The parasites of free-ranging terrestrial wildlife from Australia’s south-west
Amy S. Northover

A
B
C
D
E
F
G
Abstract
The conservation management of threatened wildlife increasingly relies upon translocations to augment populations. Translocations, however, pose various risks: from the host perspective these include the spread of parasitic disease, whereas from a broader biodiversity perspective translocation may lead to the loss of rare parasites and other dependent fauna. Although Disease Risk Analyses are recommended during translocation planning, knowledge regarding the parasites infecting threatened species or their pathogenicity is often lacking. Between March 2014 and June 2016, woylies (Bettongia penicillata) and sympatric marsupials were screened for the presence of endo- and ectoparasites, during two fauna translocations in south-western Australia. Here, we summarise the parasite taxa identified from B. penicillata, brush-tailed possums (Trichosurus vulpecula hypoleucus) and chuditch (Dasyurus geoffroii), including prevalence data for host, parasite taxon and site. Results from the opportunistic sampling of other species (Isoodon fusciventer, Phascogale tapoatafa wambenger, Tiliqua rugosa and Felis catus) are also presented. New host–parasite records including Hepatozoon spp. from T. v. hypoleucus, Trypanosoma noyesi from T. rugosa, Ixodes australiensis and Ixodes tasmani from D. geoffroii, and I. australiensis and Amblyomma sp. from a P. t. wambenger were identified. This study highlights the importance of monitoring sympatric species, particularly when compiling baseline data of parasite fauna present within translocation sites and enhances our knowledge of parasites infecting terrestrial wildlife within Australia’s south-west, a Global Biodiversity Hotspot.
Keywords: Bettongia penicillata, Dasyurus geoffroii, disease, fauna translocation, Hepatozoon spp, host-parasite ecology, Isoodon fusciventer, Phascogale tapoatafa wambenger, Tiliqua rugosa, Trichosurus vulpecula hypoleucus, trypanosome, woylie.
Introduction
Interactions between wildlife, domestic animals and humans are increasing globally, enhancing the likelihood of disease transmission including the spillover of pathogens with zoonotic potential (Leifels et al. 2022). As such, wildlife health surveillance for the detection of emerging zoonotic disease continues to receive significant attention. The importance of understanding wildlife pathogen diversity, however, also benefits the conservation management of threatened species and the broader ecosystem (Thompson et al. 2010). On the one hand, wildlife disease is poorly understood and although identified as a key threat to some species at risk of extinction (e.g. McCallum 2012), its importance is likely to be globally underestimated. On the other hand, parasites may play key roles in maintaining ecosystem function, through direct and indirect effects on free-living communities (Preston et al. 2016; Lymbery and Smit 2023). More research is needed to determine the biological significance of parasites and the effect of altering host–parasite associations on individual hosts (e.g. reproductive fitness, survivorship), host populations (e.g. population health, growth rates) and community interactions (e.g. competition, predation), particularly during conservation management interventions where disease risk may be heightened (e.g. fauna translocations: Ewen et al. 2015).
Fauna translocations play a pivotal role in the management of threatened fauna worldwide, with their conservation benefits extending beyond the species being translocated to also re-establish natural ecosystem structures and processes (IUCN/SSC 2013). Within Australia, translocations are increasingly employed to manage threatened fauna, though success rates remain low (Morris et al. 2015; Watkins et al. 2018). Although introduced predators have been unequivocally identified as a key factor contributing towards the failure of many translocations of medium-sized terrestrial mammals within Australia (Short 2010; Morris et al. 2015; Moseby et al. 2015), other factors such as disease may also impact translocation outcomes (Thompson et al. 2010; Ewen et al. 2015; Vaughan-Higgins 2019).
Parasites are often considered a major ‘health hazard’ during translocations (Ewen et al. 2015) and have been associated with translocation failures (e.g. chytridiomycosis: Scheele et al. 2021). Parasites also form a vital component of biodiversity (Hudson et al. 2006; Dougherty et al. 2016) and there is growing evidence to suggest that host immunity and the persistence of host populations may be enhanced if parasites are conserved, rather than eradicated, during translocation (Pizzi 2009; McGill et al. 2010; Boyce et al. 2011; Rideout et al. 2016). Many parasites have coevolved with their host, and do not induce disease under homeostatic conditions (Clark 2011); however, fauna translocations have the potential to disrupt the parasite community within a host (see Northover et al. 2018a), which may change the host–parasite balance to the detriment of either the host (Telfer et al. 2010) or the parasite (Moir et al. 2012). Although parasite management during translocations has been reviewed (e.g. Cunningham 1996; Stringer and Linklater 2014; Northover et al. 2018a) and frameworks to manage disease risk developed (e.g. Ewen et al. 2015; Dunlop and Watson 2022), parasites and parasitic disease are still not adequately considered during translocations. To assess both the disease risks associated with translocating wildlife and the extinction risk to parasites and other dependent fauna accompanying translocated wildlife, knowledge regarding the parasite species infecting a host and other sympatric species is required.
The woylie or brush-tailed bettong (Bettongia penicillata Gray, 1837) is an Australian macropodoid marsupial (Family Potoroidae), that is currently listed as Critically Endangered (Woinarski and Burbidge 2016). Once abundant across most of southern and central Australia, B. penicillata populations suffered catastrophic declines following European settlement (de Tores and Start 2008). By the 1960s populations of B. penicillata were confined to three isolated areas: the Upper Warren region [UWR (Kingston and Perup subpopulations)], Tutanning and Dryandra Woodland (henceforth referred to as Dryandra), within south-western Australia (Sampson 1971; Wayne et al. 2015). The Tutanning population is now considered functionally extinct (Pacioni et al. 2014; A. Wayne pers. comm.).
In response to conservation efforts (namely introduced predator control and translocations) B. penicillata numbers recovered, and in 1996 the species conservation status was downgraded (Start et al. 1998). Unexpectedly, however, B. penicillata populations declined by more than 90% between 1999 and 2006 (Wayne et al. 2013). The nature of the declines, with a distinctive spatio-temporal pattern in conjunction with a high prevalence of skin disease, was suggestive of infectious disease (Wayne et al. 2015). Health investigations conducted during the decline failed to identify an underlying cause (Wayne et al. 2013); however, research has been ongoing, and recovery initiatives have secured the persistence of the species, with the extant UWR and Dryandra populations showing signs of recovery in recent years (National Environmental Science Program Threatened Species Research Hub 2019).
Over the past decade studies have focused on the potential role of trypanosomes (blood-borne parasites) given their pathogenicity and association with B. penicillata population declines (Smith et al. 2008; Botero et al. 2013; Thompson et al. 2014a; Godfrey et al. 2018). The role of viruses has also been explored, though Pacioni et al. (2014) found no evidence of exposure to viral pathogens in several B. penicillata populations, concluding that viral disease was an unlikely cause of the declines. While Skogvold et al. (2017) subsequently detected herpesviruses in B. penicillata from Perup Sanctuary, infected hosts (n = 5) did not show signs of disease, thus the clinical significance of infection remains uncertain. The role of other parasites (e.g. Rong et al. 2012; Northover et al. 2018b) and stress (e.g. Hing et al. 2017) has also been investigated, as has the coextinction risk for the parasite community associated with B. penicillata (Thompson et al. 2018).
In comparison to B. penicillata, knowledge of host–parasite associations in wild populations of sympatric marsupials such as the koomal or brush-tailed possum (Trichosurus vulpecula hypoleucus) and chuditch or western quoll (Dasyurus geoffroii) is limited, with most records documented from captive animals or those inhabiting urban and periurban environments (e.g. Haigh 1994; Clark 2011; Hillman et al. 2018; Loh et al. 2018; Egan et al. 2021). In wild populations, Thomasz (2014) documented the parasites infecting D. geoffroii (n = 36) from Julimar Conservation Park and the UWR during a translocation project, and Cooper et al. (2018) investigated the Trypanosoma spp. infecting T. vulpecula (n = 32) and D. geoffroii (n = 3) within the UWR. Molecular surveillance of ticks parasitising marsupials has provided further insight regarding the Trypanosoma spp. infecting T. vulpecula (n = 15) (Krige et al. 2021a).
Given the poor understanding of the parasite species infecting Western Australia’s (WA) native fauna (Wayne et al. 2015), the purpose of this study was to screen wild populations of B. penicillata and sympatric species (primarily T. v. hypoleucus and D. geoffroii) within the UWR and Dryandra for the presence of blood-borne, gastrointestinal and ectoparasite taxa to better understand parasite diversity among hosts and between sites. Exposure to Toxoplasma gondii was also examined in a subset of B. penicillata. We were particularly interested in documenting the prevalence of host-generalist parasites (i.e. those able, in the same stage of the life cycle, to infect more than one species of host), because they may pose a greater disease risk to declining host populations (De Castro and Bolker 2005) and are more likely to cause emerging infectious disease (Dharmarajan et al. 2021). By obtaining a greater understanding of the parasite diversity present within WA’s native fauna populations, we hope to improve the management of threatened species and their parasite taxa during future translocations, to better inform Disease Risk Analyses and guide the conservation of parasite species, where these do not pose a disease risk to their hosts.
Methods
Trapping and sample collection
Between March 2014 and June 2016, small mammal trapping was conducted within the UWR and Dryandra in collaboration with the Department of Biodiversity, Conservation and Attractions (DBCA) (under Regulation 4 and 17 Scientific Licences), during two fauna translocations to supplement wild B. penicillata populations in south-western WA (see Northover et al. (2019a) and (2019b) for details of trapping regime). Ethics approval was obtained from Murdoch University (permit number RW2659/14). Bettongia penicillata were translocated (1) from Perup Sanctuary into two unfenced sites, Walcott and Warrup East, in June 2014; and (2) from six unfenced sites within the UWR (Balban, Boyicup, Corbal, Dudijup, Dwalgan and Winnejup), into an unfenced site within Dryandra in June 2015 (Fig. 1). Animals were monitored for up to 12 months following translocation.
Map illustrating our study sites within south-western Western Australia. The box (right) shows Walcott and Warrup East (orange) in relation to Perup Sanctuary and the six UWR transects. Dryandra is located roughly 150 km north-east of the Upper Warren region.
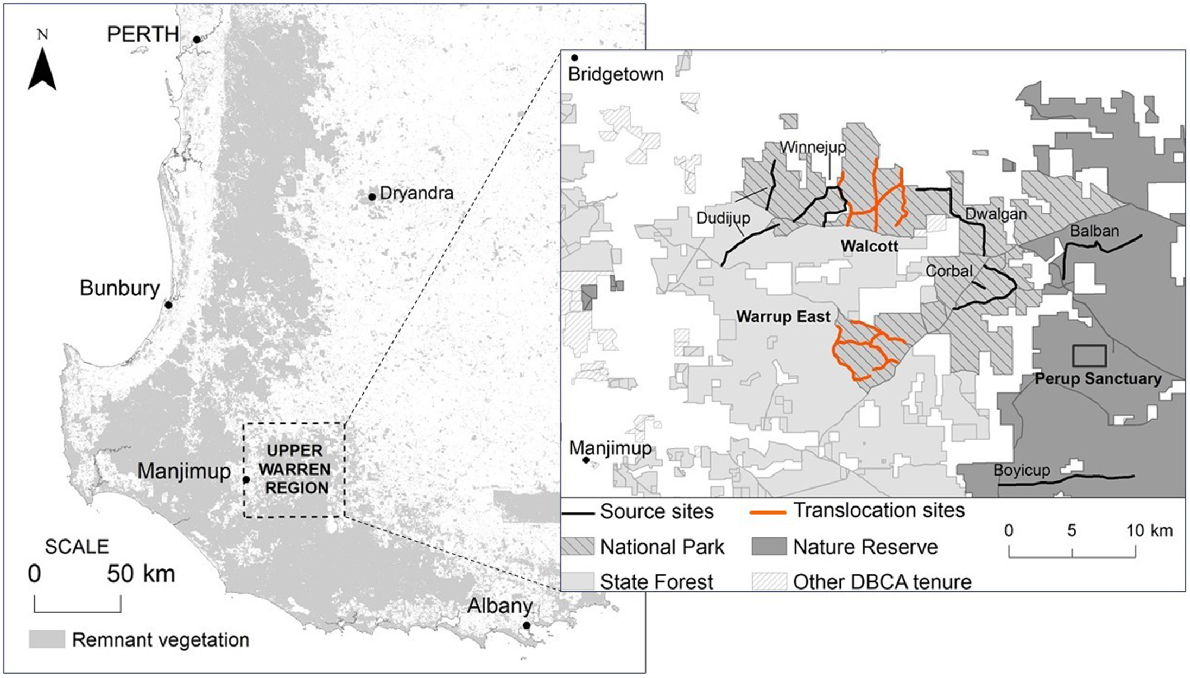
Animals were captured using Sheffield cage traps, which were set at dusk and baited with universal bait (peanut butter, rolled oats and sardines). Trapping equipment and handling bags were cleaned and sterilised prior to each trapping session, but were used for all species, over consecutive days. Bags were shaken out to remove faeces, hair, dirt etc. (or replaced if soiled) after handling each animal to reduce the risk of cross contamination. Fresh newspaper was placed under each trap after each capture, and traps were roughly cleaned (i.e. shaken out or brushed) in the field to remove large bits of faecal material prior to resetting. Except for July 2015 (night trapping), traps were cleared at first light within 3 h of sunrise each day.
Samples were collected from animals at each capture. Individual (target) animals were identified using two uniquely numbered ear-tags. Blood and ectoparasites were obtained using manual restraint in B. penicillata, bobtail (shingleback) lizards (Tiliqua rugosa), and a brush-tailed phascogale (Phascogale tapoatafa wambenger; ectoparasites opportunistically collected by DBCA staff), and under general anaesthesia for all other species. Inhalational anaesthesia using isoflurane in 100% oxygen was delivered via facemask under the supervision of a registered veterinary surgeon in the field.
Parasite analysis
Up to 1 mL of blood was collected from the lateral caudal (tail) vein in B. penicillata, and either the jugular or tail vein in other marsupials, into EDTA MiniCollect tubes (Greiner Bio-One, Germany), and frozen at −20°C before processing. In T. rugosa, up to 0.5 mL of blood was collected from the ventral coccygeal vein. Peripheral blood smears were prepared in the field using the ‘wedge’ method (see Clark et al. 2004) and stained with Diff-Quik prior to mounting with dibutylphthalate polystyrene xylene. Blood smears from a subset of Trypanosoma-positive hosts (B. penicillata and T. v. hypoleucus only) were inspected by light microscopy for the presence of haemoprotozoans. Trypanosoma spp. were the main haemoparasites monitored during this study, though the seroprevalence of T. gondii was also determined in a subset of B. penicillata. Trypanosome detection methods (PCR and Sanger sequencing) have been described by Northover et al. (2019a). While clade specific primers were used to identify T. vegrandis, T. copemani and T. noyesi, Sanger sequencing was used to identify T. sp. ANU2 and T. gilletti, as species specific primers for these trypanosomes were not available. Targeted amplicon next generation sequencing of the Trypanosoma 18S rDNA loci (see Cooper et al. 2018) was performed on samples in which species identification could not be achieved with Sanger sequencing.
A commercial modified agglutination test (Toxo-Screen DA, bioMerieux, France) was used as per the manufacturer’s instructions to determine the seroprevalence of T. gondii. In this test, formalin-treated T. gondii antigen was added to the sera and agglutination was expected to occur in the presence of specific IgG antibodies (Couzineau and Baufine-Ducrocq 1970). A denaturing agent (2-mercaptoethanol) was used to avoid agglutination by non-specific IgM antibodies (Couzineau and Baufine-Ducrocq 1970). Each sample used two dilutions (1/40 and 1/4000) and every plate contained a positive, negative, and antigen control. Results were interpreted as per the manufacturer’s guidelines, whereby a seropositive result presented as an agglutination mat covering more than half of the well, and a seronegative result presented as a red button. Borderline reactions were retested.
Newspaper was placed under each trap to collect faeces. Faecal samples were preserved in 10% buffered formalin and refrigerated at 4°C until processing. Faeces were examined for the presence of gastrointestinal parasite eggs, oocysts, and larvae, using the modified sodium nitrate (NaNO3) faecal flotation protocol described by Northover et al. (2017); see Appendices (Figs 4–7) for criteria used to differentiate gastrointestinal parasite taxa.
Each individual was examined in a systematic manner for the presence of ectoparasites (fleas, lice, mites and ticks). A subset of the different ectoparasites observed at the time of sampling were collected from each animal and stored in 70% ethanol. Ectoparasites were morphologically identified by Murdoch University parasitology staff using keys developed by Roberts (1970), von Kéler (1971), Dunnet and Mardon (1974), and Domrow (1987).
Data analysis
For each parasite taxon, prevalence of infection was calculated as the proportion of infected samples using Agresti-Coull 95% confidence intervals for sample sizes >40 and Wilson score 95% confidence intervals (with continuity correction) for sample sizes <40 (Brown et al. 2001), assuming a binomial distribution.
Results
Haemoparasites
The Trypanosoma spp. (and piroplasms) infecting B. penicillata have previously been documented, based on the analysis of 1211 blood samples from 596 individuals (Northover et al. 2019a). Here we analysed an additional 194 blood samples from 151 (119 not previously sampled) individuals for the presence of trypanosomes. With both datasets combined (1405 blood samples from 715 individuals) 54.0% of samples were Trypanosoma-positive (Table 1); 80 samples could not be identified to species. Prevalence of infection among individuals (i.e. a positive result at any time point during the monitoring period) was 65.3%. Of the remaining 1325 samples, five Trypanosoma species (T. vegrandis, T. copemani, T. noyesi, T. gilletti and T. sp. ANU2) were detected (Table 1). Trypanosoma vegrandis was most prevalent, followed by T. copemani, T. noyesi, T. sp. ANU2 and T. gilletti (Table 1).
Haemoparasite | B. penicillata | T. v. hypoleucus | D. geoffroii | |
---|---|---|---|---|
Overall Trypanosoma spp. prevalence | 759/1405 (54.0%) | 262/317 (82.6%) | 14/119 (11.8%) | |
51.4–56.6% | 78.1–86.4% | 7.1–19.0% | ||
Trypanosoma vegrandis | 402/1325 (30.3%) | 77/313 (24.6%) | 5/118 (4.2%) | |
27.9–32.9% | 20.2–29.7% | 1.6–9.9% | ||
Trypanosoma copemani | 300/1325 (22.6%) | 183/313 (58.5%) | 8/118 (6.8%) | |
20.5–25.0% | 52.9–63.8% | 3.3–13.1% | ||
Trypanosoma noyesi | 100/1325 (7.5%) | 65/313 (20.8%) | 8/118 (6.8%) | |
6.2–9.1% | 16.6–25.6% | 3.3–13.1% | ||
Trypanosoma sp. ANU2 | 30/1325 (2.3%) | 3/313 (1.0%) | 1/118 (0.8%) | |
1.6–3.2% | 0.2–2.9% | 0.0–5.2% | ||
Trypanosoma gilletti | 11/1325 (0.8%) | 0/313 (0.0%) | 0/118 (0.0%) | |
0.4–1.5% | 0.0–1.5% | 0.0–3.9% | ||
Toxoplasma gondii | 11/617 (1.8%) | 0/4 (0.0%) | N/A | |
1.0–3.2% | 0.0–60.4% | |||
Unidentified piroplasms | Present | Present | N/A | |
Unidentified Hepatozoon spp. | Not detected | Present | N/A |
Of the Trypanosoma-positive samples that could be identified to species (n = 679), the prevalence of Trypanosoma spp. coinfection was 23.1% (95% CI: 20.1–26.4%), with up to three Trypanosoma species detected in a single host (Appendix Fig. 1). Trypanosoma prevalence and diversity varied between sites, with the highest overall prevalence detected within Walcott and the lowest species diversity within Dryandra (Appendix Table 1); T. sp. ANU2 and T. gilletti were not detected within Dryandra. Bettongia penicillata translocated into Dryandra (UWR origin) were also negative for T. gilletti. Both trypanosomes and piroplasms (Theileria and Babesia spp.) were observed in peripheral blood smears from B. penicillata (Fig. 2).
Haemoparasites identified in peripheral blood smears from woylies (Bettongia penicillata): (a) Trypanosoma copemani; (b) Theileria sp. (black arrow); (c) Babesia sp. (black arrows).
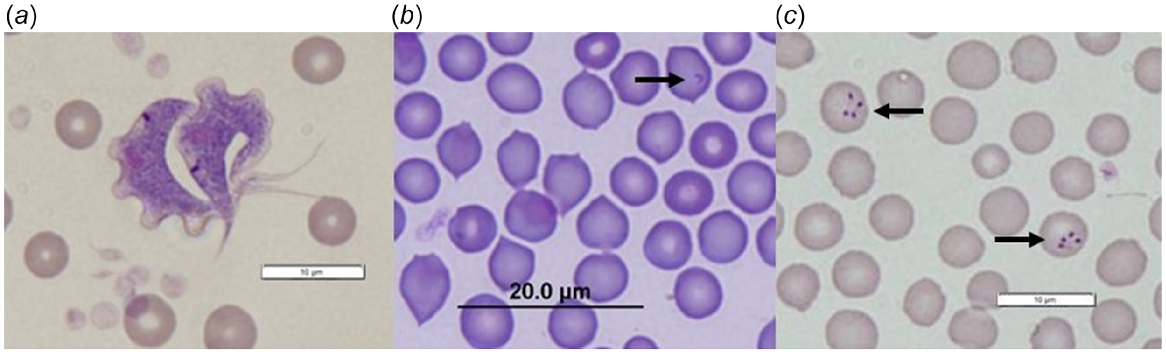
A subset of B. penicillata blood samples (n = 617) collected from 349 individuals was also screened for T. gondii, of which 11 samples (1.8%) were positive. Of these, five were from Warrup East (0.8%), four were from Walcott (0.6%; includes two positive results from the same host at different time points) and two were from Dryandra (0.3%).
In total, 317 blood samples collected from 226 individuals were screened for trypanosomes, of which 262 samples (82.6%) were positive (Table 1). Four samples that were positive on general PCR screening, could not be identified to species. Prevalence of infection among individuals was 86.7%. Four Trypanosoma species were detected in T. v. hypoleucus (T. copemani, T. vegrandis, T. noyesi and T. sp. ANU2) (Table 1), including up to three Trypanosoma species in a single host (all T. copemani, T. vegrandis, T. noyesi coinfection) (Appendix Fig. 2). Overall, T. copemani was most prevalent, followed by T. vegrandis and T. noyesi (Table 1). Trypanosoma sp. ANU2 was detected in only three hosts from Warrup East (Appendix Table 2). Coinfection with two or more trypanosomes was detected in 26.0% (95% CI: 21.0–31.7%) of positive samples. Warrup East had the greatest Trypanosoma spp. prevalence (100%) and diversity (Appendix Table 2). An unknown Hepatozoon species was identified in three blood smears from T. v. hypoleucus (Fig. 3); all three hosts originated from Dryandra and Trypanosoma spp. infection (T. copemani and T. vegrandis coinfection in two cases; T. copemani in the other) was also apparent. Trypanosomes and unidentified piroplasms were also detected in blood smears (Fig. 3). Four (UWR) T. v. hypoleucus that were screened for T. gondii were seronegative.
We screened 119 blood samples from 97 individuals for the presence of trypanosomes. Of these, 14 samples (11.8%) were positive (Table 1); one sample could not be identified to species. Prevalence of infection among individuals was 14.4%. Four Trypanosoma species were identified in D. geoffroii (Table 1), including up to three Trypanosoma species in a single host (Appendix Fig. 3). Trypanosoma copemani and T. noyesi were equally most prevalent, followed by T. vegrandis and T. sp. ANU2 (Table 1). Coinfection was detected in 46.2% (95% CI: 20.4–73.9%) of positive samples. Despite the high number of D. geoffroii sampled within Dryandra (n = 54), only one host was Trypanosoma-positive (T. noyesi). Except for T. sp. ANU2, which was detected in a single host from Warrup East, Walcott had the highest prevalence of Trypanosoma spp. infection (Appendix Table 3). Trypanosoma vegrandis was not detected in D. geoffroii from Warrup East or Dryandra, despite Warrup East (and Walcott) having high Trypanosoma spp. diversity.
Seven T. rugosa were screened for the presence of trypanosomes, three of which (42.9%) were positive; Amblyomma albolimbatum ticks were parasitising two of the Trypanosoma-positive hosts, the third had no visible ectoparasites. Trypanosoma noyesi was sequenced from two of the positive samples (Dryandra origin). The third positive sample (UWR origin) could not be interpreted with confidence due to difficulties encountered during the extraction process, thus species-level identification was not pursued. A single feral cat (Felis catus) captured during trapping in Warrup East tested negative for Trypanosoma spp. on general PCR screening. Trypanosoma vegrandis was sequenced in a quenda or south-western brown bandicoot (Isoodon fusciventer) from Perup Sanctuary; this was the only I. fusciventer screened for trypanosomes.
Gastrointestinal parasites
We examined 946 faecal samples collected from 377 individuals for the presence of gastrointestinal parasites. Five visually distinctive nematode eggs (typical strongyle, Strongyloides-like, Potoroxyuris sp., Trichuris sp. and Linstowinema sp.), a single type of cestode egg (undescribed species), coccidian oocysts (including Eimeria woyliei), and first stage (metastrongyloid) lungworm larvae were identified (Table 2, Appendix Fig. 4). Eimeria woyliei was identified in cases where sporulated oocysts were present and distinguishing morphological characteristics were observed (see Northover et al. 2019c); coccidian oocyst counts (Table 2) include E. woyliei. Unidentified nematode eggs/larvae were also found and may represent either infective nematode larvae or free-living nematodes.
Parasite taxa | B. penicillata | T. v. hypoleucus | D. geoffroii | I. fusciventer | |
---|---|---|---|---|---|
Overall prevalence | 859/946 (90.8%) | 174/234 (74.4%) | 99/112 (88.4%) | 12/12 (100%) | |
88.8–92.5% | 68.4–79.5% | 81.0–93.2% | 69.9–100% | ||
Anoplocephalid egg | 0/946 (0%) | 0/234 (0%) | 4/112 (3.6%) | 0/12 (0%) | |
0.0–0.5% | 0.0–2.0% | 1.1–9.2% | 0.0–30.1% | ||
Australiformis sp. egg | 0/946 (0%) | 0/234 (0%) | 0/112 (0%) | 1/12 (8.3%) | |
0.0–0.5% | 0.0–2.0% | 0.0–4.1% | 0.4–40.2% | ||
Bertiella sp. egg | 0/946 (0%) | 10/234 (4.3%) | 3/112 (2.7%) | 0/12 (0%) | |
0.0–0.5% | 2.3–7.8% | 0.6–8.0% | 0.0–30.1% | ||
Capillaria sp. egg | 0/946 (0%) | 0/234 (0%) | 1/112 (0.9%) | 0/12 (0%) | |
0.0–0.5% | 0.0–2.0% | 0.0–5.5% | 0.0–30.1% | ||
Cestode egg | 21/946 (2.2%) | 0/234 (0%) | 0/112 (0%) | 0/12 (0%) | |
1.4–3.4% | 0.0–2.0% | 0.0–4.1% | 0.0–30.1% | ||
Coccidian oocysts | 141/946 (14.9%) | 34/234 (14.5%) | 12/112 (10.7%) | 6/12 (50.0%) | |
12.8–17.3% | 10.6–19.7% | 6.1–18.0% | 25.4–74.6% | ||
Gravid (free-living) nematodes | 0/946 (0%) | 36/234 (15.4%) | 0/112 (0%) | 0/12 (0%) | |
0.0–0.5% | 11.3–20.6% | 0.0–4.1% | 0.0–30.1% | ||
Labiobulura sp. egg | 0/946 (0%) | 0/234 (0%) | 0/112 (0%) | 4/12 (33.3%) | |
0.0–0.5% | 0.0–2.0% | 0.0–4.1% | 11.3–64.6% | ||
Linstowinema sp. egg | 2/946 (0.2%) | 0/234 (0%) | 0/112 (0%) | 4/12 (33.3%) | |
0.0–0.8% | 0.0–2.0% | 0.0–4.1% | 11.3–64.6% | ||
Lungworm larvae | 13/946 (1.4%) | 0/234 (0%) | 0/112 (0%) | 3/12 (25.0%) | |
0.8–2.4% | 0.0–2.0% | 0.0–4.1% | 6.7–57.2% | ||
Megastrongyle egg | 0/946 (0%) | 0/234 (0%) | 9/112 (8.0%) | 0/12 (0%) | |
0.0–0.5% | 0.0–2.0% | 4.2–14.8% | 0.0–30.1% | ||
Oxyurid egg | 0/946 (0%) | 1/234 (0.4%) | 10/112 (8.9%) | 0/12 (0%) | |
0.0–0.5% | 0.0–2.7% | 4.8–15.9% | 0.0–30.1% | ||
Parastrongyloides egg | 0/946 (0%) | 33/234 (14.1%) | 0/112 (0%) | 0/12 (0%) | |
0.0–0.5% | 10.2–19.2% | 0.0–4.1% | 0.0–30.1% | ||
Physaloptera sp. egg | 0/946 (0%) | 0/234 (0%) | 1/112 (0.9%) | 0/12 (0%) | |
0.0–0.5% | 0.0–2.0% | 0.0–5.5% | 0.0–30.1% | ||
Potorolepis sp. egg | 0/946 (0%) | 0/234 (0%) | 0/112 (0%) | 3/12 (25.0%) | |
0.0–0.5% | 0.0–2.0% | 0.0–4.1% | 6.7–57.2% | ||
Potoroxyuris sp. egg | 18/946 (1.9%) | 0/234 (0%) | 0/112 (0%) | 0/12 (0%) | |
1.2–3.0% | 0.0–2.0% | 0.0–4.1% | 0.0–30.1% | ||
Protospirura sp. egg | 0/946 (0%) | 12/234 (5.1%) | 0/112 (0%) | 0/12 (0%) | |
0.0–0.5% | 2.9–8.9% | 0.0–4.1% | 0.0–30.1% | ||
Spiruroid egg | 0/946 (0%) | 0/234 (0%) | 3/112 (2.7%) | 0/12 (0%) | |
0.0–0.5% | 0.0–2.0% | 0.6–8.0% | 0.0–30.1% | ||
Strongyle egg | 712/946 (75.3%) | 157/234 (67.1%) | 92/112 (82.1%) | 10/12 (83.3%) | |
72.4–77.9% | 60.8–72.8% | 73.9–88.2% | 50.9–97.1% | ||
Strongyloides-like egg | 449/946 (47.5%) | 0/234 (0%) | 0/112 (0%) | 2/12 (16.7%) | |
44.3–50.6% | 0.0–2.0% | 0.0–4.1% | 2.9–49.1% | ||
Trichuris egg | 4/946 (0.4%) | 0/234 (0%) | 0/112 (0%) | 4/12 (33.3%) | |
0.1–1.1% | 0.0–2.0% | 0.0–4.1% | 11.3–64.6% | ||
Unidentified nematode eggs/larvae | 715/946 (75.6%) | 48/234 (20.5%) | 22/112 (19.6%) | 7/12 (58.3%) | |
72.7–78.2% | 15.8–26.2% | 13.3–28.2% | 28.6–83.5% |
Positive results are highlighted in bold.
Overall, 90.8% of samples contained at least one type of gastrointestinal parasite (including unidentified nematode eggs/larvae). Prevalence of infection among individuals was 95.2%. Excluding unidentified nematode eggs/larvae, strongyle eggs were most prevalent, with other parasite taxa detected less frequently (Table 2). Of the samples that were negative, the majority (92.1%) were from Dryandra. Except for cestode eggs, which were only detected in hosts from Dryandra, the prevalence and diversity of gastrointestinal parasite taxa was lowest within this site (Appendix Table 4). Linstowinema sp. eggs were found in just two hosts, while strongyle eggs, Strongyloides-like eggs and coccidian oocysts were ubiquitous across all sites. Potoroxyuris sp., Trichuris sp. eggs and lungworm larvae were only identified in hosts within or originating from Perup Sanctuary.
We examined 234 faecal samples from 112 hosts and detected four distinct types of nematode eggs (typical strongyle, Parastrongyloides sp., Protospirura sp. and oxyurid), a single type of anoplocephalid egg (Bertiella sp.), coccidian oocysts and unidentified nematode eggs/larvae (Table 2, Appendix Fig. 5). Distinctive, large gravid nematodes (approximately 200 μm in length) were also frequently observed (Appendix Fig. 5); we suspect these are free-living spp. based on their reproductive anatomy (Chitwood and Chitwood 1937). Overall, gastrointestinal parasites were identified in 74.4% of samples, and strongyle eggs were the most prevalent taxon detected (Table 2). Prevalence of infection among individuals was 92.0%. Species diversity was similar across all sites, with prevalences generally lower in Dryandra, except for coccidian oocysts and Bertiella sp. (Appendix Table 5). Parastrongyloides eggs were only found in hosts from the UWR, whereas strongyle eggs and coccidian oocysts were detected in all sites (Appendix Table 5).
We examined 112 faecal samples collected from 80 individuals and identified typical strongyle eggs, oxyurid eggs, spiruroid eggs (including Physaloptera sp.), two distinct anoplocephalid eggs [i.e. an unidentified sp. (Anoplocephalid egg) and Bertiella sp.], a Capillaria sp. egg, coccidian oocysts and unidentified nematode eggs/larvae (Table 2, Appendix Fig. 6). We also found a distinct morphotype of strongylid nematode egg (unidentified species referred to as ‘megastrongyle’ egg), which was much larger (approximately 150 μm × 75 μm) than typical strongyle eggs (Appendix Fig. 6). Overall, gastrointestinal parasites were present in 88.4% of samples, with strongyle eggs detected in 82.1% of samples (Table 2). Prevalence of infection among individuals was 90.0%. Species diversity was lowest in Walcott, with prevalences generally lower in Dryandra, except for Bertiella sp. (Appendix Table 6). Strongyle eggs, ‘megastrongyle’ eggs and coccidian oocysts were ubiquitous across all sites (Appendix Table 6).
Twelve faecal samples from I. fusciventer were screened for gastrointestinal parasites. Typical strongyle eggs, coccidian oocysts, Strongyloides-like eggs, lungworm (L1) larvae, Trichuris sp. eggs, Linstowinema sp. eggs, Labiobulura sp. eggs, Potorolepis sp. eggs, an Australiformis sp. egg and unidentified nematode eggs/larvae were observed (Table 2, Appendix Fig. 7). All 12 samples were positive for parasites, with strongyle eggs detected in all but two samples; coccidia were detected in 50% of samples. Two faecal samples from P. t. wambenger (Walcott origin) were also examined; both were positive for strongyle eggs; a Linstowinema sp. egg was detected in the faeces from one host and unidentified nematode larvae were present in the other (Appendix Fig. 8). Faecal samples from T. rugosa were not examined.
Ectoparasites
In total, 923 samples collected from 404 individuals were morphologically identified. Five species of tick (Amblyomma triguttatum, Ixodes australiensis, Ixodes myrmecobii, Ixodes tasmani and Ixodes woyliei), seven species of flea (Acidesta chera, Choristopsylla ochi, Echidnophaga myrmecobii, Echidnophaga perilis, Pygiopsylla hilli, Pygiopsylla tunneyi and Stephanocircus dasyuri), two species of louse (Boopia uncinata and Paraheterodoxus calcaratus), and four species of mite (Androlaelaps fahrenholzi, Haemolaelaps hattanae, Haemolaelaps marsupialis and Haemolaelaps quartus) were identified (Table 3). Larval/nymph stages of ticks and mites were also frequently encountered, but in most instances could not be identified to species. Trombiculid mites (Acari: Trombiculidae) were detected (based on their distinctive macroscopic appearance; Fig. 4a) on five hosts [Dryandra (origin UWR) n = 1; Perup Sanctuary n = 4] but were not collected for morphological identification.
Parasite taxa | B. penicillata | T. v. hypoleucus | D. geoffroii | ||
---|---|---|---|---|---|
Ticks | Amblyomma spp. | 81/923 (8.8%) | 21/194 (10.8%) | 0/84 (0%) | |
7.1–10.8% | 7.2–16.1% | 0.0–5.4% | |||
Amblyomma triguttatum | 2/923 (0.2%) | 0/194 (0%) | 0/84 (0%) | ||
0.00–0.8% | 0.0–2.4% | 0.0–5.4% | |||
Ixodes australiensis | 210/923 (22.8%) | 7/194 (3.6%) | 17/84 (20.2%) | ||
20.2–25.6% | 1.6–7.4% | 13.0–30.2% | |||
Ixodes fecialis | 0/923 (0%) | 0/194 (0%) | 11/84 (13.1%) | ||
0.0–0.5% | 0.0–2.4% | 7.4–22.2% | |||
Ixodes myrmecobii | 60/923 (6.5%) | 9/194 (4.6%) | 0/84 (0%) | ||
5.1–8.3% | 2.4–8.7% | 0.0–5.4% | |||
Ixodes tasmani | 1/923 (0.1%) | 69/194 (35.6%) | 7/84 (8.3%) | ||
0.00–0.7% | 29.2–42.5% | 3.9–16.6% | |||
Ixodes woyliei | 89/923 (9.6%) | 0/194 (0%) | 0/84 (0%) | ||
7.9–11.7% | 0.0–2.4% | 0.0–5.4% | |||
Haemaphysalis bancrofti | 0/923 (0%) | 2/194 (1.0%) | 0/84 (0%) | ||
0.0–0.5% | 0.1–4.0% | 0.0–5.4% | |||
Haemaphysalis bremneri | 0/923 (0%) | 1/194 (0.5%) | 0/84 (0%) | ||
0.0–0.5% | 0.0–3.2% | 0.0–5.4% | |||
Haemaphysalis humerosa | 0/923 (0%) | 2/194 (1.0%) | 0/84 (0%) | ||
0.0–0.5% | 0.1–4.0% | 0.0–5.4% | |||
Unidentified larval/nymph stages | 576/923 (62.4%) | 76/194 (39.2%) | 34/84 (40.5%) | ||
59.2–65.5% | 32.6–46.2% | 30.6–51.2% | |||
Unknown | 17/923 (1.8%) | 0/194 (0%) | 0/84 (0%) | ||
1.1–3.0% | 0.0–2.4% | 0.0–5.4% | |||
Fleas | Acidesta chera | 1/923 (0.1%) | 0/194 (0%) | 13/84 (15.5%) | |
0.00–0.7% | 0.0–2.4% | 9.2–24.9% | |||
Choristopsylla ochi | 1/923 (0.1%) | 71/194 (36.6%) | 1/84 (1.2%) | ||
0.00–0.7% | 30.1–43.6% | 0.0–7.2% | |||
Echidnophaga myrmecobii | 2/923 (0.2%) | 5/194 (2.6%) | 11/84 (13.1%) | ||
0.00–0.8% | 1.0–6.1% | 7.4–22.2% | |||
Echidnophaga perilis | 1/923 (0.1%) | 0/194 (0%) | 3/84 (3.6%) | ||
0.00–0.7% | 0.0–2.4% | 0.8–10.5% | |||
Pygiopsylla hilli | 82/923 (8.9%) | 0/194 (0%) | 0/84 (0%) | ||
7.2–10.9% | 0.0–2.4% | 0.0–5.4% | |||
Pygiopsylla tunneyi | 148/923 (16.0%) | 12/194 (6.2%) | 29/84 (34.5%) | ||
13.8–18.6% | 3.5–10.6% | 25.2–45.2% | |||
Stephanocircus dasyuri | 120/923 (13.0%) | 1/194 (0.5%) | 18/84 (21.4%) | ||
11.0–15.3% | 0.0–3.2% | 14.0–31.5% | |||
Unknown | 0/923 (0%) | 2/194 (1.0%) | 0/84 (0%) | ||
0.0–0.5% | 0.1–4.0% | 0.0–5.4% | |||
Lice | Boopia uncinata | 1/923 (0.1%) | 0/194 (0%) | 9/84 (10.7%) | |
0.00–0.7% | 0.0–2.4% | 5.6–19.4% | |||
Paraheterodoxus calcaratus | 624/923 (67.6%) | 2/194 (1.0%) | 3/84 (3.6%) | ||
64.5–70.5% | 0.1–4.0% | 0.8–10.5% | |||
Unidentified nymph stages | 6/923 (0.7%) | 0/194 (0%) | 0/84 (0%) | ||
0.3–1.5% | 0.0–2.4% | 0.0–5.4% | |||
Unknown | 8/923 (0.9%) | 0/194 (0%) | 1/84 (1.2%) | ||
0.4–1.7% | 0.0–2.4% | 0.0–7.2% | |||
Mites | Androlaelaps fahrenholzi | 1/923 (0.1%) | 0/194 (0%) | 0/84 (0%) | |
0.00–0.7% | 0.0–2.4% | 0.0–5.4% | |||
Haemolaelaps hattanae | 305/923 (33.0%) | 4/194 (2.1%) | 2/84 (2.4%) | ||
30.1–36.1% | 0.6–5.4% | 0.2–8.9% | |||
Haemolaelaps marsupialis | 1/923 (0.1%) | 2/194 (1.0%) | 4/84 (4.8%) | ||
0.00–0.7% | 0.1–4.0% | 1.6–12.1% | |||
Haemolaelaps quartus | 146/923 (15.8%) | 0/194 (0%) | 0/84 (0%) | ||
13.6–18.3% | 0.0–2.4% | 0.0–5.4% | |||
Liponyssoides lukoschusi | 0/923 (0%) | 6/194 (3.1%) | 0/84 (0%) | ||
0.0–0.5% | 1.3–6.8% | 0.0–5.4% | |||
Ornithonyssus dasyuri | 0/923 (0%) | 0/194 (0%) | 1/84 (1.2%) | ||
0.0–0.5% | 0.0–2.4% | 0.0–7.2% | |||
Ornithonyssus praedo | 0/923 (0%) | 1/194 (0.5%) | 0/84 (0%) | ||
0.0–0.5% | 0.0–3.2% | 0.0–5.4% | |||
Trombiculid mite | Present | 51/194 (26.3%) | 39/84 (46.4%) | ||
20.6–32.9% | 36.2–57.0% | ||||
Ulyxes penelope | 0/923 (0%) | 1/194 (0.5%) | 0/84 (0%) | ||
0.0–0.5% | 0.0–3.2% | 0.0–5.4% | |||
Unidentified larval/nymph stages | 11/923 (1.2%) | 16/194 (8.2%) | 22/84 (26.2%) | ||
0.6–2.2% | 5.1–13.1% | 18.0–36.6% | |||
Unknown | 9/923 (1.0%) | 9/194 (4.6%) | 0/84 (0%) | ||
0.5–1.9% | 2.4–8.7% | 0.0–5.4% |
Positive results are highlighted in bold.
Trombiculid mites on a woylie (Bettongia penicillata) (a; black arrow), ‘stick-fast’ fleas (Echidnophaga myrmecobii) on a brush-tailed possum (Trichosurus vulpecula hypoleucus) (b), and trombiculid mites on a chuditch (Dasyurus geoffroii) (c).
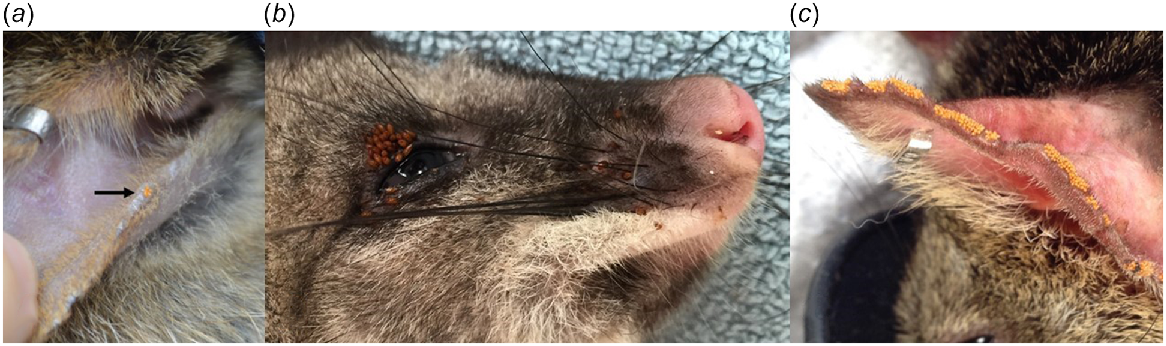
Of the 1314 B. penicillata (641 individuals) that were examined for the presence of ectoparasites in the field, 95.2% (95% CI: 93.9–96.2%) were positive for at least one ectoparasite taxon. Prevalence of infection among individuals was 98.0%. Fleas were recorded on only six hosts from Dryandra (one translocated, five resident); however, specimens were not collected for identification. Of the four ectoparasite taxonomic groups (ticks, fleas, lice and mites), ticks were most common (Appendix Table 7). From these groups, I. australiensis, P. tunneyi, P. calcaratus and H. hattanae were most prevalent, respectively; however, site differences were apparent (Appendix Table 8). For example, I. australiensis was not detected in Dryandra, despite this tick being the most prevalent species overall. In contrast, the prevalence of I. woyliei and Amblyomma spp. ticks was highest within Dryandra (Appendix Table 8), despite this site having the lowest overall prevalence of ticks (Appendix Table 7).
We morphologically identified 194 ectoparasite samples from 138 individuals. Seven species of tick (Amblyomma sp., Haemaphysalis bancrofti, Haemaphysalis bremneri, Haemaphysalis humerosa, I. australiensis, I. myrmecobii and I. tasmani), four species of flea (C. ochi, E. myrmecobii, P. tunneyi and S. dasyuri), one species of louse (P. calcaratus), and six mite taxa (H. hattanae, H. marsupialis, Liponyssoides lukoschusi, Ornithonyssus praedo, trombiculid mites and Ulyxes penelope) were identified (Table 3). Unidentified larval/nymph stages of ticks and mites were also common. ‘Stick-fast’ fleas were identified in the field based on their characteristic appearance embedded in the skin on seven hosts from Perup Sanctuary (Fig. 4b; confirmed as E. myrmecobii in five hosts).
Of the 323 T. v. hypoleucus (230 individuals) that were assessed for the presence of ectoparasites in the field, 81.1% (95% CI: 76.5–85.0%) were host to at least one ectoparasite taxon. Prevalence of infection among individuals was 88.7%. Of the three major ectoparasite taxonomic groups found on T. v. hypoleucus (ticks, fleas and mites), ticks were most common (Appendix Table 9). From these groups, I. tasmani, C. ochi, and trombiculid mites were most prevalent, respectively, though site differences were evident (Appendix Table 10).
Eighty-four ectoparasite samples collected from 80 individuals were morphologically identified. All four ectoparasite taxa were present on D. geoffroii, including three species of tick (I. australiensis, Ixodes fecialis and I. tasmani), six species of flea (A. chera, C. ochi, E. myrmecobii, E. perilis, P. tunneyi and S. dasyuri), two species of louse (B. uncinata and P. calcaratus), and four mite taxa (H. hattanae, H. marsupialis, Ornithonyssus dasyuri and trombiculid mites) (Table 3); unidentified larval/nymph stages of ticks and mites were also present.
Of the 120 D. geoffroii (99 individuals) that were examined for the presence of ectoparasites, at least one ectoparasite taxon was detected in 74.2% (95% CI: 65.6–81.2%) of cases. Prevalence of infection among individuals was 84.8%. Of the four major ectoparasite taxonomic groups, mites were the most common (Appendix Table 11). From these groups, I. australiensis, P. tunneyi, B. uncinata and trombiculid mites (Fig. 4c) were most prevalent, respectively (Appendix Table 12). Overall, the prevalence and diversity of ectoparasites was lowest in Dryandra (Appendix Tables 11 and 12).
Ticks were collected from seven T. rugosa and morphologically identified as Amblyomma albolimbatum in six hosts, and Amblyomma sp. in the other host. Fleas, lice and mites were not observed on T. rugosa. The fleas S. dasyuri and P. tunneyi were present on three I. fusciventer (origin Perup, Walcott and Warrup East); mites and ticks were also recorded as present on one of these hosts (Walcott) but were not collected for morphological identification. Amblyomma sp., I. australiensis and Ixodes sp. ticks were isolated from a P. t. wambenger.
Discussion
This study identified a diverse parasite community including nine haemoparasite taxa, 21 gastrointestinal parasite taxa and 27 ectoparasite taxa (Fig. 5) in free-ranging terrestrial wildlife from Australia’s south-west. Parasite diversity is likely to be grossly underestimated, given that species-level identification was not achieved for many taxa, particularly gastrointestinal parasites and larval/nymph stages of ectoparasites. To the best of our knowledge this is the first report of Hepatozoon spp. infection in T. v. hypoleucus, and T. noyesi in T. rugosa. Several new host–ectoparasite associations were also identified, including A. fahrenholzi and trombiculid mites on B. penicillata; L. lukoschusi on T. v. hypoleucus; I. australiensis and I. tasmani on D. geoffroii; and I. australiensis and Amblyomma sp. ticks on P. t. wambenger. Representative ectoparasite specimens, where available, have been deposited at the Western Australian Museum (reference numbers WAM E117331-E117336; T163145-T163157). We also report novel host–gastrointestinal parasite associations including the presence of Trichuris sp. and Linstowinema sp. eggs in B. penicillata, and Physaloptera sp. and Capillaria sp. eggs in D. geoffroii.
Summary of parasites identified from woylies (Bettongia penicillata), brush-tailed possums (Trichosurus vulpecula hypoleucus) and chuditch (Dasyurus geoffroii) in Western Australia (Photographs © John Lawson) (Baylis 1934; Inglis 1968; von Kéler 1971; Haigh et al. 1994; Burmej et al. 2008; Beveridge and Durette-Desset 2009; Clark 2011; Hillman 2016; Ash et al. 2017; Cooper et al. 2018; Thompson et al. 2018; Northover et al. 2019c; Egan et al. 2021; Krige et al. 2021b). Note: blue text, previously documented host–parasite associations; underlined text, new host–parasite associations; asterisk, query accidental (spurious) infection.
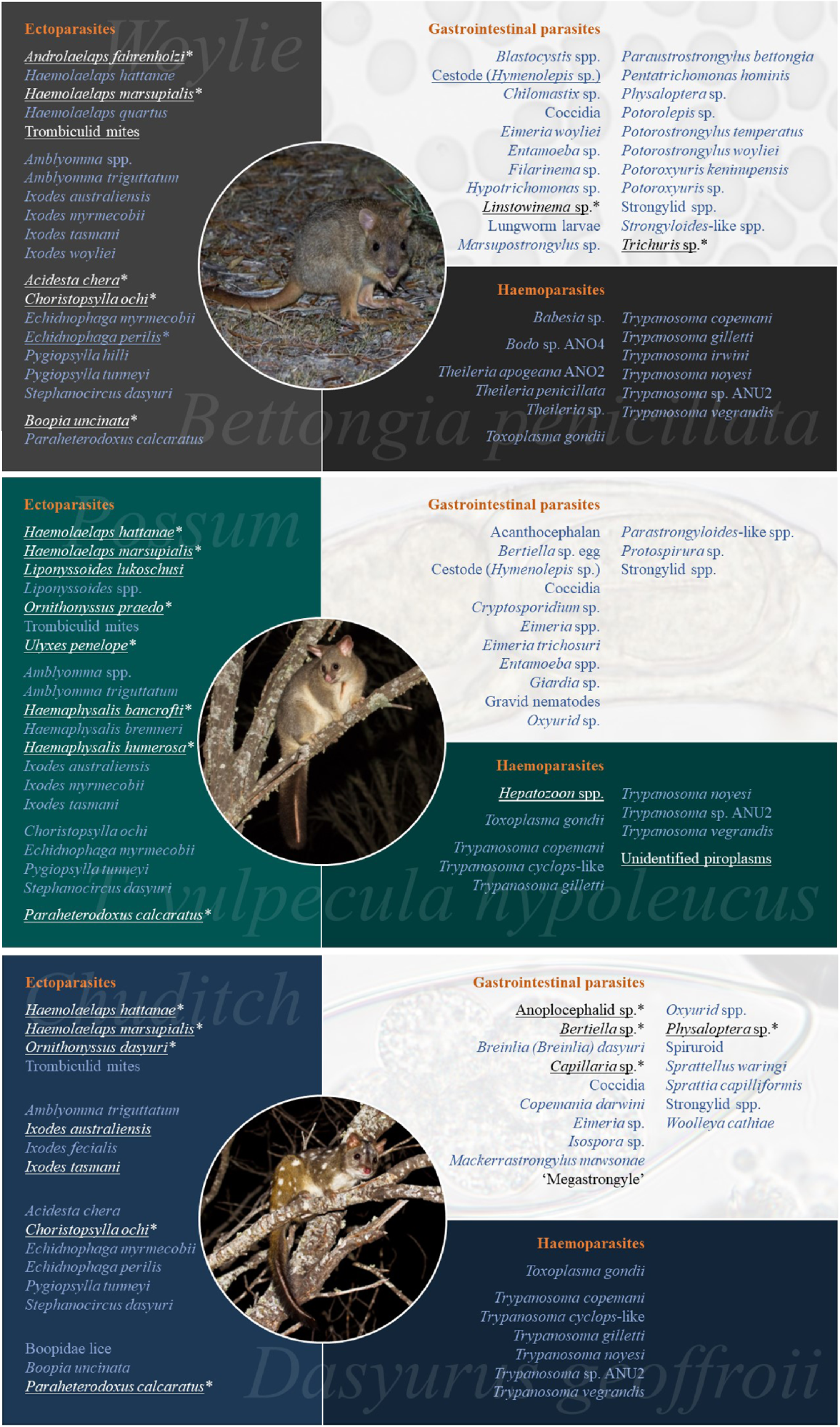
Differences in parasite species diversity and composition among sites and host species were apparent. Although some parasites were host-specific (e.g. E. woyliei, P. hilli and I. woyliei), many were generalists (e.g. Trypanosoma spp. and most ticks), though species-level identification could not be achieved for all parasite taxa. With increasing recognition of the intrinsic biodiversity value of parasites (Colwell et al. 2012; Thompson et al. 2018), the potential loss of parasite taxa should be a serious consideration when planning fauna translocations, particularly for host-specific parasites that are likely to be endangered themselves (Colwell et al. 2012). For I. woyliei, a tick with a high predilection for its critically endangered host (Ash et al. 2017), the risk of extinction following translocation is likely to be higher than for generalist ectoparasites with multiple host species (e.g. Amblyomma spp. ticks: Waudby et al. 2007). Loss of specialist parasites may also lead to biotic homogenisation of parasite communities, enhancing the risk of disease emergence (Dharmarajan et al. 2021).
For all host species and parasite groups (i.e. blood-borne, gastrointestinal and ectoparasite taxa), prevalence at the individual level was higher than prevalence at the sample level, indicating some level of sampling bias. As each host is exposed to a variety of parasites over time, resulting in fluctuations in both infection status (as shown by repeat sampling) and our ability to detect infection (e.g. measurable parasitaemia: Northover et al. 2019a), we would expect individual prevalence to be higher than sample prevalence during long-term parasite monitoring. As the purpose of this study was to document the parasite diversity of marsupials from different sites, we did not evaluate parasite prevalence at the individual level beyond these broad parasite groupings. To better inform disease risk and the need for management intervention during translocations, more research is needed to evaluate temporal parasite dynamics (e.g. the effect of season, host/vector density or diversity) including the biological significance of parasites on individuals and host populations. The incorporation of molecular techniques (e.g. Davey et al. 2021) will also help to improve parasite detection rates and more accurately characterise polyparasitism within a host/population.
Haemoparasites
Host–haemoparasite associations not previously reported (Paparini et al. 2011; Botero et al. 2013; Thompson et al. 2014b; Cooper et al. 2018; Northover et al. 2019a) include Hepatozoon spp. and piroplasm infection in T. v. hypoleucus, and T. noyesi in T. rugosa. During this study, elongate intraerythrocytic Hepatozoon gamont’s were detected in red blood cells from three T. v. hypoleucus in Dryandra: the first record of Hepatozoon infection in Trichosurus vulpecula within Australia. Organisms were not observed ‘free’ in the plasma or within leucocytes. All three hosts were coinfected with trypanosomes and I. tasmani ticks were present on two hosts. In WA, Hepatozoon sp. have been identified from other native Australian marsupials, including Isoodon obesulus (Wicks et al. 2006), with similar descriptions of intraerythrocytic gamont’s attributed to Hepatozoon peramelis. Like reports in other marsupial species (Wicks et al. 2006; Barbosa 2017), there was no evidence of clinical disease associated with Hepatozoon infection in T. v. hypoleucus during this study. Molecular sequencing methods developed for Hepatozoon spp. (e.g. amplification of 18S rRNA gene fragments: Barbosa 2017) are recommended to confirm the species identity.
Unidentified piroplasms were also detected within erythrocytes from T. v. hypoleucus during this study. This is the first report of piroplasm infection in T. vulpecula from WA, although piroplasms, including Babesia and Theileria spp., have been reported from several other marsupials in WA (Clark and Spencer 2007; Paparini et al. 2012; Rong et al. 2012; Northover et al. 2019a). Babesia lohae-like sequences have been identified from T. vulpecula in Sydney and New South Wales (NSW) (Egan et al. 2021) and also from Ixodes holocyclus and I. tasmani ticks parasitising T. vulpecula in NSW and Queensland (Qld) (Loh et al. 2018). As above, the incorporation of molecular techniques (e.g. Flaherty et al. 2021) would help to determine the species of piroplasm infecting T. v. hypoleucus in the current study.
Detection of T. noyesi infecting T. rugosa is the first record of a trypanosome isolated from a native reptile in WA. Other Trypanosoma spp. have been documented in reptiles from SA, NSW and Qld (Johnston 1907; Mackerras 1961; Jakes et al. 2001), but reptilian taxa are highly underrepresented in parasitological studies (Cooper et al. 2017). Although knowledge of the transmission of Australian trypanosomes is limited (Thompson et al. 2014b), ticks have been implicated as vectors in mammals (McInnes et al. 2009; Austen et al. 2011; McInnes et al. 2011; Barbosa et al. 2017; Krige et al. 2021a) and T. noyesi DNA has been detected within several (Amblyomma and Ixodes) species of questing tick (Krige et al. 2021b). Amblyomma albolimbatum ticks were present on one of the T. noyesi-infected T. rugosa during this study. Although A. albolimbatum ticks are generally considered host specific, more generalist species such as A. triguttatum, the ornate kangaroo tick, and ixodid ticks were present on marsupials infected with trypanosomes, though several Haemaphysalis spp. ticks were also present on Trypanosoma-positive T. v. hypoleucus.
The very low seroprevalence of T. gondii in B. penicillata (1.8%) is consistent with previous studies that used similar diagnostic methods (e.g. Parameswaran 2008; Skogvold et al. 2017). Antemortem diagnosis of T. gondii, however, is difficult, given the often acute-onset and short-lived serological response to disease (Portas 2019). Studies that used more sensitive and specific diagnostic methods to analyse tissue samples from deceased specimens (e.g. PCR: Parameswaran et al. 2010) have reported a much higher prevalence of infection (50.0%) in B. penicillata. Clinical disease was not evident in T. gondii-infected hosts during this study.
For all marsupial species, Trypanosoma species diversity was greatest within the UWR and lowest within Dryandra. Interestingly, a much higher prevalence of Trypanosoma spp. infection was detected in T. v. hypoleucus (82.6% overall) compared to other species (54.0% B. penicillata; 11.8% D. geoffroii). Within Warrup East, at least one Trypanosoma spp. was detected in every T. v. hypoleucus sampled (n = 41). In contrast, trypanosome infections were comparatively rare in D. geoffroii: only one of the 54 samples from Dryandra was Trypanosoma-positive. The prevalence of coinfection, however, was highest in D. geoffroii (46.2%) compared to T. v. hypoleucus (26.0%) or B. penicillata (23.1%). Species differences were also apparent, with T. copemani most prevalent in T. v. hypoleucus, and T. vegrandis most prevalent in B. penicillata. Both T. copemani and T. noyesi were equally most prevalent in D. geoffroii. Species differences may reflect different modes, and vectors, by which trypanosomes are transmitted.
Gastrointestinal parasites
To the author’s knowledge this is the first published record of Trichuris sp. (Nematoda: Trichuridae) and Linstowinema sp. (Nematoda: Seuratidae) in B. penicillata, and anoplocephalid (including Bertiella sp.) (Platyhelminthes: Anoplocephalidae), Capillaria sp. (Nematoda: Capillariidae) and Physaloptera sp. (Nematoda: Physalopteridae) in D. geoffroii. For B. penicillata, the low prevalence of these parasites warrants caution. For example, Linstowinema spp. are known to infect other sympatric marsupials (e.g. I. obesulus: Hillman 2016) in WA, which may have been captured during the same trapping session; thus, sample contamination cannot be ruled out. While it is important to interpret the results of faecal flotation with caution in carnivorous species such as D. geoffroii, as parasite eggs/oocysts from prey may not represent a true parasite infection, the morphological similarity of anoplocephalid eggs from multiple hosts in this study may represent a true/real primary infection. However, as there is no record of Anoplocephalidae (or Oxyuridae) infection in carnivorous hosts (Spratt and Beveridge 2016), pseudoinfection from the ingestion of infected prey is most probable. The presence of a Physaloptera sp. egg and Capillaria sp. egg in a single D. geoffroii from Warrup East and Walcott, respectively, is more difficult to interpret. Northern (Dasyurus hallucatus) and spotted-tailed (Dasyurus maculatus) quolls are known hosts of Physaloptera sp. nematodes (Spratt and Beveridge 2016); however, no Physaloptera sp. have been documented in D. geoffroii (western quoll) until now. Likewise, there are no published records of Capillaria sp. in D. geoffroii, though Capillaria ornamentata is known to infect other dasyurids (Spratt 2006) and a Eucoleus sp. was isolated from D. hallucatus (Oakwood and Spratt 2000).
An undescribed species of cestode egg was isolated from B. penicillata in Dryandra only. To date, Rodentolepsis fraterna (Spratt and Beveridge 2016) is the only species of cestode (Platyhelminthes: Hymenolepididae) formally described in B. penicillata, and the eggs of R. fraterna are morphologically different from those observed here. Work is being undertaken to characterise this putative new species, which has been isolated from the faeces of B. penicillata (Northover et al. 2019b) and T. v. hypoleucus (A. Elliot pers. comm.) previously. The absence of cestode eggs from all other sites suggests that the intermediate host for this parasite may be endemic to Dryandra.
Overall, all host species had a high prevalence of infection (B. penicillata 90.8%; D. geoffroii 88.4%; T. v. hypoleucus 74.4%). All I. fusciventer samples were positive for at least one type of parasite; though sample size was small (n = 12) and biased to the UWR. Nematodes, particularly strongyle eggs, were most prevalent, followed by coccidia; both were ubiquitous across target host species and site. The overall prevalence of coccidia infection in wild I. fusciventer (50.0%, n = 12) was considerably lower than has been reported in urban I. obesulus (96.7%, n = 123: Hillman 2016), though it is difficult to compare free-ranging and urbanised populations due to small sample size here.
Strongyle eggs (Nematoda: Strongylidae) likely represent several nematode species; however, we have limited information regarding the species of nematode infecting marsupials in WA (Spratt and Beveridge 2016) as few have been formally described (Baylis 1934; Johnston and Mawson 1939; Inglis 1968; Mawson 1973; Smales 2005; Beveridge and Durette-Desset 2009; Hobbs and Elliot 2016). Currently, Potorostrongylus woyliei (Smales 2005) and Paraustrostrongylus bettongia (Mawson 1973) have been documented in B. penicillata. Mackerrastrongylus mawsonae is the only strongylid nematode described in I. obesulus from WA (Inglis 1968). Four species of trichostrongylid nematode [Sprattellus waringi, Woolleya cathiae, Mackerrastrongylus mawsonae (Inglis 1968) and Copemania darwini (Beveridge and Durette-Desset 2009)] have been reported in D. geoffroii.
Based on current published data, the Strongyloides-like and Trichuris sp. eggs isolated from I. fusciventer may be those of Parastrongyloides australis and Trichuris peramelis, respectively, though neither species has been documented from I. obesulus in WA (Mawson 1960). Protospirura (Nematoda: Spiruridae), Parastrongyloides (Nematoda: Strongyloididae) and Bertiella spp. eggs have been detected in T. vulpecula from WA (Clark 2011); however, Protospirura marsupialis, Parastrongyloides trichosuri and Bertiella trichosuri are the only formally described species from T. vulpecula outside of WA (Mackerras 1959; Viggers and Spratt 1995; Spratt 2018). The majority of unsporulated coccidian oocysts isolated from T. v. hypoleucus were morphologically consistent with Eimeria trichosuri (O’Callaghan and O’Donoghue 2001; Power et al. 2009). The Potoroxyuris sp. eggs isolated from B. penicillata are most likely Potoroxyuris keninupensis (Nematoda: Oxiuridae) given their UWR origin, size, and characteristic mammilated outer shell covering (Hobbs and Elliot 2016). The incorporation of molecular techniques (e.g. sequencing of DNA extracted from faeces: Davey et al. 2021) would help to develop/refine existing DNA barcode libraries and provide a more detailed understanding (i.e. species level identification) of the gastrointestinal parasite taxa present.
As described for haemoparasites, a lower prevalence and diversity of gastrointestinal parasite taxa were present in samples from Dryandra, which may reflect host (e.g. density) and/or environmental (e.g. climate) differences. Strongyle eggs, for example, were detected in 32.2–69.2% of samples from Dryandra compared with 91.3–100% of samples from the UWR. In T. v. hypoleucus, Parastrongyloides eggs were not detected in Dryandra (n = 172), despite their high prevalence elsewhere. Higher rainfall within the UWR may facilitate the persistence of eggs within the environment and thus the completion of helminth life-cycles. In contrast, the prevalence of coccidia in T. v. hypoleucus was highest in Dryandra, which may be related to host density [T. v. hypoleucus capture rates = 0.17 Dryandra; 0.10 Warrup East; and 0.04 Walcott]; though differences in the timing of sampling also may have influenced these results [i.e. additional trapping conducted during winter (June and August) in Dryandra].
Ectoparasites
Host–ectoparasite associations not previously reported in B. penicillata (von Kéler 1971; Dunnet and Mardon 1974; Domrow 1987; Kaewmongkol et al. 2011; Ash et al. 2017; Thompson et al. 2018; Krige et al. 2021a) include A. chera, C. ochi, B. uncinata, H. marsupialis, A. fahrenholzi and trombiculid mites. Pygiopsylla tunneyi was the most common flea species identified on B. penicillata during this study. In contrast, B. penicillata may be an accidental host for A. chera, C. ochi and H. marsupialis as the nominal host [I. obesulus for A. chera (Dunnet and Mardon 1974) and H. marsupialis (Domrow 1987); and T. vulpecula for C. ochi (Dunnet and Mardon 1974)] was captured beforehand, making bag contamination a possibility. The presence of A. fahrenholzi on a single host from Warrup East is intriguing, as birds or their nests are the only known hosts within (south-eastern) Australia (Domrow 1987). Outside of Australia A. fahrenholzi is known to parasitise a wide range of hosts, in particular rodents (Silva-de la Fuente et al. 2020), but there is no record of this parasite in WA until now. Dasyurus species are the predominant host of B. uncinata (von Kéler 1971); however, we did not detect B. uncinata on D. geoffroii in Dryandra, and only B. penicillata were captured on the day B. uncinata was detected. While this is the first published record of trombiculid (larval stage) mites on B. penicillata, specimens were not obtained to confirm the species.
Stark site variation in parasite type and prevalence were also apparent. For example, I. australiensis and P. tunneyi were not detected on B. penicillata from Dryandra, despite these species being the most prevalent overall within their respective taxon groups. Ixodes australiensis was not found on sympatric species within Dryandra either, and P. tunneyi was only detected on a single host (T. v. hypoleucus) from Dryandra. In contrast, the host-specific tick I. woyliei was most prevalent within Dryandra, which is unexpected given the lack of I. australiensis and low prevalence of I. tasmani (n = 1), as these three species are considered sympatric, sharing the same geographical region, habitat and host (Ash et al. 2017). Fleas were also extremely rare (excluding C. ochi on T. v. hypoleucus and E. myrmecobii on D. geoffroii) within Dryandra. Encouragingly, the threatened host-specific flea, P. hilli (Kwak 2018), is persisting in all UWR sites. The critically endangered status and current reliance of B. penicillata upon periodic translocations, however, places this density dependent and site-specific coendangered parasite at risk of extinction.
Host–ectoparasite associations not previously reported in T. vulpecula (Roberts 1970; Dunnet and Mardon 1974; Viggers and Spratt 1995; Burmej et al. 2008; Clark 2011; Hillman 2016; Krige et al. 2021a) include P. calcaratus, H. hattanae, H. marsupialis, O. praedo and U. penelope. Additionally, this is the first report of P. tunneyi, H. bancrofti, H. bremneri, H. humerosa, L. lukoschusi and trombiculid mites on T. v. hypoleucus from south-western WA.) While H. bremneri (and another Haemaphysalis spp. tick) have been isolated from a T. vulpecula in the greater Perth region (Hillman 2016), H. humerosa and H. bancrofti have not been identified on T. vulpecula from WA (Roberts 1970). We identified H. humerosa on two hosts from Warrup East, one of which had two specimens. Previous studies (Roberts 1963) suggest that bandicoots are the primary host species for H. humerosa. Although the host with two H. humerosa ticks was captured after an I. fusciventer, the other host was not. We also found H. bancrofti (and H. bremneri) on T. v. hypoleucus from Dryandra. Haemaphysalis bancrofti has been reported from T. vulpecula in eastern Australia (Roberts 1963, 1970; Viggers and Spratt 1995; Laan et al. 2011), but this is the first report in WA.
The presence of P. calcaratus on T. v. hypoleucus (n = 2) from Walcott is unusual, as no lice have been recorded on T. vulpecula (Johnson and Hemsley 2008). While it is likely that T. v. hypoleucus is an accidental host (i.e. P. calcaratus-positive B. penicillata captured beforehand in one instance), we cannot rule out T. v. hypoleucus being an alternative host; particularly when lice (unidentified species) were recorded on a further six hosts in the field (Appendix Table 9).
The presence of six mite taxa is noteworthy. Haemolaelaps hattanae was found on four hosts (from all sites), though its presence could be attributed to bag contamination in two cases. The presence of two Haemolaelaps mite species and U. penelope (previously Haemolaelaps penelope; Shaw 2014), however, may indicate that T. v. hypoleucus is an alternative host. Haemolaelaps sisyphus has been recorded from T. vulpecula in NSW and Qld (Viggers and Spratt 1995). Ornithonyssus praedo has only been documented on other marsupials (Domrow 1987), and birds are the recognised host of L. lukoschusi (Domrow 1987). As we identified L. lukoschusi on six hosts from Dryandra, and Hillman et al. (2018) found a Liponyssoides sp. mite on T. vulpecula (n = 1) from the greater Perth region, T. v. hypoleucus may be an alternative host, though its arboreal nature may expose it to such parasites. The presence of O. praedo on a single host is more difficult to interpret, though no known hosts (i.e. P. tapoatafa: Domrow 1987) were captured during this trapping session.
As for B. penicillata, site differences in ectoparasite prevalence and diversity were evident. The prevalence of Ixodes spp. ticks was lowest within Dryandra; neither I. australiensis nor I. myrmecobii were detected on T. v. hypoleucus within this site. In contrast, L. lukoschusi, H. bancrofti and H. bremneri were detected only on T. v. hypoleucus within Dryandra, and the prevalence of C. ochi, trombiculid mites and Amblyomma spp. ticks was highest within this site. The majority of Haemolaelaps spp. mites (5/7) were isolated from T. v. hypoleucus in Walcott (as was S. dasyuri), whereas O. praedo was found only in Warrup East. Amblyomma spp. ticks, I. tasmani, C. ochi, P. tunneyi and H. hattanae were ubiquitous across sites.
Host–ectoparasite associations not previously reported in D. geoffroii (von Kéler 1971; Haigh 1994; Burmej et al. 2008; Thomasz 2014) include I. australiensis, I. tasmani, C. ochi, P. calcaratus, H. hattanae, H. marsupialis and O. dasyuri. Ixodes australiensis was detected on 17 animals and had the highest prevalence of any tick species, indicating that D. geoffroii is a true host. Likewise, the presence of I. tasmani on D. geoffroii across all sites suggests a legitimate host–parasite association. In contrast, the presence of C. ochi in a single host from Warrup East is questionable. Choristopsylla ochi is commonly found in arboreal species such as T. vulpecula (Dunnet and Mardon 1974) and although D. geoffroii may be an accidental host, its semiarboreal nature may expose it to such parasites. Bettongia penicillata was the only other animal captured before this host (using the same handling bag) on that day. Paraheterodoxus calcaratus has not been recorded in D. geoffroii. We believe D. geoffroii is an accidental host of P. calcaratus as two of the three hosts detected with P. calcaratus were captured immediately after B. penicillata infected with P. calcaratus using the same handling bag. The presence of four mite species was surprising. Although we detected a low prevalence of H. hattanae, H. marsupialis and O. dasyuri, O. dasyuri has been found on the eastern quoll (Dasyurus viverrinus) (Domrow 1987) and both Haemolaelaps spp. were found on D. geoffroii at multiple (UWR) sites. Trombiculid mites had the highest prevalence of all mite taxa and were observed on D. geoffroii at all sites during this study.
As for other host species, site variation in parasite prevalence and diversity was identified. For instance, nine parasite taxa that were present on D. geoffroii from Warrup East and Walcott were not detected on D. geoffroii within Dryandra. This includes I. australiensis, P. tunneyi and B. uncinata, which were the most prevalent species identified within their respective ectoparasite taxa groups. The opposite trend was observed for trombiculid mites and E. myrmecobii, which had the highest prevalence within Dryandra; O. dasyuri (n = 1) was detected only within this site. In comparison to B. penicillata and T. v. hypoleucus, in which ticks were the most prevalent ectoparasite found, mites were most prevalent on D. geoffroii.
Ticks collected from T. rugosa were identified as A. albolimbatum on six hosts, and Amblyomma sp. in the other host. Tiliqua rugosa is the primary host of A. albolimbatum (Sharrad and King 1981; Barker and Walker 2014). The two flea species found on I. fusciventer (S. dasyuri and P. tunneyi) have been previously documented on I. obesulus from WA (Dunnet and Mardon 1974; Hillman 2016). In contrast, this is the first report of I. australiensis and Amblyomma sp. ticks from P. t. wambenger. Phascogale tapoatafa wambenger is a known host of other Ixodes spp. ticks (Roberts 1970) and Amblyomma spp. ticks have a wide host range, including domestic animals and humans (Roberts 1970; Waudby et al. 2007). As we only collected a larval Amblyomma specimen from this host, we were unable to identify the species.
For all host species, many of the ectoparasites collected were larval stages of ticks and mites, which could not be identified to species using morphological keys. Unidentified ticks had the highest prevalence within their taxonomic group. Molecular techniques could be used in future to determine the species of ectoparasite and provide more accurate prevalence data. Introducing measures to reduce the risk of cross contamination will also help to reduce the likelihood of spurious host–ectoparasite associations. For example, single use handling bags or providing handling bags and equipment for each species.
Conclusion
We identified a diverse parasite community within WA’s native fauna including several new host–parasite records. This study improves our knowledge of parasites infecting terrestrial wildlife within Australia’s south-west and highlights the importance of monitoring both target and sympatric species when compiling baseline data of parasite fauna present within translocation sites. Further research to identify differences between host species over space and time, and factors driving these patterns/differences is recommended. A better understanding of the life cycle, transmission and potential effects of parasite infection on host health and populations (including the need for antiparasitic drugs), will help to provide an informed framework for the management of threatened species and their parasite taxa to minimise disease risk and improve translocation outcomes. Host-dependent threatened parasites, in particular, require careful deliberation in terms of their disease risk (if any) and benefits of preservation. Sampling in a manner that minimises contamination will help to reduce anthropogenic cross infections and improve confidence of host–parasite associations. Likewise, the incorporation of molecular sequencing techniques will help to evaluate parasite species diversity more accurately (e.g. identifying larval stages of ectoparasites or gastrointestinal species). Screening of other host species that serve as potential disease reservoirs (e.g. introduced species and livestock) and the inclusion of other parasite taxa (e.g. viruses) should also be considered.
Data availability
Data will be shared upon reasonable request to the corresponding author with permission from the Department of Biodiversity, Conservation and Attractions.
Declaration of funding
This research was principally funded by the Australian Research Council (LP130101073) but has also been supported by grants from the Holsworth Wildlife Research Endowment (HOLSW2015-1-F149) and the Ecological Society of Australia, The Royal Zoological Society of New South Wales (Paddy Pallin Grant) and the Australian Wildlife Society.
Acknowledgements
We thank Marika Maxwell, Colin Ward, Chris Vellios, Julia Wayne, Peter Wnuk, Malcolm Ovans, Brian Macmahon and other DBCA staff for their assistance in the field, and all our dedicated volunteers for their support with fieldwork, sample collection and processing. We express our gratitude to John Lawson from Dryandra Woodland, for his guidance and assistance in the field and provision of photographs. We thank Adriana Botero, Louise Pallant and Crystal Cooper for their technical guidance and laboratory support; Aileen Elliot for assisting with the morphological identification of endo- and ectoparasites, and the provision of advice regarding the development of a faecal flotation protocol; and Marika Maxwell for producing the maps.
References
Ash A, Elliot A, Godfrey S, Burmej H, Abdad MY, Northover A, Wayne A, Morris K, Clode P, Lymbery A, Thompson RCA (2017) Morphological and molecular description of Ixodes woyliei n. sp. (Ixodidae) with consideration for co-extinction with its critically endangered marsupial host. Parasites & Vectors 10, 70.
| Crossref | Google Scholar | PubMed |
Austen JM, Ryan UM, Friend JA, Ditcham WGF, Reid SA (2011) Vector of Trypanosoma copemani identified as Ixodes sp. Parasitology 138, 866-872.
| Crossref | Google Scholar | PubMed |
Barbosa AD, Gofton AW, Paparini A, Codello A, Greay T, Gillett A, Warren K, Irwin P, Ryan U (2017) Increased genetic diversity and prevalence of co-infection with Trypanosoma spp. in koalas (Phascolarctos cinereus) and their ticks identified using next-generation sequencing (NGS). PLoS ONE 12, e0181279.
| Crossref | Google Scholar | PubMed |
Barker SC, Walker AR (2014) Ticks of Australia. The species that infest domestic animals and humans. Zootaxa 3816, 1-144.
| Crossref | Google Scholar |
Baylis HA (1934) LI.—On two filariid parasites of marsupials from Queensland. Annals and Magazine of Natural History 13, 549-554.
| Crossref | Google Scholar |
Beveridge I, Durette-Desset M-C (2009) A new species of the nematode genus Copemania (Nematoda: Trichostrongylida), parasitic in the western quoll, Dasyurus geoffroii and short-nosed bandicoot, Isoodon obesulus from south-western Western Australia. Records of the Western Australian Museum 25, 345-349.
| Crossref | Google Scholar |
Botero A, Thompson CK, Peacock CS, Clode PL, Nicholls PK, Wayne AF, Lymbery AJ, Thompson RCA (2013) Trypanosomes genetic diversity, polyparasitism and the population decline of the critically endangered Australian marsupial, the brush tailed bettong or woylie (Bettongia penicillata). International Journal for Parasitology: Parasites and Wildlife 2, 77-89.
| Crossref | Google Scholar | PubMed |
Boyce WM, Weisenberger ME, Penedo MCT, Johnson CK (2011) Wildlife translocation: the conservation implications of pathogen exposure and genetic heterozygosity. BMC Ecology 11, 5.
| Crossref | Google Scholar | PubMed |
Brown LD, Cai TT, DasGupta A (2001) Interval estimation for a binomial proportion. Statistical Science 16, 101-133.
| Crossref | Google Scholar |
Clark P, Spencer PBS (2007) Description of three new species of Theileria Bettencourt, Franca & Borges, 1907 from Macropodoidea in Western Australia. Transactions of the Royal Society of South Australia 131, 100-106.
| Crossref | Google Scholar |
Colwell RK, Dunn RR, Harris NC (2012) Coextinction and persistence of dependent species in a changing world. Annual Review of Ecology, Evolution, and Systematics 43, 183-203.
| Crossref | Google Scholar |
Cooper C, Clode PL, Peacock C, Thompson RCA (2017) Host–parasite relationships and life histories of trypanosomes in Australia. Advances in Parasitology 97, 47-109.
| Crossref | Google Scholar | PubMed |
Cooper C, Keatley S, Northover A, Gofton AW, Brigg F, Lymbery AJ, Pallant L, Clode PL, Thompson RCA (2018) Next generation sequencing reveals widespread trypanosome diversity and polyparasitism in marsupials from Western Australia. International Journal for Parasitology: Parasites and Wildlife 7, 58-67.
| Crossref | Google Scholar | PubMed |
Couzineau P, Baufine-Ducrocq H (1970) Direct agglutination of Toxoplasma. Preparation of the antigen and examination f 400 serus. Annales de biologie Clinique (Paris) 28, 411-415.
| Google Scholar |
Cunningham AA (1996) Disease risks of wildlife translocations. Conservation Biology 10, 349-353.
| Crossref | Google Scholar |
Davey ML, Utaaker KS, Fossøy F (2021) Characterizing parasitic nematode faunas in faeces and soil using DNA metabarcoding. Parasites & Vectors 14, 422.
| Crossref | Google Scholar | PubMed |
De Castro F, Bolker B (2005) Mechanisms of disease-induced extinction. Ecology Letters 8, 117-126.
| Crossref | Google Scholar |
Dharmarajan G, Gupta P, Vishnudas CK, Robin VV (2021) Anthropogenic disturbance favours generalist over specialist parasites in bird communities: implications for risk of disease emergence. Ecology Letters 24, 1859-1868.
| Crossref | Google Scholar | PubMed |
Domrow R (1987) Acari Mesostigmata parasitic on Australian vertebrates: an annotated checklist, keys and bibliography. Invertebrate Systematics 1, 817-948.
| Crossref | Google Scholar |
Dougherty ER, Carlson CJ, Bueno VM, Burgio KR, Cizauskas CA, Clements CF, Seidel DP, Harris NC (2016) Paradigms for parasite conservation. Conservation Biology 30, 724-733.
| Crossref | Google Scholar | PubMed |
Dunlop JA, Watson MJ (2022) The hitchhiker’s guide to Australian conservation: a parasitological perspective on fauna translocations. Austral Ecology 47, 748-764.
| Crossref | Google Scholar |
Dunnet GM, Mardon DK (1974) A monograph of Australian fleas (Siphonaptera). Australian Journal of Zoology 22, 1-273.
| Crossref | Google Scholar |
Egan SL, Taylor CL, Austen JM, Banks PB, Northover AS, Ahlstrom LA, Ryan UM, Irwin PJ, Oskam CL (2021) Haemoprotozoan surveillance in peri-urban native and introduced wildlife from Australia. Current Research in Parasitology & Vector-Born Diseases 1, 100052.
| Crossref | Google Scholar |
Flaherty BR, Barratt J, Lane M, Talundzic E, Bradbury RS (2021) Sensitive universal detection of blood parasites by selective pathogen-DNA enrichment and deep amplicon sequencing. Microbiome 9, 1.
| Crossref | Google Scholar | PubMed |
Godfrey SS, Keatley S, Botero A, Thompson CK, Wayne AF, Lymbery AJ, Morris K, Thompson RCA (2018) Trypanosome co-infections increase in a declining marsupial population. International Journal for Parasitology: Parasites and Wildlife 7, 221-227.
| Crossref | Google Scholar | PubMed |
Haigh SA, Gaynor WT, Morris KD (1994) A health monitoring program for captive, wild and translocated chuditch (Dasyurus geoffroii). In ‘Proceedings of the 1994 Conference of the Australian Association of Veterinary Conservation Biologists, Canberra’. pp. 52–56. (Australian Association of Veterinary Conservation Biologists)
Hillman AE, Lymbery AJ, Elliot AD, Ash AL, Thompson RCA (2018) Parasitic infections of brushtail possums Trichosurus vulpecula in urbanised environments and bushland in the greater Perth region, Western Australia. Wildlife Biology 2018, 1-7.
| Crossref | Google Scholar |
Hing S, Northover AS, Narayan EJ, Wayne AF, Jones KL, Keatley S, Thompson RCA, Godfrey SS (2017) Evaluating stress physiology and parasite infection parameters in the translocation of critically endangered woylies (Bettongia penicillata). EcoHealth 14, 128-138.
| Crossref | Google Scholar | PubMed |
Hobbs RP, Elliot AD (2016) A new species of Potoroxyuris (Nematoda: Oxyuridae) from the woylie Bettongia penicillata (Marsupialia: Potoroidae) from southwestern Australia. International Journal for Parasitology: Parasites and Wildlife 5, 211-216.
| Crossref | Google Scholar | PubMed |
Hudson PJ, Dobson AP, Lafferty KD (2006) Is a healthy ecosystem one that is rich in parasites? Trends in Ecology & Evolution 21, 381-385.
| Crossref | Google Scholar | PubMed |
Inglis WG (1968) The geographical and evolutionary relationships of Australian trichostrongyloid parasites and their hosts. Zoological Journal of the Linnean Society 47, 327-347.
| Crossref | Google Scholar |
Jakes KA, O’Donoghue PJ, Adlard RD (2001) Phylogenetic relationships of Trypanosoma chelodina and Trypanosoma binneyi from Australian tortoises and platypuses inferred from small subunit rRNA analyses. Parasitology 123, 483-487.
| Crossref | Google Scholar | PubMed |
Johnston TH (1907) A trypanosome found in the river Murray turtle (Chelodina longicollis). The Australasian Medical Gazette 26, 26.
| Google Scholar |
Johnston TH, Mawson PM (1939) Some nematodes from Victorian and Western Australian marsupials. Transactions of the Royal Society of South Australia 63, 307-310.
| Google Scholar |
Kaewmongkol G, Kaewmongkol S, McInnes LM, Burmej H, Bennett MD, Adams PJ, Ryan U, Irwin PJ, Fenwick SG (2011) Genetic characterization of flea-derived Bartonella species from native animals in Australia suggests host–parasite co-evolution. Infection, Genetics and Evolution 11, 1868-1872.
| Crossref | Google Scholar | PubMed |
Krige A-S, Thompson RCA, Seidlitz A, Keatley S, Wayne J, Clode PL (2021a) Molecular detection of Trypanosoma spp. in questing and feeding ticks (Ixodidae) collected from an endemic region of south-west Australia. Pathogens 10, 1037.
| Crossref | Google Scholar | PubMed |
Krige A-S, Thompson RCA, Seidlitz A, Keatley S, Botero A, Clode PL (2021b) ‘Hook, line, and sinker’: fluorescence in situ hybridisation (FISH) uncovers Trypanosoma noyesi in Australian questing ticks. Ticks and Tick-borne Diseases 12, 101596.
| Crossref | Google Scholar | PubMed |
Kwak ML (2018) Australia’s vanishing fleas (Insecta: Siphonaptera): a case study in methods for the assessment and conservation of threatened flea species. Journal of Insect Conservation 22, 545-550.
| Crossref | Google Scholar |
Laan B, Handasyde K, Beveridge I (2011) Occurrence of the tick Haemaphysalis bancrofti Nuttall & Warburton, 1915 in Victoria with additional data on its distribution and with scanning electron micrographs of life cycle stages. Proceedings of the Royal Society of Victoria 123, 189-199.
| Google Scholar |
Leifels M, Khalilur Rahman O, Sam I-C, Cheng D, Chua FJD, Nainani D, Kim SY, Ng WJ, Kwok WC, Sirikanchana K, Wuertz S, Thompson J, Chan YF (2022) The one health perspective to improve environmental surveillance of zoonotic viruses: lessons from COVID-19 and outlook beyond. ISME Communications 2, 107.
| Crossref | Google Scholar | PubMed |
Loh S-M, Egan S, Gillett A, Banks PB, Ryan UM, Irwin PJ, Oskam CL (2018) Molecular surveillance of piroplasms in ticks from small and medium-sized urban and peri-urban mammals in Australia. International Journal for Parasitology: Parasites and Wildlife 7, 197-203.
| Crossref | Google Scholar | PubMed |
Lymbery AJ, Smit NJ (2023) Conservation of parasites: a primer. International Journal for Parasitology: Parasites and Wildlife 21, 255-263.
| Crossref | Google Scholar | PubMed |
Mackerras MJ (1959) Strongyloides and Parastrongyloides (Nematoda: Rhabdiasoidea) in Australian Marsupials. Australian Journal of Zoology 7, 87-104.
| Crossref | Google Scholar |
Mackerras MJ (1961) The haematozoa of Australian reptiles. Australian Journal of Zoology 9, 61-122.
| Crossref | Google Scholar |
Mawson PM (1960) Nematodes belonging to the Trichostrongylidae, Subuluridae, Rhabdiasidae, and Trichuridae from bandicoots. Australian Journal of Zoology 8, 261-284.
| Crossref | Google Scholar |
Mawson PM (1973) Amidostomatinae (Nematoda: Trichostrongyloidea) from Australian marsupials and monotremes. Transactions of the Royal Society of South Australia 97, 257-279.
| Google Scholar |
McCallum H (2012) Disease and the dynamics of extinction. Philosophical Transactions of the Royal Society B: Biological Sciences 367, 2828-2839.
| Crossref | Google Scholar | PubMed |
McGill I, Feltrer Y, Jeffs C, Sayers G, Marshall RN, Peirce MA, Stidworthy MF, Pocknell A, Sainsbury AW (2010) Isosporoid coccidiosis in translocated cirl buntings (Emberiza cirlus). Veterinary Record 167, 656-660.
| Crossref | Google Scholar | PubMed |
McInnes LM, Gillett A, Ryan UM, Austen J, Campbell RSF, Hanger J, Reid SA (2009) Trypanosoma irwini n. sp (Sarcomastigophora: Trypanosomatidae) from the koala (Phascolarctos cinereus). Parasitology 136, 875-885.
| Crossref | Google Scholar | PubMed |
McInnes LM, Gillett A, Hanger J, Reid SA, Ryan UM (2011) The potential impact of native Australian trypanosome infections on the health of koalas (Phascolarctos cinereus). Parasitology 138, 873-883.
| Crossref | Google Scholar |
Moir ML, Vest PA, Brennan KEC, Poulin R, Hughes L, Keith DA, McCarthy MA, Coates DJ (2012) Considering extinction of dependent species during translocation, ex situ conservation, and assisted migration of threatened hosts. Conservation Biology 26, 199-207.
| Crossref | Google Scholar | PubMed |
Morris K, Page M, Kay R, Renwick J, Desmond A, Comer S, Burbidge A, Kuchling G, Sims C (2015) Forty years of fauna translocations in Western Australia: lessons learned. In ‘Advances in Reintroduction Biology of Australian and New Zealand Fauna’. (Eds DP Armstrong, MW Hayward, D Moro, PJ Seddon) pp. 217–235. (CSIRO Publishing: Clayton South, VIC)
Moseby K, Carthey A, Schroeder T (2015) The influence of predators and prey naivety on reintroduction success: current and future directions. In ‘Advances in Reintroduction Biology of Australian and New Zealand Fauna’. (Eds DP Armstrong, MW Hayward, D Moro, PJ Seddon) pp. 29–42. (CSIRO Publishing: Clayton South, VIC)
National Environmental Science Program Threatened Species Research Hub (2019) Threatened species strategy year 3 Scorecard – Woylie. (Australian Government: Canberra) Available at http://www.environment.gov.au/biodiversity/threatened/species/20-mammals-by-2020/woylie [Accessed 1 February 2022]
Northover AS, Godfrey SS, Lymbery AJ, Morris K, Wayne AF, Thompson RCA (2017) Evaluating the effects of ivermectin treatment on communities of gastrointestinal parasites in translocated woylies (Bettongia penicillata). EcoHealth 14, 117-127.
| Crossref | Google Scholar | PubMed |
Northover AS, Lymbery AJ, Wayne AF, Godfrey SS, Thompson RCA (2018a) The hidden consequences of altering host–parasite relationships during fauna translocations. Biological Conservation 220, 140-148.
| Crossref | Google Scholar |
Northover AS, Elliot AD, Keatley S, Lim Z, Botero A, Ash A, Lymbery AJ, Wayne AF, Godfrey SS, Thompson RCA (2018b) Debilitating disease in a polyparasitised woylie (Bettongia penicillata): a diagnostic investigation. International Journal for Parasitology: Parasites and Wildlife 7, 274-279.
| Crossref | Google Scholar | PubMed |
Northover AS, Godfrey SS, Keatley S, Lymbery AJ, Wayne AF, Cooper C, Pallant L, Morris K, Thompson RCA (2019a) Increased Trypanosoma spp. richness and prevalence of haemoparasite co-infection following translocation. Parasites & Vectors 12, 126.
| Crossref | Google Scholar | PubMed |
Northover AS, Thompson RCA, Lymbery AJ, Wayne AF, Keatley S, Ash A, Elliot AD, Morris K, Godfrey SS (2019b) Altered parasite community structure in an endangered marsupial following translocation. International Journal for Parasitology: Parasites and Wildlife 10, 13-22.
| Crossref | Google Scholar | PubMed |
Northover AS, Keatley S, Elliot A, Hobbs RP, Yang R, Lymbery AJ, Godfrey SS, Wayne AF, Thompson RCA (2019c) Identification of a novel species of Eimeria Schneider, 1875 from the woylie, Bettongia penicillata Gray (Diprotodontia: Potoroidae) and the genetic characterisation of three Eimeria spp. from other potoroid marsupials. Systematic Parasitology 96, 553-563.
| Crossref | Google Scholar | PubMed |
Oakwood M, Spratt DM (2000) Parasites of the northern quoll, Dasyurus hallucatus (Marsupialia: Dasyuridae) in tropical savanna, Northern Territory. Australian Journal of Zoology 48, 79-90.
| Crossref | Google Scholar |
O’Callaghan MG, O’Donoghue PJ (2001) A new species of Eimeria (Apicomplexa: Eimeriidae) from the brushtail possum, Trichosurus vulpecula (Diprotodontia: Phalangeridae). Transactions of the Royal Society of South Australia 125, 129-132.
| Google Scholar |
Pacioni C, Johansen CA, Mahony TJ, O’Dea MA, Robertson ID, Wayne AF, Ellis T (2014) A virological investigation into declining woylie populations. Australian Journal of Zoology 61, 446-453.
| Crossref | Google Scholar |
Paparini A, Irwin PJ, Warren K, McInnes LM, de Tores P, Ryan UM (2011) Identification of novel trypanosome genotypes in native Australian marsupials. Veterinary Parasitology 183, 21-30.
| Crossref | Google Scholar | PubMed |
Paparini A, Ryan UM, Warren K, McInnes LM, de Tores P, Irwin PJ (2012) Identification of novel Babesia and Theileria genotypes in the endangered marsupials, the woylie (Bettongia penicillata ogilbyi) and boodie (Bettongia lesueur). Experimental Parasitology 131, 25-30.
| Crossref | Google Scholar | PubMed |
Parameswaran N, Thompson RCA, Sundar N, Pan S, Johnson M, Smith NC, Grigg ME (2010) Non-archetypal Type II-like and atypical strains of Toxoplasma gondii infecting marsupials of Australia. International Journal for Parasitology 40, 635-640.
| Crossref | Google Scholar | PubMed |
Pizzi R (2009) Veterinarians and taxonomic chauvinism: the dilemma of parasite conservation. Journal of Exotic Pet Medicine 18, 279-282.
| Crossref | Google Scholar |
Power ML, Richter C, Emery S, Hufschmid J, Gillings MR (2009) Eimeria trichosuri: phylogenetic position of a marsupial coccidium, based on 18S rDNA sequences. Experimental Parasitology 122, 165-168.
| Crossref | Google Scholar | PubMed |
Preston DL, Mischler JA, Townsend AR, Johnson PTJ (2016) Disease ecology meets ecosystem science. Ecosystems 19, 737-748.
| Crossref | Google Scholar |
Rideout BA, Sainsbury AW, Hudson PJ (2016) Which parasites should we be most concerned about in wildlife translocations? EcoHealth 14, 42-46.
| Crossref | Google Scholar | PubMed |
Roberts FHS (1963) A systematic study of the Australian species of the genus Haemaphysalis Koch (Acarina: Ixodidae). Australian Journal of Zoology 11, 35-80.
| Crossref | Google Scholar |
Roberts FSH (1970) Australian ticks. Commonwealth Scientific and Industrial Research Organisation, Melbourne. Available at https://doi.org/10.25919/2qs6-bv04 [Accessed 6 November 2023]
Rong J, Bunce M, Wayne A, Pacioni C, Ryan U, Irwin P (2012) A high prevalence of Theileria penicillata in woylies (Bettongia penicillata). Experimental Parasitology 131, 157-161.
| Crossref | Google Scholar | PubMed |
Scheele BC, Hollanders M, Hoffmann EP, Newell DA, Lindenmayer DB, McFadden M, Gilbert DJ, Grogan LF (2021) Conservation translocations for amphibian species threatened by chytrid fungus: a review, conceptual framework, and recommendations. Conservation Science and Practice 3, e524.
| Crossref | Google Scholar |
Sharrad RD, King DR (1981) The geographical distribution of reptile ticks in Western Australia. Australian Journal of Zoology 29, 861-873.
| Crossref | Google Scholar |
Shaw MD (2014) Ulyxes, a new Australopapuan mite genus associated with arboreal nests (Acari: Laelapidae). Zootaxa 3878, 261-290.
| Crossref | Google Scholar |
Silva-de la Fuente MC, Moreno Salas L, Casanueva ME, Lareschi M, González-Acuña D (2020) Morphometric variation of Androlaelaps fahrenholzi (Mesostigmata: Laelapidae) associated with three Sigmodontinae (Rodentia: Cricetidae) from the north of Chile. Experimental and Applied Acarology 81, 135-148.
| Crossref | Google Scholar | PubMed |
Skogvold K, Warren KS, Jackson B, Holyoake CS, Stalder K, Devlin JM, Vitali SD, Wayne AF, Legione A, Robertson I, Vaughan-Higgins RJ (2017) Infectious disease surveillance in the woylie (Bettongia penicillata). EcoHealth 14, 518-529.
| Crossref | Google Scholar | PubMed |
Smales LR (2005) Potorostrongylus woyliei n. sp. (Nematoda: Cloacinidae) from the brush-tailed bettong Bettongia penicillata (Marsupialia) from Western Australia, Australia, with comments on potoroid–potorostrongylid associations and a key to the species of Potorostrongylus. Comparative Parasitology 72, 28-32.
| Crossref | Google Scholar |
Smith A, Clark P, Averis S, Lymbery AJ, Wayne AF, Morris KD, Thompson RCA (2008) Trypanosomes in a declining species of threatened Australian marsupial, the brush-tailed bettong Bettongia penicillata (Marsupialia: Potoroidae). Parasitology 135, 1329-1335.
| Crossref | Google Scholar | PubMed |
Spratt DM (2006) Description of capillariid nematodes (Trichinelloidea: Capillariidae) parasitic in Australian marsupials and rodents. Zootaxa 1348, 1-82.
| Crossref | Google Scholar |
Spratt DM (2018) A review of species of Parastrongyloides (Nematoda: Rhabditoidea: Strongyloididae) from Australian marsupials with descriptions of three new species. Transactions of the Royal Society of South Australia 142, 162-182.
| Crossref | Google Scholar |
Spratt DM, Beveridge I (2016) Helminth parasites of Australasian monotremes and marsupials. Zootaxa 4123, 1-198.
| Crossref | Google Scholar | PubMed |
Start AN, Burbidge A, Armstrong D (1998) A review of the conservation status of the woylie, Bettongia penicillata Ogilbyi (Marsupialia: Potoroidae) using IUCN criteria. CALMscience 2, 277-289.
| Google Scholar |
Stringer AP, Linklater W (2014) Everything in moderation: principles of parasite control for wildlife conservation. BioScience 64, 932-937.
| Crossref | Google Scholar |
Telfer S, Lambin X, Birtles R, Beldomenico P, Burthe S, Paterson S, Begon M (2010) Species interactions in a parasite community drive infection risk in a wildlife population. Science 330, 243-246.
| Crossref | Google Scholar | PubMed |
Thompson RCA, Lymbery AJ, Smith A (2010) Parasites, emerging disease and wildlife conservation. International Journal for Parasitology 40, 1163-1170.
| Crossref | Google Scholar | PubMed |
Thompson CK, Wayne AF, Godfrey SS, Thompson RCA (2014a) Temporal and spatial dynamics of trypanosomes infecting the brush-tailed bettong (Bettongia penicillata): a cautionary note of disease-induced population decline. Parasites & Vectors 7, 169.
| Crossref | Google Scholar | PubMed |
Thompson CK, Godfrey SS, Thompson RCA (2014b) Trypanosomes of Australian mammals: a review. International Journal for Parasitology: Parasites and Wildlife 3, 57-66.
| Crossref | Google Scholar | PubMed |
Thompson RCA, Lymbery AJ, Godfrey SS (2018) Parasites at risk – insights from an endangered marsupial. Trends in Parasitology 34, 12-22.
| Crossref | Google Scholar | PubMed |
Viggers KL, Spratt DM (1995) The parasites recorded from Trichosurus species (Marsupialia: Phalangeridae). Wildlife Research 22, 311-332.
| Crossref | Google Scholar |
von Kéler KS (1971) A revision of the Australasian Boopiidae (Insecta: Phthiraptera), with notes on the Trimenoponidae. Australian Journal of Zoology 19, 1-126.
| Crossref | Google Scholar |
Watkins GE, Willers N, Raudino H, Kinloch J, van Dongen R (2018) Success criteria not met, but valuable information gained: monitoring a reintroduction of the tammar wallaby. Wildlife Research 45, 421-435.
| Crossref | Google Scholar |
Waudby HP, Petit S, Dixon B, Andrews RH (2007) Hosts of the exotic ornate kangaroo tick, Amblyomma triguttatum triguttatum Koch, on southern Yorke Peninsula, South Australia. Parasitology Research 101, 1323-1330.
| Crossref | Google Scholar | PubMed |
Wayne AF, Maxwell MA, Ward CG, Vellios CV, Wilson I, Wayne JC, Williams MR (2015) Sudden and rapid decline of the abundant marsupial Bettongia penicillata in Australia. Oryx 49, 175-185.
| Crossref | Google Scholar |
Wicks RM, Spencer PBS, Moolhuijzen P, Clark P (2006) Morphological and molecular characteristics of a species of Hepatozoon Miller, 1908 (Apicomplexa: Adeleorina) from the blood of Isoodon obesulus (Marsupialia: Peramelidae) in Western Australia. Systematic Parasitology 65, 19-25.
| Crossref | Google Scholar | PubMed |
Woinarski J, Burbidge AA (2016) Bettongia penicillata. The IUCN Red List of Threatened Species 2016: e.T2785A21961347. Available at http://dx.doi.org/10.2305/IUCN.UK.2016-2.RLTS.T2785A21961347.en [Accessed 2 May 2022]