Melatonin as a master regulatory hormone for genetic responses to biotic and abiotic stresses in model plant Arabidopsis thaliana: a comprehensive review
Muaz Ameen

A
B
C
D
E
Abstract
Melatonin is a naturally occurring biologically active amine produced by plants, animals and microbes. This review explores the biosynthesis of melatonin in plants, with a particular focus on its diverse roles in Arabidopsis thaliana, a model species. Melatonin affects abiotic and biotic stress resistance in A. thaliana. Exogenous and endogenous melatonin is addressed in association with various conditions, including cold stress, high light stress, intense heat and infection with Botrytis cinerea or Pseudomonas, as well as in seed germination and lateral root formation. Furthermore, melatonin confers stress resistance in Arabidopsis by initiating the antioxidant system, remedying photosynthesis suppression, regulating transcription factors involved with stress resistance (CBF, DREB, ZAT, CAMTA, WRKY33, MYC2, TGA) and other stress-related hormones (abscisic acid, auxin, ethylene, jasmonic acid and salicylic acid). This article additionally addresses other precursors, metabolic components, expression of genes (COR, CBF, SNAT, ASMT, PIN, PR1, PDF1.2 and HSFA) and proteins (JAZ, NPR1) associated with melatonin and reducing both biological and environmental stressors. Furthermore, the future perspective of melatonin rich agri-crops is explored to enhance plant tolerance to abiotic and biotic stresses, maximise crop productivity and enhance nutritional worth, which may help improve food security.
Keywords: Arabidopsis thaliana, autophagy, gene expression, hormones, infection, melatonin, ROS, stress.
Introduction
Melatonin is an essential indoleamine derivative that was initially discovered in 1958 in the bovine pineal glands. For the subsequent four decades it was commonly regarded as an animal hormone, specifically as a neurohormone (Lerner et al. 1958; Ferrieri et al. 2015). Melatonin (MT) was found in Japanese morning glory (Pharbitis nil L.) in 1993 and reported in 1995 (Wu et al. 2021). MT safeguards lymphocytes from DNA injuries caused by ionising radiation through its antioxidant properties, maintains the circadian rhythm, provides skin protection and relieves diabetic people by reducing oxidative stress and improving insulin sensitivity. In livestock, it governs body temperature, sleeping and promotes dairy productivity (Gunata et al. 2020). In 1967, Sagan (1967) proposed that mitochondria and chloroplasts synthesised MT from precursors the same as Rhodospirillum rubrum and cyanobacteria. Plant stressors that are biotic (such as diseases and pests) and abiotic (such as drought and severe temperatures) may cause a variety of morpho-physiological and biochemical consequences in the plants. Abiotic stress causes oxidative stress and damage, whereas biotic stress activates the salicylic acid pathway. Responses of plants to environmental stressors relies on the autophagic, antioxidant and anti-inflammatory capabilities of MT. Recently, several investigations have looked at the possibility of using MT as an adjuvant therapy that might slow the adverse effects of COVID-19 (Wichniak et al. 2021; Alizadeh et al. 2022). According to Market Data Forecast (2022), the MT market was estimated at US$437.9 million in 2021, with a projected rise to US$3.4 billion by 2026.
MT is a kind of indole molecule that may be produced in both plants and animals via a biochemical mechanism that relies on the amino acid tryptophan. Notably, auxin, a plant hormone, belongs to the indole group and has a biosynthetic pathway with tryptophan (Mannino et al. 2021). Auxin and MT have biosynthetic and structural resemblances, indicating a signalling pathways association. The research on MT has emphasised auxin and MT’s physiological effects since its identification in plants (Cheng et al. 2021). MT stimulates plant development in a similar manner to auxin, in commercially significant crops such as wheat, maize, barley, rice, tomato, cucumber, soybean and pepper, together with Arabidopsis thaliana (Nawaz et al. 2021). In adverse environmental circumstances, the MT function as a growth stimulator for plants is even more significant. For example, ultraviolet (UV) radiation boosted MT levels in alpine and Mediterranean plants, revealing that its antioxidant capabilities protect against light-induced stresses (Khan et al. 2020c). There is a considerable amount of research that supports the role of MT in plants enabling resistance towards oxidative stress and unfavourable environmental circumstances (Table 1).
Species | Crops name | Stress tolerances | References | |
---|---|---|---|---|
Medicago sativa | Alfalfa | Drought, heavy metal | Hoque et al. (2021); Roy et al. (2021) | |
Arabidopsis thaliana | Thale cress | Drought, high temperature, low temperature, pathogen, salinity | Bajwa et al. (2014); Lee and Back (2018); Lee et al. (2014); Su et al. (2021); Wang et al. (2023) | |
Musa acuminata | Banana | Pathogen | Wei et al. (2017) | |
Malus hupehensis | Crabapple | Salinity, alkaline | Gong et al. (2017); Li et al. (2012) | |
Cucumis sativus | Cucumber | Disease, drought, low temperature, nitrate, salinity | Brengi et al. (2022); Sun et al. (2019); Uzal et al. (2023); Zhang et al. (2013); Zhang et al. (2017c) | |
Actinidia deliciosa | Kiwifruit | High temperature | Xia et al. (2021) | |
Zea mays | Maize | Drought, high temperature, low temperature, salinity | Hussain et al. (2022); Kołodziejczyk et al. (2016); Li et al. (2019b); Zhao et al. (2021a) | |
Avena nuda | Naked oat | Drought, heavy metals, salinity | Gao et al. (2019); Wang et al. (2022a); Wang et al. (2022b); Zhang et al. (2022b) | |
Prunus persica | Peach | Low temperature | Gao et al. (2018) | |
Solanum tuberosum | Potato | Pathogen | Zhang et al. (2017d) | |
Brassica napus | Rapeseed | Drought | Khan et al. (2020b) | |
Lolium perenne | Rye grass | High temperature | Zhang et al. (2017a) | |
Oryza sativa | Rice | Drought, low temperature | Li et al. (2021b); Li et al. (2022a) | |
Helianthus annuus | Sunflower | Salinity | Arora and Bhatla (2017) | |
Festuca arundinacea | Tall fescue grass | High temperature | Alam et al. (2022) | |
Camellia sinensis | Tea plant | Drought, low temperature, salinity | Langaroudi et al. (2023); Li et al. (2019a); Li et al. (2018) | |
Solanum lycopersicum | Tomato | Acid rain, drought, heavy metal, high temperature, low temperature, salinity, sodic alkaline | Ahammed et al. (2019); Bhardwaj et al. (2022); Debnath et al. (2020); Ding et al. (2022); Umapathi et al. (2022); Yan et al. (2019); Yang et al. (2022b) | |
Citrullus lanatus | Watermelon | Heavy metal, low temperature, salinity | Chang et al. (2021); Li et al. (2017); Nawaz et al. (2018) | |
Triticum aestivum | Wheat | Drought, heavy metal, low temperature, salinity | Ahmad et al. (2022); Chen et al. (2023a); Shamloo-Dashtpagerdi et al. (2022); Zhang et al. (2021) |
Plant MT research is steadily growing, and its applications in several plants have been identified. Over the past 20 years, there has been a notable increase in the volume of literature focused on plant MT. This accomplishment has been realised through the synergistic application of techniques such as material fractionation and identification, molecular sequencing and biological methodologies (Sun et al. 2021; Hassan et al. 2022b). Through the implementation of these methods, valuable insights have been gleaned into the levels, spatial distribution, synthesis pathways, metabolic characteristics, as well as the physiological and biochemical functions of MT in plants. It plays a crucial role in diverse plant growth and developmental processes, including its involvement in abiotic and biotic stress responses. Blask et al. (2004) proposed the name ‘Phyto-Melatonin’ to describe MT, and they considered it as a multi-regulatory compound. This was because MT has a broad range of biological properties. The MT is manufactured by most plants, as well as crop plants, in volumes that range from picograms to micrograms per gram of tissue mass (Pérez-Llamas et al. 2020). Furthermore, the genes responsible for MT production in plants have been proficiently replicated from a range of plant species, beginning with the cloning of tryptophan decarboxylase, the pivotal enzyme in the biosynthesis pathway of MT (Byeon et al. 2016). In addition, the latest replication of N-acetylserotonin deacetylase, which transforms MT into serotonin, reveals that metabolic enzymes closely control plant MT production (Lee et al. 2018). There is no explanation for plants to generate large amounts of MT in comparison to mammals because of the abundance of MT metabolic enzymes as well as other mechanisms with fairly low kinetics of MT biosynthetic enzymes in plants (Bhowal et al. 2021).
Recent research on Arabidopsis thaliana, which resulted in the identification of the initial proposed MT receptor of plant, has led researchers to propose that MT may have a role as a phytohormone (Khan et al. 2022). Arabidopsis thaliana belongs to the mustard family and has been extensively employed as a model plant in the realm of biomedical research focused on plants. Significant improvements have been made in the research on the development and growth of plants by emphasising the molecular genetics of this basic angiosperm (Beilstein et al. 2010). In addition, MT exhibits a wide variety of hormone-like properties, such as the regulation of postharvest fruit ripening, germination of seeds, circadian rhythms, root growth and development, leaf senescence, and the adaptations of plants to a wide range of ecological challenges, such as cold, heat, light and water stress (Yan et al. 2020). Arabidopsis has emerged as a more refined model organism for studying plant biology and complex organisms.
Melatonin biosynthesis in plants
Melatonin was originally recognised in plants in 2000 and detected in several tissues, with chloroplasts and mitochondria being its key manufacturing hotspots. From an evolutionary perspective, it is assumed that chloroplasts are the direct ancestors of cyanobacteria, and it is thought that the progenitor of mitochondria shared similarities with a purple non-sulfur bacterium (Khan et al. 2023). The amino acid L-tryptophan may be transformed into the MT by two distinct metabolic pathways: the first occurs in young, healthy plants, whereas the second occurs in older plants or under conditions of biological stress (Back 2021). The prevalence of MT in a diverse range of plant species is shown in Table 2. The MT may be found in high concentrations in the leaves, roots, bulbs, flowers and seeds of most plant species. It has been discovered that the roots of the plant known as Scutellaria biacalensis (Huang-Qin), which is a species of the Lamiaceae family, have an unusually high amount of MT, up to 7110 ng/g (Altaf et al. 2021). The Rosaceae, Brassicaceae, Poaceae, Apiaceae and Vitaceae families have the most MT-containing plants, and other families also include considerable quantities. The genotype, environmental conditions like photoperiod and temperature, and growth level of plants impact MT production (Nawaz et al. 2016; Ahammed and Li 2022).
Plant species | Common name | Family | Organs | Reference | |
---|---|---|---|---|---|
Allium cepa | Onion | Amaryllidaceae | Corm | Paredes et al. (2016) | |
Aloe vera | Aloe vera | Liliaceae | Shoot | Chen et al. (2011) | |
Angelica biserrata | Du-Huo | Apiaceae | Stem | Fan et al. (2018) | |
Artemisia annua | Anual mugwort | Asteraceae | Root | Erland et al. (2016) | |
Beta vulgaris | Beet | Amaranthaceae | Leaf | Yan et al. (2020) | |
Brassica rapa | Mustard | Brassicaceae | Corm | Fan et al. (2018) | |
Citrus reticulata | Common mandarin | Rutaceae | Fruit | Chen et al. (2003) | |
Cucumis sativus | Cucumber | Cucurbitaceae | Cortex | Fan et al. (2018) | |
Daucus carota | Carrot | Apiaceae | Fruit | Badria (2002) | |
Fragaria magna | Strawberry | Rosaceae | Root | Bhattacharjee and Chakraborty (2018) | |
Malus domestica | Apple | Rosaceae | Tuber | Yan et al. (2020) | |
Morus alba | White mulberry | Moraceae | Leaf | Yuan and Zhao (2017) | |
Musa ensete | Banana | Musaceae | Fruit | Feng et al. (2014) | |
Nicotiana tabacum | Tobacco | Solanaceae | Stem tuber | Back (2021) | |
Ophiopogon japonicus | Mondo grass | Asparagaceae | Root | Paredes et al. (2008) | |
Oryza sativa | Rice | Poaceae | Seed | Back (2021) | |
Portulaca oleracea | Purslane | Portulacaceae | Fruit | Reiter et al. (2007) | |
Prunella vulgaris | Woundwort | Lamiaceae | Whole plant | Fan et al. (2018) | |
Raphanus sativus | Radish | Brassicaceae | Flower | Yan et al. (2020) | |
Salvia miltiorrhiza | Red sage | Labiatae | Root | Fan et al. (2018) | |
Vitis vinifera | Grapes | Vitaceae | Leaf | Bose and Howlader (2020); Mannino et al. (2021) | |
Zea mays | Maize | Poaceae | Fruit | Mannino et al. (2021) |
In healthy plants, tryptamine is produced from L-tryptophan by the process of carboxylation, which takes place in the cytoplasm and is mediated by TDC (tryptophan decarboxylase). Tryptamine is metabolised into serotonin on the endoplasmic reticulum (ER) by a second enzyme called tryptamine-5-hydroxylase (T5H). This process takes place in the presence of acetyl-coenzyme A. In the chloroplast serotonin is generally acetylated into N-acetylserotonin by the enzyme SNAT (serotonin N-acetyltransferase). This is then accomplished by the processing of N-acetylserotonin into MT by N-acetylserotonin methyltransferase (ASMT), which takes place through methylation in the cytoplasm. Serotonin may amass during senescence and cadmium stress, and the MT synthesis pathway may promote the utilisation of ASMT to metabolise 5-methoxytryptamine from serotonin, which is ultimately transformed by SNAT into MT. The last step involves the simultaneous conversion of SAH (S-adenosyl-L-homocysteine) from SAM (S-adenosyl-L-methionine), along with the MT conversion from acetyl-5-hydroxytryptamine (Ye et al. 2019; Ahammed and Li 2022).
Serotonin is synthesised from tryptophan by carboxylation and hydroxylation during healthy growth and development; but in situations of biological stress and senescence, serotonin firstly goes through the process of methylation to form 5-methyltryptamine, which is subsequently acetylated to make MT (Wu et al. 2021). The presence of two MT biosynthetic pathways in plants may be mediated by precise mechanisms (Fig. 1), but the actual regulatory mechanisms of MT biosynthetic enzymes during various situations need additional research.
Biosynthesis of melatonin in plants: insights and mechanisms (Xie et al. 2022b). Ac-CoA, acetyl-coenzyme A; CoA, coenzyme A; COMT, caffeic acid O-methyltransferase.
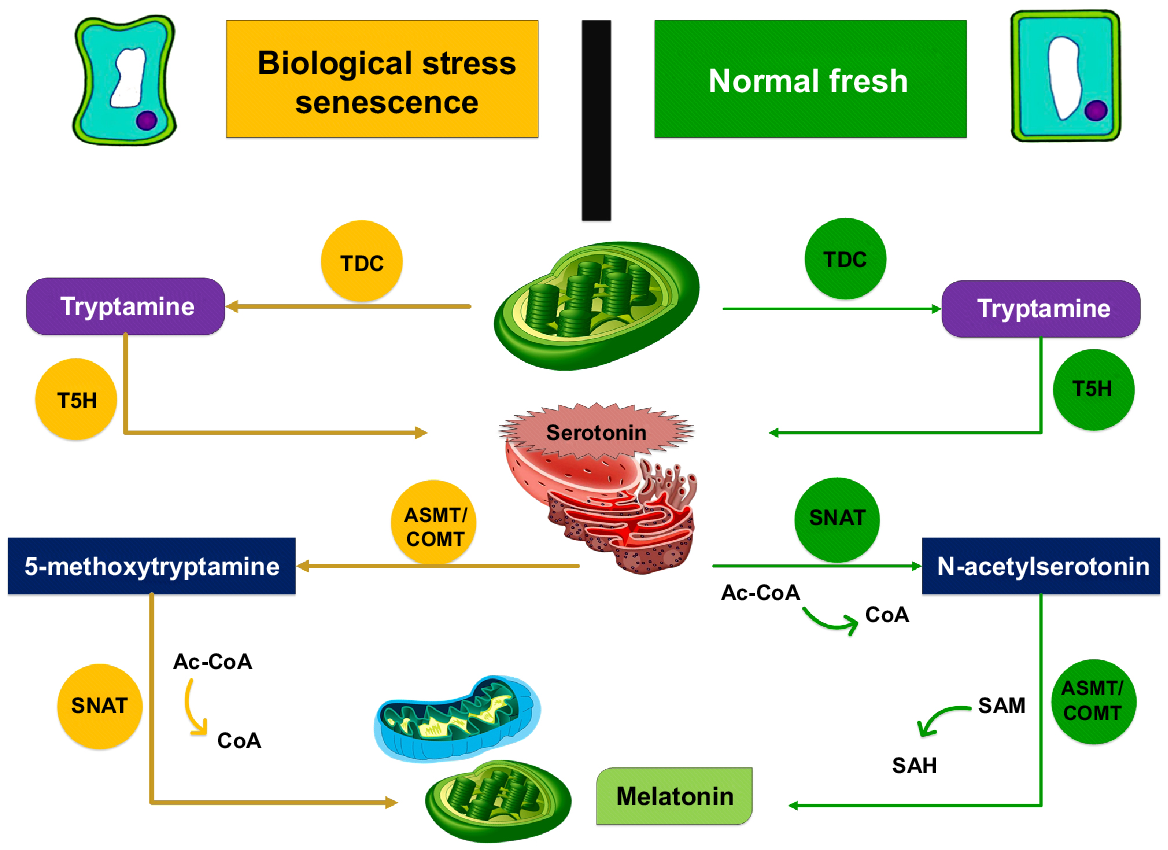
Vital roles of melatonin discovered in Arabidopsis thaliana
Alleviating cold stress tolerance
One of the most challenging problems in crop production in the way of environmental factors is low temperature. Temperatures below freezing present a significant challenge for agricultural production all over the world because of the negative effect they have on agricultural output (Praveen and Sharma 2019; Ameen et al. 2023a). Since cold resistance is a quantitative feature regulated by numerous genes and developing cold resistance plants using traditional breeding or transgenic strategies is complicated. The physiology, molecular biology and biochemistry of plants are all affected in a variety of ways by cold exposure (Ritonga and Chen 2020; Kidokoro et al. 2022). Cold influences membrane fluidity and deposits both high and low molecular weight cryoprotectants, which also inhibit oxidative injury at the cellular level (Adhikari et al. 2022). In plants, particularly Arabidopsis where several cold-regulated genes are recognised, a lot has been learned about how cold resistance works at the molecular level. Cold adaptation in Arabidopsis includes COR (cold regulated) genes and transcription activators. C-repeat-binding factor (CBF1, CBF2 and CBF3), also referred as Drought Response Element Binding factor (DREB1a, DREB1b and DREB1c), is involved in modulating expression of COR genes through promoter binding (Kidokoro et al. 2022). COR15a is a significant cryoprotective protein that improves protoplast and chloroplast freezing resistance by stabilising lipid bilayers and modifying inner-membrane curvature (Feng et al. 2019). In Arabidopsis plants, CBF1 boosts the expression of COR genes like COR15a, which may improve freezing resistance according to Liang et al. (2022). Transgenic Arabidopsis plants that overexpress COR and CBF genes are not only resilient to the cold, but they are also resistant to other environmental factors such as water and salt stress (Li et al. 2022d). The cold resistance of plants depends on ethyne (C2H2) ZAT10 and ZAT12 (zinc-finger transcription activators). ZAT10 may sub-regulate CBF-target genes, whereas ZAT12 regulates CBF genes’ expression (Xie et al. 2019). The CAMTAs (Calmodulin-binding transcription activators) are another essential transcription factor that performs a significant role in cold resistance in Arabidopsis. These activators serve as positive regulators of the CBF2 gene (Noman et al. 2021; Yang et al. 2022a).
The recent findings suggest that MT protects plants from environmental and biological stressors and boosts development and growth in small doses. It regulates antioxidant activity and gene expression (Qari et al. 2022). Bajwa et al. (2014) investigated the effects of MT in Arabidopsis thaliana subjected to cold exposure. The foliar applications of MT on plants reduced cold stress and improved growth. Arabidopsis seedlings treated with 10 and 20 μM MT had increased primary root expansion as well as fresh weight. MT applications of 100 μM or above adversely impact plant growth and development. Chang et al. (2023) also found non-significant variations in primary root growth among normal plants and those medicated with 100 μM and 200 μM MT. Nevertheless, Bajwa et al. (2014) found that lower MT quantities (10–40 μM) boosted Arabidopsis plant growth and secondary root formation. This is similar to the results of bio-assays by Xiao et al. (2019) and Uzal et al. (2023), who discovered the ideal growth promoting impacts of MT. CBF1 and CBF3 transcripts formed soon after a few minutes of cold exposure, whereas CBF2 transcripts were unaffected. Enhanced COR15a expression was also observed. The utilisation of these most effective cold responsive genes in Arabidopsis MT applications may have resulted in an amplification of factors like cold stress signalling pathways.
The CBF transcription factors are responsible for controlling the expression of approximately 100 genes that make up the CBF regulon. These genes work harmoniously to give plants the ability to withstand cold conditions (Kashyap et al. 2021). The MT therapy might have a considerable beneficial impact in regulating the entire CBF regulon by enhancing the expression of CBF regulatory genes. CBFs modulate COR15a, a cold-responsive gene with cryoprotective properties (Gusain et al. 2023). Membrane stability by COR15a safeguards Arabidopsis protoplasts against freezing damage (Fig. 2). MT has recently been believed to have a cryoprotective function, protecting American elm shoot buds from the effects of being preserved in liquid nitrogen (Knox-Brown et al. 2020).
Understanding the mechanistic framework of AtZAT6 in melatonin-driven freezing stress response (Shi and Chan 2014).
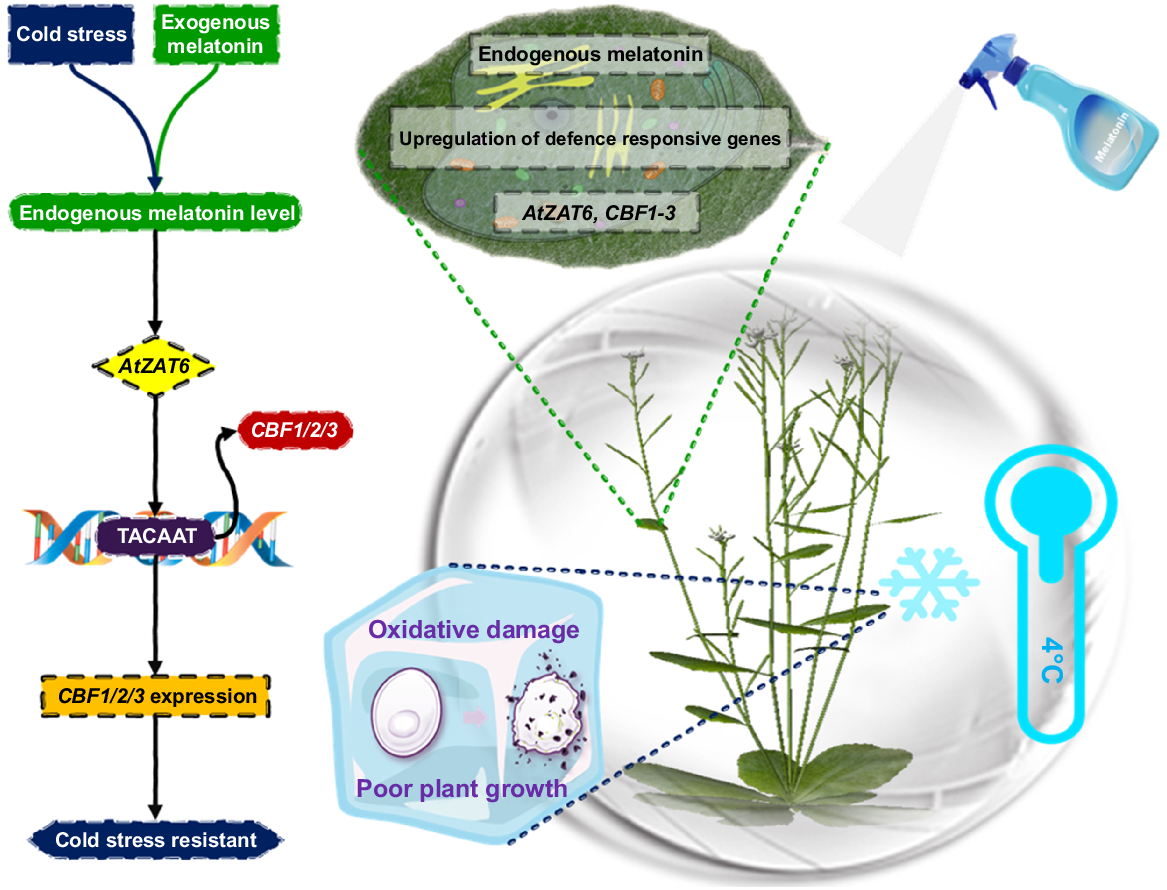
The reactive oxygen species (ROS)-related antioxidant system, activated by ZAT10 and ZAT12 transcription factors, helps plants tolerate drought, cold and excessive salt. In Arabidopsis, foundational overexpression of ZAT10 and ZAT12 increased drought and cold tolerance (Yang et al. 2020). ZAT12 upregulated approximately 8% of fundamental cold stress inducing genes. After cold exposure, MT treated plants upregulate ZAT10 and ZAT12, suggesting that CBF and ZAT10/ZAT12 pathways may contribute to cold stress resistance. The ability of MT to activate other antioxidants, such as ZAT10/ZAT12, may protect plants against oxidative damage (Ma et al. 2023). Previous experiments prove that MT increases cold resistance in Arabidopsis by the upregulation of CBFs and COR15a genes. CAMTA1, ZAT10 and ZAT12, transcriptional activators essential in cold stress mitigation, are also stimulated by MT (Ayyaz et al. 2022).
Tan et al. (2021) found that CAMTA1, a transcription factor, regulates cold and drought resilience in Arabidopsis. After a few hours of cold treatment, MT administered plants upregulated CAMTA1, suggesting that it regulates genes associated with plant resistance to numerous abiotic stressors. CAMTA proteins in Arabidopsis are thought to be controlled by at least one of the six CaM (calmodulin) proteins and incorporate the calcium Ca2+-CaM signalling pathway with the cold signalling pathway. The modulators of Ca2+-CaM signalling in animal cells include MT and serotonin. The MT directly binds to CaM to modulate numerous physiological activities, including membrane receptor-mediated cytoskeletal consequences. However, it is not known how MT is responsible for physiological changes in plants cells via CaM (Iqbal et al. 2020). The fact that MT makes CAMTA1 work better suggests that MT might also work as Ca2+-CaM signalling, and this may give a starting point for future research.
Severe light stress tolerance
The physiological functions and development of plants are put at risk by severe light stress. The response of melatonin, a major regulatory chemical, to this stressor may provide new ways to mitigate the negative effects of light stress on plants and improve their resistance under harsh climatic circumstances (Gull et al. 2023). Alpine and Mediterranean plants, which get more UV radiation, have higher MT levels than low latitude plants. Water hyacinth grown in direct sunshine generated more MT than those grown in low artificial light, suggesting high light (HL) promotes MT production (Sajjad et al. 2020). The MT levels were much higher in apple leaves when they were exposed to HL stress (in the summer) than when they were exposed to low light stress (in the early fall) (Faghih et al. 2019). In another research, MT administration accelerated nonphotochemical quenching to absorb excess light energy and increase tomato seedling cold stress resilience (Zhang et al. 2022c).
It has been hypothesised that singlet oxygen (1O2) is the predominant form of reactive oxygen species (ROS) generated in chloroplasts because of triplet excited molecules of chlorophyll under conditions of high light (HL) stress in plants. This ROS has a substantial and destructive part in the process of damaging plant tissues (Dmitrieva et al. 2020). Prior studies demonstrate that chloroplasts are the chief sub-cellular organelles in plants that make ROS when exposed to light, whereas mitochondria are the primary factors responsible for producing them when there is no light. It is conceivable that the capacity of chloroplasts to efficiently scavenge ROS is essential for plants’ survival against biotic and abiotic stressors (Mittler et al. 2022). Due to its short lifespan in plant cells and strong reactivity, effects of 1O2 on plants have been challenging to investigate. However, this problem has been solved by the exploration of a conditional mutant of Arabidopsis thaliana (flu), which accumulates a precursor of chlorophyll in darkness and makes a lot of 1O2 when flu seedlings are exposed to light. This shows that there are good genetic assets for making 1O2 in leaf tissues (Wang et al. 2020).
Recent study has shown, for the first time at the genetic level, that 1O2 in chloroplasts has a meaningful role in initiating MT production in Arabidopsis that has been subjected to HL stress (Kohli et al. 2019). Furthermore, it has been observed in several investigations that HL stress causes an increase in MT production. Lee and Back (2018) evaluated the connection between MT and the amount of light and conducted research on many Arabidopsis mutant lines. In Arabidopsis, HL stress promotes MT production, which either scavenges ROS or stimulates antioxidant enzymes (Fig. 3). A body of genetic data presented by Lee and Back (2018) supports the hypothesis that MT performs a mediating part in HL resistance of Arabidopsis. Firstly, MT deficient snat1 (mutant) Arabidopsis was vulnerable to the effects of HL stress. Secondly, the HL susceptible phenotype was proven to be triggered by the lack of SNAT1, which prevented MT production. Achievement of this objective was attained through the introduction of SNAT1 genomic DNA into the snat1 mutant, thereby complementing the mutant phenotype. Lastly, the overexpression of SNAT1 in Arabidopsis allowed the plant to tolerate HL stress. Along with other antioxidant molecules including carotenoids, plastoquinones and tocopherol, MT is unquestionably implicated in the effective detoxification of 1O2 in chloroplasts. This is because 1O2 is the agent that causes HL stress in chloroplasts (Dogra and Kim 2019; Tiwari et al. 2021).
Melatonin-facilitated HL-stress resistance model (Lee and Back 2018). Col-0, wild-type; APX, ascorbate peroxidase; SOD, superoxide dismutase; CAT, catalase.
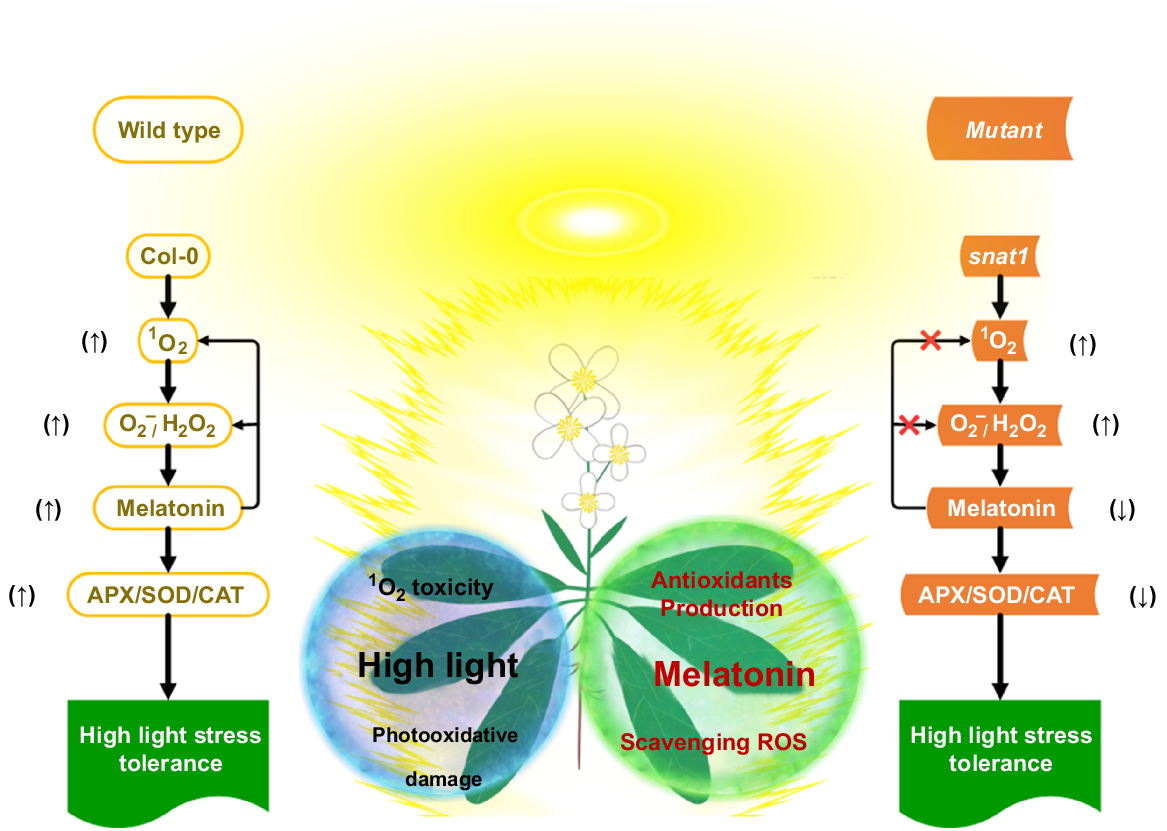
Resistance to Botrytis cinerea infection
Complex signalling networks, which might include the expression of linked genes, are involved in the defensive strategies that plants use against diseases. Necrotrophic pathogen resistance is generally attributed to the PR1 gene. Necrotrophic fungus Botrytis cinerea has a wide host plant range (Ferreira-Saab et al. 2018). B. cinerea spores from formerly infected plants invade other plants when disturbed. MT may have an effect on plant–B. cinerea interactions, such as examined in cherry tomato fruit by Li et al. (2022b), in grapes by Li et al. (2022e), and in tomato by Liu et al. (2019). Salicylic acid (SA) is correlated with PR1, which is upregulated in defence mechanisms against biotrophic fungus but not against necrotrophic B. cinerea. Through immunological responses and systemic acquired resistance, SA protects plants against biotrophic fungus. The involvement of SA in tolerance to B. cinerea in plants is unknown because of its complex nature (Islam et al. 2021). In plants, the PR5 gene is synergically expressed with the PR1 gene and similarly engaged in the signal-transduction process. The transcription factor (TF) WRKY33 is a key regulatory component that performs a vital role in the control of responses of plant to the invasion of fungi (Sham et al. 2017). Modifications in the development and physiological functions of plants that have been infected with B. cinerea are connected to a series of metabolic activities. These metabolic processes include the breakdown of enzymes and the deposition of soluble sugars in the cell walls, as well as variations in pH and a significantly reduced formation of antifungal chemicals and metabolites. Abscisic acid (ABA), ethylene (ET), salicylic acid (SA) and jasmonic acid (JA) hormone signals control most of these alterations (Blanco-Ulate et al. 2015; AbuQamar et al. 2017). Based on the research results of Qu et al. (2022), which was constructed from an examination of transcriptomes, the participation of MT in plant–fungal relations may change the gene expression associated with JA, and MT can combine with JA to regulate how plants respond defensively to threats. The MT and antioxidant enzyme activity increased when ROS levels of plants increased in the presence of environmental stimuli.
We are going to conduct research to determine whether MT helps A. thaliana to be robust to the fungus B. cinerea or other similar species of fungi. Several studies have shown that MT foliar application boosts plant fungal tolerance. It increases plant tolerance to B. cinerea, an observation pretty much identical with earlier research showing that endogenous MT boosts plants immunity to different fungi (Ahammed et al. 2020). The involvement of MT in plant tolerance against B. cinerea, especially endogenous MT, is not fully known. Upregulation or downregulation of SNAT and ASMT genes affects endogenous MT levels. The MT and antioxidant enzymes work together to eliminate ROS, improve photosynthesis and latency in metabolite formation, increase plant antioxidant potential, alleviate oxidative stress in organisms, and protect plants against extreme conditions.
Zhu et al. (2021) investigated how resistance developed because of alteration in the concentration of endogenous MT and found that ASMT and SNAT overexpression lines had higher PR1 expression than Col-0 (the Arabidopsis ecotype), whereas gene silencing lines had lower PR1. Overexpression lines upregulated WRKY33, whereas gene silencing lines downregulated it, correlating with WRKY33 mutants’ enhanced vulnerability to B. cinerea (wrky33-1, wrky33-2). These results support prior observations that PR1 overexpression boosts plant resistance towords B. cinerea (Chen et al. 2022). Overexpression lines showed significant JA content, whereas gene silencing lines showed reduced JA content. Therefore, MT enhanced JA deposition and fungal tolerance. A higher concentration of JA will activate signalling pathways that are downstream of JA. Increased JA content degrades JAZ protein, lowering JAZ-MYC2 association. JAZ protein inhibits the JA-signalling pathway. Within the JA-signalling pathway, MYC2 performs a crucial function as a key regulatory factor. It exerts a negative regulatory effect on downstream signalling genes (ORA59/ERF1) that stimulate plant systemic resistance and promote PDF1.2 genes, which positively regulate JA-dependent disease resistance. In another study conducted by Zhu et al. (2021), PDF1.2 expression was decreased in gene silencing lines asmt-1 and snat-2 but elevated considerably in overexpression lines SNAT-OE-2 and ASMT-OE-1. In earlier investigations, WRKY33 was shown to engage in JA-dependent mechanisms and negatively regulate JA-mediated protective response. WRKY33 elevation did not lower JA concentration (Liu et al. 2017).
The latest research shows that WRKY33 is triggered by a JA-independent mechanism in plants infected by B. cinerea. In infected plants, mitogen-activated protein kinase (MPK3/MPK6) phosphorylates WRKY33 to promote camalexin biosynthesis, indicating that MPK3/MPK6 may cause the WRKY33 overexpression (Li et al. 2021c; Wang et al. 2022d). These observations provide further evidence that MT performs a key function in the stimulation of the JA signalling system and in the increase of plant defence to B. cinerea disease. In plant–pathogen interactions, ROS are early signalling molecules. Plants have developed several antioxidant (enzymatic/non-enzymatic) defense mechanisms to remove ROS and prevent oxidative damage (Khan et al. 2020a). Superoxide dismutase (SOD) and peroxidase (POD) control B. cinerea resilience by regulating oxidative reaction equilibrium. Therefore, elevated levels of endogenous MT improve plant defence against B. cinerea by increasing SOD and POD activity and boosting the rapid elimination of excess ROS (Fig. 4). In a comparable way, the elimination of ROS by MT makes penicillin fungal infections less hazardous to citrus crops (Zeng et al. 2022). So according to prior studies, endogenous MT increased plant resilience to B. cinerea.
Melatonin-mediated plant resistance to B. cinerea infection (Zhu et al. 2021). PRs, pathogenesis-related proteins.
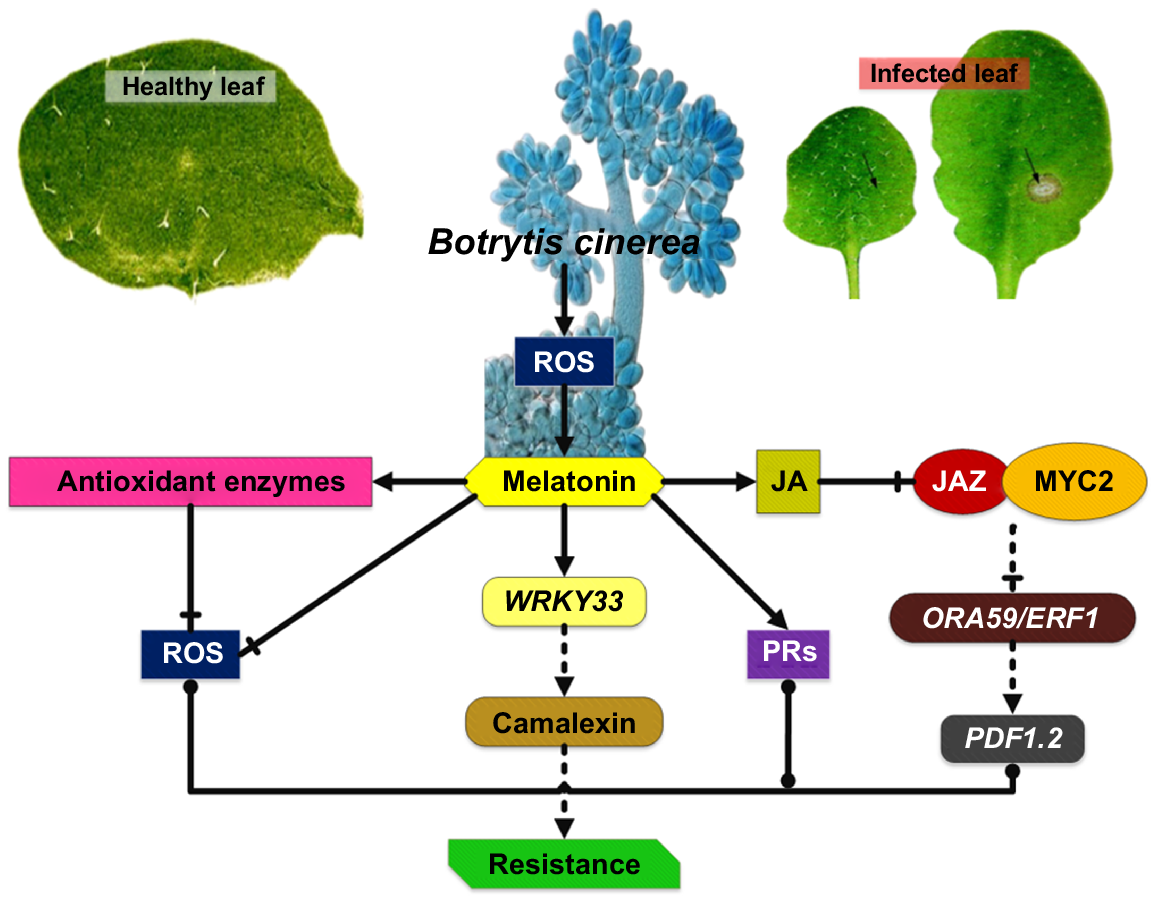
Lateral root development
Auxin and MT have been studied a lot because they are structurally similar, and both use tryptophan as a precursor. The MT regulates auxin (IAA) response, however Zia et al. (2019) and Liu et al. (2023) revealed that it functions independently of auxin signalling. The majority of the research that has been done until now has concentrated on the physiological function of MT and analysed its auxin-like functions in plants (Arabia et al. 2023). Limited research has been conducted to investigate the impacts of MT and auxin on lateral root formation. Some recent research includes tomato (Wen et al. 2016), rice (Liang et al. 2017; Liu et al. 2023), grapes (Gökbayrak et al. 2020) and Arabidopsis (Ren et al. 2019). Although initial research did not reach the conclusion that MT and auxin had a synergistic impact on lateral roots formation, the latest findings revealed that there are a few associations between both compounds.
Ren et al. (2019) discovered that small MT dosages of 10 pM to 100 μM had no discernible impact on primary root growth, on the other hand high doses of MT inhibited it. Additionally, they showed that modest quantities of MT had no significant effect on lateral root growth, whereas substantial doses significantly promoted it. The signalling pathway of auxin plays a pivotal role in the lateral root formation. Auxin signalling is affected by a lot of mutations. Liang et al. (2017) and Liu et al. (2023) discovered a minimum of six rice IAA genes upregulated by MT during lateral root elongation. During an assessment of Arabidopsis gene expression by Ren et al. (2019), MT drastically downregulated five auxin transporter or protein enzyme genes. This considerable downregulation by MT clearly shows that MT facilitated the lateral root growth in Arabidopsis, and is intimately linked with an optimisation of auxin allocation inside the cells by modulation of auxin transport pathways.
Unlike the other members of the PIN protein family, which are typically localised in the cell membrane and govern the directional fluxes of auxin between tissues, PIN5 is uniquely situated in the endoplasmic reticulum and plays a role in the intracellular partitioning and homeostasis of auxin (Včelařová et al. 2021). Current findings have shown that the transport of auxin into the nucleus is regulated by mechanisms beyond simple diffusion, with the predominant flow from the ER to the nucleus superseding diffusion. This challenges the notion that auxin, being a tiny molecule, can freely diffuse into the nucleus without any constraints (Zhang et al. 2023). It is believed that PIN5, which is found in the ER, has a rate limiting function in the modulation of nuclear transport of auxin. This is because the ER is where PIN5 is found (Middleton et al. 2018). Many variables have the potential to control how PIN proteins perform their roles. Auxin downregulates just the PIN5 gene but upregulates several PIN genes. Moreover, the phosphorylation state of PIN proteins is associated with the functionality of PIN proteins. In regard to the phosphorylation of PIN proteins, a significant contribution is made by PINOID protein kinase and its homologs WAG1 and WAG2 (Bai et al. 2022). Auxin distribution and transportation are regulated by plant protein kinase PINOID. Auxin transporter polarisation affects plant development. The genes WAG1 and WAG2 regulate cellular expansions, leaf shape and growth of plants. Wasson et al. (2006) reported that the transcription of PIN family genes did not exhibit considerable alteration in a developed mutant of Medicago truncatula with flavonoid deficiency. In contrast, in Arabidopsis potential kinase WAG1 and PIN5 protein are downregulated by MT. Whenever a mutation took place on either TT4 or TT5 enzymes, which are responsible for flavonoid biosynthesis, they adversely disrupted long-distance auxin transportation and reduced the ability of the plant to grow lateral roots (Liu et al. 2022b). The genes TT4 and TT5 produce flavonoids, plant secondary metabolites. Flavonoids defend against ultraviolet (UV) rays and infections. This finding is in accordance with the findings that were reported by Ren et al. (2019) about flavonoid deficiencies in Arabidopsis. So, in accordance with the current investigations, we conclude that the expression of both tt4 and tt5 genes greatly decreased in the presence of MT in Arabidopsis.
Exogenous auxin stimulates cellular membranes’ auxin transporters and enters root cells. Meanwhile, exogenous auxin directly or indirectly downregulates the PIN5 influx channel, impacting auxin transportation from the ER to the nucleus. Auxin transporters in the cell membrane are indirectly activated by downregulation of flavonoids regulating protein function (Guan et al. 2020). Moreover, exogenous MT blocks the transport of auxin from the cytosol to the ER and nucleus by downregulating PIN5, either directly or indirectly through the regulatory protein kinase WAG1. TT4 and TT5 transcripts are also reduced by exogenous MT, which further inhibits flavonoid production. Exogenous MT increases lateral root development by increasing auxin in the cytosol (Fig. 5) (Banerjee and Sharma 2021). One theory postulates that cytosolic auxin promotes the levels of cytosolic Ca2+ ions, which control lateral root growth through calcium signalling. Auxin may enter the nucleus even when PIN5 is downregulated by auxin and MT. Diffusion or ER-based auxin influx transporters like PIN6 or recently discovered PIN-LIKE (PILS) proteins allow some auxin to enter the nucleus. They affect auxin distribution in cells and plant development and growth (Zhang et al. 2022a). All these negotiations, lead towards the idea that MT may have a role equivalent to that of auxin in determining root growth; however, the amounts of MT required to produce equal degrees of promotion or repression of root development are far greater than those required by auxin. So, MT and auxin synergistically govern lateral root growth in Arabidopsis according to our assumptions.
A diagram illustrating auxin–melatonin dynamic interplay in regulating lateral root growth (Ren et al. 2019).
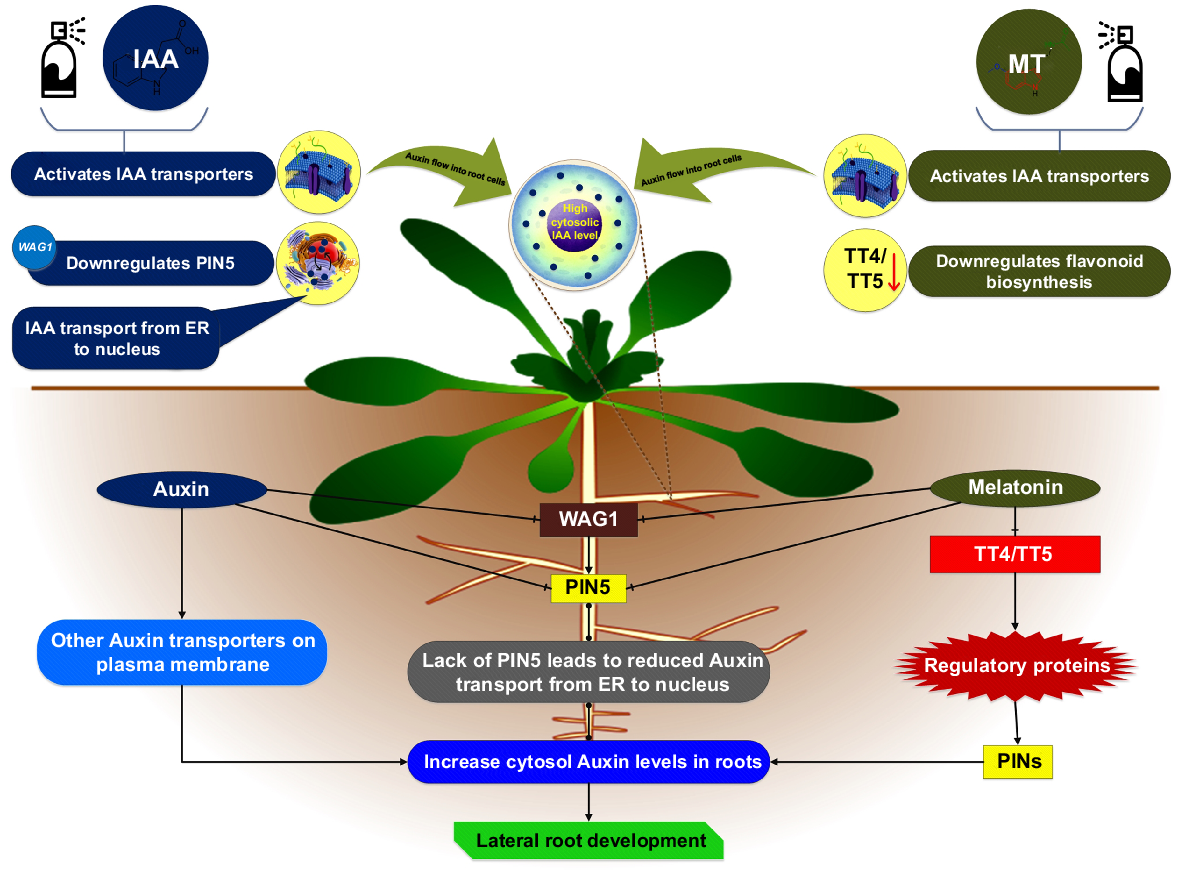
Thermotolerance
Genes that respond to heat stress (HS) in plants are governed by heat-shock transcription factors (HSFs) that are widely expressed throughout the plant. The extensive family of HSFs in higher plants plays a crucial role in conferring tolerance to intense heat and other ecological strains such as cold, drought, floods and salt stressors (Hassan et al. 2022a; Tanveer et al. 2023). Structural properties and phylogenetic analyses classify 21 HSF homologues of Arabidopsis HSFs into fourteen groups (A1 to A9, B1 to B4, and C1) and three primary classes (A, B and C) based on their sequence similarities and evolutionary relationships (Liu and Charng 2013).
Genomic evidence suggests that representatives of the AtHSFA1 group play a pivotal role in regulating HS responses and are important for activating various heat-inducible transcriptional factors and HS-responsive genes (HSFA2, HSA32, HSP90 and HSP101). Specifically, HSFA1a and HSFA1d promote thermotolerance in Arabidopsis, but HSFA1b and HSFA1e may be less efficient (Mishra et al. 2019; Gai et al. 2020). Just a few papers proposed that MT may be involved in HS responsiveness in Arabidopsis and the underlying molecular process. It showed reverse inhibitory impact of high temperature and light on the germination of Phacelia tanacetifolia seedlings according to (Tiryaki and Keles 2012). The researchers Byeon and Back (2014) demonstrated that a rise in temperature causes an upregulation of the internal MT biosynthesis in rice plants. This occurs because of an increase in enzymatic activities of N-acetylserotonin methyltransferase and serotonin N-acetyltransferase.
Shi et al. (2015) reported that the transcriptional upregulation of positive regulators of thermotolerance (AtHSFA1s) by MT were recognised as being engaged in MT-mediated HS adaptations in Arabidopsis. Shi et al. (2015) studied the effect of HS on endogenous MT levels and observed a swift and significant rise in MT levels.
Moreover, the increased level of MT further promoted the AtHSFA1s gene expression, which in turn upregulated the transcript levels of various heat-responsive genes. The overexpression of these genes was associated with greater thermotolerance in Arabidopsis. Several recent investigations suggest that MT is engaged in long-distance signal transduction in plants and promotes significant transcriptional reprogramming (He and He 2020; Liu et al. 2022a), but the relationship between the fundamental signalling pathway and in vivo involvement in plants needs more investigations. Zia et al. (2019) performed a transcriptome study of foliar MT applications in Arabidopsis that increased numerous defence related genes. Several genes associated with stress may also have benefited from the ability of MT to influence adaptations to HS (Fig. 6). Zhang et al. (2017b) discovered 309 proteins that were differently expressed in cucumber (Cucumis sativus L.), because of the supplementation of exogenous MT. It is possible that these proteins are associated with the functioning of MT. Sun et al. (2021) also evaluated the gene regulation capability of MT, which upregulates anti-stress genes. Numerous investigations resulted in the identification of three major concerns. In the first the endogenous MT concentration in Arabidopsis seedlings was promptly and significantly increased during HS treatment, and administering Arabidopsis with exogenous MT provided enhanced thermotolerance in the plant. Secondly, the fact that exogenous MT and HS administrations both bring about an elevation in the transcript level of AtHSFA1s showed that AtHSFA1s could be a part in the MT–HS responses that take place in Arabidopsis. In addition, the transcript levels of HS genes that were triggered by AtHSFA1s were also linked to MT-mediated thermotolerance. Thus, MT plays a pivotal role in Arabidopsis thermotolerance via activating heat-responsive genes that were triggered by AtHSFA1s.
Model representing the machinery of HSFs in melatonin-activated thermostability (Shi et al. 2015).
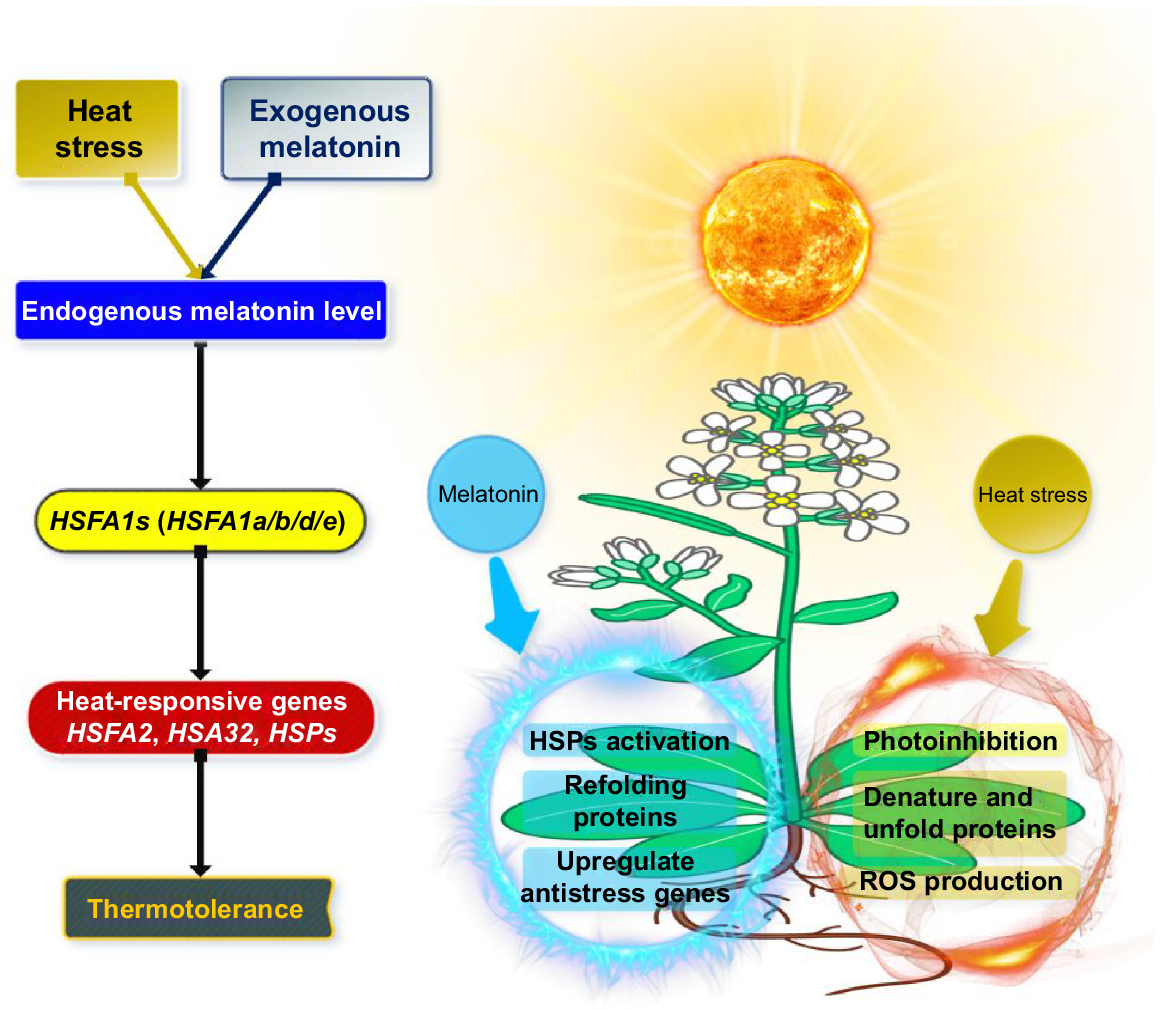
Defence against PsPto infection
Plant growth depends on non-reducing disaccharide sucrose, which regulates higher plant metabolism. Sucrose, a primary carbohydrate, is synthesised by photosynthesis and distributed to sink tissues from the phloem to sustain plant development. The source–sink concentration gradient drives long-distance assimilate transportation (Aluko et al. 2021). Furthermore, sugar also functions as a signalling molecule. Sucrose relies on irreversible hydrolysis of cell wall invertase (CWI) and vacuolar invertase (VI) into glucose and fructose (Tauzin and Giardina 2014). Auxin and MT possess the same precursor tryptophan and are structurally similar. It may act as a hormone or stimulation of invertase-related sucrose metabolism due to the precise management of plant growth and metabolism by hormonal pathways and sugar signalling, notably IAA (Li et al. 2022c). Several genes involved in the biosynthesis of MT have been synthesised, however it is unknown whether MT itself causes auxin-like effects or if it is transformed into IAA.
Zhao et al. (2015) determined that foliar application with MT resulted in higher invertase activities and increased root and leaf fresh weights. After treatment with MT, increased expression of CWIs and VIs genes was observed. In addition to this, specific invertase inhibitor proteins regulate invertase activity post-translationally. They have been found in Arabidopsis, in which C/VIF1 suppresses just VI activity, whereas C/VIF2 suppresses VI as well as CWI activity (Zhao et al. 2015). Zhao et al. (2015) experimented on Arabidopsis and revealed that the administration of exogenous MT had a role in the plant’s defensive mechanism against the aggressive bacterial pathogen Pseudomonas syringae pv. tomato DC3000 (PsPto). Plant infections enhance both carbon and energy sources like sucrose, glucose and fructose by altering their hosts’ metabolic activity. CWI transcripts are upregulated after disease attack, as discovered by researchers investigating the connection between response of plants to bacterial infections and CWI activity. Previous experiments indicate that acarbose’s post-translational CWI inhibition increased PsPto replication in Arabidopsis (Ferrieri et al. 2015). In adult Arabidopsis leaves, only C/VIF2 is expressed, hence repressing its expression may unleash invertase activity and boost plant resilience. The MT may have strengthened Arabidopsis’ PsPto resistance by downregulating C/VIF2 or upregulating CWI, which promoted CWI activity and the plant defensive system.
When it comes to plants, structural barriers are very necessary to prevent the transmission of infections. The recognition of an assault and MT-induced CWI activity triggers the production of cell wall components (galactose, cellulose and xylose), which serve as an initial barrier to pathogens that are trying to invade the cell and protects plants against different infections (Lamalakshmi Devi et al. 2017). Callose, a b-(1,3)-glucan cell wall polymer, regulates plasmodesmata and sieve plate permeability to prevent pathogen penetration (Mathur and Pramanik 2020). As we know, sugars are needed for callose deposition to boost plant defence. CWI also led host plants to deposit callose, reinforcing cell walls and creating a physical barrier against pathogens (He et al. 2021).
During systemic acquired resistance (SAR) activation, salicylic acid (SA) is essential. Abiotic stressors, infections and developmental triggers, excluding CWI, increase its production (Hartmann and Zeier 2019). The NPR1 protein controls the SA signalling pathway’s primary branch by binding to the TGA transcription factor and activating defence responsive genes including PR1 (Kim et al. 2022). Yang et al. (2015) states that exogenous MT increased SA production and upregulated marker genes via the JA (PDF1.2) and SA (PR1) pathways during PsPto infection. According to Zhao et al. (2015) experiment, Arabidopsis with knockout serotonin N-acetyltransferase had lower MT and SA levels and was vulnerable to a non-virulent infectious agent. The MT triggered two separate defensive mechanisms for diminished PsPto vulnerability in Arabidopsis, while SA mediated plant resistance exhibited no association with higher CWI functionality. Several metabolites and plant hormones may affect plant resistance, but SA signalling pathways are the cornerstone of plant defences against infections (Fig. 7) (Zhang et al. 2020). It may also be an antibacterial secondary metabolite in plants. Plants generate antimicrobial substances camalexin and glucosinolates, which hinder pathogen replication. It is interesting to note that the production of MT, glucosinolates and camalexin all use tryptophan as a precursor (Teng et al. 2022; Serag et al. 2023).
Inhibits seed germination
Many environmental and internal factors influence the germination rate of seed, which is a crucial step in early plant growth. MT has also been linked to seed germination during stress, but the molecular pathways are unexplored (Zhang et al. 2017c; Chen et al. 2021). ABA promotes and inhibits seed dormancy. Seed dormancy is caused by ABA accumulation during seed maturation. During seed germination, intracellular ABA quantities in the seed decline after vernalisation and imbibition (Javaid et al. 2020; Xie et al. 2022a). In a similar fashion, we noticed that the amount of Phyto-MT is maximum in the dry seeds and lowers as the germination process starts. Accordingly, it is possible that ABA and MT will work together to prevent the germination of seeds. The relationship of MT-ABA in inducing stomatal conductance is compatible with MT and ABA governing seed germination. These findings demonstrate the synergistic impact of MT and ABA on seed germination.
Lv et al. (2021) examined MT regulation of Arabidopsis seed germination with phytohormones ABA, gibberellic acid (GA) and IAA. The phenotypic study indicated that high MT doses inhibited seed germination, but low quantities had no impact. After being treated with MT, the levels of ABA and auxin were found to have risen, although the levels of GA did not substantially decrease. This finding lends credence to the hypothesis that the elevation in hormonal concentrations in auxin and ABA are the primary components that inhibit seed germination. As previously explained, auxin and MT share a biosynthetic precursor tryptophan (Pérez-Llorca et al. 2019), therefore, it is plausible that there could be a reciprocal mechanism involved in generating endogenous auxin-MT in response to MT application, potentially contributing to supporting greater germination rates of Arabidopsis on medium supplemented with both auxin and MT versus MT individually (Wang et al. 2016). Based on these data, it seemed as if MT had a dose-dependent effect on a large number of physiological activities occurring in plants. To optimise MT consumption, the dosage of exogenous MT must be considered.
ABA signalling is essential for inhibition of seed germination by MT. Experiments conducted in the past have shown that MT enhances the stability of DELLA proteins, which in turn modulates the antagonistic effects that GA and MT have on each other when it comes to the modulation of floral transitions (Erland and Saxena 2018). According to the findings presented by Lv et al. (2021), exogenous MT and GA both have antagonistic effects on the germination of seeds. Hence, GA and the antagonistic impact of MT may affect several essential growth attributes. This suggests that auxin inhibits seed germination through an ABA-dependent route. MT and IAA share a biosynthetic precursor and are indole derivatives. Hence, when the seeds are administered with both MT and auxin, the suppressive activity of MT on the germination of seeds will be affected by the IAA treatment.
Accelerate ROS induction and seeds germination
The germination of seeds involves several complicated physiological processes, hormones, gene expression sequences and signalling molecules. Hydrogen peroxide (H2O2) and nitric oxide (NO), both of which are significant signalling chemicals, collaborate to bring about the process of germination of seeds (Liu et al. 2021). In most cases, the addition of H2O2 to seed treatment helps the seed germinate more quickly, particularly when combined with a booster of NO (Hajihashemi et al. 2020). In seed germination, H2O2 directly regulates GA production and indirectly regulates ABA catabolism. The concentrations of H2O2 rise during seed imbibition and germination, which is consistent with the promoting roles of external H2O2 administration (Li et al. 2021a). Yet very little is understood about the upstream-signalling substances that cause ROS production, even though ROS serve critical signalling functions during the early stages of both senescence and seed germination (Talaat 2019). Since they play crucial roles in numerous biological processes, plant hormones like ethylene and ABA also promote ROS formation in plants. In senescence of leaves and seed germination, 2-hydroxymelatonin (2-OHM) induces tissue-specific ROS as a signalling molecule, compared to ethylene and ABA (Lee and Back 2021). These results suggest ROS are important signalling molecules for upstream of GA and ABA.
On the other hand, MT is a powerful antioxidant that modulates ROS homeostasis to control numerous environmental and biological factors. It was recently theorised that the pleiotropic physiological activity of MT in plants is regulated by a synergistic effect of MT and its metabolites like 2-OHM (2-hydroxymelatonin) (Song et al. 2022). This is for the reason that 2-OHM also works as an essential signalling molecule but performs a job that is entirely opposite from the antioxidant activity that MT performs. For instance, MT is an effective antioxidant, but 2-OHM is a super oxidant because the treatment of plants with it produces ROS in a respiratory burst NADPH oxidase homolog (RBOH)-dependent mechanism (Lee and Back 2021). It is significant to observe that the triggering of melatonin-2-hydroxylase (M2H) enzymes in rice resulted in the speedy transformation of MT into 2-OHM (Lee et al. 2016). 2-OHM production after MT administration is closely controlled by circadian rhythm and stress, because M2H shows better regulation at the tissue level (Gao et al. 2023). Arabidopsis was the first species in which the ROS induction-related biological mechanism of 2-OHM was explored.
Lee and Back (2022) explored additional undiscovered capabilities of 2-OHM in Arabidopsis. They focused their attention on a possible function of 2-OHM in the germination of seeds, because ROS are essential substances in plants that promote seed germination. 2-OHM administration increased ROS generation in Arabidopsis seeds and improved the germination rates. Importantly, GA serves as an endogenous elicitor in the process of MT synthesis, supplying substrates for M2H enzymes to participate in the production of 2-OHM (Liu et al. 2022c). The recent research shows that MT is an antioxidant and 2-OHM is a super oxidant, thus we believe that their equilibrium may coordinate a large number of biological activities, from the germination of seeds to embryogenesis and senescence (Fig. 8).
Model exhibiting 2-OHM and melatonin facilitated seeds germination (Lee and Back 2022). EXP2, GA-responsive expansin 2; GA3ox2, gibberellin 3-oxidase 2; KS, ent-kaurene synthase.
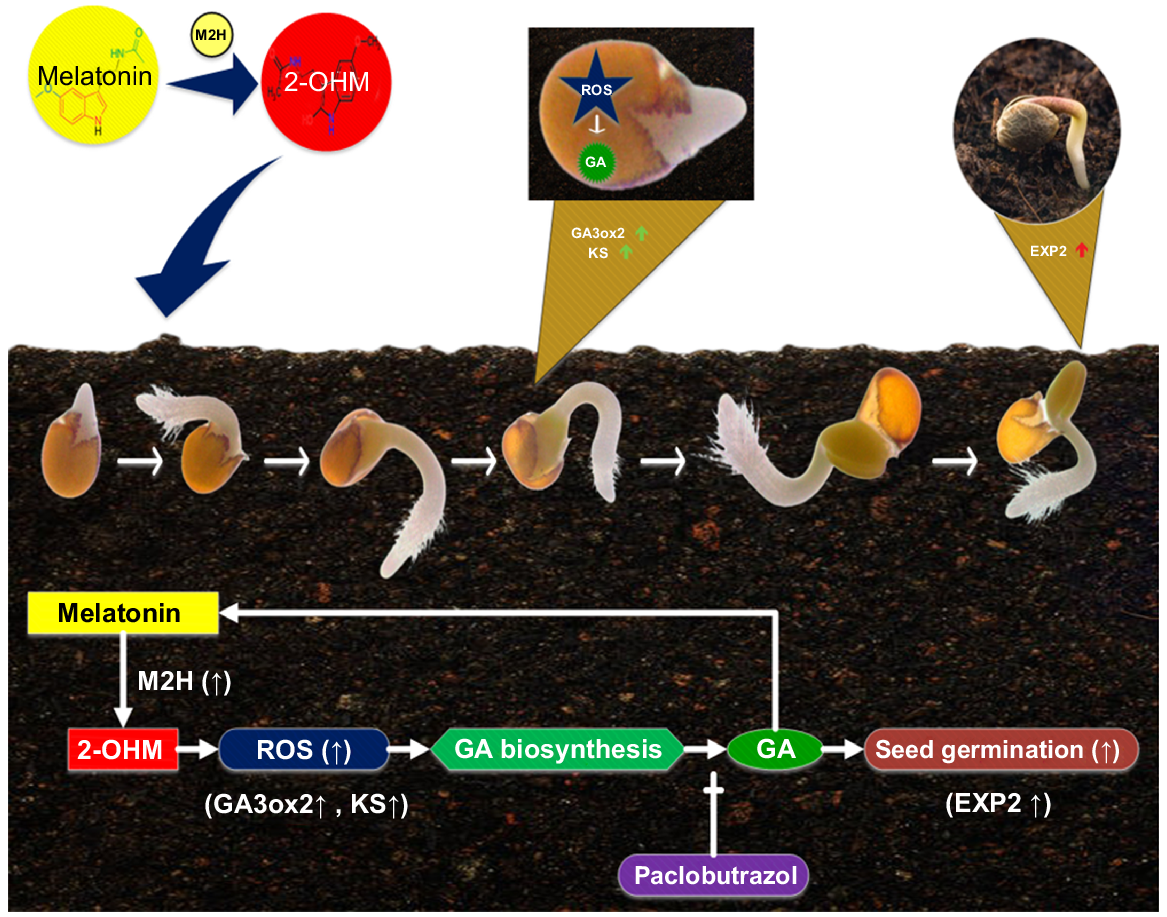
Future perspectives
The MT has emerged in recent years as a multi-functional extensive signalling molecule and master-regulator of plant resistance against unfavourable abiotic and biotic circumstances. Due to its unique capabilities as a plant cell growth-type regulator and in boosting plant tolerance to biotic and abiotic stressors, MT has attracted considerable attention (Wang et al. 2022c). Several upstream components of the MT related defensive signalling pathways still need justification. When Arabidopsis’ first phyto-MT receptor, AtPMTR1, was found (Li et al. 2020), this information gap was partly filled. Since several transcriptome strategies have been explored, this key finding has explained the upstream mechanism for the stomata closure phenotype. Nevertheless, additional MT-receptor-induced signalling pathways under biological and environmental plant stress still need to be explored. Current proteomic studies that found that MT upregulates PAMP-triggered immunity (PTI) and effector-triggered immunity (ETI) defence-related genes highlight the need to develop a signal-transduction mechanism for this phenomenon. Due to their association in shielding plants from detrimental infections, siderophore synthesis, nitrogen fixation, phosphate solubilisation, plant growth-type promotion rhizobacteria (PGPR) and plant hormone accumulation, have been extensively investigated (Tiwari et al. 2022). PGPR produces MT and raises its intracellular concentrations in plant organs, agreeing with numerous findings (EL Sabagh et al. 2022; Sharma et al. 2023). Nevertheless, further research is needed to determine how MT and PGPR affect plant resistance toward several abiotic stresses.
The synergistic treatment of MT with antifungals increases plant disease resilience with lower chemical fungicide levels, highlighting the most important features of MT in relation to abiotic and biotic factors (Anderson and Kim 2021). ROS generation is the initial cellular signal of an oxidative stress, which promotes biotic and abiotic stress consequences. The MT-synthesising genes are upregulated by this ROS explosion, raising endogenous MT production (Zhao et al. 2021b). Stressors negatively affect photosynthetic activity, membrane permeability and other physiological and cellular activities. Intracellular MT may affect the regulation of multiple genes and regulatory factors to mitigate or restore the damaging impacts of environmental function on physiological functions. It may also immediately scavenge lipid peroxides, ROS/RNS (reactive nitrogen species) and hazardous compounds, managing permeability of plasma membrane and redox network functionality (Malik et al. 2022; Sati et al. 2023). As a result, MT protects against the ROS explosion by changing the activity of numerous sensitive stress genes. Whenever plants are exposed to problematic physiological circumstances, such as when they are exposed to adverse environmental challenges like pathogen invasions, the significance of MT obviously becomes more noticeable and precise.
The MT contents in certain prominent types of transgenic plants that have incorporated genes from vertebrates have been reported to be dramatically raised. Modern transgenic crops may utilise modified MT biosynthesis to improve agricultural yields by surviving biotic and abiotic stressors. As MT is important and therapeutic for humans, so the pharmaceutical sector should focus on the viable human advantages of linked pharmacological preparations for plants, humans and animals. Modern and traditional breeding methods will likely create vegetables and fruits with elevated MT levels in the upcoming years (Cocetta and Natalini 2022; Ameen et al. 2023b). Little data exist on the potential significance of MT in infection and pest management. Such a field of study must be vigorously pursued with a characterisation of the particular defensive mechanisms. It will be essential to determine wither the presence of MT increases the phyto-remediative ability of the hyperaccumulator plant species, otherwise the molecular mechanism must be further described. It has been frequently reported that MT enhances root development, although its effects on nutrient intake and transport have yet to be explored. In a broader sense, there is a little existing knowledge on how exogenous application of MT affects the plant’s ability to accumulate the hormone and how this influences the development and growth type of plants. MT is widely present in plants (Table 3), however not all organs produce this indoleamine. Furthermore, studies must be conducted on the mechanism that regulates its mobility throughout the plant. Auxin-like behaviour and pleiotropic activities of MT have been identified by various researchers.
Plant/food/drink | Phyto-MT content | References | |
---|---|---|---|
Apple | 48 pg/g | Zhang et al. (2018) | |
Banana | 0.655 ng/g | Bhattacharjee and Dey (2018) | |
Beer | 52–170 pg/mL | Salehi et al. (2019) | |
Beef | 2.1 ± 0.13 ng/g | Bonomini et al. (2018) | |
Cereals | 1000–1300 pg/g | Arnao and Hernández-Ruiz (2015) | |
Coffee | 780 ng/mL | Johns and Padumanonda (2016) | |
Cow milk | 3–25 pg/mL | Kennaway (2020) | |
Chamomile | 300 ng/g | Cheng et al. (2021) | |
Chicken meat and skin | 2.3 ± 0.23 ng/g | Bonomini et al. (2018) | |
Extra virgin olive oil | 70–119 pg/mL | Paredes et al. (2008) | |
Green tea | 250 ng/g | Herrera et al. (2018) | |
Human milk | 0–42 pg/mL | Kennaway (2020) | |
Orange | 0.15 ng/g | Johns and Johns (2016) | |
Pineapple | 0.28 ng/g | Cheng et al. (2021) | |
Pork | 2.4 ± 0.2 ng/g | Bonomini et al. (2018) | |
Salmon | 3.6 ± 0.22 ng/g | Bonomini et al. (2018) | |
Solid dried eggs | 6.3 ± 0.95 ng/g | Bonomini et al. (2018) | |
Strawberry | 12 pg/g | Arnao (2014) | |
Tomato | 32 pg/g | Kennaway (2020) | |
Walnut flour | 7.7 ± 0.9 ng/g | Garcia et al. (2015) | |
Wine | 4000–5000 pg/mL | Bonomini et al. (2018) |
The MT is now in high demand because of the stressful nature of the modern world. Microbial fermentation seems to be the technique that has the highest chances of succeeding; although, as the annual requirement keeps expanding, a sustainable and effective protocol of biosynthesis is required in order to satisfy the market demand (Back 2021). The MT is produced by a variety of alternate pathways depending on the species. The first two stages of MT biosynthesis are accomplished in reverse order in plants relative to animals, and the Km values of the enzymes responsible are quite dissimilar (Mannino et al. 2021; Chen et al. 2023b).
There are now a limitless variety of techniques to alter enzymes relying on ‘in silico’ research because of the rapidly developing computer technology. Proteomics and bioinformatics ideas have been used to increase enzyme activity using protein design tools. The Alpha-Fold system, developed by DeepMind, enhances protein structure estimation by combining innovative neural network models and instructional strategies relying on geometrical, evolutionary and physical aspects of protein structure (Bäuerlein and Baumeister 2021; Vasina et al. 2022). It might be implemented as a computer assisted enzyme customisation tool in the future. Because of the advancement of metabolic engineering, there is now a higher appreciation for the viability of its use in the economic manufacturing of MT, and it is believed that considerable progress will be made in the coming years.
Although MT acts like an auxin, its significance in vascular reconnection must be explored. Lately, biologists have discovered that auxin actively participates in vascular connection formation and grafting (Sharma and Zheng 2019). Although the physiological and biochemical significance of MT in plants are somewhat understood, there isn’t any research on MT-specific biosynthesis, or existence, or absence of MT receptors, or activity inhibitors in the plants. Taking into consideration the biochemical, physiological, epigenetic and genetic properties of MT at an extensive scale across species, it seems that MT may work as an essential molecule to impact agricultural crops in particular (Fig. 9). It may also demonstrate its worth in boosting crop production and nutritional value, which may contribute to resolving problems with food security all over the world.
Summary of roles of melatonin in plants. AsA, ascorbate; GSH, reduced glutathione; APX, ascorbate peroxidase; POX, peroxidase; GR, glutathione reductase; ETH, ethylene.
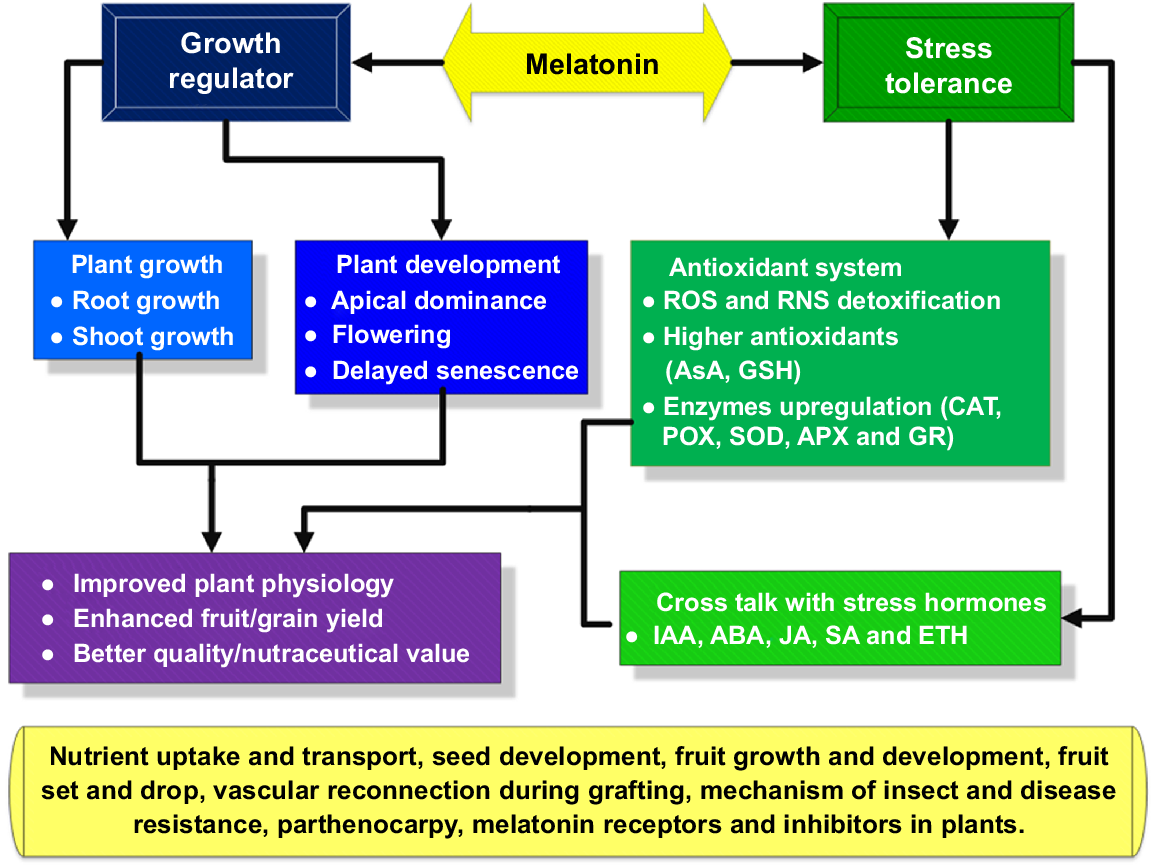
To summarise, the ability of MT to reinforce plants subjected to diverse environmental and biological factors makes it a fascinating natural ingredient to research for agricultural enhancement and disease management. In addition, there is a shortage of enough data about the consequences that MT may have on the infection of plants by insects, viruses and nematodes; therefore, additional research is recommended in this sector.
Conclusion
Our analyses provide several conclusions. MT is an essential signalling molecule, as shown by extensive studies. It is produced endogenously by the plant and is essential for growth and development. We investigated how applying MT to Arabidopsis thaliana affected its ability to deal with both biotic and abiotic stress. Exogenous MT may increase seed viability, germination of seed, lateral root growth and abiotic and biotic stress resilience (cold, drought, high light, intense heat, infection and diseases). In addition, MT has a major impact on the control of gene expression as well as in the interrelationship with other phytohormones. This plays a noteworthy function in many of the biological activities that take place in plants, regardless of whether the environmental circumstances are favourable or unfavourable. Nevertheless, endogenous MT may not be adequate for challenging circumstances. To maintain resistance in plants and normal development, exogenous MT and other methods have been used to promote endogenous MT. Consequently, its use in agriculture might minimise stress and improve plant growth across changing environments. To summarise, MT exhibited particular significance across a variety of plant scientific fields. Despite this, there is currently little data available about how the application of MT affects resilience to viruses, insects, or nematodes; this needs more research.
Data availability
The data that support this study will be shared upon reasonable request to the corresponding author.
References
AbuQamar S, Moustafa K, Tran LS (2017) Mechanisms and strategies of plant defense against Botrytis cinerea. Critical Reviews in Biotechnology 37(2), 262-274.
| Crossref | Google Scholar |
Adhikari L, Baral R, Paudel D, Min D, Makaju SO, Poudel HP, Acharya JP, Missaoui AM (2022) Cold stress in plants: strategies to improve cold tolerance in forage species. Plant Stress 4, 100081.
| Crossref | Google Scholar |
Ahammed GJ, Li X (2022) Melatonin-induced detoxification of organic pollutants and alleviation of phytotoxicity in selected horticultural crops. Horticulturae 8(12), 1142.
| Crossref | Google Scholar |
Ahammed GJ, Xu W, Liu A, Chen S (2019) Endogenous melatonin deficiency aggravates high temperature-induced oxidative stress in Solanum lycopersicum L. Environmental and Experimental Botany 161, 303-311.
| Crossref | Google Scholar |
Ahammed GJ, Mao Q, Yan Y, Wu M, Wang Y, Ren J, Guo P, Liu A, Chen S (2020) Role of melatonin in arbuscular mycorrhizal fungi-induced resistance to fusarium wilt in cucumber. Phytopathology 110(5), 999-1009.
| Crossref | Google Scholar |
Ahmad I, Munsif F, Mihoub A, Jamal A, Saeed MF, Babar S, Fawad M, Zia A (2022) Beneficial effect of melatonin on growth and chlorophyll content in wheat (Triticum aestivum L.) grown under salt stress conditions. Gesunde Pflanzen 74(4), 997-1009.
| Crossref | Google Scholar |
Alam MN, Yang L, Yi X, Wang Q, Robin AHK (2022) Role of melatonin in inducing the physiological and biochemical processes associated with heat stress tolerance in tall fescue (Festuca arundinaceous). Journal of Plant Growth Regulation 41(7), 2759-2768.
| Crossref | Google Scholar |
Alizadeh N, Dianatkhah M, Alimohamadi Y, Moradi H, Akbarpour S, Akrami M, Mansouri F, Faraji N, Rezaie Z, Alizadeh M, Hosamirudsari H (2022) High dose melatonin as an adjuvant therapy in intubated patients with COVID-19: a randomized clinical trial. Journal of Taibah University Medical Sciences 17(3), 454-460.
| Crossref | Google Scholar |
Altaf MA, Shahid R, Ren M-X, Mora-Poblete F, Arnao MB, Naz S, Anwar M, Altaf MM, Shahid S, Shakoor A, Sohail H, Ahmar S, Kamran M, Chen J-T (2021) Phytomelatonin: an overview of the importance and mediating functions of melatonin against environmental stresses. Physiologia Plantarum 172(2), 820-846.
| Crossref | Google Scholar |
Aluko OO, Li C, Wang Q, Liu H (2021) Sucrose utilization for improved crop yields: a review article. International Journal of Molecular Sciences 22(9), 4704.
| Crossref | Google Scholar |
Ameen M, Mahmood A, Ahmad M, Mansoor Javaid M, Nadeem MA, Asif M, Balal RM, Khan BA (2023a) Impacts of climate change on fruit physiology and quality. In ‘Climate-Resilient agriculture: crop responses and agroecological perspectives’. (Ed. M Hasanuzzaman) pp. 93–124. (Springer International Publishing: Cham, Switzerland)
Ameen M, Zafar A, Javaid MM, Zia MA, Mahmood A, Naqve M, Bibi S (2023b) Climate-resilient technology for maize production. In ‘Climate-resilient agriculture: agro-biotechnological advancement for crop production’. (Ed. M Hasanuzzaman) pp. 157–188. (Springer International Publishing: Cham, Switzerland)
Anderson AJ, Kim YC (2021) The plant-stress metabolites, hexanoic aacid and melatonin, are potential “Vaccines” for plant health promotion. Plant Pathology Journal 37(5), 415-427.
| Crossref | Google Scholar |
Arabia A, Muñoz P, Pallarés N, Munné-Bosch S (2023) Experimental approaches in studying active biomolecules modulating fruit ripening: melatonin as a case study. Plant Physiology 192, 1747-1767.
| Crossref | Google Scholar | PubMed |
Arnao MB (2014) Phytomelatonin: discovery, content, and role in plants. Advances in Botany 2014, 815769.
| Crossref | Google Scholar |
Arora D, Bhatla SC (2017) Melatonin and nitric oxide regulate sunflower seedling growth under salt stress accompanying differential expression of Cu/Zn SOD and Mn SOD. Free Radical Biology and Medicine 106, 315-328.
| Crossref | Google Scholar |
Ayyaz A, Shahzadi AK, Fatima S, Yasin G, Zafar ZU, Athar H-u-R, Farooq MA (2022) Uncovering the role of melatonin in plant stress tolerance. Theoretical and Experimental Plant Physiology 34(3), 335-346.
| Crossref | Google Scholar |
Back K (2021) Melatonin metabolism, signaling and possible roles in plants. The Plant Journal 105(2), 376-391.
| Crossref | Google Scholar |
Badria FA (2002) Melatonin, serotonin, and tryptamine in some Egyptian food and medicinal plants. Journal of Medicinal Food 5(3), 153-157.
| Crossref | Google Scholar |
Bai J, Song MJ, Gao J, Li G (2022) Whole genome duplication and dispersed duplication characterize the evolution of the plant PINOID gene family across plant species. Gene 829, 146494.
| Crossref | Google Scholar |
Bajwa VS, Shukla MR, Sherif SM, Murch SJ, Saxena PK (2014) Role of melatonin in alleviating cold stress in Arabidopsis thaliana. Journal of Pineal Research 56(3), 238-245.
| Crossref | Google Scholar |
Beilstein MA, Nagalingum NS, Clements MD, Manchester SR, Mathews S (2010) Dated molecular phylogenies indicate a Miocene origin for Arabidopsis thaliana. Proceedings of the National Academy of Sciences 107(43), 18724-18728.
| Crossref | Google Scholar |
Bhardwaj K, Raina M, Sanfratello GM, Pandey P, Singh A, Rajwanshi R, Negi NP, Rustagi A, Khushboo , Kumar D (2022) Exogenous melatonin counteracts salinity and cadmium stress via photosynthetic machinery and antioxidant modulation in Solanum lycopersicum L. Journal of Plant Growth Regulation 42, 6332-6348.
| Crossref | Google Scholar |
Bhattacharjee A, Dey BK (2018) Phytomelatonin: a comprehensive literature review and recent advance on medicinal meadow. International Journal of Hydrology 2(3), 396-403.
| Crossref | Google Scholar |
Bhowal B, Bhattacharjee A, Goswami K, Sanan-Mishra N, Singla-Pareek SL, Kaur C, Sopory S (2021) Serotonin and melatonin biosynthesis in plants: genome-wide identification of the genes and their expression reveal a conserved role in stress and development. International Journal of Molecular Sciences 22(20), 11034.
| Crossref | Google Scholar |
Blanco-Ulate B, Amrine KCH, Collins TS, Rivero RM, Vicente AR, Morales-Cruz A, Doyle CL, Ye Z, Allen G, Heymann H, Ebeler SE, Cantu D (2015) Developmental and metabolic plasticity of white-skinned grape berries in response to Botrytis cinerea during noble rot. Plant Physiology 169(4), 2422-2443.
| Crossref | Google Scholar |
Blask DE, Dauchy RT, Sauer LA, Krause JA (2004) Melatonin uptake and growth prevention in rat hepatoma 7288CTC in response to dietary melatonin: melatonin receptor-mediated inhibition of tumor linoleic acid metabolism to the growth signaling molecule 13-hydroxyoctadecadienoic acid and the potential role of phytomelatonin. Carcinogenesis 25(6), 951-960.
| Crossref | Google Scholar |
Bonomini F, Borsani E, Favero G, Rodella LF, Rezzani R (2018) Dietary melatonin supplementation could be a promising preventing/therapeutic approach for a variety of liver diseases. Nutrients 10(9), 1135.
| Crossref | Google Scholar |
Bose SK, Howlader P (2020) Melatonin plays multifunctional role in horticultural crops against environmental stresses: a review. Environmental and Experimental Botany 176, 104063.
| Crossref | Google Scholar |
Brengi SH, Khedr AAEM, Abouelsaad IA (2022) Effect of melatonin or cobalt on growth, yield and physiological responses of cucumber (Cucumis sativus L.) plants under salt stress. Journal of the Saudi Society of Agricultural Sciences 21(1), 51-60.
| Crossref | Google Scholar |
Byeon Y, Back K (2014) Melatonin synthesis in rice seedlings in vivo is enhanced at high temperatures and under dark conditions due to increased serotonin N-acetyltransferase and N-acetylserotonin methyltransferase activities. Journal of Pineal Research 56(2), 189-195.
| Crossref | Google Scholar |
Byeon Y, Lee H-J, Lee HY, Back K (2016) Cloning and functional characterization of the Arabidopsis N-acetylserotonin O-methyltransferase responsible for melatonin synthesis. Journal of Pineal Research 60(1), 65-73.
| Crossref | Google Scholar |
Bäuerlein FJB, Baumeister W (2021) Towards visual proteomics at high resolution. Journal of Molecular Biology 433(20), 167187.
| Crossref | Google Scholar |
Chang J, Guo Y, Li J, Su Z, Wang C, Zhang R, Wei C, Ma J, Zhang X, Li H (2021) Positive interaction between H2O2 and Ca2+ mediates melatonin-induced CBF pathway and cold tolerance in watermelon (Citrullus lanatus L.). Antioxidants 10(9), 1457.
| Crossref | Google Scholar |
Chang Q, Zhang L, Chen S, Gong M, Liu L, Hou X, Mi Y, Wang X, Wang J, Zhang Y, Sun Y (2023) Exogenous melatonin enhances the yield and secondary metabolite contents of Prunella vulgaris by modulating antioxidant system, root architecture and photosynthetic capacity. Plants 12(5), 1129.
| Crossref | Google Scholar |
Chen G, Huo Y, Tan D-X, Liang Z, Zhang W, Zhang Y (2003) Melatonin in Chinese medicinal herbs. Life sciences 73(1), 19-26.
| Crossref | Google Scholar |
Chen C-Q, Fichna J, Bashashati M, Li Y-Y, Storr M (2011) Distribution, function and physiological role of melatonin in the lower gut. World Journal of Gastroenterology: WJG 17(34), 3888-3898.
| Crossref | Google Scholar |
Chen L, Lu B, Liu L, Duan W, Jiang D, Li J, Zhang K, Sun H, Zhang Y, Li C, Bai Z (2021) Melatonin promotes seed germination under salt stress by regulating ABA and GA3 in cotton (Gossypium hirsutum L.). Plant Physiology and Biochemistry 162, 506-516.
| Crossref | Google Scholar |
Chen C, Zhang X, Wei X, Zhu Y, Chen W, Han Y (2022) Postharvest biological control of Botrytis cinerea and the mechanisms underlying the induction of disease resistance in grapes by Lactobacillus plantarum CM-3. Biological Control 172, 104982.
| Crossref | Google Scholar |
Chen F, Li Y, Zia-ur-Rehman M, Hussain SM, Qayyum MF, Rizwan M, Alharby HF, Alabdallah NM, Alharbi BM, Ali S (2023a) Combined effects of zinc oxide nanoparticles and melatonin on wheat growth, chlorophyll contents, cadmium (Cd) and zinc uptake under Cd stress. Science of The Total Environment 864, 161061.
| Crossref | Google Scholar |
Chen J, Zhang Y, Yin H, Liu W, Hu X, Li D, Lan C, Gao L, He Z, Cui F, Fernie AR, Chen W (2023b) The pathway of melatonin biosynthesis in common wheat (Triticum aestivum). Journal of Pineal Research 74(2), e12841.
| Crossref | Google Scholar |
Cheng G, Ma T, Deng Z, Gutiérrez-Gamboa G, Ge Q, Xu P, Zhang Q, Zhang J, Meng J, Reiter RJ, Fang Y, Sun X (2021) Plant-derived melatonin from food: a gift of nature. Food & Function 12(7), 2829-2849.
| Crossref | Google Scholar |
Cocetta G, Natalini A (2022) Ethylene: management and breeding for postharvest quality in vegetable crops. A review. Frontiers in Plant Science 13, 968315.
| Crossref | Google Scholar |
Debnath B, Li M, Liu S, Pan T, Ma C, Qiu D (2020) Melatonin-mediate acid rain stress tolerance mechanism through alteration of transcriptional factors and secondary metabolites gene expression in tomato. Ecotoxicology and Environmental Safety 200, 110720.
| Crossref | Google Scholar |
Ding F, Ren L, Xie F, Wang M, Zhang S (2022) Jasmonate and melatonin act synergistically to potentiate cold tolerance in tomato plants. Frontiers in Plant Science 12, 763284.
| Crossref | Google Scholar |
Dmitrieva VA, Tyutereva EV, Voitsekhovskaja OV (2020) Singlet oxygen in plants: generation, detection, and signaling roles. International Journal of Molecular Sciences 21(9), 3237.
| Crossref | Google Scholar |
Dogra V, Kim C (2019) Chloroplast protein homeostasis is coupled with retrograde signaling. Plant Signaling & Behavior 14(11), 1656037.
| Crossref | Google Scholar |
EL Sabagh A, Islam MS, Hossain A, Iqbal MA, Mubeen M, Waleed M, Reginato M, Battaglia M, Ahmed S, Rehman A, Arif M, Athar H-U-R, Ratnasekera D, Danish S, Raza MA, Rajendran K, Mushtaq M, Skalicky M, Brestic M, Soufan W, Fahad S, Pandey S, Kamran M, Datta R, Abdelhamid MT (2022) Phytohormones as growth regulators during abiotic stress tolerance in plants. Frontiers in Agronomy 4, 765068.
| Crossref | Google Scholar |
Erland LAE, Saxena PK (2018) Melatonin in plant morphogenesis. In Vitro Cellular & Developmental Biology – Plant 54(1), 3-24.
| Crossref | Google Scholar |
Erland LAE, Chattopadhyay A, Jones AMP, Saxena PK (2016) Melatonin in plants and plant culture systems: variability, stability and efficient quantification. Frontiers in Plant Science 7, 1721.
| Crossref | Google Scholar |
Faghih S, Zamani Z, Fatahi R, Liaghat A (2019) Effects of deficit irrigation and kaolin application on vegetative growth and fruit traits of two early ripening apple cultivars. Biological Research 52(1), 43.
| Crossref | Google Scholar |
Fan J, Xie Y, Zhang Z, Chen L (2018) Melatonin: a multifunctional factor in plants. International Journal of Molecular Sciences 19(5), 1528.
| Crossref | Google Scholar |
Feng X, Wang M, Zhao Y, Han P, Dai Y (2014) Melatonin from different fruit sources, functional roles, and analytical methods. Trends in Food Science & Technology 37(1), 21-31.
| Crossref | Google Scholar |
Feng W, Li J, Long S, Wei S (2019) A DREB1 gene from zoysiagrass enhances Arabidopsis tolerance to temperature stresses without growth inhibition. Plant Science 278, 20-31.
| Crossref | Google Scholar |
Ferreira-Saab M, Formey D, Torres M, Aragón W, Padilla EA, Tromas A, Sohlenkamp C, Schwan-Estrada KRF, Serrano M (2018) Compounds released by the biocontrol yeast Hanseniaspora opuntiae protect plants against Corynespora cassiicola and Botrytis cinerea. Frontiers in Microbiology 9, 1596.
| Crossref | Google Scholar |
Ferrieri AP, Arce CCM, Machado RAR, Meza-Canales ID, Lima E, Baldwin IT, Erb M (2015) A Nicotiana attenuata cell wall invertase inhibitor (NaCWII) reduces growth and increases secondary metabolite biosynthesis in herbivore-attacked plants. New Phytologist 208(2), 519-530.
| Crossref | Google Scholar | PubMed |
Gai W-X, Ma X, Li Y, Xiao J-J, Khan A, Li Q-H, Gong Z-H (2020) CaHsfA1d improves plant thermotolerance via regulating the expression of stress- and antioxidant-related genes. International Journal of Molecular Sciences 21(21), 8374.
| Crossref | Google Scholar | PubMed |
Gao H, Lu ZM, Yang Y, Wang DN, Yang T, Cao MM, Cao W (2018) Melatonin treatment reduces chilling injury in peach fruit through its regulation of membrane fatty acid contents and phenolic metabolism. Food Chemistry 245, 659-666.
| Crossref | Google Scholar | PubMed |
Gao W, Feng Z, Bai Q, He J, Wang Y (2019) Melatonin-mediated regulation of growth and antioxidant capacity in salt-tolerant naked oat under salt stress. International Journal of Molecular Sciences 20(5), 1176.
| Crossref | Google Scholar | PubMed |
Gao Y, Chen H, Chen D, Hao G (2023) Genetic and evolutionary dissection of melatonin response signaling facilitates the regulation of plant growth and stress responses. Journal of Pineal Research 74(2), e12850.
| Crossref | Google Scholar | PubMed |
Garcia CP, Lamarque AL, Comba A, Berra MA, Silva RA, Labuckas DO, Das UN, Eynard AR, Pasqualini ME (2015) Synergistic anti-tumor effects of melatonin and PUFAs from walnuts in a murine mammary adenocarcinoma model. Nutrition 31(4), 570-577.
| Crossref | Google Scholar |
Gong X, Shi S, Dou F, Song Y, Ma F (2017) Exogenous melatonin alleviates alkaline stress in Malus hupehensis Rehd. by regulating the biosynthesis of polyamines. Molecules 22(9), 1542.
| Crossref | Google Scholar |
Guan L, Li Y, Huang K, Cheng Z-M (2020) Auxin regulation and MdPIN expression during adventitious root initiation in apple cuttings. Horticulture Research 7, 143.
| Crossref | Google Scholar |
Gull T, Mahmood A, Shaheen C, Javaid MM, Zia MA, Naqve M, Bibi S, Nadeem MA, Ameen M, Nargis J, Khan SR (2023) Climate change and nutrient use efficiency of plants. In ‘Climate-resilient agriculture: crop responses and agroecological perspectives’. (Ed. M Hasanuzzaman) pp. 291–312. (Springer International Publishing: Cham, Switzerland)
Gunata M, Parlakpinar H, Acet HA (2020) Melatonin: a review of its potential functions and effects on neurological diseases. Revue Neurologique 176(3), 148-165.
| Crossref | Google Scholar | PubMed |
Gusain S, Joshi S, Joshi R (2023) Sensing, signalling, and regulatory mechanism of cold-stress tolerance in plants. Plant Physiology and Biochemistry 197, 107646.
| Crossref | Google Scholar | PubMed |
Gökbayrak Z, Engin H, Kiraz H (2020) Effects of Melatonin and IAA on Adventitious Root Formation in Rootstock 5BB and cv. Cabernet Sauvignon (Vitis vinifera L.). Kahramanmaraş Sütçü İmam Üniversitesi Tarım ve Doğa Dergisi 23(4), 835-841.
| Google Scholar |
Hajihashemi S, Skalicky M, Brestic M, Pavla V (2020) Cross-talk between nitric oxide, hydrogen peroxide and calcium in salt-stressed Chenopodium quinoa Willd. at seed germination stage. Plant Physiology and Biochemistry 154, 657-664.
| Crossref | Google Scholar |
Hartmann M, Zeier J (2019) N-hydroxypipecolic acid and salicylic acid: a metabolic duo for systemic acquired resistance. Current Opinion in Plant Biology 50, 44-57.
| Crossref | Google Scholar | PubMed |
Hassan MU, Ghareeb RY, Nawaz M, Mahmood A, Shah AN, Abdel-Megeed A, Abdelsalam NR, Hashem M, Alamri S, Thabit MA, Qari SH (2022a) Melatonin: a vital pro-tectant for crops against heat stress: mechanisms and prospects. Agronomy 12(5), 1116.
| Crossref | Google Scholar |
Hassan MU, Mahmood A, Awan MI, Maqbool R, Aamer M, Alhaithloul HAS, Huang G, Skalicky M, Brestic M, Pandey S, El Sabagh A, Qari SH (2022b) Melatonin-induced protection against plant abiotic stress: mechanisms and prospects. Frontiers in Plant Science 13, 902694.
| Crossref | Google Scholar |
He H, He L-F (2020) Crosstalk between melatonin and nitric oxide in plant development and stress responses. Physiologia Plantarum 170(2), 218-226.
| Crossref | Google Scholar |
He F, Wu X, Zhang Q, Li Y, Ye Y, Li P, Chen S, Peng Y, Hardeland R, Xia Y (2021) Bacteriostatic potential of melatonin: therapeutic standing and mechanistic insights. Frontiers in Immunology 12, 683879.
| Crossref | Google Scholar | PubMed |
Herrera T, Aguilera Y, Rebollo-Hernanz M, Bravo E, Benítez V, Martínez-Sáez N, Arribas SM, del Castillo MD, Martín-Cabrejas MA (2018) Teas and herbal infusions as sources of melatonin and other bioactive non-nutrient components. LWT 89, 65-73.
| Crossref | Google Scholar |
Hoque MN, Tahjib-Ul-Arif M, Hannan A, Sultana N, Akhter S, Hasanuzzaman M, Akter F, Hossain MS, Sayed MA, Hasan MT, Skalicky M, Li X, Brestič M (2021) Melatonin modulates plant tolerance to heavy metal stress: morphological responses to molecular mechanisms. International Journal of Molecular Sciences 22(21), 11445.
| Crossref | Google Scholar |
Hussain S, Rasheed M, Saleem MH, Ahmed ZI, Hafeez A, Jilani G, Alamri S, Hashem M, Ali S (2022) Salt tolerance in maize with melatonin priming to achieve sustainability in yield in salt affected soils. Pakistan Journal of Botany 55, 19-35.
| Google Scholar |
Iqbal Z, Shariq Iqbal M, Singh SP, Buaboocha T (2020) Ca2+/Calmodulin Complex Triggers CAMTA transcriptional machinery under stress in plants: signaling cascade and molecular regulation. Frontiers in Plant Science 11, 598327.
| Crossref | Google Scholar |
Islam MT, Mamun MA, Lee B-R, La VH, Jung W-J, Bae D-W, Kim T-H (2021) Role of salicylic acid signaling in the biotrophy-necrotrophy transition of Xanthomonas campestris pv. campestris infection in Brassica napus. Physiological and Molecular Plant Pathology 113, 101578.
| Crossref | Google Scholar |
Javaid MM, Abbas Z, Waheed H, Mahmood A, Abbas T, Amin MM, Asif M (2020) Studies on germination ecology and seedling characteristics of Cleome viscosa as affected by various environmental factors. Pakistan Journal of Botany 52(6), 1911-1919.
| Crossref | Google Scholar |
Kashyap SP, Kumari N, Mishra P, Moharana DP, Aamir M (2021) Tapping the potential of Solanum lycopersicum L. pertaining to salinity tolerance: perspectives and challenges. Genetic Resources and Crop Evolution 68(6), 2207-2233.
| Crossref | Google Scholar |
Kennaway DJ (2020) Melatonin rich foods in our diet: food for thought or wishful thinking? Food & Function 11(11), 9359-9369.
| Crossref | Google Scholar |
Khan A, Numan M, Khan AL, Lee I-J, Imran M, Asaf S, Al-Harrasi A (2020a) Melatonin: awakening the defense mechanisms during plant oxidative stress. Plants 9(4), 407.
| Crossref | Google Scholar | PubMed |
Khan MN, Khan Z, Luo T, Liu J, Rizwan M, Zhang J, Xu Z, Wu H, Hu L (2020b) Seed priming with gibberellic acid and melatonin in rapeseed: consequences for improving yield and seed quality under drought and non-stress conditions. Industrial Crops and Products 156, 112850.
| Crossref | Google Scholar |
Khan TA, Fariduddin Q, Nazir F, Saleem M (2020c) Melatonin in business with abiotic stresses in plants. Physiology and Molecular Biology of Plants 26(10), 1931-1944.
| Crossref | Google Scholar |
Khan M, Ali S, Manghwar H, Saqib S, Ullah F, Ayaz A, Zaman W (2022) Melatonin function and crosstalk with other phytohormones under normal and stressful conditions. Genes 13(10), 1699.
| Crossref | Google Scholar |
Khan M, Ali S, Al Azzawi TNI, Saqib S, Ullah F, Ayaz A, Zaman W (2023) The key roles of ROS and RNS as a signaling molecule in plant–microbe interactions. Antioxidants 12(2), 268.
| Crossref | Google Scholar |
Kidokoro S, Shinozaki K, Yamaguchi-Shinozaki K (2022) Transcriptional regulatory network of plant cold-stress responses. Trends in Plant Science 27(9), 922-935.
| Crossref | Google Scholar |
Kim Y-W, Youn J-H, Roh J, Kim J-M, Kim S-K, Kim T-W (2022) Brassinosteroids enhance salicylic acid-mediated immune responses by inhibiting BIN2 phosphorylation of clade I TGA transcription factors in Arabidopsis. Molecular Plant 15(6), 991-1007.
| Crossref | Google Scholar |
Knox-Brown P, Rindfleisch T, Günther A, Balow K, Bremer A, Walther D, Miettinen MS, Hincha DK, Thalhammer A (2020) Similar yet different–structural and functional diversity among Arabidopsis thaliana LEA_4 proteins. International Journal of Molecular Sciences 21(8), 2794.
| Crossref | Google Scholar | PubMed |
Kohli SK, Khanna K, Bhardwaj R, Abd Allah EF, Ahmad P, Corpas FJ (2019) Assessment of subcellular ROS and NO metabolism in higher plants: multifunctional signaling molecules. Antioxidants 8(12), 641.
| Crossref | Google Scholar | PubMed |
Kołodziejczyk I, Dzitko K, Szewczyk R, Posmyk MM (2016) Exogenous melatonin improves corn (Zea mays L.) embryo proteome in seeds subjected to chilling stress. Journal of Plant Physiology 193, 47-56.
| Crossref | Google Scholar | PubMed |
Lamalakshmi Devi E, Kumar S, Basanta Singh T, Sharma SK, Beemrote A, Devi CP, Chongtham SK, Singh CH, Yumlembam RA, Haribhushan A, Prakash N, Wani SH (2017) Adaptation strategies and defence mechanisms of plants during environmental stress. In ‘Medicinal plants and environmental challenges’. (Eds M Ghorbanpour, A Varma) pp. 359–413. (Springer International Publishing: Cham, Switzerland)
Langaroudi IK, Piri S, Chaeikar SS, Salehi B (2023) Evaluating drought stress tolerance in different Camellia sinensis L. cultivars and effect of melatonin on strengthening antioxidant system. Scientia Horticulturae 307, 111517.
| Crossref | Google Scholar |
Lee HY, Back K (2018) Melatonin induction and its role in high light stress tolerance in Arabidopsis thaliana. Journal of Pineal Research 65(3), e12504.
| Crossref | Google Scholar | PubMed |
Lee HY, Back K (2021) 2-hydroxymelatonin, rather than melatonin, is responsible for RBOH-dependent reactive oxygen species production leading to premature senescence in plants. Antioxidants 10(11), 1728.
| Crossref | Google Scholar | PubMed |
Lee HY, Back K (2022) 2-Hydroxymelatonin promotes seed germination by increasing reactive oxygen species production and gibberellin synthesis in Arabidopsis thaliana. Antioxidants 11(4), 737.
| Crossref | Google Scholar | PubMed |
Lee HY, Byeon Y, Back K (2014) Melatonin as a signal molecule triggering defense responses against pathogen attack in Arabidopsis and tobacco. Journal of Pineal Research 57(3), 262-268.
| Crossref | Google Scholar |
Lee K, Zawadzka A, Czarnocki Z, Reiter RJ, Back K (2016) Molecular cloning of melatonin 3-hydroxylase and its production of cyclic 3-hydroxymelatonin in rice (Oryza sativa). Journal of Pineal Research 61(4), 470-478.
| Crossref | Google Scholar | PubMed |
Lee K, Lee HY, Back K (2018) Rice histone deacetylase 10 and Arabidopsis histone deacetylase 14 genes encode N-acetylserotonin deacetylase, which catalyzes conversion of N-acetylserotonin into serotonin, a reverse reaction for melatonin biosynthesis in plants. Journal of Pineal Research 64(2), e12460.
| Crossref | Google Scholar | PubMed |
Lerner AB, Case JD, Takahashi Y, Lee TH, Mori W (1958) Isolation of melatonin, the pineal gland factor that lightens MELANOCYTES. Journal of the American Chemical Society 80(10), 2587.
| Crossref | Google Scholar |
Li C, Wang P, Wei Z, Liang D, Liu C, Yin L, Jia D, Fu M, Ma F (2012) The mitigation effects of exogenous melatonin on salinity-induced stress in Malus hupehensis. Journal of Pineal Research 53(3), 298-306.
| Crossref | Google Scholar |
Li H, Chang J, Chen H, Wang Z, Gu X, Wei C, Zhang Y, Ma J, Yang J, Zhang X (2017) Exogenous melatonin confers salt stress tolerance to watermelon by improving photosynthesis and redox homeostasis. Frontiers in Plant Science 8, 295.
| Crossref | Google Scholar |
Li X, Wei J-P, Scott ER, Liu J-W, Guo S, Li Y, Zhang L, Han W-Y (2018) Exogenous melatonin alleviates cold stress by promoting antioxidant defense and redox homeostasis in Camellia sinensis L. Molecules 23(1), 165.
| Crossref | Google Scholar |
Li J, Yang Y, Sun K, Chen Y, Chen X, Li X (2019a) Exogenous melatonin enhances cold, salt and drought stress tolerance by improving antioxidant defense in tea plant (Camellia sinensis (L.) O. Kuntze). Molecules 24(9), 1826.
| Crossref | Google Scholar | PubMed |
Li Z-G, Xu Y, Bai L-K, Zhang S-Y, Wang Y (2019b) Melatonin enhances thermotolerance of maize seedlings (Zea mays L.) by modulating antioxidant defense, methylglyoxal detoxification, and osmoregulation systems. Protoplasma 256(2), 471-490.
| Crossref | Google Scholar | PubMed |
Li D, Wei J, Peng Z, Ma W, Yang Q, Song Z, Sun W, Yang W, Yuan L, Xu X, Chang W, Rengel Z, Shen J, Reiter RJ, Cui X, Yu D, Chen Q (2020) Daily rhythms of phytomelatonin signaling modulate diurnal stomatal closure via regulating reactive oxygen species dynamics in Arabidopsis. Journal of Pineal Research 68(3), e12640.
| Crossref | Google Scholar |
Li H, Guo Y, Lan Z, Zhang Z, Ahammed GJ, Chang J, Zhang Y, Wei C, Zhang X (2021a) Melatonin antagonizes ABA action to promote seed germination by regulating Ca2+ efflux and H2O2 accumulation. Plant Science 303, 110761.
| Crossref | Google Scholar |
Li R, Jiang M, Song Y, Zhang H (2021b) Melatonin alleviates low-temperature stress via ABI5-mediated signals during seed germination in rice (Oryza sativa L.). Frontiers in Plant Science 12 727596.
| Crossref | Google Scholar |
Li Y, Liu K, Tong G, Xi C, Liu J, Zhao H, Wang Y, Ren D, Han S (2021c) MPK3/MPK6-mediated phosphorylation of ERF72 positively regulates resistance to Botrytis cinerea through directly and indirectly activating the transcription of camalexin biosynthesis enzymes. Journal of Experimental Botany 73(1), 413-428.
| Crossref | Google Scholar |
Li R, Yang R, Zheng W, Wu L, Zhang C, Zhang H (2022a) Melatonin promotes SGT1-involved signals to ameliorate drought stress adaption in rice. International Journal of Molecular Sciences 23(2), 599.
| Crossref | Google Scholar |
Li S, Huan C, Liu Y, Zheng X, Bi Y (2022b) Melatonin induces improved protection against Botrytis cinerea in cherry tomato fruit by activating salicylic acid signaling pathway. Scientia Horticulturae 304, 111299.
| Crossref | Google Scholar |
Li S, Wang Y, Gao X, Lan J, Fu B (2022c) Comparative physiological and transcriptome analysis reveal the molecular mechanism of melatonin in regulating salt tolerance in alfalfa (Medicago sativa L.). Frontiers in Plant Science 13, 919177.
| Crossref | Google Scholar |
Li X, Liang X, Li W, Yao A, Liu W, Wang Y, Yang G, Han D (2022d) Isolation and functional analysis of MbCBF2, a Malus baccata (L.) Borkh CBF transcription factor gene, with functions in tolerance to cold and salt stress in transgenic Arabidopsis thaliana. International Journal of Molecular Sciences 23(17), 9827.
| Crossref | Google Scholar | PubMed |
Li Z, Zhang S, Xue J, Mu B, Song H, Liu Y (2022e) Exogenous melatonin treatment induces disease resistance against Botrytis cinerea on post-harvest grapes by activating defence responses. Foods 11(15), 2231.
| Crossref | Google Scholar | PubMed |
Liang C, Li A, Yu H, Li W, Liang C, Guo S, Zhang R, Chu C (2017) Melatonin regulates root architecture by modulating auxin response in rice. Frontiers in Plant Science 8, 134.
| Crossref | Google Scholar | PubMed |
Liang X, Luo G, Li W, Yao A, Liu W, Xie L, Han M, Li X, Han D (2022) Overexpression of a Malus baccata CBF transcription factor gene, MbCBF1, increases cold and salinity tolerance in Arabidopsis thaliana. Plant Physiology and Biochemistry 192, 230-242.
| Crossref | Google Scholar |
Liu H-c, Charng Y-y (2013) Common and distinct functions of Arabidopsis class A1 and A2 heat shock factors in diverse abiotic stress responses and development. Plant Physiology 163(1), 276-290.
| Crossref | Google Scholar |
Liu S, Ziegler J, Zeier J, Birkenbihl RP, Somssich IE (2017) Botrytis cinerea B05.10 promotes disease development in Arabidopsis by suppressing WRKY33-mediated host immunity. Plant, Cell & Environment 40(10), 2189-2206.
| Crossref | Google Scholar | PubMed |
Liu C, Chen L, Zhao R, Li R, Zhang S, Yu W, Sheng J, Shen L (2019) Melatonin induces disease resistance to Botrytis cinerea in tomato fruit by activating jasmonic acid signaling pathway. Journal of Agricultural and Food Chemistry 67(22), 6116-6124.
| Crossref | Google Scholar |
Liu L, Huang L, Sun C, Wang L, Jin C, Lin X (2021) Cross-talk between hydrogen peroxide and nitric oxide during plant development and responses to stress. Journal of Agricultural and Food Chemistry 69(33), 9485-9497.
| Crossref | Google Scholar |
Liu G, Hu Q, Zhang X, Jiang J, Zhang Y, Zhang Z (2022a) Melatonin biosynthesis and signal transduction in plants in response to environmental conditions. Journal of Experimental Botany 73(17), 5818-5827.
| Crossref | Google Scholar |
Liu W, Chen T, Liu Y, Le QT, Wang R, Lee H, Xiong L (2022b) The Plastidial DIG5 Protein Affects Lateral Root Development by Regulating Flavonoid Biosynthesis and Auxin Transport in Arabidopsis. International Journal of Molecular Sciences 23(18), 10642.
| Crossref | Google Scholar |
Liu Y, Wang X, Lv H, Cao M, Li Y, Yuan X, Zhang X, Guo Y-D, Zhang N (2022c) Anabolism and signaling pathways of phytomelatonin. Journal of Experimental Botany 73(17), 5801-5817.
| Crossref | Google Scholar |
Liu J, Wang J, Zhang T, Li M, Yan H, Liu Q, Wei Y, Ji X, Zhao Q (2023) Exogenous melatonin positively regulates rice root growth through promoting the antioxidant system and mediating the auxin signaling under root-zone hypoxia stress. Agronomy 13(2), 386.
| Crossref | Google Scholar |
Lv Y, Pan J, Wang H, Reiter RJ, Li X, Mou Z, Zhang J, Yao Z, Zhao D, Yu D (2021) Melatonin inhibits seed germination by crosstalk with abscisic acid, gibberellin, and auxin in Arabidopsis. Journal of Pineal Research 70(4), e12736.
| Crossref | Google Scholar |
Ma X, Jin Q, Wang Y, Wang X, Wang X, Yang M, Ye C, Yang Z, Xu Y (2023) Comparative transcriptome analysis reveals the regulatory mechanisms of two tropical water lilies in response to cold stress. BMC Genomics 24(1), 82.
| Crossref | Google Scholar | PubMed |
Malik Z, Afzal S, Dawood M, Abbasi GH, Khan MI, Kamran M, Zhran M, Hayat MT, Aslam MN, Rafay M (2022) Exogenous melatonin mitigates chromium toxicity in maize seedlings by modulating antioxidant system and suppresses chromium uptake and oxidative stress. Environmental Geochemistry and Health 44(5), 1451-1469.
| Crossref | Google Scholar |
Mannino G, Pernici C, Serio G, Gentile C, Bertea CM (2021) Melatonin and phytomelatonin: chemistry, biosynthesis, metabolism, distribution and bioactivity in plants and animals—an overview. International Journal of Molecular Sciences 22(18), 9996.
| Crossref | Google Scholar | PubMed |
Market Data Forecast (2022) Melatonin Market Segmentation By Type (Natural melatonin and Synthetic melatonin); By Application (Dietary supplement, Construction industry, Medical industry, and Others); and Region – Industry Forecast of 2022 to 2027. Available at https://www.marketdataforecast.com/market-reports/melatonin-market [Retrieved 18/03/2023]
Middleton AM, Dal Bosco C, Chlap P, Bensch R, Harz H, Ren F, Bergmann S, Wend S, Weber W, Hayashi K-i, Zurbriggen MD, Uhl R, Ronneberger O, Palme K, Fleck C, Dovzhenko A (2018) Data-driven modeling of intracellular auxin fluxes indicates a dominant role of the ER in controlling nuclear auxin uptake. Cell Reports 22(11), 3044-3057.
| Crossref | Google Scholar |
Mittler R, Zandalinas SI, Fichman Y, Van Breusegem F (2022) Reactive oxygen species signalling in plant stress responses. Nature Reviews Molecular Cell Biology 23(10), 663-679.
| Crossref | Google Scholar |
Nawaz MA, Huang Y, Bie Z, Ahmed W, Reiter RJ, Niu M, Hameed S (2016) Melatonin: current status and future perspectives in plant science. Frontiers in Plant Science 6, 1230.
| Crossref | Google Scholar |
Nawaz MA, Jiao Y, Chen C, Shireen F, Zheng Z, Imtiaz M, Bie Z, Huang Y (2018) Melatonin pretreatment improves vanadium stress tolerance of watermelon seedlings by reducing vanadium concentration in the leaves and regulating melatonin biosynthesis and antioxidant-related gene expression. Journal of Plant Physiology 220, 115-127.
| Crossref | Google Scholar | PubMed |
Nawaz K, Chaudhary R, Sarwar A, Ahmad B, Gul A, Hano C, Abbasi BH, Anjum S (2021) Melatonin as master regulator in plant growth, development and stress alleviator for sustainable agricultural production: current status and future perspectives. Sustainability 13(1), 294.
| Crossref | Google Scholar |
Noman M, Aysha J, Ketehouli T, Yang J, Du L, Wang F, Li H (2021) Calmodulin binding transcription activators: an interplay between calcium signalling and plant stress tolerance. Journal of Plant Physiology 256, 153327.
| Crossref | Google Scholar |
Paredes SD, Korkmaz A, Manchester LC, Tan D-X, Reiter RJ (2008) Phytomelatonin: a review. Journal of Experimental Botany 60(1), 57-69.
| Crossref | Google Scholar |
Paredes SD, Rancan L, García C, Vara E, Tresguerres JAF (2016) Occurrence of serotonin, melatonin, and their derivatives in plants. In ‘Serotonin and melatonin: their functional role in plants, food, phytomedicine, and human health’. (Eds GA Ravishankar, A Ramakrishna) pp. 37–52. (CRC Press: Boca Raton, FL, USA)
Praveen B, Sharma P (2019) A review of literature on climate change and its impacts on agriculture productivity. Journal of Public Affairs 19(4), e1960.
| Crossref | Google Scholar |
Pérez-Llamas F, Hernández-Ruiz J, Cuesta A, Zamora S, Arnao MB (2020) Development of a phytomelatonin-rich extract from cultured plants with excellent biochemical and functional properties as an alternative to synthetic melatonin. Antioxidants 9(2), 158.
| Crossref | Google Scholar | PubMed |
Pérez-Llorca M, Muñoz P, Müller M, Munné-Bosch S (2019) Biosynthesis, metabolism and function of auxin, salicylic acid and melatonin in climacteric and non-climacteric fruits. Frontiers in Plant Science 10, 136.
| Crossref | Google Scholar |
Qari SH, Hassan MU, Chattha MU, Mahmood A, Naqve M, Nawaz M, Barbanti L, Alahdal MA, Aljabri M (2022) Melatonin induced cold tolerance in plants: physiological and molecular responses. Frontiers in Plant Science 13, 843071.
| Crossref | Google Scholar | PubMed |
Qu G, Wu W, Ba L, Ma C, Ji N, Cao S (2022) Melatonin enhances the postharvest disease resistance of blueberries fruit by modulating the jasmonic acid signaling pathway and phenylpropanoid metabolites. Front Chem 10, 957581.
| Crossref | Google Scholar | PubMed |
Reiter RJ, Tan D-x, Manchester LC, Simopoulos AP, Maldonado MD, Flores LJ, Terron MP (2007) Melatonin in edible plants (phytomelatonin): identification, concentrations, bioavailability and proposed functions. World Review of Nutrition and Dietetics 97, 211-230.
| Crossref | Google Scholar | PubMed |
Ren S, Rutto L, Katuuramu D (2019) Melatonin acts synergistically with auxin to promote lateral root development through fine tuning auxin transport in Arabidopsis thaliana. PLoS ONE 14(8), e0221687.
| Crossref | Google Scholar | PubMed |
Ritonga FN, Chen S (2020) Physiological and molecular mechanism involved in cold stress tolerance in plants. Plants 9(5), 560.
| Crossref | Google Scholar |
Roy M, Niu J, Irshad A, Kareem HA, Hassan MU, Xu N, Sui X, Guo Z, Amo A, Wang Q (2021) Exogenous melatonin protects alfalfa (Medicago sativa L.) seedlings from drought-induced damage by modulating reactive oxygen species metabolism, mineral balance and photosynthetic efficiency. Plant Stress 2, 100044.
| Crossref | Google Scholar |
Sagan L (1967) On the origin of mitosing cells. Journal of Theoretical Biology 14(3), 225-274 IN1–IN6.
| Crossref | Google Scholar |
Salehi B, Sharopov F, Fokou PVT, Kobylinska A, Jonge Ld, Tadio K, Sharifi-Rad J, Posmyk MM, Martorell M, Martins N, Iriti M (2019) Melatonin in medicinal and food plants: occurrence, bioavailability, and health potential for humans. Cells 8(7), 681.
| Crossref | Google Scholar | PubMed |
Sati H, Khandelwal A, Pareek S (2023) Effect of exogenous melatonin in fruit postharvest, crosstalk with hormones, and defense mechanism for oxidative stress management. Food Frontiers 4(1), 233–261. 10.1002/fft2.180
Serag A, Salem MA, Gong S, Wu J-L, Farag MA (2023) Decoding metabolic reprogramming in plants under pathogen attacks, a comprehensive review of emerging metabolomics technologies to maximize their applications. Metabolites 13(3), 424.
| Crossref | Google Scholar | PubMed |
Sham A, Moustafa K, Al-Shamisi S, Alyan S, Iratni R, AbuQamar S (2017) Microarray analysis of Arabidopsis WRKY33 mutants in response to the necrotrophic fungus Botrytis cinerea. PLoS ONE 12(2), e0172343.
| Crossref | Google Scholar |
Shamloo-Dashtpagerdi R, Aliakbari M, Lindlöf A, Tahmasebi S (2022) A systems biology study unveils the association between a melatonin biosynthesis gene, O-methyl transferase 1 (OMT1) and wheat (Triticum aestivum L.) combined drought and salinity stress tolerance. Planta 255(5), 99.
| Crossref | Google Scholar |
Sharma A, Zheng B (2019) Molecular responses during plant grafting and its regulation by auxins, cytokinins, and gibberellins. Biomolecules 9(9), 397.
| Crossref | Google Scholar |
Sharma P, Bakshi P, Kaur R, Sharma A, Bhardwaj R, El-Sheikh MA, Tyagi A, Ahmad P (2023) Inoculation of plant-growth-promoting rhizobacteria and earthworms in the rhizosphere reinstates photosynthetic attributes and secondary metabolites in Brassica juncea L. under chromium toxicity. Plant and Soil 483(1), 573-587.
| Crossref | Google Scholar |
Shi H, Chan Z (2014) The cysteine2/histidine2-type transcription factor ZINC FINGER OF ARABIDOPSIS THALIANA 6-activated C-REPEAT-BINDING FACTOR pathway is essential for melatonin-mediated freezing stress resistance in Arabidopsis. Journal of Pineal Research 57(2), 185-191.
| Crossref | Google Scholar | PubMed |
Shi H, Tan D-X, Reiter RJ, Ye T, Yang F, Chan Z (2015) Melatonin induces class A1 heat-shock factors (HSFA1s) and their possible involvement of thermotolerance in Arabidopsis. Journal of Pineal Research 58(3), 335-342.
| Crossref | Google Scholar |
Song R, Ritonga FN, Yu H, Ding C, Zhao X (2022) Plant melatonin: regulatory and protective role. Horticulturae 8(9), 810.
| Crossref | Google Scholar |
Su J, Yang X, Shao Y, Chen Z, Shen W (2021) Molecular hydrogen-induced salinity tolerance requires melatonin signalling in Arabidopsis thaliana. Plant, Cell & Environment 44(2), 476-490.
| Crossref | Google Scholar |
Sun Y, Liu Z, Lan G, Jiao C, Sun Y (2019) Effect ofexogenous melatonin on resistance of cucumber to downy mildew. Scientia Horticulturae 255, 231-241.
| Crossref | Google Scholar |
Sun C, Liu L, Wang L, Li B, Jin C, Lin X (2021) Melatonin: a master regulator of plant development and stress responses. Journal of Integrative Plant Biology 63(1), 126-145.
| Crossref | Google Scholar | PubMed |
Tan J, Zhou Z, Feng H, Xing J, Niu Y, Deng Z (2021) Data-independent acquisition-based proteome and phosphoproteome profiling reveals early protein phosphorylation and dephosphorylation events in arabidopsis seedlings upon cold exposure. International Journal of Molecular Sciences 22(23), 12856.
| Crossref | Google Scholar |
Tanveer M, Mahmood A, Sarfraz B, Zia MA, Javaid MM, Bibi S, Naqve M, Nadeem MA, Azeem M, Jabbar A (2023) Mechanism and approaches to enhancing heat stress tolerance in crop plants. In ‘Climate-resilient agriculture: agro-biotechnological advancement for crop production’. (Ed. M Hasanuzzaman) pp. 499–520. (Springer International Publishing: Cham, Switzerland)
Tauzin AS, Giardina T (2014) Sucrose and invertases, a part of the plant defense response to the biotic stresses. Frontiers in Plant Science 5, 293.
| Crossref | Google Scholar | PubMed |
Teng Z, Zheng W, Yu Y, Hong S-B, Zhu Z, Zang Y (2022) Melatonin regulated glucosinolate profile via modulation of genes related with sulfur and nitrogen metabolism in Brassica rapa ssp. pekinensis. Industrial Crops and Products 177, 114538.
| Crossref | Google Scholar |
Tiryaki I, Keles H (2012) Reversal of the inhibitory effect of light and high temperature on germination of Phacelia tanacetifolia seeds by melatonin. Journal of Pineal Research 52(3), 332-339.
| Crossref | Google Scholar |
Tiwari RK, Lal MK, Kumar R, Chourasia KN, Naga KC, Kumar D, Das SK, Zinta G (2021) Mechanistic insights on melatonin-mediated drought stress mitigation in plants. Physiologia Plantarum 172(2), 1212-1226.
| Crossref | Google Scholar | PubMed |
Tiwari RK, Lal MK, Kumar R, Mangal V, Altaf MA, Sharma S, Singh B, Kumar M (2022) Insight into melatonin-mediated response and signaling in the regulation of plant defense under biotic stress. Plant Molecular Biology 109(4), 385-399.
| Crossref | Google Scholar | PubMed |
Umapathi M, Kalarani MK, Srinivasan S, Kalaiselvi P (2022) Alleviation of cadmium phytotoxicity through melatonin modulated physiological functions, antioxidants, and metabolites in tomato (Solanum lycopersicum L.). BioMetals 35(5), 1113-1132.
| Crossref | Google Scholar | PubMed |
Uzal O, Baslak L, Yasar F (2023) Effects of external melatonin treatments on morphological and physiological changes in cucumber (Cucumis sativus L.) seedlings against chilling stress. Gesunde Pflanzen 75(1), 115-125.
| Crossref | Google Scholar |
Vasina M, Velecký J, Planas-Iglesias J, Marques SM, Skarupova J, Damborsky J, Bednar D, Mazurenko S, Prokop Z (2022) Tools for computational design and high-throughput screening of therapeutic enzymes. Advanced Drug Delivery Reviews 183, 114143.
| Crossref | Google Scholar |
Včelařová L, Skalický V, Chamrád I, Lenobel R, Kubeš MF, Pěnčík A, Novák O (2021) Auxin metabolome profiling in the Arabidopsis endoplasmic reticulum using an optimised organelle isolation protocol. International Journal of Molecular Sciences 22(17), 9370.
| Crossref | Google Scholar | PubMed |
Wang Q, An B, Wei Y, Reiter RJ, Shi H, Luo H, He C (2016) Melatonin regulates root meristem by repressing auxin synthesis and polar auxin transport in Arabidopsis. Frontiers in Plant Science 7, 1882.
| Crossref | Google Scholar | PubMed |
Wang L, Leister D, Guan L, Zheng Y, Schneider K, Lehmann M, Apel K, Kleine T (2020) The Arabidopsis SAFEGUARD1 suppresses singlet oxygen-induced stress responses by protecting grana margins. Proceedings of the National Academy of Sciences 117(12), 6918-6927.
| Crossref | Google Scholar | PubMed |
Wang K, He J, Gao Y, Han K, Liu J, Wang Y (2022a) Exogenous melatonin improved the growth and development of naked oat seedlings under cadmium stress. Environmental Science and Pollution Research 29(58), 88109-88118.
| Crossref | Google Scholar | PubMed |
Wang K, He J, Zhao N, Zhao Y, Qi F, Fan F, Wang Y (2022b) Effects of melatonin on growth and antioxidant capacity of naked oat (Avena nuda L) seedlings under lead stress. PeerJ 10, e13978.
| Crossref | Google Scholar |
Wang K, Xing Q, Ahammed GJ, Zhou J (2022c) Functions and prospects of melatonin in plant growth, yield, and quality. Journal of Experimental Botany 73(17), 5928-5946.
| Crossref | Google Scholar | PubMed |
Wang X, Meng H, Tang Y, Zhang Y, He Y, Zhou J, Meng X (2022d) Phosphorylation of an ethylene response factor by MPK3/MPK6 mediates negative feedback regulation of pathogen-induced ethylene biosynthesis in Arabidopsis. Journal of Genetics and Genomics 49(8), 810-822.
| Crossref | Google Scholar | PubMed |
Wang Z, Mu Y, Zhang L, Liu Z, Liu D, Jin Z, Pei Y (2023) Hydrogen sulfide mediated the melatonin induced stoma closure by regulating the K+ channel in Arabidopsis thaliana. Environmental and Experimental Botany 205, 105125.
| Crossref | Google Scholar |
Wasson AP, Pellerone FI, Mathesius U (2006) Silencing the flavonoid pathway in medicago truncatula inhibits root nodule formation and prevents auxin transport regulation by rhizobia. The Plant Cell 18(7), 1617-1629.
| Crossref | Google Scholar |
Wei Y, Hu W, Wang Q, Zeng H, Li X, Yan Y, Reiter RJ, He C, Shi H (2017) Identification, transcriptional and functional analysis of heat-shock protein 90s in banana (Musa acuminata L.) highlight their novel role in melatonin-mediated plant response to Fusarium wilt. Journal of Pineal Research 62(1), e12367.
| Crossref | Google Scholar | PubMed |
Wen D, Gong B, Sun S, Liu S, Wang X, Wei M, Yang F, Li Y, Shi Q (2016) Promoting roles of melatonin in adventitious root development of Solanum lycopersicum L. by regulating auxin and nitric oxide signaling. Frontiers in Plant Science 7, 718.
| Crossref | Google Scholar | PubMed |
Wichniak A, Kania A, Siemiński M, Cubała WJ (2021) Melatonin as a potential adjuvant treatment for COVID-19 beyond sleep disorders. International Journal of Molecular Sciences 22(16), 8623.
| Crossref | Google Scholar | PubMed |
Wu X, Ren J, Huang X, Zheng X, Tian Y, Shi L, Dong P, Li Z (2021) Melatonin: biosynthesis, content, and function in horticultural plants and potential application. Scientia Horticulturae 288, 110392.
| Crossref | Google Scholar |
Xia H, Zhou Y, Deng H, Lin L, Deng Q, Wang J, Lv X, Zhang X, Liang D (2021) Melatonin improves heat tolerance in Actinidia deliciosa via carotenoid biosynthesis and heat shock proteins expression. Physiologia Plantarum 172(3), 1582-1593.
| Crossref | Google Scholar | PubMed |
Xiao S, Liu L, Wang H, Li D, Bai Z, Zhang Y, Sun H, Zhang K, Li C (2019) Exogenous melatonin accelerates seed germination in cotton (Gossypium hirsutum L.). PLoS ONE 14(6), e0216575.
| Crossref | Google Scholar |
Xie M, Sun J, Gong D, Kong Y (2019) The roles of Arabidopsis C1-2i subclass of C2H2-type zinc-finger transcription factors. Genes 10(9), 653.
| Crossref | Google Scholar | PubMed |
Xie W, Li X, Wang S, Yuan M (2022a) OsWRKY53 promotes abscisic acid accumulation to accelerate leaf senescence and inhibit seed germination by downregulating abscisic acid catabolic genes in rice. Frontiers in Plant Science 12, 816156.
| Crossref | Google Scholar | PubMed |
Xie X, Ding D, Bai D, Zhu Y, Sun W, Sun Y, Zhang D (2022b) Melatonin biosynthesis pathways in nature and its production in engineered microorganisms. Synthetic and Systems Biotechnology 7(1), 544-553.
| Crossref | Google Scholar | PubMed |
Yan Y, Jing X, Tang H, Li X, Gong B, Shi Q (2019) Using transcriptome to discover a novel melatonin-induced sodic alkaline stress resistant pathway in Solanum lycopersicum L. Plant and Cell Physiology 60(9), 2051-2064.
| Crossref | Google Scholar | PubMed |
Yang Y-X, Ahammed GJ, Wu C, Fan S-y, Zhou Y-H (2015) Crosstalk among jasmonate, salicylate and ethylene signaling pathways in plant disease and immune responses. Current Protein and Peptide Science 16(5), 450-461.
| Crossref | Google Scholar | PubMed |
Yang R, Yu G, Li H, Li X, Mu C (2020) Overexpression of small heat shock protein LimHSP16.45 in Arabidopsis hsp17.6II mutant enhances tolerance to abiotic stresses. Russian Journal of Plant Physiology 67(2), 231-241.
| Crossref | Google Scholar |
Yang C, Li Z, Cao X, Duan W, Wei C, Zhang C, Jiang D, Li M, Chen K, Qiao Y, Liu H, Zhang B (2022a) Genome-wide analysis of Calmodulin Binding Transcription Activator (CAMTA) gene family in Peach (Prunus persica L. Batsch) and ectopic expression of PpCAMTA1 in Arabidopsis camta2,3 mutant restore plant development. International Journal of Molecular Sciences 23(18), 10500.
| Crossref | Google Scholar | PubMed |
Yang L, Bu S, Zhao S, Wang N, Xiao J, He F, Gao X (2022b) Transcriptome and physiological analysis of increase in drought stress tolerance by melatonin in tomato. PLoS ONE 17(5), e0267594.
| Crossref | Google Scholar |
Ye T, Yin X, Yu L, Zheng SJ, Cai WJ, Wu Y, Feng YQ (2019) Metabolic analysis of the melatonin biosynthesis pathway using chemical labeling coupled with liquid chromatography-mass spectrometry. Journal of Pineal Research 66(1), e12531.
| Crossref | Google Scholar | PubMed |
Yuan Q, Zhao L (2017) The Mulberry (Morus alba L.) Fruit—a review of characteristic components and health benefits. Journal of Agricultural and Food Chemistry 65(48), 10383-10394.
| Crossref | Google Scholar |
Zeng H, Bai Y, Wei Y, Reiter RJ, Shi H (2022) Phytomelatonin as a central molecule in plant disease resistance. Journal of Experimental Botany 73(17), 5874-5885.
| Crossref | Google Scholar | PubMed |
Zhang N, Zhao B, Zhang H-J, Weeda S, Yang C, Yang Z-C, Ren S, Guo Y-D (2013) Melatonin promotes water-stress tolerance, lateral root formation, and seed germination in cucumber (Cucumis sativus L.). Journal of Pineal Research 54(1), 15-23.
| Crossref | Google Scholar | PubMed |
Zhang J, Shi Y, Zhang X, Du H, Xu B, Huang B (2017a) Melatonin suppression of heat-induced leaf senescence involves changes in abscisic acid and cytokinin biosynthesis and signaling pathways in perennial ryegrass (Lolium perenne L.). Environmental and Experimental Botany 138, 36-45.
| Crossref | Google Scholar |
Zhang N, Zhang H-J, Sun Q-Q, Cao Y-Y, Li X, Zhao B, Wu P, Guo Y-D (2017b) Proteomic analysis reveals a role of melatonin in promoting cucumber seed germination under high salinity by regulating energy production. Scientific Reports 7(1), 503.
| Crossref | Google Scholar |
Zhang R, Sun Y, Liu Z, Jin W, Sun Y (2017c) Effects of melatonin on seedling growth, mineral nutrition, and nitrogen metabolism in cucumber under nitrate stress. Journal of Pineal Research 62(4), e12403.
| Crossref | Google Scholar | PubMed |
Zhang S, Zheng X, Reiter RJ, Feng S, Wang Y, Liu S, Jin L, Li Z, Datla R, Ren M (2017d) Melatonin attenuates potato late blight by disrupting cell growth, stress tolerance, fungicide susceptibility and homeostasis of gene expression in Phytophthora infestans. Frontiers in Plant Science 8, 1993.
| Crossref | Google Scholar | PubMed |
Zhang H, Liu X, Chen T, Ji Y, Shi K, Wang L, Zheng X, Kong J (2018) Melatonin in apples and juice: inhibition of browning and microorganism growth in apple juice. Molecules 23(3), 521.
| Crossref | Google Scholar | PubMed |
Zhang X, Ménard R, Li Y, Coruzzi GM, Heitz T, Shen W-H, Berr A (2020) Arabidopsis SDG8 potentiates the sustainable transcriptional induction of the pathogenesis-related genes PR1 and PR2 during plant defense response. Frontiers in Plant Science 11, 277.
| Crossref | Google Scholar |
Zhang H, Liu L, Wang Z, Feng G, Gao Q, Li X (2021) Induction of low temperature tolerance in wheat by pre-soaking and parental treatment with melatonin. Molecules 26(4), 1192.
| Crossref | Google Scholar | PubMed |
Zhang M, Gao C, Xu L, Niu H, Liu Q, Huang Y, Lv G, Yang H, Li M (2022a) Melatonin and indole-3-acetic acid synergistically regulate plant growth and stress resistance. Cells 11(20), 3250.
| Crossref | Google Scholar |
Zhang X, Liu W, Lv Y, Bai J, Li T, Yang X, Liu L, Zhou H (2022b) Comparative transcriptomics reveals new insights into melatonin-enhanced drought tolerance in naked oat seedlings. PeerJ 10, e13669.
| Crossref | Google Scholar | PubMed |
Zhang Z, Yuan L, Ma Y, Kang Z, Zhou F, Gao Y, Yang S, Li T, Hu X (2022c) Exogenous 5-aminolevulinic acid alleviates low-temperature damage by modulating the xanthophyll cycle and nutrient uptake in tomato seedlings. Plant Physiology and Biochemistry 189, 83-93.
| Crossref | Google Scholar | PubMed |
Zhang Y, Berman A, Shani E (2023) Plant hormone transport and localization: signaling molecules on the move. Annual Review of Plant Biology 74(1), 453-479.
| Crossref | Google Scholar | PubMed |
Zhao H, Xu L, Su T, Jiang Y, Hu L, Ma F (2015) Melatonin regulates carbohydrate metabolism and defenses against Pseudomonas syringae pv. tomato DC3000 infection in Arabidopsis thaliana. Journal of Pineal Research 59(1), 109-119.
| Crossref | Google Scholar | PubMed |
Zhao C, Yang M, Wu X, Wang Y, Zhang R (2021a) Physiological and transcriptomic analyses of the effects of exogenous melatonin on drought tolerance in maize (Zea mays L.). Plant Physiology and Biochemistry 168, 128-142.
| Crossref | Google Scholar | PubMed |
Zhao D, Wang H, Chen S, Yu D, Reiter RJ (2021b) Phytomelatonin: an emerging regulator of plant biotic stress resistance. Trends in Plant Science 26(1), 70-82.
| Crossref | Google Scholar | PubMed |
Zhu Y, Guo M-J, Song J-B, Zhang S-Y, Guo R, Hou D-R, Hao C-Y, An H-L, Huang X (2021) Roles of Endogenous Melatonin in Resistance to Botrytis cinerea Infection in an Arabidopsis Model [Original Research]. Frontiers in Plant Science 12, 683228.
| Crossref | Google Scholar | PubMed |
Zia SF, Berkowitz O, Bedon F, Whelan J, Franks AE, Plummer KM (2019) Direct comparison of Arabidopsis gene expression reveals different responses to melatonin versus auxin. BMC Plant Biology 19(1), 567.
| Crossref | Google Scholar | PubMed |