Shedding light on predator detections: evaluating the impact of camera-trap flash type for feral cat monitoring through in-field observations
Alexandra J. Paton


A
B
Abstract
White flash camera-traps are avoided in predator monitoring as they are assumed to lower redetection, despite infrared cameras producing lower-quality night images thus limiting the scope for individual identification and, consequently, the accuracy of density estimates. We sought to determine whether flash type impacts the behaviour of cats (Felis catus). We identified different behavioural responses exhibited by photographed cats, and quantitatively assessed relative activity, redetection rates, and the activity of specific individuals using 11,389 images of feral cats from 62 white flash and 62 infrared camera-traps across Tasmania. We found no difference in the relative activity of cats between flash types (odds ratio of 0.90, [null expectation = 1], CI = 0.55, 1.47), and there was no evidence of a reduction in redetection rate of feral cats following initial detection (odds ratio = 0.83, CI = 0.47, 1.46). The activity of individuals was similar between white flash (average = 0.026, CI = 0.021, 0.032), and infrared cameras (average = 0.028, CI = 0.022, 0.035). White flash cameras appear suitable for monitoring feral cats without resulting in a negative bias, highlighting the need for researchers to critically examine assumptions regarding best methodology.
Keywords: activity, avoidance, detection, feline, invasive species, methodology, monitor, trail camera.
Introduction
Camera-traps are a popular tool for wildlife monitoring. They can be deployed for long periods without human presence and are considered a low disturbance monitoring method (O’Connell et al. 2011; Meek et al. 2014a; Kays et al. 2020), making them particularly useful for surveying vertebrate predators, many of which are nocturnal, trap-shy, wide ranging, and often found in remote locations (Wegge et al. 2004; Rees et al. 2019). Previously widespread methods used to monitor predators, such as sand plots and spotlight surveys, only provide crude activity indices, are dependent on weather conditions to undertake, are generally more costly and can be difficult to resolve to the species level compared to camera traps (Ballard et al. 2014). Additionally, due to the obscuring vegetation and consequent limited line of sight, spotlight surveys cannot reliably be undertaken to monitor predators in dense, forested environments (Rees et al. 2019). As such, camera-traps have become the method of choice for wildlife managers and researchers monitoring predators.
Despite their rising popularity, assumptions that camera-traps are a truly passive monitoring method have been called into question, as they have been demonstrated to affect behaviour in several ways. For example, many animals can hear the camera-trap trigger (Meek et al. 2014b), and animals may notice the presence of a new object in their region, which often elicits a curiosity response (Cove and Jackson 2011; Meek et al. 2016).
The most common speculation by researchers and wildlife managers is that animal behaviour may be influenced by seeing the white flash or glow of the camera-trap if an image is taken at night (Meek et al. 2014b; Henrich et al. 2020; Ladd et al. 2023). Wildlife managers and researchers can choose between white flash, either xenon bulb or white LED (visible light), infrared (red glow, ~850 nm wavelength) or black (low glow, ~940 nm) flash units to capture night images. Cameras with a white flash are the only type that produce colour images at night, with xenon white flash producing clearer colour images than LEDs (Herrera et al. 2021), which facilitates the identification of species and individuals (when unique coat patterns permit this), allowing for sight–resight density estimates to be calculated (Bengsen et al. 2011; Stokeld et al. 2016; Burns et al. 2018) (Fig. 1). However, it has been hypothesised that the use of white flash cameras might reduce the probability of redetection if animals avoid future encounters with the ‘startling’ white flash (Wegge et al. 2004; Cove and Jackson 2011). A disturbance like this would potentially reduce the number of images captured over time, biasing density estimates downwards and reducing the power of the resultant analysis. By contrast, infrared and black flash cameras produce little to no visible flash (alternative hypothesis: little or no behavioural impact), and as a result many wildlife managers consider them ideal for minimising disturbance, with a practical benefit of lowering the risk of theft. The downside is that the night images produced are black and white and often grainy or blurred due to the longer required exposure times, making individual identification, and sometimes species identification, difficult (Glen et al. 2013; Burns et al. 2018). For individual identification, the use of images taken by infrared units can lead to an inflation of unidentified (or ‘unknown’) individuals within a dataset, and an increased risk of misidentification of individuals (Nelson and Scroggie 2009; Glen et al. 2013; Sparkes et al. 2021). These measurement errors and uncertainties act to reduce the accuracy of subsequent density estimates, and can result in underestimates of real population sizes (Efford and Hunter 2018).
A comparison of infrared images (left) and white flash images (right) of feral cats (Felis catus) captured at the same camera site in Tasmania, Australia. All images show a cat with a broken, brown tabby coat. The top images show the cat far from the camera-trap, whereas the bottom images provide examples of when the cat is closer.
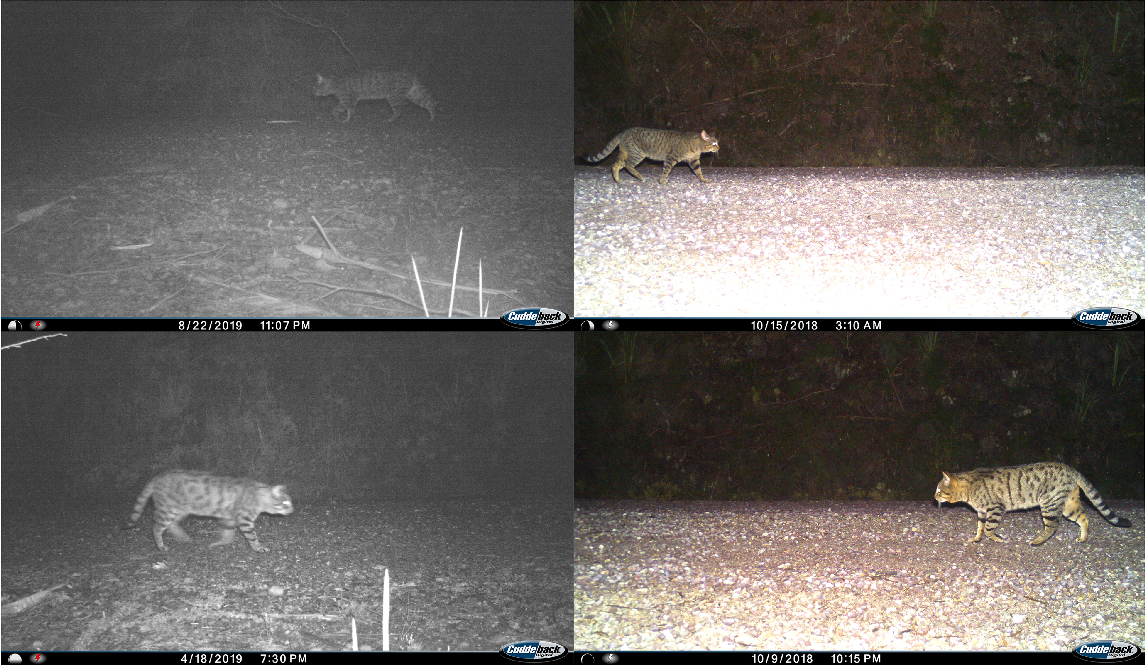
Both flash types seem to present potential problems for data analysis (Glen et al. 2013). It seems clear that infrared images lower the accuracy of individual identification, but what evidence exists to support the claim that white flash units negatively influence predator behaviour? The distinct coat patterns of numerous felid species, coupled with their hypervigilant nature, make them ideal candidates for camera-trap monitoring. However, concerns about the influence of flash-type on behaviour are most pronounced within studies of this taxonomic group. Two different in-field studies, of tigers (Panthera tigris) (Wegge et al. 2004) and of bobcats (Lynx rufus) (Cove and Jackson 2011), both noted a reduction in images captured over time and a difference in behaviour towards the camera flash types. However, neither study aimed to assess the impact of flash type on animal behaviour/redetection rate. Instead, these studies sought to estimate animal occupancy and density, and simply noted declines in detection over time. They did not control or measure additional covariates that might have also impacted redetection rates. Wegge et al. (2004) used only white flash cameras within their study, so no baseline comparison with black or infrared flash cameras was available to quantify any flash-driven differences. Cove and Jackson (2011) did look at alternative flash types, but the sample size was small, with only six bobcat detections over 306 trap-nights. Of these, five were captured on infrared cameras and only a single bobcat observation on the white flash cameras. Sparse data like these are a recurring problem in felid monitoring (Taggart et al. 2020), and longer-term studies with large sample sizes across treatment types are needed to more robustly assess whether these differences in detection probability are real, or just due to chance.
Among the best-studied of the felids are feral cats (Felis catus), an invasive species of management concern within Australia (Doherty et al. 2017). Cats provide an opportunity to better understand the influence of camera-traps on felid behaviour compared to rare species, because they are so widespread and abundant (Legge et al. 2017). They are a current focus for wildlife management throughout Australia, and their behaviour is easy to interpret due to their domesticated origin. Past studies have attempted to explore feral cat response to camera-traps in an experimental setting. One study was done under laboratory conditions, where captured feral cats were placed in an enclosure and exposed to different camera-traps, some using white flash and others using infrared units (Glen et al. 2013). In this study, three of the six cats used exhibited a ‘fright’ response to the white flash camera-traps, and one to an infrared unit. However, these stressful conditions are not necessarily reflective of cat behaviour in the wild, and the study lacked statistical power. Taggart et al. (2020) attempted to quantify the response of feral cats to different flash units under natural field conditions by examining the responses of feral cats to 25 white flash and 30 infrared camera-traps for 24 days, with known wild cat populations. They found no difference in feral cat capture rate for either flash type. However, they were again constrained by low sample sizes, and only captured 84 cat-detection events, with an estimated nine individuals present. Given this limitation, they called for further research to explore this methodological question.
As it stands, there remains no definitive evidence to support the theory that feral cats are afraid of, or avoid, camera-traps in the wild, even if white flash cameras are used. If we had this information, we could evaluate the trade-off between using infrared flashes, where image fidelity is reduced, and white flash cameras, which might result in fewer wildlife images for a given management objective. Given funding constraints for wildlife management and monitoring (Hoffmann and Broadhurst 2016), we need to ensure we have optimised our monitoring methods to achieve conservation goals.
In this study, we aimed to comprehensively investigate the impact of camera-trap flash type on felid behaviour, using feral cats as our study species. We used a sample of 62 white flash and 62 infrared camera-traps from a large camera-trap network for wildlife monitoring in Tasmania, an island state in the south-east of Australia. A subsidiary aim was to quantify responses of feral cats to camera-traps, wherein the behaviours of cats were categorised and analysed. To achieve these aims, we examined the differences in daily capture rates of cats between different flash types and tested for evidence of a development in trap shyness by examining changes in feral cat capture rate with time since camera establishment. We also created a metric for individual return rate and examined how this differed between flash types.
Materials and methods
Field sites and camera-trap network
The data used in this study were derived from a pre-existing network of camera-traps established in 2018 for the purpose of wildlife monitoring across Tasmania, Australia. From this network we used a subset of 124 camera sites: 62 white flash and 62 infrared cameras (Fig. 2). Sites were selected based on the proximity of each white flash camera-trap to the nearest infrared camera-trap, with these camera-traps generally operating concurrently. Each infrared camera was an average of 3.8 km from its nearest white flash camera (s.e. = 392 m, minimum distance of 20 m, max 16 km). Camera-trap pairs were deployed in equivalent habitat and within the distance of a feral cat’s potential home-range (Nottingham et al. 2022).
A map of the north-west, south-west, and south-east of Tasmania, with satellite imagery provided by Google Earth. A scale is provided in red, and each point represents a camera-trap site, where red points are infrared camera-traps and white points are white flash camera-traps.
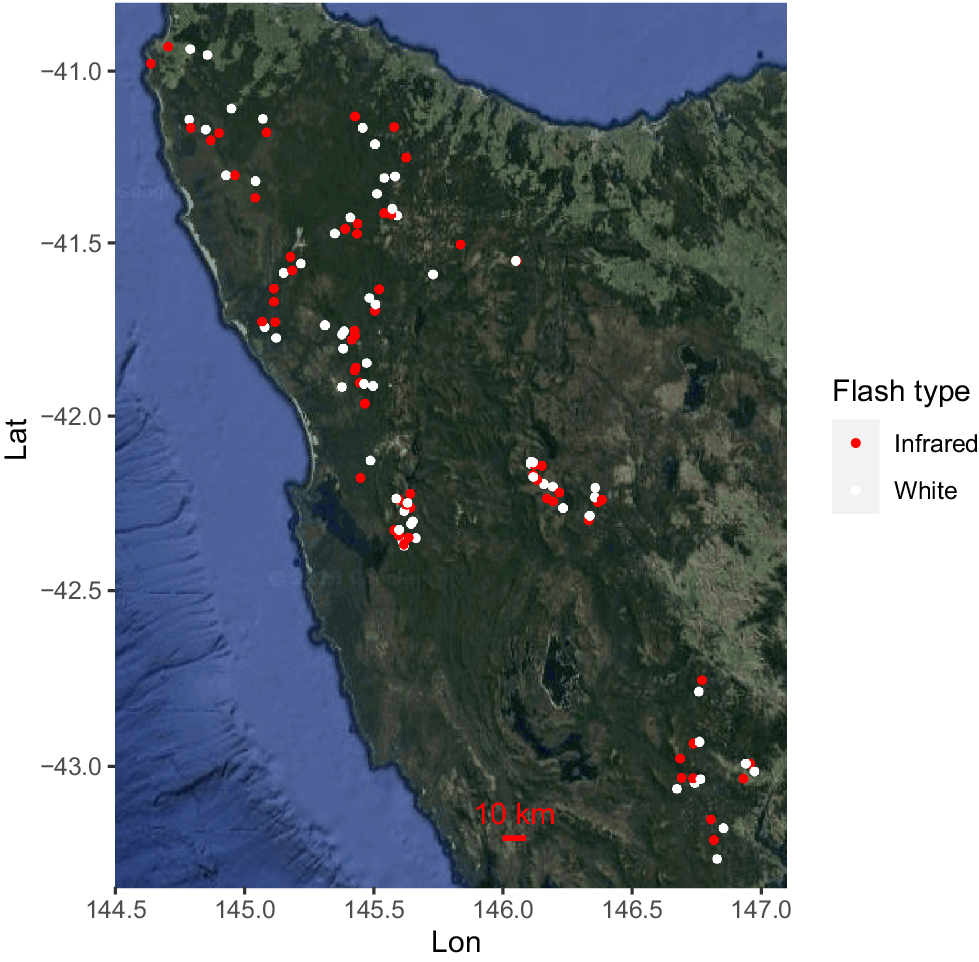
Cuddeback X-Change™ Model 1279 units were programmed to capture one image every 30 s in response to movement and heat during the day or during the night if the camera used an infrared flash. White flash cameras were programmed with a 1-min delay between image captures at night to allow sufficient recovery time for the capacitor to recharge. Feral cats rarely spent more than 30 s at the camera-trap, and if sequential images were captured of a feral cat within 1 min of each other, only one image was used in subsequent analyses.
All camera-traps were positioned on the side of roads and wide man-made trails. They were mounted ~30–50 cm from the ground on tree trunks or fallen logs, pointed across the road at an appropriate angle to maximise the zone of detection. No lures were used on any cameras. Disposable alkaline, and later lithium batteries were used during this monitoring period, with cameras being serviced quarterly for cameras using alkaline batteries, and every 5–7 months for cameras using lithium batteries. Each camera site was monitored continuously for a period of between ~6 months and >3 years, with most paired infrared and white flash cameras operating simultaneously during this time. This research was conducted under scientific license number FA20109 and Animal Research Authority Number A0017229.
Data analysis
All analyses were undertaken in R (R Development Core Team 2010). In total, 11,389 feral cat events were collected from 124 camera-traps. Of these, 7151 were from infrared cameras, with 2114 events captured during the day, and 5037 captured at night. The remaining 4238 events were from white flash cameras, with 1349 captured during the day, and 2889 captured at night. The infrared cameras operated for a total of 31,632 days, and the white flash cameras monitored 21,392 days.
To correct for differences in effort between camera-traps, the total number of days cats were captured walking past a camera-trap was divided by that camera-trap’s total number of operating days. This provides a metric of activity, referred to here as ‘proportion of days with events’ or ‘PDE’, though it has been previously reported as ‘relative activity index’ or ‘RAI’ (O’Brien 2011). To avoid confusion with other activity metrics, such as those that evaluate the duration or times over which an animal is active, we have opted to use the acronym PDE.
We calculated the average PDE across camera-traps using all cat events for night events and for day events, as well as for flash type. These averages and their 97.5% confidence intervals were derived using the bootstrap method (Canty and Ripley 2017).
To examine if there was a difference in cat capture rate between camera-trap flash types, or during the day and night, we used mixed-effects linear modelling (Bates 2016). The PDE calculated from night events and for day events for each camera-trap was logit transformed to normalise the data distribution to meet the assumptions of a linear regression model. This was more appropriate than using a Poisson distribution as camera-traps did not have fixed operating durations. Additionally, this method was more appropriate than a logistic regression analysis based on the presence or absence of cats on a given day as it avoids problem of 0 inflated data from low activity of a far roaming predator. The model included the following predictors: vegetation type (grassland, human/other, rainforest, shrubland, tall eucalypt, and woodland; source: National Vegetation Information System Major Vegetation Groups layer; www.environment.gov.au), elevation (metres above sea level; Department of agricultural resources; www.data.daff.gov.au), fraction of photosynthetically absorbed radiation (FPAR) (Biodiversity and Climate Change Virtual Laboratory; bccvl.org.au, recorded from 2000 to 2014), topographic roughness (index of elevation variability; created by Grant Williamson, University of Tasmania), time (day or night), and flash type. All continuous predictors were scaled prior to analysis. We also included an interaction term between time and flash type to examine the a priori hypothesis that cats will avoid white flash camera-traps at night. Lastly, we included a random effect for camera-trap site, given that we resampled from each camera-trap site twice to obtain a PDE for day events and a PDE for night events. No model selection was undertaken as we were interested in effect size rather than predictive modelling (Yates et al. 2023).
In our investigation, cat behaviour in camera-trap images was categorised as ‘camera focused’ or ‘other focus’ (Fig. 3). ‘Camera focused’ describes instances where the cat is directly facing the camera, often accompanied by resting in front of the camera-trap. All other behaviours fall under ‘other focus’. Images where only part of a cat was visible (e.g. tail tips) or image quality was poor were labelled as ‘unknown’ and excluded from further analysis. Acknowledging that we are using still images with a one-minute delay, as opposed to ‘burst’ captures or video, we recognise the potential for behaviours to emerge after the initial image was captured. We assumed that feral cats would display camera-focused behaviours at the time of capture, and we believe that the potential for camera focused behaviours to be missed is consistent between flash types and time of capture. Thus, to address the hypothesis that infrared cameras are less conspicuous to cats than white flash, we compare the relative rates of camera focused behaviours between flash types and day/night images, avoiding a precise estimate of cats’ overall awareness of camera-traps.
Examples of ‘camera focused’ behaviours are shown on the left, where the cat is staring directly at the camera-trap, often resting in front of the unit. Examples of ‘other focus’ behaviours are shown on the right and include any behaviour where the cat is ignoring or unaware of the camera unit, such as walking, grooming, excreting, interacting with other cats, or carrying prey.
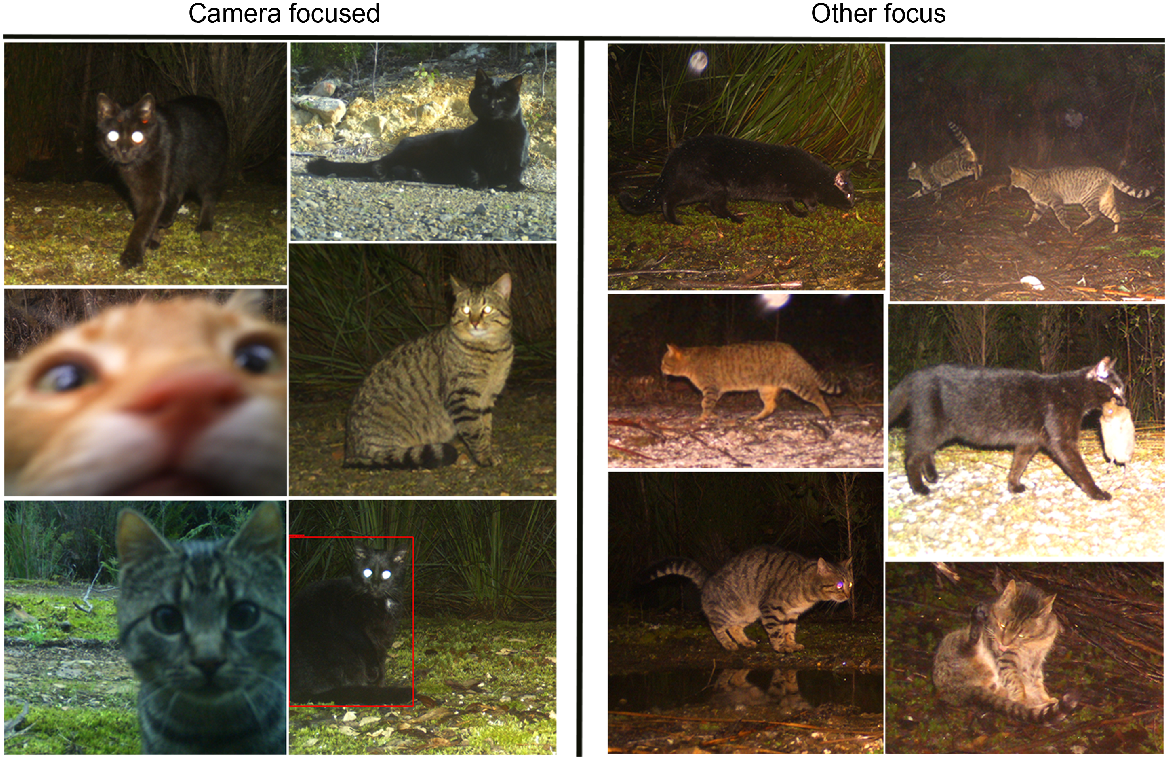
To quantify how flash type and/or time of capture influenced the chance of a cat being captured focusing on a camera-trap, we used a mixed-effects logistic regression model. The model used the same predictors as the capture-rate model and had behaviour (categorised as camera focused or other focus) as the response variable. As with PDE, no model selection was undertaken as we are interested in evaluating effect sizes.
We tested for evidence of trap shyness by following the methods of Wegge et al. (2004). This involved subdividing the data based on the number of trap-nights at each station and investigating the frequency of cat visitations at intervals of 15 days, 15–30 days, and 30–45 days since the first camera deployment at that site. An analysis of variance (ANOVA) was used to assess the difference in mean cat visitations between these periods, with a post hoc examination of the difference in means using a Tukey-test.
We wanted to determine if the same individual cats were returning to the camera-traps regardless of flash type, or whether individuals had a higher turn-over rate for one flash type relative to another, presumably due to avoidance behaviours. To do this, we assigned an individual identity to each cat image using distinct coat markings and colours, excluding black cats or unidentifiable images (e.g. poor quality images, tail tips). We calculated the total number of days an individual was captured at a camera-trap, then divided that by the camera-trap’s total operating days, which provided an individual’s relative activity index (IRAI) for each camera-trap, allowing us to correct for differences in each camera’s operation time (as with PDE). We calculated the average IRAI for white flash and infrared cameras using the bootstrap method with 97.5 confidence intervals (Canty and Ripley 2017).
Results
The proportion of days with events (PDE) for feral cats was similar across white flash and infrared camera-traps, with white flash camera-traps having a slightly lower PDE (Fig. 4). This was true regardless of whether the events were captured at night or during the day (Fig. 4). The average PDE for feral cats across the 124 camera-traps was 0.16 (97.5% confidence interval (CI) = 0.14, 0.19) for all events, including day and night captures. White flash average PDE for all events was 0.14 (CI = 0.11, 0.17), and infrared flash average PDE for all events was 0.18 (CI = 0.15, 0.22).
Box-and-whisker plot showing the fraction of days a camera-trap detected a feral cat (Cat PDE: proportion of days with cat events), for white flash and infrared (IR) camera-traps within Tasmania. All represents PDE calculated using all events, while day PDE was calculated using only events captured during the day, and night using only events captured at night. IR cameras are shown in red, white flash cameras in blue. Horizontal lines show the median activity (50th percentile), the box shows the interquartile range (IQR: 25th and 75th percentiles), the vertical lines [whiskers] represent 1.5 × IQR and the solid dots are outliers.
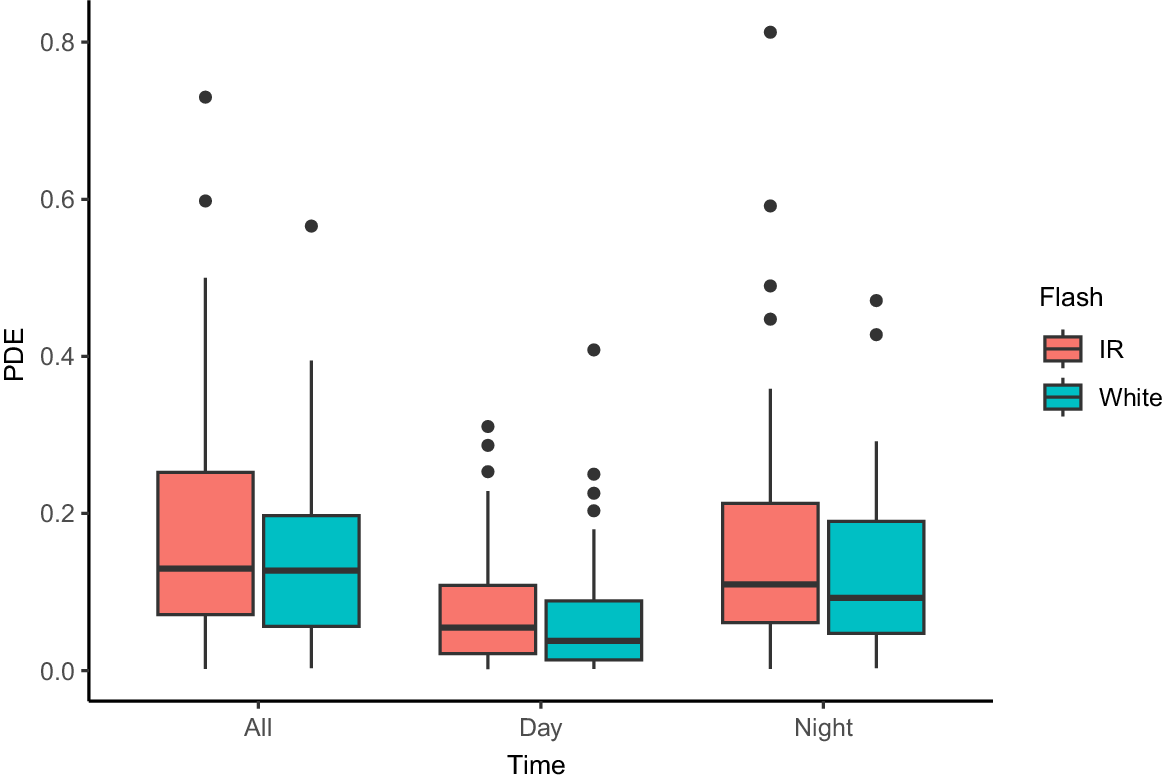
Flash type did not have a significant impact on feral cat PDE at a camera (odds ratio = 0.9, CI = 0.55, 1.47). We found no evidence of an interaction between flash type and time (odds ratio = 0.75, CI = 0.51, 1.12), though cat PDE was 3.16 times higher at night (CI = 2.4, 4.16).
There were 200 cat events collected from 44 IR cameras, and 154 cat events from 44 white flash cameras across the first 45 days of their deployment. There was no significant change in the average number of daily feral cat captures over time (Fig. 5). No significant difference was detected between means for these three periods (odds ratio = 0.87 (CI = 0.49, 1.52) and 0.94 (CI = 0.53, 1.69) for 30 days and 45 days compared to 15-day grouping, respectively), nor for flash type (odds ratio = 0.83, CI = 0.47, 1.46).
Box-and-whisker plots showing the average proportion of days a cat event was captured at a camera-trap for each 15-day period for the first 45 days of deployment between white and infrared (IR) cameras. IR cameras are shown in red, white flash cameras in blue. Horizontal lines show the median activity (50th percentile), the box shows the interquartile range (IQR: 25th and 75th percentiles), the vertical lines [whiskers] represent 1.5 × IQR and the solid dots are outliers.
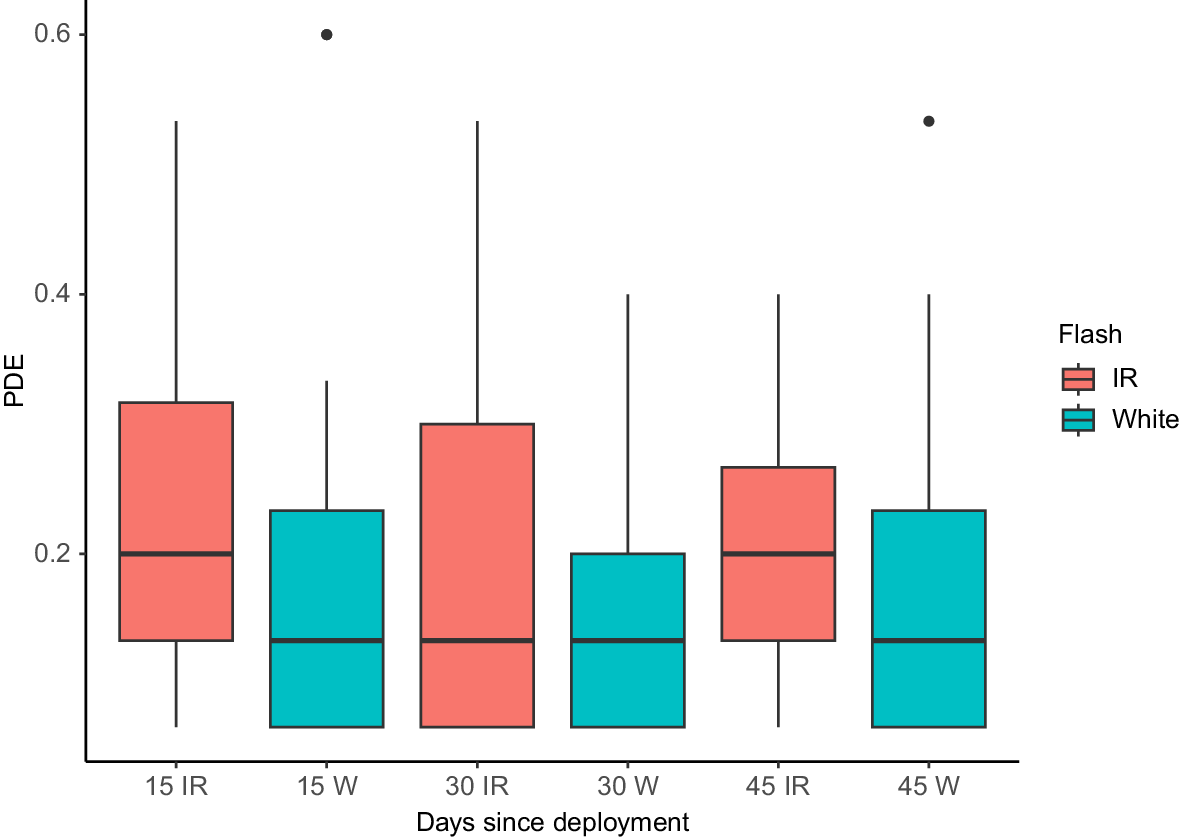
Feral cat behaviour was classified in 8731 events, with 5593 events from infrared cameras and 3138 from white flash cameras. During the night, 7.2% of the infrared events and 5.1% of the white flash events were camera focused. During the day, 8.1% of events from infrared cameras were camera focused, and 8.9% of events from white flash cameras were camera focused.
There was no significant difference in the probability of capturing a cat focusing on a camera-trap between flash types (odds ratio = 0.82, CI = 0.5, 1.36). This was true regardless of whether the image was captured during the day or at night (odds ratio = 1.32 CI = 0.89, 1.97). Feral cats were 0.68 times less likely to be captured investigating camera-traps at night, regardless of flash type (CI = 0.49, 0.94).
We assigned individual identifications to 4901 events from 106 camera-traps (55 IR and 51 white flash), excluding events that captured unmarked or unknown cats. Within these, we identified 259 individual cats. The average individual relative activity index (IRAI) was 0.027 (97.5% CI = 0.023, 0.032) across all cameras and individuals. The IRAI was similar between camera types, with white flash cameras having an average IRAI of 0.026 (CI = 0.021, 0.032), and infrared cameras having an IRAI of 0.028 (CI = 0.022, 0.035).
Discussion
We found no evidence to suggest that the tendency of feral cats to visit a camera-trap site differed between flash types. There was no evidence of the development of trap shyness over time, both when examining overall cat activity or the activity of individuals. Cats are aware of camera-traps in their environment, regardless of flash type used, but this does not appear to be detrimental to their capture.
There was no difference in the relative activity of feral cats across flash types, supporting the findings of Taggart et al. (2020). As we were utilising data from a pre-existing camera-trap network, the placement of the camera-traps was undertaken with the goal to maximise survey effort and spatial coverage, rather than to specifically compare flash types (i.e. with a paired design). Despite all camera-traps being placed on roads or man-made tracks, we are aware that infrared camera-traps were preferentially placed on roads where an existing theft of a camera had occurred. These roads might be more easily accessible and better maintained, as these conditions intuitively attract higher human traffic. By nature of the infrared cameras being on roads with higher human activity, these may also have been tracks that were better maintained. Feral cats have been reported to preferentially utilise roads and tracks (Wysong et al. 2020), which begs the question as to whether track or road quality also influences cat activity. This may explain why we saw slightly higher average cat PDE on the infrared cameras. The influence of track quality on feral cat activity remains unknown, though we suspect it may have contributed to this insignificant yet consistent trend we observed.
Our examination of individual relative activity rate at each camera-trap (IRAI) further confirms that feral cats generally return to a camera-trap regardless of the flash type used. We would expect a lower IRAI for white flash cameras if individuals avoided these camera-traps after first or second capture, even if immigration by a new individual into that area made the PDE appear higher. An ideal experiment to confirm this idea could involve a radio or GPS collared population of cat, with movements monitored both before and after white and infrared camera-trap deployment. For now, our opportunistic analysis reveals no difference in the rates at which individual cats return to infrared or white flash camera-traps.
We found no evidence to support claims that white flash cameras result in trap shyness in felids when following the methods of Wegge et al. (2004). Their study saw a decline in tiger (Panthera tigris) visitation to white flash camera-traps over 15 trap-nights. This contrast between our findings may be due to differences in the species’ behaviour (i.e. tigers may be more neophobic than feral cats), or the methodology. Within Wegge et al. (2004), camera-traps were serviced daily, and impression pads were made by removing leaves and digging up topsoil to capture tracks either side of the camera-trap. The increased disturbance and repeated reinforcement of human scent at the site might have influenced tiger response more than the camera’s flash, with Wegge et al. (2004) even reporting that the big cats would often make it to the impression pads and then turn around before they reached the camera-trap. In comparison, our camera-traps were serviced an average of every five months, meaning disturbance to the environment, at least by the researchers, was low. The influence of human activity at trap sites on felid return rate ought to be investigated further so that this potential disturbance can be quantified.
The general assumption from past studies is that white flash cameras will be more conspicuous to felids, with the bright light from the camera-trap being reported to ‘shock’ captive Felis catus (Glen et al. 2013 ). Due to the nature of the survey design from which we have taken these data, we do not know if feral cats exhibited a delayed fearful response to the camera-traps, as we only capture a single image of the animal rather than a burst of images or a video. As such, we sought to identify if infrared images or day images were less conspicuous than white flash, as is often assumed. Our evidence shows that feral cats are aware of camera-traps irrespective of the flash type used and are more likely to notice the camera-traps during the day when flash type is irrelevant. It appears that infrared camera-traps are no more ‘hidden’ to feral cats than white flash cameras. Infrared camera-traps produce a low hum briefly before firing, and this auditory output can be heard by feral cats (Meek et al. 2014b), giving the animal a second of warning, allowing them to look at, or move closer to, the trap before the image is taken. Despite having similar rates of investigation, we suspect that cats notice these flash types for different reasons. Whether feral cats exhibit a difference in their rate of distressed behaviours in response to these sudden auditory or visual disruptions requires further in-field trials (Meek et al. 2016).
The lack of predators of feral cats across our study sites may mean that feral cats are bolder and more inquisitive here than they would be in areas containing dingos or foxes. Feral cats are often outcompeted by Tasmanian devils (Cunningham et al. 2020; Fielding et al. 2021), which were detected across most of our study sites, though it is unlikely that devils often prey on cats directly. Considering that the only other study directly comparing camera trap flash types for feral cats in Australia was from Kangaroo Island (Taggart et al. 2020), where predators of feral cats are also absent, it is worth considering whether the presence of a predator could change feral cat behaviour. Meek et al. (2016) found that feral cats were curious about infrared camera traps during the day and at night despite the presence of wild dogs and foxes. Nonetheless, it is uncertain whether those results will extrapolate to white flash cameras. Our results should be transferable to other predator-free regions with feral cats, such as New Zealand, across Tasmania, or on many off-shore islands.
With the largest-scale study on the topic to date, we found no evidence to suggest that camera-trap flash type influenced the relative activity of feral cats, both as a group and as individuals, nor that the presence of camera-traps in the environment resulted in trap shyness. Given the improved image quality provided by white flash cameras, these results suggest that this flash type can be used in studies that require the individual identification of feral cats without reducing redetection. Overall, our findings indicate that camera-trap flash type should be chosen based on survey practicalities, not for fear of impacting cat detections. These practicalities could include increasing battery life or reducing the risk of theft, where less-visible infrared flash cameras are preferable, or where high-quality images are required, white flash may be favoured.
Data availability
The data and code used in this paper are available on GitHub (https://github.com/AlexJPaton).
Declaration of funding
Funding provided by the Australian Research Council (FL160100101), http://dx.doi.org/10.13039/501100000923.
Acknowledgements
We thank additional members of the DEEP research group at the University of Tasmania for their contribution towards data collection.
References
Bengsen A, Butler J, Masters P (2011) Estimating and indexing feral cat population abundances using camera traps. Wildlife Research 38(8), 732-739.
| Crossref | Google Scholar |
Burns PA, Parrott ML, Rowe KC, Phillips BL (2018) Identification of threatened rodent species using infrared and white-flash camera traps. Australian Mammalogy 40(2), 188-197.
| Crossref | Google Scholar |
Cove MV, Jackson VL (2011) Differences in detection probability between camera trap types for surveying bobcats in a fragmented suburban landscape. Wild Felid Monitor 4(2), 24.
| Google Scholar |
Cunningham CX, Johnson CN, Jones ME (2020) A native apex predator limits an invasive mesopredator and protects native prey: Tasmanian devils protecting bandicoots from cats. Ecology Letters 23(4), 711-721.
| Crossref | Google Scholar | PubMed |
Doherty TS, Dickman CR, Johnson CN, Legge SM, Ritchie EG, Woinarski JCZ (2017) Impacts and management of feral cats Felis catus in Australia. Mammal Review 47(2), 83-97.
| Crossref | Google Scholar |
Efford MG, Hunter CM (2018) Spatial capture–mark–resight estimation of animal population density. Biometrics 74(2), 411-420.
| Crossref | Google Scholar | PubMed |
Fielding MW, Cunningham CX, Buettel JC, Stojanovic D, Jones ME, Brook BW (2021) Dominant carnivore loss benefits native avian and invasive mammalian scavengers. Proceedings of the Royal Society B 289(1985), 20220521.
| Google Scholar |
Glen AS, Cockburn S, Nichols M, Ekanayake J, Warburton B (2013) Optimising camera traps for monitoring small mammals. PLoS ONE 8(6), e67940.
| Crossref | Google Scholar | PubMed |
Henrich M, Niederlechner S, Kröschel M, Thoma S, Dormann CF, Hartig F, Heurich M (2020) The influence of camera trap flash type on the behavioural reactions and trapping rates of red deer and roe deer. Remote Sensing in Ecology and Conservation 6(3), 399-410.
| Crossref | Google Scholar |
Herrera DJ, Moore SM, Herrmann V, McShea WJ, Cove MV (2021) A shot in the dark: white and infrared LED flash camera traps yield similar detection probabilities for common urban mammal species. Hystrix 32(1), 72-75.
| Crossref | Google Scholar |
Hoffmann BD, Broadhurst LM (2016) The economic cost of managing invasive species in Australia. NeoBiota 31, 1-18.
| Crossref | Google Scholar |
Kays R, Arbogast BS, Baker-Whatton M, Beirne C, Boone HM, Bowler M, Burneo SF, Cove MV, Ding P, Espinosa S, et al. (2020) An empirical evaluation of camera trap study design: how many, how long and when? Methods in Ecology and Evolution 11, 700-713.
| Crossref | Google Scholar |
Ladd R, Meek P, Leung LK-P (2023) The influence of camera-trap flash type on the behavioural response, detection rate and individual recognition of Eld’s deer. Wildlife Research 50, 475-483.
| Crossref | Google Scholar |
Legge S, Murphy BP, McGregor H, Woinarski JCZ, Augusteyn J, Ballard G, Baseler M, Buckmaster T, Dickman CR, Doherty T, et al. (2017) Enumerating a continental-scale threat: how many feral cats are in Australia? Biological Conservation 206, 293-303.
| Crossref | Google Scholar |
Meek PD, Ballard G, Claridge A, Kays R, Moseby K, O’brien T, O’connell A, Sanderson J, Swann DE, Tobler M, Townsend S (2014a) Recommended guiding principles for reporting on camera trapping research. Biodiversity and Conservation 23(9), 2321-2343.
| Crossref | Google Scholar |
Meek PD, Ballard G-A, Fleming PJS, Schaefer M, Williams W, Falzon G (2014b) Camera traps can be heard and seen by animals. PLoS ONE 9(10),.
| Crossref | Google Scholar |
Meek P, Ballard G, Fleming P, Falzon G (2016) Are we getting the full picture? Animal responses to camera traps and implications for predator studies. Ecology and Evolution 6(10), 3216-3225.
| Crossref | Google Scholar | PubMed |
Nottingham CM, Buckley HL, Case BS, Glen AS, Stanley MC (2022) Factors affecting home range size of feral cats: a meta-analysis. New Zealand Journal of Ecology 46(2), 3476.
| Crossref | Google Scholar |
R Development Core Team (2010) ‘R: A language and environment for statistical computing.’ (R Foundation for Statistical Computing: Vienna, Austria) Available at https://www.r-project.org/
Rees MW, Pascoe JH, Wintle BA, Le Pla M, Birnbaum EK, Hradsky BA (2019) Unexpectedly high densities of feral cats in a rugged temperate forest. Biological Conservation 239, 108287.
| Crossref | Google Scholar |
Sparkes J, Fleming PJS, McSorley A, Mitchell B (2021) How many feral cats can be individually identified from camera trap images? Population monitoring, ecological utility and camera trap settings. Ecological Management & Restoration 22(3), 246-255.
| Crossref | Google Scholar |
Stokeld D, Frank ASK, Hill B, Choy JL, Mahney T, Stevens A, Young S, Rangers D, Rangers W, Gillespie GR (2016) Multiple cameras required to reliably detect feral cats in northern Australian tropical savanna: an evaluation of sampling design when using camera traps. Wildlife Research 42(8), 642-649.
| Crossref | Google Scholar |
Taggart PL, Peacock DE, Fancourt BA (2020) Camera trap flash-type does not influence the behaviour of feral cats (Felis catus). Australian Mammalogy 42(2), 220-222.
| Crossref | Google Scholar |
Wegge P, Pokheral CP, Jnawali SR (2004) Effects of trapping effort and trap shyness on estimates of tiger abundance from camera trap studies. Animal Conservation 7, 251-256.
| Crossref | Google Scholar |
Wysong ML, Iacona GD, Valentine LE, Morris K, Ritchie EG (2020) On the right track: placement of camera traps on roads improves detection of predators and shows non-target impacts of feral cat baiting. Wildlife Research 47, 557-569.
| Crossref | Google Scholar |
Yates LA, Aandahl Z, Richards SA, Brook BW (2023) Cross validation for model selection: a review with examples from ecology. Ecological Monographs 93(1), e1557.
| Crossref | Google Scholar |