Root photosynthesis prevents hypoxia in the epiphytic orchid Phalaenopsis
Luca Brunello


A
B
C
Abstract
Orchids (Phalaenopsis spp.) growing in tropical and subtropical regions are epiphytes. As such, they grow on trees with the root system utilised to anchor themselves to tree branches. These roots are highly specialised, display a large diameter and are often green, suggesting the ability to carry out photosynthesis. However, the role of photosynthesis in orchid roots is controversial. Orchids that are leafless can photosynthesise in their roots, thus indicating that some orchid roots carry out photosynthesis in a similar manner to leaves. However, the primary site of photosynthesis in orchids are in their leaves, and the roots of epiphytic orchids may mostly conduct internal refixation of respiratory CO2. Besides contributing to the overall carbon metabolism of orchid plants, oxygen produced through root photosynthesis may also be important by alleviating potential root hypoxia. The bulky tissue of most epiphytic orchid roots suggests that oxygen diffusion in these roots can be limited. Here, we demonstrate that the bulky roots of a widely commercially cultivated orchid belonging to the genus Phalaenopsis are hypoxic in the dark. These roots are photosynthetically active and produce oxygen when exposed to light, thus mitigating root hypoxia.
Keywords: epiphytes, hypoxic niches, orchid, oxygen, Phalaenopsis, photosynthesis, root.
Introduction
Orchids (Phalaenopsis spp.) are a diverse and popular flowering plants belonging to the Orchidaceae family, which is one of the largest and most varied plant families (Dressler 1993). The diversity of orchids is large, with approximately 25,000–30,000 recognised species and thousands of hybrids. This diversity extends to their ecological adaptations, with some orchids being epiphytic (growing on other plants or structures), while others are lithophytic (growing on rocks) and terrestrial (growing in soil).
Epiphytism in orchids refers to a specialised adaptation where these plants grow on the surfaces of other plants or structures, such as trees, rocks, or even telephone wires, without relying on direct contact with soil for their nutrients and support (Rasmussen and Rasmussen 2018). Epiphytic orchids display a wide range of adaptations due to the different environment they inhabit, especially with regard to intermittent availability of light, nutrients, and water (Benzing et al. 1983). The existence of a peculiar root system allowing strong anchorage to tree branches is one of these adaptive anatomical modifications allowing epiphytic orchids to thrive in tropical and subtropical forests. The roots of epiphytic orchids are totally devoid of root hairs and are covered by the velamen, a specialised tissue in the outer layer of the orchid root, having cell walls with patterned, secondary cell wall thickenings (Idris et al. 2021). The velamen are usually white or grey when dry (however, they appear green when wet because of the appearance of underlying photosynthetic cells). They cover the aerial roots and consists of many cell layers thick, and are capable of absorbing atmospheric moisture and nutrients roots (Porembski and Barthlott 1988). Epiphytic orchid roots absorb nutrients dissolved in rainwater, from air humidity, and organic materials surrounding the roots themselves (Zotz and Winkler 2013). The roots of epiphytic orchids can vary widely in shape and structure depending on the species and environment. Some species develop long, thin aerial roots that hang from the host tree, while others have shorter, fleshy roots that also serve as water reservoirs.
Photosynthesis is a vital process in plants, allowing them to convert light energy into chemical energy stored in energy and reducing power (ATP and NADPH). This process typically occurs in chloroplasts within the leaves. While photosynthesis in roots is rare, there is some evidence suggesting that certain orchid species might have photosynthetic activity in their roots. Some orchids indeed possess chloroplasts in their roots, potentially enabling limited photosynthetic activity (GOH et al. 1983; Aschan and Pfanz 2003; Martin et al. 2010; Sma-Air and Ritchie 2020).
Despite these findings, it is important to note that the extent of photosynthesis in orchid roots is likely minimal compared to leaves. Orchids primarily rely on photosynthesis occurring in their leaves to meet their energy needs. Orchids also form symbiotic relationships with mycorrhizal fungi, which provide them with nutrients in exchange with compounds derived from the plant’s photosynthetic activity. However, it was recently discovered that the leafless epiphytic orchid, Taeniophyllum aphyllum, can photosynthesise in its roots (Suetsugu et al. 2023). Both the green roots of this peculiar leafless orchid and the leaves of a closely-related leafy orchid species (Thrixspermum japonicum) show a considerable amount of photosynthetic pigments and as well as photosynthetic activity, indicating that some orchid roots photosynthesise in a similar manner to leaves (Suetsugu et al. 2023).
The significance of root photosynthesis in the overall energy budget of orchids is still a subject of research and debate. While some evidence suggests that orchid roots might exhibit limited photosynthetic activity, the primary site of photosynthesis in orchids remains their leaves. Many epiphytic orchids utilise the crassulacean acid metabolism (CAM) photosynthetic pathway (Silvera et al. 2009). However, this does not imply that the roots of orchids also utilise the same CAM metabolism as their leaves (Benzing and Ott 1981; Goh et al. 1983; Martin et al. 2010). Interestingly, CO2 uptake during the night occurs only in the roots of one species (Phalaenopsis taenialis) of nine orchid species with CAM leaves studied (Benzing and Ott 1981). T. japonicum operates CAM photosynthesis in leaves and C3 photosynthesis in roots, while the leafless T. aphyllum carries out CAM photosynthesis in roots (Suetsugu et al. 2023).
The roots of epiphytic orchids mostly conduct internal refixation of respiratory CO2 (Aschan and Pfanz 2003). However, in addition to refixing respiratory CO2, leafless epiphytic orchid roots undertake autotrophic functions more efficiently than those of leafy epiphytic orchids to ensure an effective autotrophic C gain (Suetsugu et al. 2023).
Indeed, the large diameter of most epiphytic orchid roots suggests that oxygen diffusion in these roots can be limited. To the best of our knowledge, the occurrence of hypoxia in orchid root is yet to be studied. Besides contributing to the overall carbon metabolism of orchid plants, oxygen produced through root photosynthesis may also be important by reducing potential root hypoxia, since water-saturated velamen severely constrains the gas exchange with radicular cortex (Moreira et al. 2009). Here, we hypothesised that oxygen produced by photosynthesis in orchid roots may alleviate the occurrence of root hypoxia. We demonstrate that the roots of a widely commercially cultivated orchid belonging to the genus Phalaenopsis are indeed hypoxic in the dark but hypoxia is mitigated by the photosynthesis occurring in the roots exposed to light.
Material and methods
Plant materials
Adult, flowering stage, hybrids of the Phalaenopsis genus (Phalaenopsis Ravello) were used for all experiments. Plants were all clones obtained from in vitro propagation, and grown in transparent plastic pots filled with orchid-grade bark substrate. The plants were kept in a growth chamber with a 12 h light/12 h dark photoperiod (120 μmol photons m−2 s−1) at 22°C day/18°C night. Irrigations were carried out once a week with distilled water.
Histochemical analysis
Histochemical analysis was performed using 1-cm sections of roots that were cut from the root body and immobilised in 7% agarose blocks. Transverse 200 μM sections were then obtained using a TPI Vibratome Series 1000 Classic. Plant material was dehydrated by washing in growing concentration of ethanol (30/50/75%) for 5 min each and stained with 0.02% toluidine blue. Root slices were then rinsed with distilled water to remove the excess staining solution. Images were captured using the Leica THUNDER Imager Model Organism stereo microscope.
Chlorophyll and carotenoid quantification
Total content of chlorophylls and carotenoids was determined spectrophotometrically and expressed on a fresh weight (FW) basis. Plant material includes leaves and different parts of the root. Between 10 and 20 mg of tissue were extracted with 100% methanol with a 1:100 (w/v) ratio overnight at 4°C in the dark, under continuous agitation. The extracts were centrifuged to remove debris and subsequently measured at 665.2 nm, 654.6 nm (chlorophylls) and 470 nm (carotenoids). Pigments content was calculated according to Lichtenthaler (1987), and normalised against the FW.
Total RNA extraction and qPCR
Total RNA was extracted from orchid tissues (100 mg) using the TRI Reagent (Sigma-Aldrich, St. Louis, MO, USA) according to the manufacturer′s instruction. To ensure the best quality of the RNA, one more step with chloroform was added. Electrophoresis using a 1% agarose gel was performed for all RNA samples to check for RNA integrity, followed by spectrophotometric quantification. cDNA was synthesised from 1 μg of total RNA using the Maxima Reverse Transcriptase kit (Life Technologies). Quantitative PCR (qPCR) amplification was performed on 30 ng of cDNA with a CFX384 TouchTM Real-Time PCR Detection System (Bio-Rad Laboratories), using the PowerUp SYBRGreen Master Mix (Applied Biosystems). ACT4 was used as the housekeeping gene. Relative expression levels were calculated using GeNorm (https://genorm.cmgg.be/). For a full list of the primers used for qPCR, see Supplementary Table S1.
Oxygen measurement
Oxygen concentration was measured according to Valeri et al. (2021), using a FireStingO2 high-precision, PC-controlled fibre-optic oxygen meter (Pyro Science). The needle-type oxygen probe used in the experiments is the OXR50-OI. A MM33 micromanipulator (Unisense) was used to precisely insert the sensor into the root tissue. The oxygen measurements were rapidly performed on roots that were sectioned transversally to allow measurement in the outer and inner parts of the root. Roots were kept for the time indicated in Figures together with 3-(3,4-ddichlorophenyl)-1,1-dimethylurea (DCMU, Sigma-Aldrich), and then cut to expose the surface into which the needle-type oxygen probe was inserted. Although diffusion of oxygen may occur through the surface, the differences detected between the outer/inner parts of the root tissue as well as the impact of light and DCMU on the actual oxygen levels indicate that the diffusion of oxygen during the measurement is negligible. Air temperature was measured simultaneously using the TDIP15 temperature probe. The sensor was calibrated using a 0.1 M sodium ascorbate solution (Sigma-Aldrich). As physical support for the roots during the measurements, 5% agarose plate were used.
Oxygen-modified atmosphere treatments
Hypoxic treatment was performed using an enclosed anaerobic workstation (O2 Control InVitro Glove Box, Coy Lab Products) flushing an oxygen-modified atmospheres [1% (v/v) O2/N2] for 4 h.
Chemical treatment
DCMU (Sigma-Aldrich) was applied to chemically inhibit PSII at the concentration of 100 μM. Roots were cut approximately 5 cm from the root tip and completely submerged in a 100 μM DCMU solution for 5 min. Control roots were immersed in an aqueous solution containing an equal volume of ethanol, the solvent used to dissolve the DCMU, for the same amount of time. The final ethanol concentration was 1%. Subsequently, all samples were transferred to Petri dishes for 1–5 h. Inside the Petri dishes, roots were placed on filter paper imbibed with the respective solution and the plates were placed under a light of approximately 60 μmol of photon m−2 s−1. For the control treatment, plates were kept in both the light and the dark.
Chlorophyll (chl) a fluorescence imaging
Chl a fluorescence parameters were recorded on cut root tips using an IMAGING PAM Chlorophyll Fluorometer (Walz, Effeltrich, Germany), which displayed images of the fluorescence parameters by means of a false colour code ranging from black to purple and representing values from 0.0 to 1.0, respectively. Details of chl fluorescence imaging capture are reported by Guidi et al. (2007). The current fluorescence yield (Ft) was continuously measured, and the F0 images were recorded in a quasi-dark state. Maximum fluorescence yield Fm was then measured after a saturating pulse of 5000 μmol m−2 s−1 PPFD for 1–2 s. Maximum quantum efficiency of PSII photochemistry (Fv/Fm) image was then obtained as:
Under actinic illumination (200 μmol m−2 s−1) were determined both Ft and the maximum light-adapted fluorescence (), which allowed the evaluation of effective PSII quantum yield (ΦPSII) (Genty et al. 1989) as:
The quantum yield of regulated (ΦNPQ) and non-regulated (ΦNO) energy dissipation in PSII were calculated according to Kramer et al. (2004) as:
where NPQ is a parameter of non-photochemical quenching, and qL a measure of the fraction of open PSII centres based on the ‘Lake model’.
Lastly, the apparent electron transport rate (ETR) was calculated as: ETR = ΦPSII × PAR × 0.5 × Absorptivity.
As F0 determination requires the application of far-red light, which could disturb the fluorescence imaging, , was calculated using the Oxborough and Baker (1997) formula:
Results
In the wild, Phalaenopsis orchids derive moisture and nutrients from the air, rain, and debris that accumulates around them (Fig. 1a). Phalaenopsis are popular and widely cultivated as houseplants (Griesbach 2002). They are typically potted in a well-draining mix that includes bark, sphagnum moss, and other materials (Hwang and Jeong 2007). Clear transparent pots are a popular choice for growing Phalaenopsis orchids (Magar et al. 2020). These pots allow better observation of the orchid’s root system, and the transparency also enables light to reach the roots, which can be beneficial for epiphytic orchids like Phalaenopsis that receive light in their natural habitats (Fig. 1b). Phalaenopsis roots avoid darkness and tend to grow outside the opaque pot, which is an undesirable trait for ornamental pot plants (Blanchard and Runkle 2008). The use of transparent pots ensures that growing roots can be contained inside the pot (De et al. 2013).
(a) Epiphytic Phalaenopsis growing on a wood raft. (b) Phalaenopsis root system growing in a transparent plastic pot. (c) A Phalaenopsis root growing on the surface of the substrate. (d) Root section at the microscope in bright field (scale bar, 1 mm). (e, f) Root section stained with toluidine blue and observed in bright field (scale bar, 1 mm). Orange arrows point at putative air pores. Scale bars are (e) 1 mm, and (f) 0.5 mm. (g, h) Total chlorophyll (g) and carotenoids (h) content. Pigments content is expressed as a function of the fresh weight of the sample. Different letters indicate significant differences (P < 0.05) after Kruskal–Wallis test followed by Dunn’s post hoc test. At least 10 biological replicates were used for the analysis.
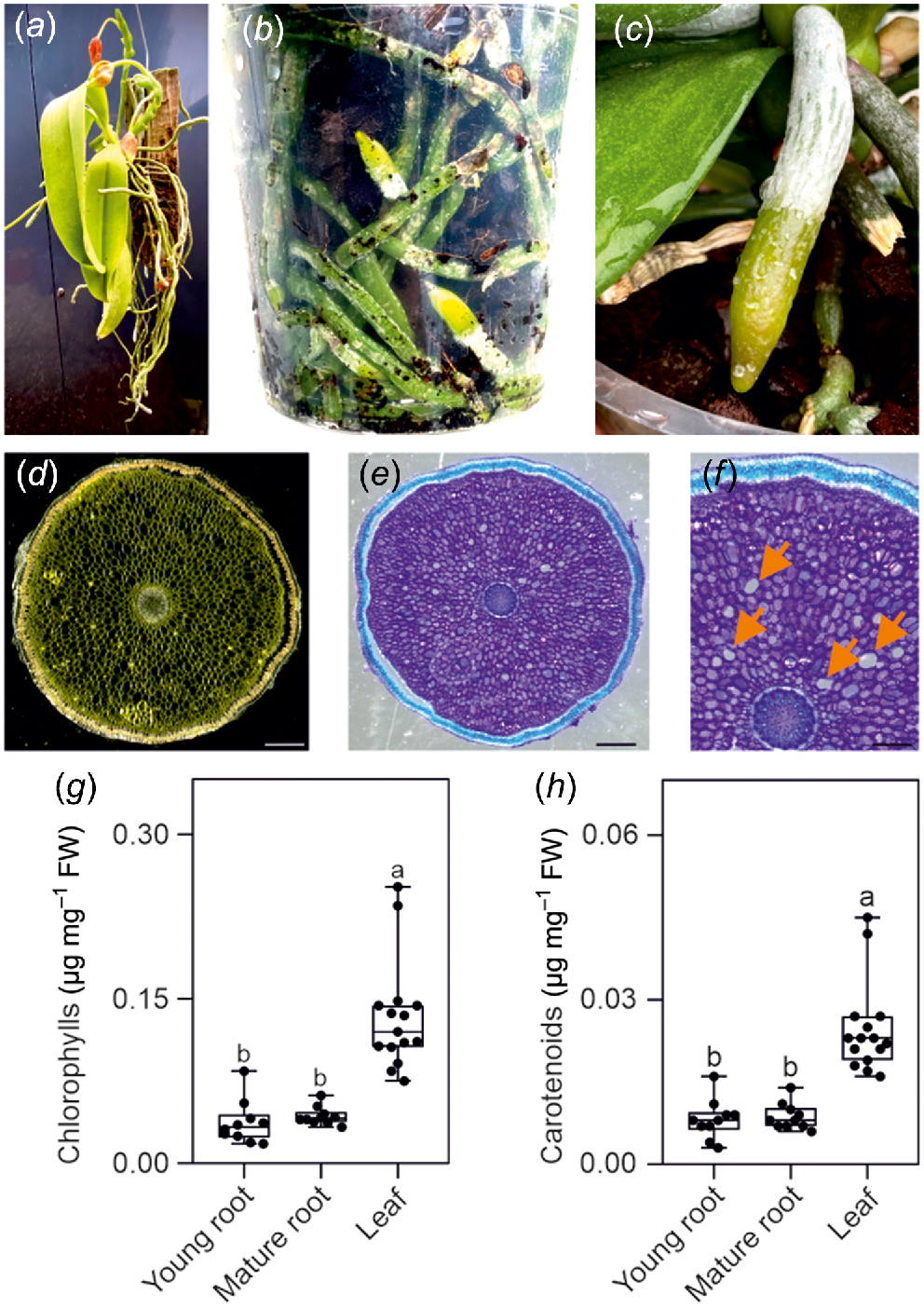
Phalaenopsis orchid roots are thick and covered by a specialised tissue called velamen. Velamen protects the root against UV damage (Chomicki et al. 2015) and acts like a sponge, absorbing water, and nutrients from the environment (Fig. 1c). The diameter of Phalaenopsis roots can exceed 5 mm and this suggests that they may possess histological structures such as aerenchyma, allowing the aeration of the root cells. However, sectioning the root reveals a compact parenchymatic tissue surrounded by the velamen (Fig. 1d, e), with only a few air pores but not a proper, well developed aerenchyma (Fig. 1f). The roots displayed an obvious green colour (Fig. 1b, c) and analysis for chlorophyll and carotenoid content confirmed their presence in roots, although at a lower level compered to leaves (Fig. 1g, h).
We investigated the possibility that Phalaenopsis roots were hypoxic by analysing the expression of a set of genes that is well known to be induced by low oxygen conditions (ALCOHOL DEHYDROGENASE 1, ADH1; PIRUVATE DECARBOXYLASE 2, PDC2; PLANT CYSTEINE OXIDASE 2, PCO2; and ALANINE AMINOTRANSFERASE 2, AlaAT2). All four hypoxia responsive genes (HRGs) were expressed in Phalaenopsis roots, with the highest level of expression detected in the mature root section, already covered by the velamen (Fig. 2). Using a transversal root section, an analysis of oxygen content in this region of the root showed that the green parenchymatic tissue is aerobic, while the root’s inner part (stele), delimited by the pericycle and the vascular bundle, is hypoxic (Fig. 3). The phloem is often hypoxic (van Dongen et al. 2003) and given that all these analysis were performed in the light, the hypoxic expression of the genes reported in Fig. 2 is likely associated to the phloematic tissue.
Real time RT-qPCR analysis in orchid roots. The inset shows a representative root section utilised for the analysis. Relative transcript level of ADH1, PCO2, PDC2 and AlaAT2 in different root sections. Different letters indicate significant differences (P < 0.05) after one-way ANOVA followed by Tukey’s post hoc test. Lines in the boxes indicate the median, the bottom and top of each box denote the first and third quartile, respectively, the dots represent the single data points and whiskers denote the min/max values. Six biological replicates were used for the analysis. The relative expression level is calculated with the root apex data set to one. Data are mean ± s.d. (n = 6).
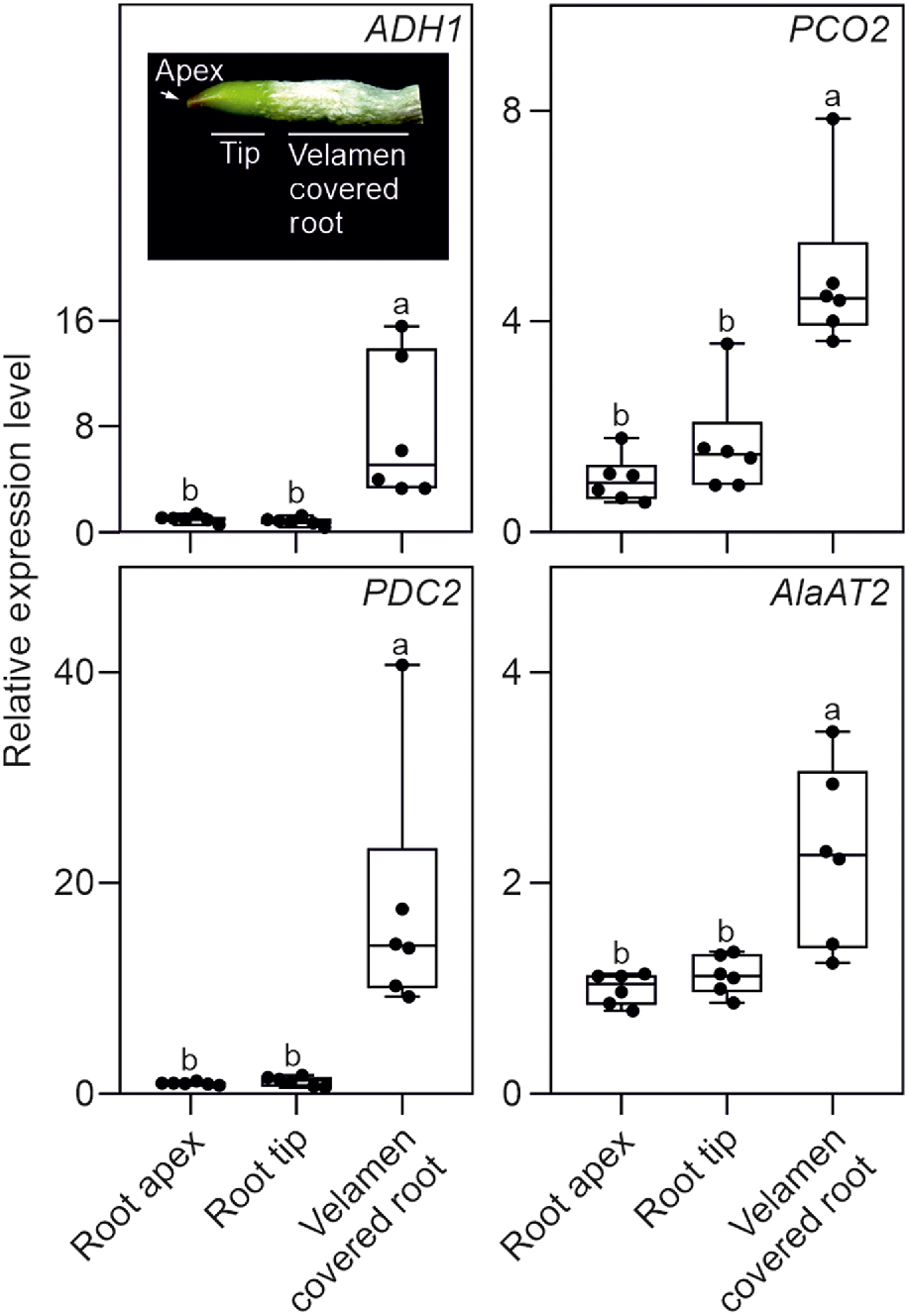
Oxygen tension in the root. (a) Image showing the probe inserted in the root section. (b) Representation of the regions where the probe was inserted in the root section (same image used for Fig. 1d). CC, central cylinder; CP, cortical parenchyma. (c) Oxygen tension measured using an oxygen microsensor probe (immediately after cutting the tissue). Statistically significant differences are indicated by asterisks (Student t-test, unpaired comparison, *P < 0.05; **P < 0.01; ***P < 0.001; ****P < 0.0001). Data are mean of three biological replicates (n = 3) ± s.d.
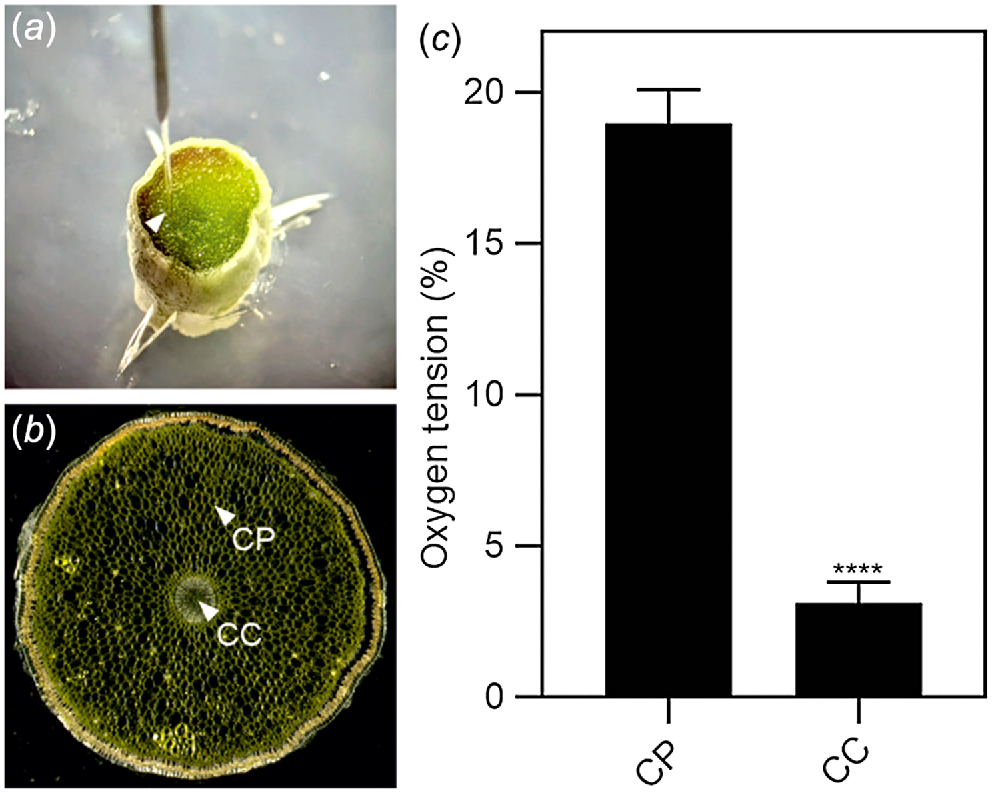
We next defined an experimental design aimed to ascertain the contribution of root photosynthesis in preventing root hypoxia in the parenchymatic tissue and in the ring of vascular bundles in the stele. Chl a fluorescence was monitored to determine the maximum PSII efficiency (Fv/Fm, which represents an indicator of the maximum photochemical efficiency of PSII (Adams et al. 1990), the proportion of absorbed light that is utilised for photosynthetic electron transport (quantum yield of PSII; ΦPSII), and the non-photochemical quenching (qNP) coefficient, which estimates the amount of energy non-photochemically principally dissipated as heat. In Phalaenopsis roots, the average Fv/Fm value was 0.62 for light-exposed control plants (Fig. 4a, b). The very low value of Fv/Fm (0.17; 1–5 h average, Table 1) in roots treated with DCMU was due to the very high value of minimal fluorescence F0 (Fig. 4b). Values of Fv/Fm, as well as those of F0, were not influenced by the dark condition.
Analysis of chlorophyll fluorescence parameters after light/dark exposure and DCMU treatment. (a) Representative chlorophyll fluorescence images of maximum photochemical efficiency of PSII (Fv/Fm) in roots of Phalaenopsis after 1, 3 or 5 h of light exposure, dark condition, or DMCU treatment. Images were reported in a scale of false colours ranging from black to purple and representing values from 0.0 to 1.0, respectively. (b) Values of minimum (F0) and maximum (Fm) chl α fluorescence yield in the dark-adapted state, maximum photochemical efficiency of PSII (Fv/Fm) and electron transport rate (ETR) in roots of Phalaenopsis after 1, 3 or 5 h of light exposure (yellow symbols), dark condition (black symbols), or DMCU treatment (red symbols). Statistically significant differences are indicated by asterisks (*P < 0.05; **P < 0.01; ***P < 0.001; ****P < 0.0001) after two-way ANOVA followed by Dunnett’s post hoc test. Comparisons were carried out within each timepoint using ‘light’ samples as control. Three different roots were used for each treatment/timepoint in the analysis. (c) Values of effective quantum yield of PSII photochemistry (ΦPSII; black bars), quantum yield of light-dependent non-photochemical quenching (ΦNPQ; grey bars), and quantum yield of non-regulated nonphotochemical energy loss in PSII (ΦNO; white bars) in roots of Phalaenopsis after 1, 3 or 5 h of light exposure, dark condition, or DMCU treatment.
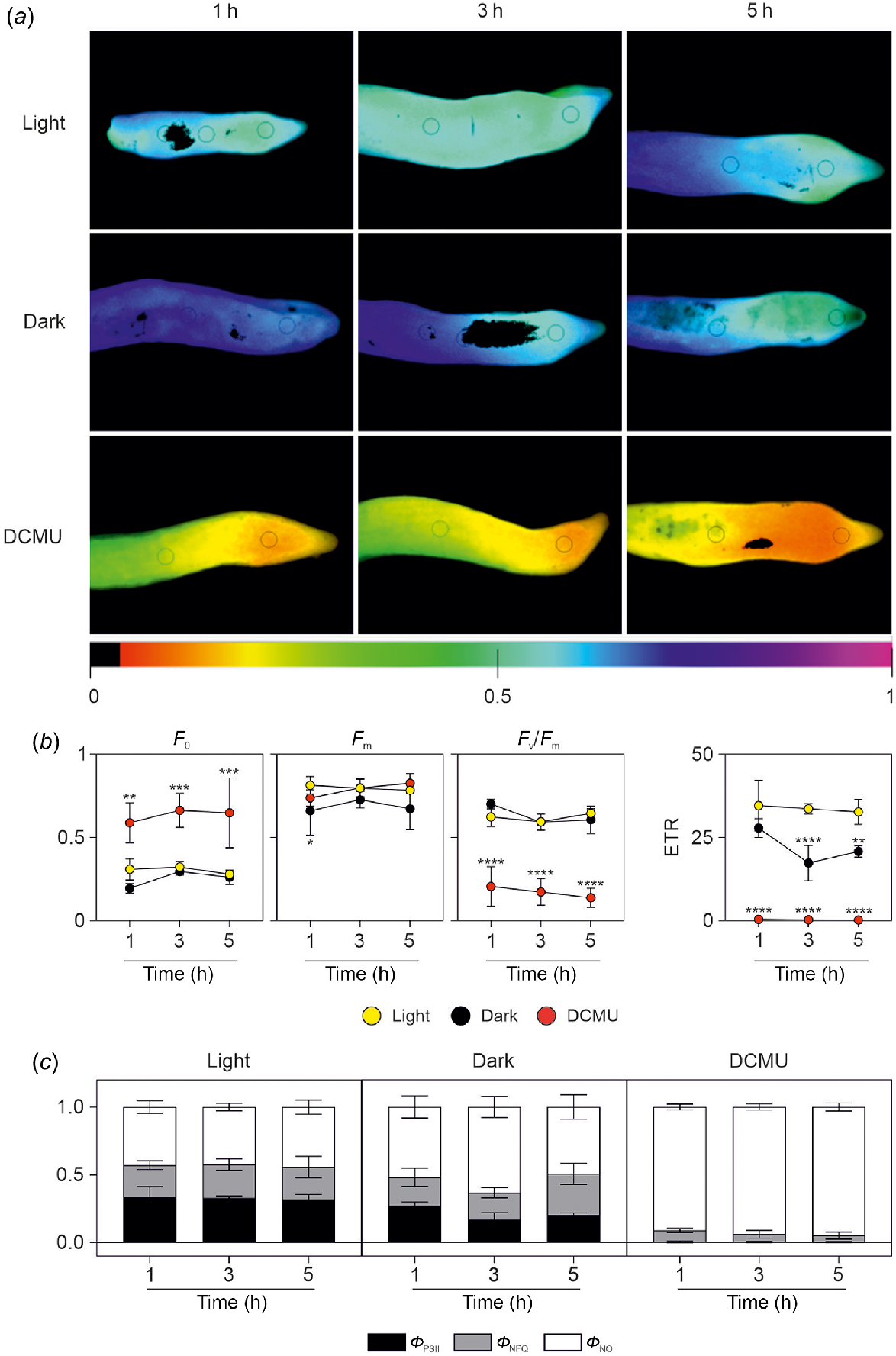
1 h | 3 h | 5 h | ||||||||
---|---|---|---|---|---|---|---|---|---|---|
Light | Dark | DCMU | Light | Dark | DCMU | Light | Dark | DCMU | ||
ΦPSII | 0.34 ± 0.07a | 0.27 ± 0.03a | 0.00 ± 0.01b | 0.33 ± 0.02a | 0.17 ± 0.05b | 0.00 ± 0.01c | 0.32 ± 0.04a | 0.20 ± 0.02b | 0.00 ± 0.00c | |
ΦNPQ | 0.23 ± 0.03a | 0.21 ± 0.07a | 0.09 ± 0.02b | 0.25 ± 0.04a | 0.20 ± 0.04a | 0.06 ± 0.03b | 0.22 ± 0.11a | 0.30 ± 0.08a | 0.05 ± 0.03b | |
ΦNO | 0.43 ± 0.05b | 0.52 ± 0.08b | 0.91 ± 0.02a | 0.42 ± 0.03c | 0.63 ± 0.08b | 0.94 ± 0.02a | 0.44 ± 0.05b | 0.49 ± 0.09b | 0.95 ± 0.03a |
Each point is the average of three replicates (±s.d.). Different lowercase letters indicate significant difference (P < 0.05) after one-way ANOVA followed by Tukey’s post hoc test.
Average Fm values (Fig. 4b) after 1 h of treatment was lower in dark conditions compared to light and DCMU treatments. ETR, estimated from chlorophyll fluorescence, is a widely-used indicator of photosynthetic activity and the ETR is the most representative parameter of the efficiency of photochemical conversion (Guo et al. 2020; Zhang et al. 2020) and there is a theoretical value that can be established for the ratio between ETR and net photosynthesis (Perera-Castro and Flexas 2023). The ETR parameter in Phalaenopsis roots was closer to 0 in roots treated with DCMU (Fig. 4b), while in dark conditions, a decrease of ETR was induced after 3 h in the dark. The values of ΦPSII in light conditions were almost erased in DCMU-treated roots, while in dark conditions, ΦPSII values were lower than samples exposed after 3 h of exposure to light (Fig. 4c, Table 1). Values of ΦNPQ were similar in light and dark conditions but lower in DCMU-treated roots (0.23–0.24 vs 0.06, respectively; 1–5 h average; Fig. 4c, Table 1). Values of ΦNO were higher in DCMU-treated samples for which these dissipative mechanisms represent approximately 93% of total absorbed radiation energy (Fig. 4c). Lower values of this parameter were found in light vs dark roots (Fig. 4c, Table 1).
Having ascertained that a treatment with DCMU is very effective in blocking PSII activity, we tested if blocking photosynthesis either by darkness or DCMU treatment resulted in root hypoxia. The results in Fig. 5 demonstrate that even a short exposure of roots to darkness or DCMU (1 h) results in a significant drop in oxygen concentration in the cortical root parenchyma (Fig. 5). Interestingly, after 5 h of either darkness or DCMU treatment, the cortical root parenchyma is anoxic (<1% O2; Fig. 5), while its oxygen concentration is close to 15% if the roots are exposed to light. The root area enclosed by the endodermis is instead always hypoxic, regardless of light availability and/or photosynthetic activity (Fig. 5).
Oxygen tension measured in dark, light and DCMU treatments. Plant material was kept in dark for 1, 3 and 5 h or treated with DCMU and analysed at the same time points. Control roots were kept in the light for the same time points. Statistically significant differences are indicated by asterisks (*P < 0.05; **P < 0.01; ***P < 0.001; ****P < 0.0001) after two-way ANOVA followed by Dunnett’s post hoc test. Comparisons were carried out within each timepoint using ‘Light’ samples as control. Data are mean of three biological replicates (n = 3) ± s.d. for each treatment/timepoint.
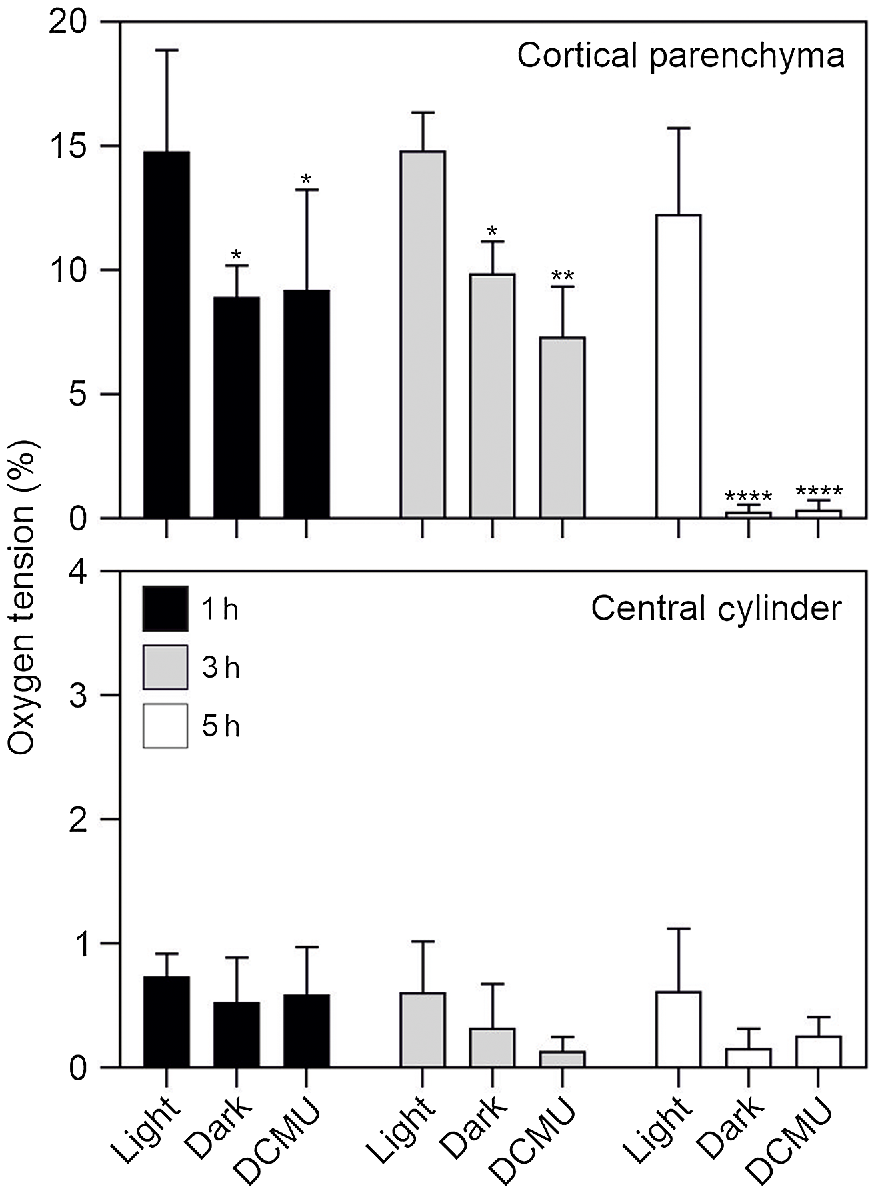
The expression of ADH1, PDC2, PCO2, and AlaAT2 (Fig. 6) is consistent with the oxygen analysis reported in Fig. 5, with all these HRGs expression strongly induced in Phalaenopsis roots (either in the green apex or velamen sections) exposed to either darkness or DCMU. The fact that the HRGs tested are hypoxia responsive was confirmed by exposing Phalaenopsis roots to gaseous hypoxia (Fig. S1).
Expression pattern in orchid root of HRGs typically induced upon hypoxia. Plant material was kept in light or dark for 5 h, or treated with DCMU and sampled after the same amount of time. Lines in the boxes indicate the median, the bottom and top of each box denote the first and third quartile, respectively, the dots represent the single data points and whiskers denote the min/max values. Different letters indicate significant difference (P < 0.05) after one-way ANOVA followed by Tukey’s post hoc test or Kruskal–Wallis followed by Dunn’s post hoc test according to the data distribution. For each analysis the ‘Light’ sample was used as control (value equal to 1). Six biological replicates were used for the analysis for each treatment in the analysis. Data are mean ± s.d. (n = 6).
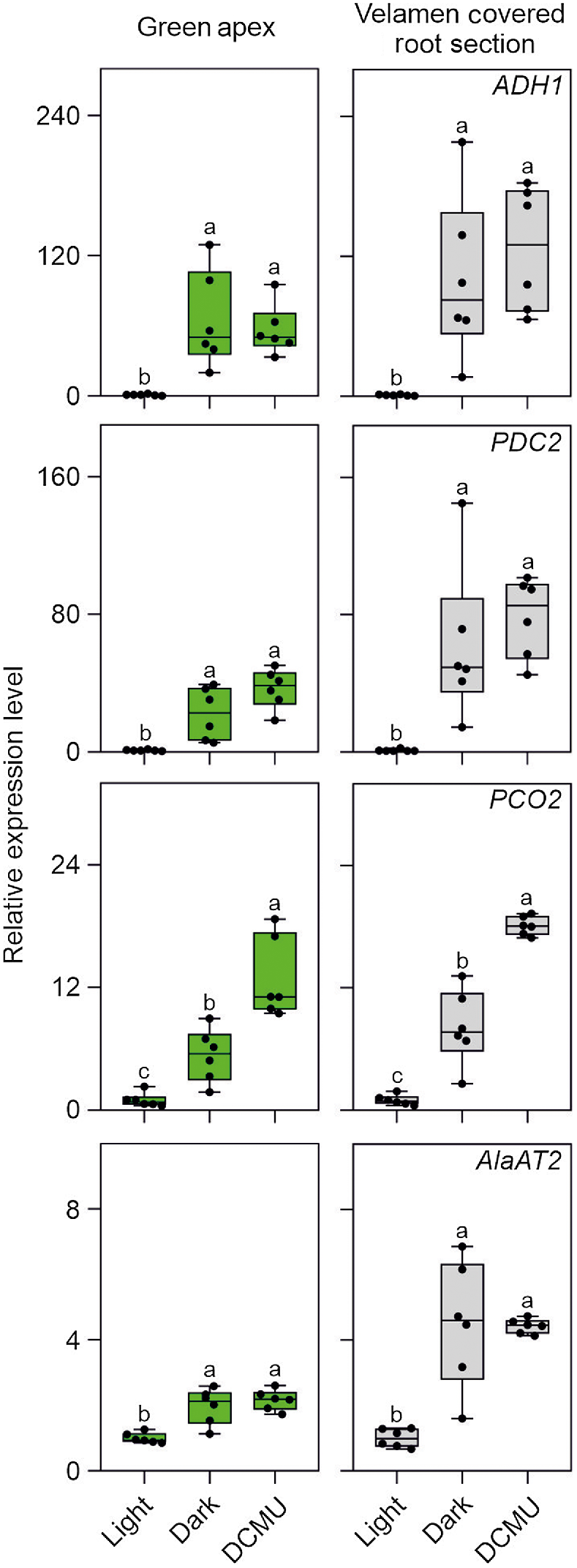
Discussion
Here, we demonstrated that Phalaenopsis roots not only contain photosynthetic pigments but are photosynthetically active. The maximum photochemical efficiency of PSII, represented by the ratio Fv/Fm, showed that Phalaenopsis roots possess a photochemical efficiency lower than leaves given that the Fv/Fm value in Phalaenopsis root had a constant average value of 0.62, which is lower than the range 0.80–0.83 that is typical of healthy leaves (Björkman and Demmig 1987). However, Phalaenopsis root cells can efficiently utilise light energy for photosynthesis as evidenced by results obtained in roots growth in light conditions. In contrast, Phalaenopsis root cells, adapted to dark conditions (from 1 to 5 h) showed a reduction in ETR, an indicator of CO2 photoassimilation (Flexas et al. 1999), but no damage to PSII, as confirmed by the unchanged Fv/Fm ratio, was observed. This is supportive for no permanent damages to PSII reaction centres, which are still able to tunnel absorbed energy to photochemistry (i.e. ΦPSII) and dissipate excessive energy via regulated mechanisms (i.e. ΦNPQ). DCMU strongly decreased the ETR values thereby dramatically affecting the photosynthetic performance of Phalaenopsis roots. In addition, the effect of DCMU resulted in a possible not reversible damage to PSII reaction centres as revealed by the strong decline of Fv/Fm ratio, paralleled by the root inability to dissipate excessive energy via regulated mechanisms (i.e. severe increase in ΦNO values and decline of ΦNPQ).
The role of photosynthesis in the roots of epiphytic orchids has been a matter of debate, mostly on the contribution of root photosynthesis to the overall carbon balance of the plant. There is some consensus that photosynthesis in the roots of epiphytic orchids is mostly aimed to internal refixation of respiratory CO2 (Aschan and Pfanz 2003). However, there can be little doubt that root photosynthesis in leafless orchids is essential to provide photoassimilates to the plant (Suetsugu et al. 2023).
We propose that the role of photosynthesis in the roots of Phalaenopsis is to minimise the occurrence of hypoxia in their bulky aerial roots. Under light conditions, the root parenchyma is photosynthetically active and aerobic, while the vascular bundle is hypoxic, a condition also seen in several plant species (van Dongen et al. 2003). In the wild, Phalaenopsis plants grow on tree branches under shade, and it is important to note that photosynthesis of Phalaenopsis saturates at approximately 130–180 μmol m−2 s−1 (Lin and Hsu 2004). However, the aerobic status of the root parenchyma is rapidly lost under darkness, indicating that Phalaenopsis roots may experience root hypoxia when the roots are not properly exposed to light. This is a condition that may occur when the root is buried in the substrate, either when growing on root branches or most likely in the potting substrate, especially if opaque pots are used. Cultivation of orchids in transparent pots is thus not only effective in preventing roots to grow outside the pot, which are more attractive for ornamental plants, but also allows proper root photosynthetic oxygen production for most of the day. During the night, the roots will be inevitably hypoxic, given that their oxygen content is extremely low after 5 h of darkness (Fig. 5). Hypoxia will be the consequence of the absence of the oxygenic reaction in PSII coupled to oxygen consumption by respiration. The absence of an aerenchyma in the Phalaenopsis roots suggests that oxygen transfer for the aerial part of the plant to the roots is minimal, presumably restricted to the few air pores detected in the root section (Fig. 1). Given the successful induction of HRGs in the hypoxic Phalaenopsis roots (Figs 2 and 6), together with the fact that hypoxia occurs transiently during the night (or in absence of light), it is unlikely that root hypoxia exerts detrimental effects on the growth of Phalaenopsis plants. However, given the impact that hypoxia exerts of root architecture in plants, it is tempting to speculate that the occurrence of hypoxia in Phalaenopsis roots may affect their grow and development. Hypoxic niches in the root primordia result in the downregulation of auxin-induced genes and regulates the production of lateral roots in Arabidopsis thaliana (Shukla et al. 2019). Additionally, hypoxia induces Arabidopsis root bending by inducing an asymmetric auxin distribution (Eysholdt-Derzsó and Sauter 2017). Auxin content in the Arabidopsis root apex is also increased under hypoxia (Eysholdt-Derzsó and Sauter 2017). In Catasetum fimbriatum, an epiphytic orchid, light influences auxin distribution in the plant (Oliveira et al. 2019). Darkness-treated roots in C. fimbriatum contain more auxin than light-exposed roots and light conditions changed the auxin transport from the roots to the shoot (Oliveira et al. 2019). The roots of C. fimbriatum naturally produce a nest-like structure, accumulating moisture and organic debris (Benzing 1986). The size of the nest-like structure of the root system in C. fimbriatum and therefore, the amount of moisture and nutrients the roots will collect, depends on the light hitting the roots, which influences the amount of auxin produced (Oliveira et al. 2019). Given that hypoxia influences auxin physiology in roots, it is reasonable to hypothesise that, given that root photosynthesis generates an alternate aerobic/hypoxic root status, this impacts not only on the sugar and energy root metabolism as expected for any plant tissue under hypoxia, but also auxin waves influencing the root architecture of epiphytic orchids, a hypothesis deserving future investigation.
We conclude that photosynthesis in orchid roots plays an important role in preventing hypoxia that could limit root physiological activities. Aerial roots with a wide diameter ensures adequate anchoring of the plant to tree branches. However, this results in poor oxygen diffusion in the roots, which is balanced by internal oxygen production though photosynthesis.
Acknowledgements
The authors thank Giulio Celandroni Orchidee (San Giuliano Terme, Pisa) for providing us with the Phalaenopsis plants.
References
Adams WW, III, Demmig-Adams B, Winter K, Schreiber U (1990) The ratio of variable to maximum chlorophyll fluorescence from photosystem II, measured in leaves at ambient temperature and at 77K, as an indicator of the photon yield of photosynthesis. Planta 180, 166-174.
| Crossref | Google Scholar | PubMed |
Aschan G, Pfanz H (2003) Non-foliar photosynthesis – a strategy of additional carbon acquisition. Flora – Morphology, Distribution, Functional Ecology of Plants 198, 81-97.
| Crossref | Google Scholar |
Benzing DH (1986) The vegetative basis of vascular epiphytism. Selbyana 9, 23-43 Available at https://www.jstor.org/stable/41888783.
| Google Scholar |
Benzing DH, Ott DW (1981) Vegetative reduction in epiphytic Bromeliaceae and Orchidaceae: its origin and significance. Biotropica 13, 131.
| Crossref | Google Scholar |
Benzing DH, Friedman WE, Peterson G, Renfrow A (1983) Shootlessness, velamentous roots, and the pre-eminence of Orchidaceae in the epiphytic biotope. American Journal of Botany 70, 121-133.
| Crossref | Google Scholar | PubMed |
Björkman O, Demmig B (1987) Photon yield of O2 evolution and chlorophyll fluorescence characteristics at 77 K among vascular plants of diverse origins. Planta 170, 489-504.
| Crossref | Google Scholar | PubMed |
Blanchard MG, Runkle ES (2008) Container opacity and media components influence rooting of potted Phalaenopsis and Doritaenopsis orchids. In ‘ISHS Acta Horticulturae’. pp. 115–120. (International Society for Horticultural Science) doi:10.17660/ActaHortic.2008.788.13
Chomicki G, Bidel LPR, Ming F, Coiro M, Zhang X, Wang Y, Baissac Y, Jay-Allemand C, Renner SS (2015) The velamen protects photosynthetic orchid roots against UV-B damage, and a large dated phylogeny implies multiple gains and losses of this function during the Cenozoic. New Phytologist 205, 1330-1341.
| Crossref | Google Scholar | PubMed |
De LC, Barman D, Medhi RP, Chhetri G, Pokhrel H (2013) Production technology of Phalaenopsis. Technical Bulletin No. 15. (National Research Centre for Orchids Bullettin: Sikkim, India) Available at https://doi.org/10.13140/RG.2.2.28080.30726
Eysholdt-Derzsó E, Sauter M (2017) Root bending is antagonistically affected by hypoxia and ERF-mediated transcription via auxin signaling. Plant Physiology 175, 412-423.
| Crossref | Google Scholar | PubMed |
Flexas J, Escalona JM, Medrano H (1999) Water stress induces different levels of photosynthesis and electron transport rate regulation in grapevines. Plant, Cell & Environment 22, 39-48.
| Crossref | Google Scholar |
Genty B, Briantais J-M, Baker NR (1989) The relationship between the quantum yield of photosynthetic electron transport and quenching of chlorophyll fluorescence. Biochimica et Biophysica Acta (BBA) – General Subjects 990, 87-92.
| Crossref | Google Scholar |
Goh CJ, Arditti J, Avadhani PN (1983) Carbon fixation in orchid aerial roots. New Phytologist 95, 367-374.
| Crossref | Google Scholar |
Guidi L, Mori S, Degl’Innocenti E, Pecchia S (2007) Effects of ozone exposure or fungal pathogen on white lupin leaves as determined by imaging of chlorophyll a fluorescence. Plant Physiology and Biochemistry 45, 851-857.
| Crossref | Google Scholar | PubMed |
Guo Y, Lu Y, Goltsev V, Strasser RJ, Kalaji HM, Wang H, Wang X, Chen S, Qiang S (2020) Comparative effect of tenuazonic acid, diuron, bentazone, dibromothymoquinone and methyl viologen on the kinetics of Chl a fluorescence rise OJIP and the MR820 signal. Plant Physiology and Biochemistry 156, 39-48.
| Crossref | Google Scholar | PubMed |
Hwang SJ, Jeong BR (2007) Growth of Phalaenopsis plants in five different potting media. Journal of the Japanese Society for Horticultural Science 76, 319-326.
| Crossref | Google Scholar |
Idris NA, Aleamotuʻa M, McCurdy DW, Collings DA (2021) The orchid velamen: a model system for studying patterned secondary cell wall development? Plants 10, 1358.
| Crossref | Google Scholar |
Kramer DM, Johnson G, Kiirats O, Edwards GE (2004) New fluorescence parameters for the determination of QA redox state and excitation energy fluxes. Photosynthesis Research 79, 209-218.
| Crossref | Google Scholar | PubMed |
Lichtenthaler HK (1987) [34] Chlorophylls and carotenoids: pigments of photosynthetic biomembranes. Methods in Enzymology 148, 350-382.
| Crossref | Google Scholar |
Lin M-J, Hsu B-D (2004) Photosynthetic plasticity of Phalaenopsis in response to different light environments. Journal of Plant Physiology 161, 1259-1268.
| Crossref | Google Scholar | PubMed |
Magar YG, Koshioka S, Noguchi A, Amaki W (2020) Effects of light quality during cultivation on the flowering and floret arrangement in Phalaenopsis amabilis. Acta Horticolturae 1271, 135-140.
| Crossref | Google Scholar |
Martin CE, Mas EJ, Lu C, Ong BL (2010) The photosynthetic pathway of the roots of twelve epiphytic orchids with CAM leaves. Photosynthetica 48, 42-50.
| Crossref | Google Scholar |
Moreira ASFP, de Lemos Filho JP, Zotz G, dos Santos Isaias RM (2009) Anatomy and photosynthetic parameters of roots and leaves of two shade-adapted orchids, Dichaea cogniauxiana Shltr. and Epidendrum secundum Jacq. Flora: Morphology, Distribution, Functional Ecology of Plants 204, 604-611.
| Crossref | Google Scholar |
Oliveira PMR, Rodrigues MA, Gonçalves AZ, Kerbauy GB (2019) Exposure of Catasetum fimbriatum aerial roots to light coordinates carbon partitioning between source and sink organs in an auxin dependent manner. Plant Physiology and Biochemistry 135, 341-347.
| Crossref | Google Scholar | PubMed |
Oxborough K, Baker NR (1997) Resolving chlorophyll a fluorescence images of photosynthetic efficiency into photochemical and non-photochemical components – calculation of qP and Fv′/Fm′ without measuring Fo′. Photosynthesis Research 54, 135-142.
| Crossref | Google Scholar |
Perera-Castro AV, Flexas J (2023) The ratio of electron transport to assimilation (ETR/AN): underutilized but essential for assessing both equipment’s proper performance and plant status. Planta 257, 29.
| Crossref | Google Scholar | PubMed |
Porembski S, Barthlott W (1988) Velamen radicum micromorphology and classification of Orchidaceae. Nordic Journal of Botany 8, 117-137.
| Crossref | Google Scholar |
Rasmussen HN, Rasmussen FN (2018) The epiphytic habitat on a living host: reflections on the orchid–tree relationship. Botanical Journal of the Linnean Society 186, 456-472.
| Crossref | Google Scholar |
Shukla V, Lombardi L, Iacopino S, Pencik A, Novak O, Perata P, Giuntoli B, Licausi F (2019) Endogenous hypoxia in lateral root primordia controls root architecture by antagonizing auxin signaling in Arabidopsis. Molecular Plant 12, 538-551.
| Crossref | Google Scholar | PubMed |
Silvera K, Santiago LS, Cushman JC, Winter K (2009) Crassulacean acid metabolism and epiphytism linked to adaptive radiations in the Orchidaceae. Plant Physiology 149, 1838-1847.
| Crossref | Google Scholar | PubMed |
Sma-Air S, Ritchie RJ (2020) Photosynthesis in a Vanda sp orchid with photosynthetic roots. Journal of Plant Physiology 251, 153187.
| Crossref | Google Scholar | PubMed |
Suetsugu K, Sugita R, Yoshihara A, Okada H, Akita K, Nagata N, Tanoi K, Kobayashi K (2023) Aerial roots of the leafless epiphytic orchid Taeniophyllum are specialized for performing crassulacean acid metabolism photosynthesis. New Phytologist 238, 932-937.
| Crossref | Google Scholar | PubMed |
Valeri MC, Novi G, Weits DA, Mensuali A, Perata P, Loreti E (2021) Botrytis cinerea induces local hypoxia in Arabidopsis leaves. New Phytologist 229, 173-185.
| Crossref | Google Scholar | PubMed |
van Dongen JT, Schurr U, Pfister M, Geigenberger P (2003) Phloem metabolism and function have to cope with low internal oxygen. Plant Physiology 131, 1529-1543.
| Crossref | Google Scholar | PubMed |
Zhang P, Zhang Z, Li B, Zhang H, Hu J, Zhao J (2020) Photosynthetic rate prediction model of newborn leaves verified by core fluorescence parameters. Scientific Reports 10, 3013.
| Crossref | Google Scholar |
Zotz G, Winkler U (2013) Aerial roots of epiphytic orchids: the velamen radicum and its role in water and nutrient uptake. Oecologia 171, 733-741.
| Crossref | Google Scholar | PubMed |