumanović J, Nepovimova E, Natić M, Kuča K, Jaćević V (2021) The significance of reactive oxygen species and antioxidant defense system in plants: a concise overview. Frontiers in Plant Science 11, 552969.
| Crossref | Google Scholar | PubMed |
Durrant WE, Dong X (2004) Systemic acquired resistance. Annual Review of Phytopathology 42, 185-209.
| Crossref | Google Scholar | PubMed |
Dutta TK, Banakar P, Rao U (2015) The status of RNAi-based transgenic research in plant nematology. Frontiers in Microbiology 5, 760.
| Crossref | Google Scholar | PubMed |
Ellendorff U, Fradin EF, de Jonge R, Thomma BPHJ (2009) RNA silencing is required for Arabidopsis defence against Verticillium wilt disease. Journal of Experimental Botany 60, 591-602.
| Crossref | Google Scholar | PubMed |
Farhatullah A, Stayton MM, Groose RW, Khan MJ (2011) Genetic analysis of race-specificity of Pseudomonas syringae pv. glycinea. Pakistan Journal of Botany 43, 7-13.
| Google Scholar |
Feng L, Sun J, Jiang Y, Duan X (2022) Role of reactive oxygen species against pathogens in relation to postharvest disease of papaya fruit. Horticulturae 8, 205.
| Crossref | Google Scholar |
Flor HH (1971) Current status of the gene-for-gene concept. Annual Review of Phytopathology 9, 275-296.
| Crossref | Google Scholar |
Forghani F, Hajihassani A (2020) Recent advances in the development of environmentally benign treatments to control root-knot nematodes. Frontiers in Plant Science 11, 1125.
| Crossref | Google Scholar | PubMed |
Freeman BC, Beattie GA (2009) Bacterial growth restriction during host resistance to Pseudomonas syringae is associated with leaf water loss and localized cessation of vascular activity in Arabidopsis thaliana. Molecular Plant-Microbe Interactions 22, 857-867.
| Crossref | Google Scholar | PubMed |
Garcia A, Calvo ÉS, de Souza Kiihl RA, Harada A, Hiromoto DM, Vieira LGE (2008) Molecular mapping of soybean rust (Phakopsora pachyrhizi) resistance genes: discovery of a novel locus and alleles. Theoretical and Applied Genetics 117, 545-553.
| Crossref | Google Scholar | PubMed |
Geng X, Jin L, Shimada M, Kim MG, Mackey D (2014) The phytotoxin coronatine is a multifunctional component of the virulence armament of Pseudomonas syringae. Planta 240, 1149-1165.
| Crossref | Google Scholar | PubMed |
Goel AK, Lundberg D, Torres MA, Matthews R, Akimoto-Tomiyama C, Farmer L, Dangl JL, Grant SR (2008) The Pseudomonas syringae type III effector HopAM1 enhances virulence on water-stressed plants. Molecular Plant-Microbe Interactions 21, 361-370.
| Crossref | Google Scholar | PubMed |
Gouveia BC, Calil IP, Machado JPB, Santos AA, Fontes EPB (2017) Immune receptors and co-receptors in antiviral innate immunity in plants. Frontiers in Microbiology 7, 2139.
| Crossref | Google Scholar | PubMed |
Grennan AK (2006) Plant response to bacterial pathogens. Overlap between innate and gene-for-gene defense response. Plant Physiology 142, 809-811.
| Crossref | Google Scholar | PubMed |
Gudesblat GE, Torres PS, Vojno AA (2009a) Stomata and pathogens: warfare at the gates. Plant Signaling & Behavior 4, 1114-1116.
| Crossref | Google Scholar | PubMed |
Gudesblat GE, Torres PS, Vojnov AA (2009b) Xanthomonas campestris overcomes Arabidopsis stomatal innate immunity through a DSF cell-to-cell signal-regulated virulence factor. Plant Physiology 149, 1017-1027.
| Crossref | Google Scholar | PubMed |
Guimarães RL, Stotz HU (2004) Oxalate production by Sclerotinia sclerotiorum deregulates guard cells during infection. Plant Physiology 136, 3703-3711.
| Crossref | Google Scholar | PubMed |
Gunduz I, Buss GR, Ma G, Chen P, Tolin SA (2001) Genetic analysis of resistance to Soybean mosaic virus in OX670 and Harosoy soybean. Crop Science 41, 1785-1791.
| Crossref | Google Scholar |
Gunduz I, Buss GR, Chen P, Tolin SA (2002) Characterization of SMV resistance genes in Tousan 140 and Hourei soybean. Crop Science 42, 90-95.
| Crossref | Google Scholar | PubMed |
Gunduz I, Buss GR, Chen P, Tolin SA (2004) Genetic and phenotypic analysis of Soybean mosaic virus resistance in PI 88788 soybean. Phytopathology 94, 687-692.
| Crossref | Google Scholar | PubMed |
Guo X, Chronis D, De La Torre CM, Smeda J, Wang X, Mitchum MG (2015) Enhanced resistance to soybean cyst nematode Heterodera glycines in transgenic soybean by silencing putative CLE receptors. Plant Biotechnology Journal 13, 801-810.
| Crossref | Google Scholar | PubMed |
Hartwig EE (1986) Identification of a fourth major gene conferring resistance to soybean rust. Crop Science 26, 1135-1136.
| Crossref | Google Scholar |
Hartwig EE, Bromfield KR (1983) Relationships among three genes conferring specific resistance to rust in soybeans. Crop Science 23, 237-239.
| Crossref | Google Scholar |
Hidayat O, Somaatmadja S (1979) Screening of soybean breeding lines for resistance to soybean rust (Phakopsora pachyrhizi Sydow). Soybean Rust Newsletter 2, 9-22.
| Google Scholar |
Hina A, Cao Y, Song S, Li S, Sharmin RA, Elattar MA, Bhat JA, Zhao T (2020) High-resolution mapping in two RIL populations refines major “QTL Hotspot” regions for seed size and shape in soybean (Glycine max L.). International Journal of Molecular Sciences 21, 1040.
| Crossref | Google Scholar | PubMed |
Huang J, Yang M, Lu L, Zhang X (2016) Diverse functions of small RNAs in different plant–pathogen communications. Frontiers in Microbiology 7, 1552.
| Crossref | Google Scholar | PubMed |
Hwang T-Y, Moon J-K, Yu S, Yang K, Mohankumar S, Yu YH, Lee YH, Kim HS, Kim HM, Maroof MAS, Jeong S-C (2006) Application of comparative genomics in developing molecular markers tightly linked to the virus resistance gene Rsv4 in soybean. Genome 49, 380-388.
| Crossref | Google Scholar | PubMed |
Ibrahim HMM, Alkharouf NW, Meyer SLF, Aly MAM, Gamal El-Din AEKY, Hussein EHA, Matthews BF (2011) Post-transcriptional gene silencing of root-knot nematode in transformed soybean roots. Experimental Parasitology 127, 90-99.
| Crossref | Google Scholar | PubMed |
Islam W, Islam Su, Qasim M, Wang L (2017) Host-Pathogen interactions modulated by small RNAs. RNA Biology 14, 891-904.
| Crossref | Google Scholar | PubMed |
Jahan MA, Harris B, Lowery M, Infante AM, Percifield RJ, Kovinich N (2020) Glyceollin transcription factor GmMYB29A2 regulates soybean resistance to Phytophthora sojae. Plant Physiology 183, 530-546.
| Crossref | Google Scholar | PubMed |
Jiang J, Zhu H, Li N, Batley J, Wang Y (2022) The miR393-target module regulates plant development and responses to biotic and abiotic stresses. International Journal of Molecular Sciences 23, 9477.
| Crossref | Google Scholar | PubMed |
Jones JDG, Dangl JL (2006) The plant immune system. Nature 444, 323-329.
| Crossref | Google Scholar | PubMed |
Jones JT, Haegeman A, Danchin EGJ, Gaur HS, Helder J, Jones MGK, Kikuchi T, Manzanilla-López R, Palomares-Rius JE, Wesemael WML, Perry RN (2013) Top 10 plant-parasitic nematodes in molecular plant pathology. Molecular Plant Pathology 14, 946-961.
| Crossref | Google Scholar | PubMed |
Joshi I, Kohli D, Pal A, Chaudhury A, Sirohi A, Jain PK (2022) Host delivered-RNAi of effector genes for imparting resistance against root-knot and cyst nematodes in plants. Physiological and Molecular Plant Pathology 118, 101802.
| Crossref | Google Scholar |
Jwa N-S, Hwang BK (2017) Convergent evolution of pathogen effectors toward reactive oxygen species signaling networks in plants. Frontiers in Plant Science 8, 1687.
| Crossref | Google Scholar | PubMed |
Kandoth PK, Heinz R, Yeckel G, Gross NW, Juvale PS, Hill J, Whitham SA, Baum TJ, Mitchum MG (2013) A virus-induced gene silencing method to study soybean cyst nematode parasitism in Glycine max. BMC Research Notes 6, 255.
| Crossref | Google Scholar |
Kang YJ, Kim KH, Shim S, Yoon MY, Sun S, Kim MY, Van K, Lee S-H (2012) Genome-wide mapping of NBS-LRR genes and their association with disease resistance in soybean. BMC Plant Biology 12, 139.
| Crossref | Google Scholar |
Katiyar-Agarwal S, Morgan R, Dahlbeck D, Borsani O, Villegas A, Jr., Zhu J-K, Staskawicz BJ, Jin H (2006) A pathogen-inducible endogenous siRNA in plant immunity. Proceedings of the National Academy of Sciences 103, 18002-18007.
| Crossref | Google Scholar |
Keen NT, Buzzell RI (1991) New disease resistance genes in soybean against Pseudomonas syringae pv glycinea: evidence that one of them interacts with a bacterial elicitor. Theoretical and Applied Genetics 81, 133-138.
| Crossref | Google Scholar | PubMed |
Kiihl RAS, Hartwig EE (1979) Inheritance of reaction to soybean mosaic virus in soybeans. Crop Science 19, 372-375.
| Crossref | Google Scholar |
Kim DH, Kim KH, Van K, Kim MY, Lee S-H (2010) Fine mapping of a resistance gene to bacterial leaf pustule in soybean. Theoretical and Applied Genetics 120, 1443-1450.
| Crossref | Google Scholar | PubMed |
Kim KH, Park J-H, Kim MY, Heu S, Lee S-H (2011) Genetic mapping of novel symptom in response to soybean bacterial leaf pustule in PI 96188. Journal of Crop Science and Biotechnology 14, 119-123.
| Crossref | Google Scholar |
Klepadlo M, Chen P, Wu C (2016) Genetic analysis of resistance to Soybean mosaic virus in PI 438307 soybean accession. Crop Science 56, 3016-3023.
| Crossref | Google Scholar |
Klepadlo M, Chen P, Shi A, Mason RE, Korth KL, Srivastava V, Wu C (2017a) Two tightly linked genes for soybean mosaic virus resistance in soybean. Crop Science 57, 1844-1853.
| Crossref | Google Scholar |
Klepadlo M, Chen P, Shi A, Mason RE, Korth KL, Srivastava V (2017b) Single nucleotide polymorphism markers for rapid detection of the Rsv4 locus for soybean mosaic virus resistance in diverse germplasm. Molecular Breeding 37, 10.
| Crossref | Google Scholar |
Klosterman SJ, Atallah ZK, Vallad GE, Subbarao KV (2009) Diversity, pathogenicity, and management of Verticillium species. Annual Review of Phytopathology 47, 39-62.
| Crossref | Google Scholar | PubMed |
Lee TG, Diers BW, Hudson ME (2016) An efficient method for measuring copy number variation applied to improvement of nematode resistance in soybean. The Plant Journal 88, 143-153.
| Crossref | Google Scholar | PubMed |
Leibman-Markus M, Schneider A, Gupta R, Marash I, Rav-David D, Carmeli-Weissberg M, Elad Y, Bar M (2023) Immunity priming uncouples the growth–defense trade-off in tomato. Development 150, dev201158.
| Crossref | Google Scholar |
Li D, Chen P, Alloatti J, Shi A, Chen YF (2010) Identification of new alleles for resistance to Soybean mosaic virus in soybean. Crop Science 50, 649-655.
| Crossref | Google Scholar |
Li S, Smith JR, Ray JD, Frederick RD (2012) Identification of a new soybean rust resistance gene in PI 567102B. Theoretical and Applied Genetics 125, 133-142.
| Crossref | Google Scholar | PubMed |
Li B, Lu D, Shan L (2014) Ubiquitination of pattern recognition receptors in plant innate immunity. Molecular Plant Pathology 15, 737-746.
| Crossref | Google Scholar | PubMed |
Li S, Castillo-González C, Yu B, Zhang X (2017) The functions of plant small RNAs in development and in stress responses. The Plant Journal 90, 654-670.
| Crossref | Google Scholar | PubMed |
Li X, Qin R, Du Q, Cai L, Hu D, Du H, Yang H, Wang J, Huang F, Wang H, Yu D (2020) Knockdown of GmVQ58 encoding a VQ motif-containing protein enhances soybean resistance to the common cutworm (Spodoptera litura Fabricius). Journal of Experimental Botany 71, 3198-3210.
| Crossref | Google Scholar | PubMed |
Liao L, Chen P, Buss GR, Yang Q, Tolin SA (2002) Inheritance and allelism of resistance to soybean mosaic virus in Zao18 soybean from China. Journal of Heredity 93, 447-452.
| Crossref | Google Scholar | PubMed |
Liao L, Chen P, Rajcan I, Buss GR, Tolin SA (2011) Genetic analysis of “8101” soybean containing three genes for resistance to soybean mosaic virus. Crop Science 51, 503-511.
| Crossref | Google Scholar |
Lin J, Mazarei M, Zhao N, Hatcher CN, Wuddineh WA, Rudis M, Tschaplinski TJ, Pantalone VR, Arelli PR, Hewezi T, Chen F, Stewart CN, Jr (2016) Transgenic soybean overexpressing GmSAMT1 exhibits resistance to multiple-HG types of soybean cyst nematode Heterodera glycines. Plant Biotechnology Journal 14, 2100-2109.
| Crossref | Google Scholar | PubMed |
Liston A, Masters SL (2017) Homeostasis-altering molecular processes as mechanisms of inflammasome activation. Nature Reviews Immunology 17, 208-214.
| Crossref | Google Scholar | PubMed |
Liu C-W, Murray JD (2016) The role of flavonoids in nodulation host-range specificity: an update. Plants 5, 33.
| Crossref | Google Scholar | PubMed |
Liu J-Z, Horstman HD, Braun E, Graham MA, Zhang C, Navarre D, Qiu W-L, Lee Y, Nettleton D, Hill JH, Whitham SA (2011) Soybean homologs of MPK4 negatively regulate defense responses and positively regulate growth and development. Plant Physiology 157, 1363-1378.
| Crossref | Google Scholar | PubMed |
Liu S, Kandoth PK, Warren SD, Yeckel G, Heinz R, Alden J, Yang C, Jamai A, El-Mellouki T, Juvale PS, et al. (2012) A soybean cyst nematode resistance gene points to a new mechanism of plant resistance to pathogens. Nature 492, 256-260.
| Crossref | Google Scholar | PubMed |
Liu J-Z, Braun E, Qiu W-L, Shi Y-F, Marcelino-Guimarães FC, Navarre D, Hill JH, Whitham SA (2014) Positive and negative roles for soybean MPK6 in regulating defense responses. Molecular Plant-Microbe Interactions 27, 824-834.
| Crossref | Google Scholar | PubMed |
Liu Z, Shi L, Yang S, Lin Y, Weng Y, Li X, Hussain A, Noman A, He S (2017) Functional and promoter analysis of ChiIV3, a chitinase of pepper plant, in response to Phytophthora capsici infection. International Journal of Molecular Sciences 18, 1661.
| Crossref | Google Scholar | PubMed |
Liu S, Liu Z, Hou X, Li X (2023) Genetic mapping and functional genomics of soybean seed protein. Molecular Breeding 43, 29.
| Crossref | Google Scholar | PubMed |
Lozano-Durán R, Bourdais G, He SY, Robatzek S (2014) The bacterial effector HopM1 suppresses PAMP-triggered oxidative burst and stomatal immunity. New Phytologist 202, 259-269.
| Crossref | Google Scholar | PubMed |
Ma G, Chen P, Buss GR, Tolin SA (1995) Genetic characteristics of two genes for resistance to soybean mosaic virus in PI486355 soybean. Theoretical and Applied Genetics 91, 907-914.
| Crossref | Google Scholar | PubMed |
Ma G, Chen P, Buss GR, Tolin SA (2002) Complementary action of two independent dominant genes in Columbia soybean for resistance to soybean mosaic virus. Journal of Heredity 93, 179-184.
| Crossref | Google Scholar | PubMed |
Ma G, Chen P, Buss GR, Tolin SA (2003) Genetic study of a lethal necrosis to soybean mosaic virus in PI 507389 soybean. Journal of Heredity 94, 205-211.
| Crossref | Google Scholar | PubMed |
Machado ACZ (2014) Current nematode threats to Brazilian agriculture. Current Agricultural Science and Technology 20, 26-55.
| Google Scholar |
Martinez G, Köhler C (2017) Role of small RNAs in epigenetic reprogramming during plant sexual reproduction. Current Opinion in Plant Biology 36, 22-28.
| Crossref | Google Scholar | PubMed |
McHale LK, Haun WJ, Xu WW, Bhaskar PB, Anderson JE, Hyten DL, Gerhardt DJ, Jeddeloh JA, Stupar RM (2012) Structural variants in the soybean genome localize to clusters of biotic stress-response genes. Plant Physiology 159, 1295-1308.
| Crossref | Google Scholar | PubMed |
McLachlan DH, Kopischke M, Robatzek S (2014) Gate control: guard cell regulation by microbial stress. New Phytologist 203, 1049-1063.
| Crossref | Google Scholar | PubMed |
McLean RJ, Byth DE (1980) Inheritance of resistance to rust (Phakopsora pachyrhizi) in soybeans. Australian Journal of Agricultural Research 31, 951-956.
| Crossref | Google Scholar |
Melotto M, Underwood W, Koczan J, Nomura K, He SY (2006) Plant stomata function in innate immunity against bacterial invasion. Cell 126, 969-980.
| Crossref | Google Scholar | PubMed |
Meyer JDF, Silva DCG, Yang C, Pedley KF, Zhang C, van de Mortel M, Hill JH, Shoemaker RC, Abdelnoor RV, Whitham SA, Graham MA (2009) Identification and analyses of candidate genes for Rpp4-mediated resistance to Asian soybean rust in soybean. Plant Physiology 150, 295-307.
| Crossref | Google Scholar | PubMed |
Mitchum MG (2016) Soybean resistance to the soybean cyst nematode Heterodera glycines: an update. Phytopathology 106, 1444-1450.
| Crossref | Google Scholar | PubMed |
Monteros MJ, Missaoui AM, Phillips DV, Walker DR, Boerma HR (2007) Mapping and confirmation of the ‘Hyuuga’ red-brown lesion resistance gene for Asian soybean rust. Crop Science 47, 829-834.
| Crossref | Google Scholar |
Morales AMAP, O’Rourke JA, Van De Mortel M, Scheider KT, Bancroft TJ, Borém A, Nelson RT, Nettleton D, Baum TJ, Shoemaker RC, et al. (2013) Transcriptome analyses and virus induced gene silencing identify genes in the Rpp4-mediated Asian soybean rust resistance pathway. Functional Plant Biology 40, 1029-1047.
| Crossref | Google Scholar | PubMed |
Mubarik MS, Wang X, Khan SH, Ahmad A, Khan Z, Amjid MW, Razzaq MK, Ali Z, Azhar MT (2021) Engineering broad-spectrum resistance to cotton leaf curl disease by CRISPR-Cas9 based multiplex editing in plants. GM Crops & Food 12, 647-658.
| Crossref | Google Scholar | PubMed |
Narvel J, Jakkula L, Phillips D, Wang T, Lee S-H, Boerma H (2001) Molecular mapping of Rxp conditioning reaction to bacterial pustule in soybean. Journal of Heredity 92, 267-270.
| Crossref | Google Scholar | PubMed |
Natarajan S, Tavakolan M, Alkharouf NW, Matthews BF (2014) SCNProDB: a database for the identification of soybean cyst nematode proteins. Bioinformation 10, 387-389.
| Crossref | Google Scholar | PubMed |
Newman M-A, Sundelin T, Nielsen JT, Erbs G (2013) MAMP (microbe-associated molecular pattern) triggered immunity in plants. Frontiers in Plant Science 4, 139.
| Crossref | Google Scholar | PubMed |
Nicaise V (2014) Crop immunity against viruses: outcomes and future challenges. Frontiers in Plant Science 5, 660.
| Crossref | Google Scholar | PubMed |
Niu J, Guo N, Sun J, Li L, Cao Y, Li S, Huang J, Zhao J, Zhao T, Xing H (2017) Fine mapping of a resistance gene RpsHN that controls Phytophthora sojae using recombinant inbred lines and secondary populations. Frontiers in Plant Science 8, 538.
| Google Scholar | PubMed |
Noman A, Fahad S, Aqeel M, Ali U, Amanullah , Anwar S, Baloch SK, Zainab M (2017) miRNAs: major modulators for crop growth and development under abiotic stresses. Biotechnology Letters 39, 685-700.
| Crossref | Google Scholar | PubMed |
Noon JB, Hewezi T, Baum TJ (2019) Homeostasis in the soybean miRNA396–GRF network is essential for productive soybean cyst nematode infections. Journal of Experimental Botany 70, 1653-1668.
| Crossref | Google Scholar | PubMed |
Ochola S, Huang J, Ali H, Shu H, Shen D, Qiu M, Wang L, Li X, Chen H, Kange A, Qutob D, Dong S (2020) Editing of an effector gene promoter sequence impacts plant-Phytophthora interaction. Journal of Integrative Plant Biology 62, 378-392.
| Crossref | Google Scholar | PubMed |
Ogle HJ, Byth DE, McLean R (1979) Effect of rust (Phakopsora pachyrhizi) on soybean yield and quality in south-eastern Queensland. Australian Journal of Agricultural Research 30, 883-893.
| Crossref | Google Scholar |
Park C-J, Caddell DF, Ronald PC (2012) Protein phosphorylation in plant immunity: insights into the regulation of pattern recognition receptor-mediated signaling. Frontiers in Plant Science 3, 177.
| Crossref | Google Scholar | PubMed |
Patil VS, Wuike RV, Thakare CS, Chirame BB (1997) Viability of uredospores of Phakopsora pachyrhizi Syd. at different storage conditions. Journal of Maharashtra Agricultural Universities 22, 260-261.
| Google Scholar |
Patil G, Mian R, Vuong T, Pantalone V, Song Q, Chen P, Shannon GJ, Carter TC, Nguyen HT (2017) Molecular mapping and genomics of soybean seed protein: a review and perspective for the future. Theoretical and Applied Genetics 130, 1975-1991.
| Crossref | Google Scholar | PubMed |
Pedley KF, Pandey AK, Ruck A, Lincoln LM, Whitham SA, Graham MA (2019) Rpp1 encodes a ULP1-NBS-LRR protein that controls immunity to Phakopsora pachyrhizi in soybean. Molecular Plant-Microbe Interactions 32, 120-133.
| Crossref | Google Scholar | PubMed |
Peláez P, Sanchez F (2013) Small RNAs in plant defense responses during viral and bacterial interactions: similarities and differences. Frontiers in Plant Science 4, 343.
| Crossref | Google Scholar | PubMed |
Petitot A-S, Kyndt T, Haidar R, Dereeper A, Collin M, de Almeida Engler J, Gheysen G, Fernandez D (2017) Transcriptomic and histological responses of African rice (Oryza glaberrima) to Meloidogyne graminicola provide new insights into root-knot nematode resistance in monocots. Annals of Botany 119, 885-899.
| Crossref | Google Scholar | PubMed |
Rambani A, Pantalone V, Yang S, Rice JH, Song Q, Mazarei M, Arelli PR, Meksem K, Stewart CN, Hewezi T (2020) Identification of introduced and stably inherited DNA methylation variants in soybean associated with soybean cyst nematode parasitism. New Phytologist 227, 168-184.
| Crossref | Google Scholar | PubMed |
Rani R, Raza G, Ashfaq H, Rizwan M, Razzaq MK, Waheed MQ, Shimelis H, Babar AD, Arif M (2023a) Genome-wide association study of soybean (Glycine max [L.] Merr.) germplasm for dissecting the quantitative trait nucleotides and candidate genes underlying yield-related traits. Frontiers in Plant Science 14, 1229495.
| Crossref | Google Scholar |
Rani R, Raza G, Tung MH, Rizwan M, Ashfaq H, Shimelis H, Razzaq MK, Arif M (2023b) Genetic diversity and population structure analysis in cultivated soybean (Glycine max [L.] Merr.) using SSR and EST-SSR markers. PLoS ONE 18, e0286099.
| Crossref | Google Scholar | PubMed |
Razzaq MK, Aleem M, Mansoor S, Khan MA, Rauf S, Iqbal S, Siddique KHM (2021) Omics and CRISPR-Cas9 approaches for molecular insight, functional gene analysis, and stress tolerance development in crops. International Journal of Molecular Sciences 22, 1292.
| Crossref | Google Scholar | PubMed |
Razzaq MK, Akhter M, Ahmad RM, Cheema KL, Hina A, Karikari B, Raza G, Xing G, Gai J, Khurshid M (2022) CRISPR-Cas9 based stress tolerance: new hope for abiotic stress tolerance in chickpea (Cicer arietinum). Molecular Biology Reports 49, 8977-8985.
| Crossref | Google Scholar | PubMed |
Razzaq MK, Hina A, Abbasi A, Karikari B, Ashraf HJ, Mohiuddin M, Maqsood S, Maqsood A, Haq IU, Xing G, Raza G, Bhat JA (2023a) Molecular and genetic insights into secondary metabolic regulation underlying insect-pest resistance in legumes. Functional & Integrative Genomics 23, 217.
| Crossref | Google Scholar | PubMed |
Razzaq MK, Rani R, Xing G, Xu Y, Raza G, Aleem M, Iqbal S, Arif M, Mukhtar Z, Nguyen HT, et al. (2023b) Genome-wide identification and analysis of the Hsp40/J-protein family reveals its role in soybean (Glycine max) growth and development. Genes 14, 1254.
| Crossref | Google Scholar | PubMed |
Rosso M-N, Dubrana MP, Cimbolini N, Jaubert S, Abad P (2005) Application of RNA interference to root-knot nematode genes encoding esophageal gland proteins. Molecular Plant-Microbe Interactions 18, 615-620.
| Crossref | Google Scholar | PubMed |
Roth MG, Webster RW, Mueller DS, Chilvers MI, Faske TR, Mathew FM, Bradley CA, Damicone JP, Kabbage M, Smith DL (2020) Integrated management of important soybean pathogens of the United States in changing climate. Journal of Integrated Pest Management 11, 17.
| Crossref | Google Scholar |
Rushton PJ, Somssich IE, Ringler P, Shen QJ (2010) WRKY transcription factors. Trends in Plant Science 15, 247-258.
| Crossref | Google Scholar | PubMed |
Saghai Maroof MA, Jeong SC, Gunduz I, Tucker DM, Buss GR, Tolin SA (2008) Pyramiding of soybean mosaic virus resistance genes by marker-assisted selection. Crop Science 48, 517-526.
| Crossref | Google Scholar |
Samac DA, Peñuela S, Schnurr JA, Hunt EN, Foster-Hartnett D, Vandenbosch KA, Gantt JS (2011) Expression of coordinately regulated defence response genes and analysis of their role in disease resistance in Medicago truncatula. Molecular Plant Pathology 12, 786-798.
| Crossref | Google Scholar | PubMed |
Santoni G, Cardinali C, Morelli MB, Santoni M, Nabissi M, Amantini C (2015) Danger- and pathogen-associated molecular patterns recognition by pattern-recognition receptors and ion channels of the transient receptor potential family triggers the inflammasome activation in immune cells and sensory neurons. Journal of Neuroinflammation 12, 21.
| Crossref | Google Scholar | PubMed |
Saur IML, Panstruga R, Schulze-Lefert P (2021) NOD-like receptor-mediated plant immunity: from structure to cell death. Nature Reviews Immunology 21, 305-318.
| Crossref | Google Scholar | PubMed |
Schmitthenner AF (2000) Phytophthora rot of soybean. Plant Health Progress 1, 13.
| Crossref | Google Scholar |
Schmutz J, Cannon SB, Schlueter J, Ma J, Mitros T, Nelson W, Hyten DL, Song Q, Thelen JJ, Cheng J, et al. (2010) Genome sequence of the palaeopolyploid soybean. Nature 463, 178-183.
| Crossref | Google Scholar | PubMed |
Schuebel F, Rocker A, Edelmann D, Schessner J, Brieke C, Meinhart A (2016) 3′-NADP and 3′-NAADP, two metabolites formed by the bacterial type III effector AvrRxo1. Journal of Biological Chemistry 291, 22868-22880.
| Crossref | Google Scholar | PubMed |
Selote D, Shine MB, Robin GP, Kachroo A (2014) Soybean NDR 1-like proteins bind pathogen effectors and regulate resistance signaling. New Phytologist 202, 485-498.
| Crossref | Google Scholar | PubMed |
Senthil-Kumar M, Mysore KS (2011) New dimensions for VIGS in plant functional genomics. Trends in Plant Science 16, 656-665.
| Crossref | Google Scholar | PubMed |
Seo M, Kang S-T, Moon J-K, Lee S-K, Kim Y-H, Jeong K-H, Yun H-T (2009) Identification of quantitative trait loci associated with resistance to bacterial pustule (Xanthomonas axonopodis pv. glycines) in Soybean. Korean Journal of Breeding Science 41, 456-462.
| Google Scholar |
Shakiba E, Chen P, Shi A, Li D, Dong D, Brye K (2012) Two novel alleles at the Rsv 3 locus for resistance to Soybean mosaic virus in PI 399091 and PI 61947 soybeans. Crop Science 52, 2587-2594.
| Crossref | Google Scholar |
Shakiba E, Chen P, Shi A, Li D, Dong D, Brye K (2013) Inheritance and allelic relationships of resistance genes for Soybean mosaic virus in ‘Corsica’ and ‘Beeson’ soybean. Crop Science 53, 1455-1463.
| Crossref | Google Scholar |
Sharmin RA, Karikari B, Chang F, Al Amin GM, Bhuiyan MR, Hina A, Lv W, Chunting Z, Begum N, Zhao T (2021) Genome-wide association study uncovers major genetic loci associated with seed flooding tolerance in soybean. BMC Plant Biology 21, 497.
| Crossref | Google Scholar |
Shi A, Chen P, Li DX, Zheng C, Hou A, Zhang B (2008) Genetic confirmation of 2 independent genes for resistance to soybean mosaic virus in J05 soybean using SSR markers. Journal of Heredity 99, 598-603.
| Crossref | Google Scholar | PubMed |
Silva DCG, Yamanaka N, Brogin RL, Arias CAA, Nepomuceno AL, Di Mauro AO, Pereira SS, Nogueira LM, Passianotto ALL, Abdelnoor RV (2008) Molecular mapping of two loci that confer resistance to Asian rust in soybean. Theoretical and Applied Genetics 117, 57-63.
| Crossref | Google Scholar | PubMed |
Singh BB (1977) Breeding for resistance to soybean rust in India. Soybean Rust Newsletter 1, 13-16.
| Google Scholar |
Souza TP, Dias RO, Silva-Filho MC (2017) Defense-related proteins involved in sugarcane responses to biotic stress. Genetics and Molecular Biology 40, 360-372.
| Crossref | Google Scholar | PubMed |
Staskawicz BJ, Dahlbeck D, Keen NT (1984) Cloned avirulence gene of Pseudomonas syringae pv. glycinea determines race-specific incompatibility on Glycine max (L.) Merr. Proceedings of the National Academy of Sciences 81, 6024-6028.
| Crossref | Google Scholar |
Sugimoto T, Kato M, Yoshida S, Matsumoto I, Kobayashi T, Kaga A, Hajika M, Yamamoto R, Watanabe K, Aino M, et al. (2012) Pathogenic diversity of Phytophthora sojae and breeding strategies to develop Phytophthora-resistant soybeans. Breeding Science 61, 511-522.
| Crossref | Google Scholar | PubMed |
Sun Q, Sun Y, Walker MA, Labavitch JM (2013) Vascular occlusions in grapevines with Pierce’s disease make disease symptom development worse. Plant Physiology 161, 1529-1541.
| Crossref | Google Scholar | PubMed |
Trdá L, Boutrot F, Claverie J, Brulé D, Dorey S, Poinssot B (2015) Perception of pathogenic or beneficial bacteria and their evasion of host immunity: pattern recognition receptors in the frontline. Frontiers in Plant Science 6, 219.
| Crossref | Google Scholar | PubMed |
Tucker ML, Xue P, Yang R (2010) 1-Aminocyclopropane-1-carboxylic acid (ACC) concentration and ACC synthase expression in soybean roots, root tips, and soybean cyst nematode (Heterodera glycines)-infected roots. Journal of Experimental Botany 61, 463-472.
| Crossref | Google Scholar | PubMed |
Underwood W (2012) The plant cell wall: a dynamic barrier against pathogen invasion. Frontiers in Plant Science 3, 85.
| Crossref | Google Scholar | PubMed |
Urwin PE, Lilley CJ, Atkinson HJ (2002) Ingestion of double-stranded RNA by preparasitic juvenile cyst nematodes leads to RNA interference. Molecular Plant-Microbe Interactions 15, 747-752.
| Crossref | Google Scholar | PubMed |
van de Mortel M, Recknor JC, Graham MA, Nettleton D, Dittman JD, Nelson RT, Godoy CV, Abdelnoor RV, Almeida ÁMR, Baum TJ, Whitham SA (2007) Distinct biphasic mRNA changes in response to Asian soybean rust infection. Molecular Plant-Microbe Interactions 20, 887-899.
| Crossref | Google Scholar | PubMed |
Van Loon LC, Van Strien EA (1999) The families of pathogenesis-related proteins, their activities, and comparative analysis of PR-1 type proteins. Physiological and Molecular Plant Pathology 55, 85-97.
| Crossref | Google Scholar |
van Loon LC, Rep M, Pieterse CMJ (2006) Significance of inducible defense-related proteins in infected plants. Annual Review of Phytopathology 44, 135-162.
| Crossref | Google Scholar | PubMed |
Wang M, Sun Y, Sun G, Liu X, Zhai L, Shen Q, Guo S (2015) Water balance altered in cucumber plants infected with Fusarium oxysporum f. sp. cucumerinum. Scientific Reports 5, 7722.
| Crossref | Google Scholar | PubMed |
Weiberg A, Wang M, Lin F-M, Zhao H, Zhang Z, Kaloshian I, Huang H-D, Jin H (2013) Fungal small RNAs suppress plant immunity by hijacking host RNA interference pathways. Science 342, 118-123.
| Crossref | Google Scholar | PubMed |
Whitham SA, Qi M, Innes RW, Ma W, Lopes-Caitar V, Hewezi T (2016) Molecular soybean-pathogen interactions. Annual Review of Phytopathology 54, 443-468.
| Crossref | Google Scholar | PubMed |
Withers J, Dong X (2017) Post-translational regulation of plant immunity. Current Opinion in Plant Biology 38, 124-132.
| Crossref | Google Scholar | PubMed |
Wrather JA, Koenning SR (2006) Estimates of disease effects on soybean yields in the United States 2003 to 2005. Journal of Nematology 38, 173-180.
| Google Scholar | PubMed |
Wrather JA, Anderson TR, Arsyad DM, Tan Y, Ploper LD, Porta-Puglia A, Ram HH, Yorinori JT (2001a) Soybean disease loss estimates for the top ten soybean-producing counries in 1998. Canadian Journal of Plant Pathology 23, 115-121.
| Crossref | Google Scholar |
Wrather JA, Stienstra WC, Koenning SR (2001b) Soybean disease loss estimates for the United States from 1996 to 1998. Canadian Journal of Plant Pathology 23, 122-131.
| Crossref | Google Scholar |
Xin X-F, Nomura K, Aung K, Velásquez AC, Yao J, Boutrot F, Chang JH, Zipfel C, He SY (2016) Bacteria establish an aqueous living space in plants crucial for virulence. Nature 539, 524-529.
| Crossref | Google Scholar | PubMed |
Xu X, Zeng L, Tao Y, Vuong T, Wan J, Boerma R, Noe J, Li Z, Finnerty S, Pathan SM, Shannon JG, Nguyen HT (2013) Pinpointing genes underlying the quantitative trait loci for root-knot nematode resistance in palaeopolyploid soybean by whole genome resequencing. Proceedings of the National Academy of Sciences 110, 13469-13474.
| Crossref | Google Scholar |
Xun H, Yang X, He H, Wang M, Guo P, Wang Y, Pang J, Dong Y, Feng X, Wang S, Liu B (2019) Over-expression of GmKR3, a TIR–NBS–LRR type R gene, confers resistance to multiple viruses in soybean. Plant Molecular Biology 99, 95-111.
| Crossref | Google Scholar | PubMed |
Yadeta KA, Thomma BPHJ (2013) The xylem as battleground for plant hosts and vascular wilt pathogens. Frontiers in Plant Science 4, 97.
| Crossref | Google Scholar | PubMed |
Yang X, Li Y, Wang A (2021) Research advances in potyviruses: from the laboratory bench to the field. Annual Review of Phytopathology 59, 1-29.
| Crossref | Google Scholar | PubMed |
Yang Z, Zhi P, Chang C (2022) Priming seeds for the future: plant immune memory and application in crop protection. Frontiers in Plant Science 13, 961840.
| Crossref | Google Scholar | PubMed |
Zaynab M, Kanwal S, Hussain I, Qasim M, Noman A, Iqbal U, Ali GM, Bahadar K, Jamil A, Sughra K (2017) Rice chitinase gene expression in genetically engineered potato confers resistance against Fusarium solani and Rhizictonia solani. PSM Microbiology 2, 63-73.
| Crossref | Google Scholar |
Zhang C, Yang C, Whitham SA, Hill JH (2009) Development and use of an efficient DNA-based viral gene silencing vector for soybean. Molecular Plant-Microbe Interactions 22, 123-131.
| Crossref | Google Scholar | PubMed |
Zhang C, Bradshaw JD, Whitham SA, Hill JH (2010) The development of an efficient multipurpose bean pod mottle virus viral vector set for foreign gene expression and RNA silencing. Plant Physiology 153, 52-65.
| Crossref | Google Scholar | PubMed |
Zhang X, Li H, Zhang J, Zhang C, Gong P, Ziaf K, Xiao F, Ye Z (2011) Expression of artificial microRNAs in tomato confers efficient and stable virus resistance in a cell-autonomous manner. Transgenic Research 20, 569-581.
| Crossref | Google Scholar | PubMed |
Zhang C, Ding Z, Wu K, Yang L, Li Y, Yang Z, Shi S, Liu X, Zhao S, Yang Z, et al. (2016) Suppression of jasmonic acid-mediated defense by viral-inducible microRNA319 facilitates virus infection in rice. Molecular Plant 9, 1302-1314.
| Crossref | Google Scholar | PubMed |
Zheng C, Chen P, Gergerich R (2005) Characterization of resistance to Soybean mosaic virus in diverse soybean germplasm. Crop Science 45, 2503-2509.
| Crossref | Google Scholar |
Zheng C, Chen P, Gergerich R (2006) Genetic analysis of resistance to soybean mosaic virus in J05 soybean. Journal of Heredity 97, 429-437.
| Crossref | Google Scholar | PubMed |
Zhong C, Sun S, Li Y, Duan C, Zhu Z (2018a) Next-generation sequencing to identify candidate genes and develop diagnostic markers for a novel Phytophthora resistance gene, RpsHC18, in soybean. Theoretical and Applied Genetics 131, 525-538.
| Crossref | Google Scholar | PubMed |
Zhong C, Sun S, Yao L, Ding J, Duan C, Zhu Z (2018b) Fine mapping and identification of a novel Phytophthora root rot resistance locus RpsZS18 on chromosome 2 in soybean. Frontiers in Plant Science 9, 44.
| Crossref | Google Scholar | PubMed |
Zhong C, Li Y, Sun S, Duan C, Zhu Z (2019) Genetic mapping and molecular characterization of a broad-spectrum Phytophthora sojae resistance gene in Chinese soybean. International Journal of Molecular Sciences 20, 1809.
| Crossref | Google Scholar | PubMed |
Zubaidah S, Mujtahida I, Kuswantoro H (2023) Response of morphological, anatomical, and agronomic characteristics of soybean genotypes to Cowpea Mild Mottle Virus. Biodiversitas Journal of Biological Diversity 24, 4017-4026.
| Crossref | Google Scholar |
Zvereva AS, Pooggin MM (2012) Silencing and innate immunity in plant defense against viral and non-viral pathogens. Viruses 4, 2578-2597.
| Crossref | Google Scholar | PubMed |