Impact of green manure crop species on rhizosphere soil phosphorus
P. V. Nguyen


A
B
Abstract
Green manure crops have the potential to improve phosphorus (P) use efficiency in agroecosystems by enhancing the mobilisation of soil P reserves.
This study investigated and quantified the short-term mobilisation and uptake of soil P in the rhizosphere of several green manure crops.
Five plant species/varieties (Lupinus angustifolius (lupin – early and late flowering varieties), Pisum sativum (pea), Cicer Arietinum (chickpea), and Fagopyrum escolentum (buckwheat)) were grown in two contrasting soils, pumice (1100 mg total P kg−1, anion storage capacity 39%) and volcanic ash (2800 mg total P kg−1, anion storage capacity 95%) in rhizosphere study containers. After 40 days, rhizosphere (0–5 mm) and bulk (>5 mm) soils were sampled and subjected to P fractionation. Organic anions were collected from the rhizoplane using an anion exchange membrane.
Dry matter yield, P uptake, and rhizoplane organic anion exudation were affected by plant species, soil type, and their interaction. Rhizosphere P changes of labile inorganic organic P and stable inorganic P were influenced by plant species and soil type, while moderately labile inorganic P was affected by only plant species. Interaction between plant species and soil type had no effect on rhizosphere P depletion or accumulation. The quantities and composition of organic anions determined in rhizoplane exudates were highly variable (0.01–0.1 μmol cm−2 h−1). However, significant correlations were observed between the depletion of moderately labile and stable soil inorganic P and concentrations of malate in exudates.
The findings of this study clearly demonstrated the capacity of green manure crops (especially blue lupin) to rapidly mobilise and deplete different forms of soil P across the soil types.
Keywords: bulk soil, Cicer arietinum, Fagopyrum escolentum, green manure crops, legacy soil phosphorus, Lupinus angustifolius, organic anion, phosphorus accumulation, phosphorus depletion, phosphorus fractions, Pisum sativum, rhizosphere soil.
Introduction
Inputs of phosphorus (P) are required to enhance and maintain primary production, although this may be constrained in the future by continued depletion of known rock phosphate reserves (Faucon et al. 2015). Currently, around 17.5 million tonnes of phosphate rock are used for fertilisers annually (Lu and Tian 2017). Only 5–20% of this applied P is taken up by plants in the growing season following application (Loehr 1974; Sattari et al. 2012), while most is retained in the soil by combination of adsorption, precipitation, and biological immobilisation (Menezes-Blackburn et al. 2018). Accordingly, continued applications of P are required to maintain production, which leads to the accumulation of significant quantities of P in soil over time (Sattari et al. 2012; Condron et al. 2013). This accumulated P is known as ‘legacy P’ and can pose a long-term risk to water quality. Sattari et al. (2012) showed that globally approximately 60% of P applied between 1965 and 2007 had accumulated in soil, which amounted to 325 kg P per ha. Enhanced mobilisation of legacy soil P could contribute to improving overall P use efficiency in agroecosystems (Condron et al. 2013; Nash et al. 2014; Menezes-Blackburn et al. 2018). Several options have been suggested to improve P use efficiency and enhance utilisation of soil legacy P, including withholding/reducing P fertiliser inputs, changes to crop rotations, the development of alternative fertilisers (e.g. enzyme–nanoclay complexes), nanofertilisers, and inoculation with specific microorganisms (e.g. phosphate solubilising bacteria) (Condron et al. 2013; Haygarth et al. 2013; Calabi-Floody et al. 2018; Menezes-Blackburn et al. 2018; Raymond et al. 2021).
Inclusion of green manure or cover crops in crop rotations can have a significant impact on nutrient dynamics and bioavailability, including P (Eichler-Löbermann et al. 2008; Haygarth et al. 2013; Hallama et al. 2019). Green manures are short-term crops grown after the main crop, which are incorporated into the soil at an immature stage and provide an available source of energy and nutrients for soil biota. Green manures have been shown to significantly reduce nitrate-nitrogen leaching losses from soil (Fowler et al. 2004), and improve soil carbon cycling, biological activity, and physical properties (Dapaah and Vyn 1998; Dabney et al. 2001). With respect to P, Randhawa et al. (2005) found that the rate of organic P mineralisation increased five fold in soil after two cycles of growing green manure (Lupinus angustifolius) with maize (Zea mays) compared with a maize-fallow system. Eichler-Löbermann et al. (2008) showed that inclusion of a green manure can significantly increase the supply of P to the following crop. These limited findings indicate that the inclusion of green manure has the potential to enhance biological cycling of P in soil, which may in turn contribute to improving the availability and utilisation of applied P and legacy soil P (Condron et al. 2013; Haygarth et al. 2013). Some green manure plant species such as blue lupin (L. angustifolius) and buckwheat (Fagopyrum escolentum) have been shown to enhance dissolution and utilisation of sparingly-soluble phosphate rock applied to soil (Haynes 1992; McLenaghen et al. 2004), which was at least partly attributed to enhanced root exudation of low molecular weight organic anions such as citrate and oxalate. Furthermore, it has been proposed that the combined actions of exuded low molecular weight organic anions and phosphatase enzymes may be involved in the dissolution and hydrolysis of soil organic P by plants and microorganisms (Clarholm et al. 2015).
Much work has focused on impacts and benefits of green manure crops on soil P changes in P-deficient soils (Randhawa et al. 2005; Alamgir et al. 2012), but much less in cropping systems where P was in ample supply. The objectives of this study were to investigate and quantify the short-term mobilisation of P in medium-high fertility soils by a selection of potential green manure crops, and determine the relative effect on soil P concentrations and forms of the low molecular weight organic anions in root exudates. Our hypothesis was that green manure crops can mobilise soil legacy P at medium to high P status. We also hypothesised that low molecular weight organic anion exudation by roots of green manures may mobilise less-labile soil P in the rhizosphere.
Materials and methods
Green manure species
Based on a review of the literature relating to P demand and aquisition, 11 plant species were selected for preliminary screening, including L. angustifolius (two varieties including early and late flowering varieties (Egle et al. 2003; Pearse et al. 2007), Pisum sativum (pea) (Nuruzzaman et al. 2006), Cicer arietinum (chickpea) (Veneklaas et al. 2003; Wouterlood et al. 2004; Vu et al. 2008), F. escolentum (buckwheat) (Teboh and Franzen 2011; Possinger et al. 2013), Trifolium repens (white clover) (Deguchi et al. 2017), Tithonia speciosa (tithonia), Lupinus polyphyllus (russell lupin) (White et al. 1995), Brassica napus (rape) (Hedley et al. 1983; Hoffland 1992), Raphanus sativus (forage radish) (Zhang et al. 1997), and Brassica juncea (mustard) (Marschner et al. 2007). The short-term growth and root morphology of these plant species were assessed, and five plant species with high shoot biomass and extensive root systems were selected for further investigation: early and late flowering blue lupin, pea, chickpea, and buckwheat. Our experiment was intended to provide the foundation for more a detailed subsequent study.
Soils
The two topsoils (0–10 cm) selected for this study had been maintained under grazed pasture for over 20 years with annual inputs of P in the form of superphosphate. The soils were derived from different volcanic parent materials, namely pumice (Immature Orthic Pumice (NZ Classification), Typic udvitrand (USDA Classification)) and volcanic ash (Typic Orthic Allophanic (New Zealand Classification); Typic hapludand (USDA Classification)). They were sourced from the Taupo and Taranaki regions of the North Island of New Zealand, respectively. Selected chemical properties of the pumice and volcanic soils are presented in Table 1. The pH and cation exchange capacity of both soils were similar. The Olsen P and total P of both soils were relatively enriched compared to other soils used for pastoral grazing, especially in the volcanic soil (Roberts and Morton 2009; Morton and Roberts 2016). Total carbon and nitrogen were higher in the pumice soil, although corresponding carbon:nitrogen ratios were similar in both soils. These two soils were used for one experiment.
Rhizosphere containers
A modified version of the rhizosphere study container system originally developed by Chen et al. (2002) was used to separate and quantify short-term soil P depletion or accretion by the different green manure plant species (Fig. 1). The study container was comprised of two 40 mm diameter plastic cylinders with 20 μm nylon mesh (Sefar Nitex, Switzerland) attached to the base of the top 40 mm cylinder. The mesh created a rhizoplane to the lower cylinder of which the top 5 mm of soil was classified as ‘rhizosphere’ soil, and the remaining 15 mm cylinder represented ‘non-rhizosphere’ or ‘bulk’ soil (sealed with 100 μm nylon mesh). Each container was placed on a layer of cotton felt (5 mm) atop a 25 mm base of acid-washed sand connected to a water reservoir maintained at 0 KPa, allowing water to wick into the container. The pumice and volcanic soils were air-dried, ground to <2 mm and packed into the upper and lower containers at densities of 0.90 and 0.89 g cm−3 based on volume weight, respectively. Due to variability in soil P forms noted by Chen et al. (2002), eight replicates of each soil–plant combination were included in the experiment. Plants were grown from seed in the upper compartment for 21 days with the addition of nitrogen, sulfur, and molybdenum (5 mL 8.1 g L−1 ammonium sulfate ((NH4)2SO4) and 1 mL 3.6 g L−1 ammonium molybdate ((NH4)2MoO4) – equivalent to 360, 390 and 70 kg ha−1 of nitrogen, sulfur, and molybdemum) in a glasshouse maintained at 15–23°C. After 21 days, the lower compartment was attached, and the plants were allowed to grow for an additional 40 days prior to plant and soil sampling. The plants were arranged in the completely randomised design.
Sampling and analysis
After 61 days, plant shoots were cut at the soil surface, dried at 60°C, weighed, and ground (<1 mm) prior to determination of P concentration by ICP-OES following digestion with nitric acid-hydogen peroxide (Metson 1972; Anderson 1996). P concentration was used to calculate P uptake by the following equation:
Forty days after attachment, the top 5 mm ring of the lower container was carefully separated from the top container mesh and the bulk soil cylinder. Soils from the rhizosphere and bulk soil cylinders were dried at 30°C and sieved to <1 mm prior to P fractionation by sequential extraction. We used the sequential extraction scheme developed by Boitt et al. (2018a) based on Chen et al. (2000) and Condron and Newman (2011). This involved sequential extraction with 1 mol L−1 ammonium chloride (NH4Cl), 0.5 mol L−1 sodium bicarbonate (NaHCO3) at pH 8.5, 0.1 mol L−1 sodium hydroxide (NaOH) (NaOH I), 1 mol L−1 hydrochloric acid (HCl), 0.1 mol L−1 NaOH (NaOH II), followed by sulfuric acid-hydrogen peroxide digestion of the residue. Inorganic P in the extracts was determined by colourimetry (Dick and Tabatabai 1977; He and Honeycutt 2005), and total P was determined by the same method after ammonium persulfate digestion (Murphy and Riley 1962; Eisenreich et al. 1975). The difference between total and inorganic P in the NaHCO3 and both NaOH extracts represented organic P. Inorganic and organic P extracted by NaHCO3 were designated ‘labile P’, while inorganic and organic P extracted by the first and second NaOH extracts were designated ‘moderately labile P’ (NaOH I) and ‘stable P’ (NaOH II), respectively. Inorganic P determined in the HCl extract and the final acid digest were designated ‘acid-soluble-P’ and ‘residual P’, respectively (Boitt et al. 2018a). NH4Cl extraction was used to remove cations including calcium which significantly affects the following P pool analysis (Condron and Newman 2011). Although soluble and readily extractable P was extracted by NH4Cl, this P pool was not reported in the results due to very low P pool (under limit of detection).
Low molecular weight organic anions (hereafter referred to as organic anions) released by roots in exudates were collected by adsorption onto the anion exchange membrane (No. 55164 2S, BDH Laboratory Supplies, England) at the rhizoplane. Preparation of the membranes involved cutting 2.5 cm × 2.5 cm squares, soaking them in deionised water for 24 h, and in 0.5 M mol L−1 NaHCO3 for 24 h before being rinsed with deionised water and stored in 0.1 mol L−1 NaCl at 4°C (Schefe et al. 2008; Cheesman et al. 2010; Shi et al. 2011a). The squares, backed with moist filter paper (Whatman GFC), were placed under the root mat (rhizoplane) for 2 h (Shi et al. 2011a). After removal, the membranes were rinsed with deionised water and anions desorbed with 4 mL of 0.5 mol L−1 HCl (3 h at 4°C). Seven organic anions (acetate, citrate, formate, lactate, malate, malonate, and pyruvate) were analysed by high-performance liquid chromatography using a Shimadzu HPLC with UV-Vis Detector (Shi et al. 2011a). The column used to separate and analyse organic acids was Prevail TM organic acid column (250 mm × 4.6 mm, 5 μm particle size; Grace Davison Discovery Sciences) with a Guard column (7.5 mm × 4.6 mm, 5 μm particle size; Grace Davison Discovery Sciences). The mobile phase was 25 mM KH2PO4 (pH 2.35, adjusted by H3PO4), filtered through a 0.45 μm cellulose acetate membrane. The flow rate was 0.6 mL per min with column temperature of 50°C and 30 μL for sample injection volume. The detector wavelength was 210 nm.
Statistical analysis
Linear models including soil type, plant species, and their interaction (i.e. two-way) were fitted to above-ground plant yield, plant P uptake, and total rhizoplane organic anions; when subsequent analyses of variance (ANOVAs) suggested one or both factors were important sources of variance, post hoc differences were calculated via Tukey’s honest significant differences (α = 0.05). The assumptions are that the data are independent and identically distributed; further, it is assumed the error is normally distributed. We inspected residual plots to determine if our assumptions were justified. Differences between bulk and rhizosphere soils for the P fractions were calculated and also analysed via the two-way model; these differences were summarised via estimated marginal means (‘emmeans’ package; Lenth 2023). Total inorganic P and total organic P depletion were summed up depletion of all inorganic and organic P pools, respectively. Pearson correlation coefficients (r) were used to determine correlations between root organic anion exudation and P pools, and between rhizosphere P depletion and dry matter yield and P uptake; data were log-transformed when necessary. To compare organic anion percentages across soil type and plant species, generalised linear models were fitted assuming the percentages were beta distributed. Two-way ANOVA was also appended for each corresponding organic anion analysis. All analysis was done via R statistical software (v.4.3.0).
Results
Plant yield and phosphorus uptake
Plant dry matter yield and P uptake were affected by the interaction between green manure species and soil types (Table 2). For the pumice soil, plant yield and P uptake were similar for all plant species except early flowering lupin (Table 3). On the other hand, the volcanic soil yield was significantly higher for pea and buckwheat, and P uptake was significantly greater for buckwheat compared with other species (Table 3).
Source of variation | P-value | |||||||||||
---|---|---|---|---|---|---|---|---|---|---|---|---|
Dry matter yield | P uptake | Total rhizosphere organic anions | Labile inorganic P | Labile organic P | Moderately labile inorganic P | Moderately labile organic P | Acid-soluble inorganic P | Stable inorganic P | Stable organic P | Residual P | ||
Soil type | <0.0001 | <0.0001 | 0.0047 | 0.0045 | <0.0001 | 0.5976 | 0.1387 | 0.6377 | 0.0038 | 0.2153 | 0.1541 | |
Plant species | <0.0001 | <0.0001 | <0.0001 | 0.0032 | <0.0001 | 0.0017 | 0.1547 | 0.5860 | 0.0070 | 0.0371 | 0.5226 | |
Soil type × Plant species | <0.0001 | <0.0001 | 0.0089 | 0.8253 | 0.1294 | 0.3420 | 0.9794 | 0.7205 | 0.4872 | 0.8865 | 0.4008 |
DMY (g pot−1) | P uptake (mg P pot−1) | ||||
---|---|---|---|---|---|
Pumice | Volcanic ash | Pumice | Volcanic ash | ||
Ef-lupin | 1.095 ± 0.302c | 1.188 ± 0.427c | 1.20 ± 0.33b | 1.43 ± 0.51b | |
Lf-lupin | 1.813 ± 0.087a | 1.462 ± 0.240c | 1.45 ± 0.07ab | 1.46 ± 0.34b | |
Pea | 1.465 ± 0.201b | 2.350 ± 0.354ab | 1.61 ± 0.22a | 2.11 ± 0.32b | |
Chickpea | 1.699 ± 0.072ab | 1.641 ± 0.591c | 1.53 ± 0.07a | 1.48 ± 0.53b | |
Buckwheat | 1.650 ± 0.174ab | 2.945 ± 0.517a | 1.49 ± 0.15a | 3.54 ± 0.62a |
Values for differences without a common letter in the same column are significantly different at α = 0.05.
Ef, early flowering; Lf, late flowering.
Soil phosphorus fractions
More plant species depleted various soil P fractions in the pumice soil compared with the volcanic ash soil after 40 days’ growth (Fig. 2 and Supplementary Table S1). For the pumice soil, depletion of labile inorganic P (5–8 mg P kg−1) and accretion of labile organic P (11–28 mg P kg−1) were determined for all plant species except pea. For the volcanic ash soil, the difference was the depletion of inorganic P under all plant species (5–13 mg P kg−1), while the accretion of labile organic P occurred under only Lf-lupin (10 mg P kg−1). Results for moderately labile P showed that for the pumice soil there was a depletion of inorganic P for both blue lupin varieties (62–63 mg P kg−1), which was observed for Ef-lupin (101 mg P kg−1) and pea (39 mg P kg−1) in the volcanic ash soil. Moderately labile organic P depletion occurred in the pumice soil for pea (62 mg P kg−1). Data for acid-soluble inorganic P showed that non-significant depletion occurred for all plant species in both soils. Corresponding results for stable P revealed that depletion of inorganic P (7–18 mg P kg−1) occurred under all plant species except pea in the volcanic ash soil. Depletion of stable organic P (17–35 mg P kg−1) was also found under all plant species but pea in the pumice soil. Depletion of stable organic P (27–37 mg P kg−1) was observed under both blue lupin varieties for the volcanic ash soil.
Mean differences in soil P pools between bulk and rhizosphere soils for each P fraction, conditional on soil type and green manure plant species (based on two-way linear models including interactions). Lines are the 95% confidence intervals. Negative values indicate depletion of the pool in the rhizosphere, relative to bulk soil.
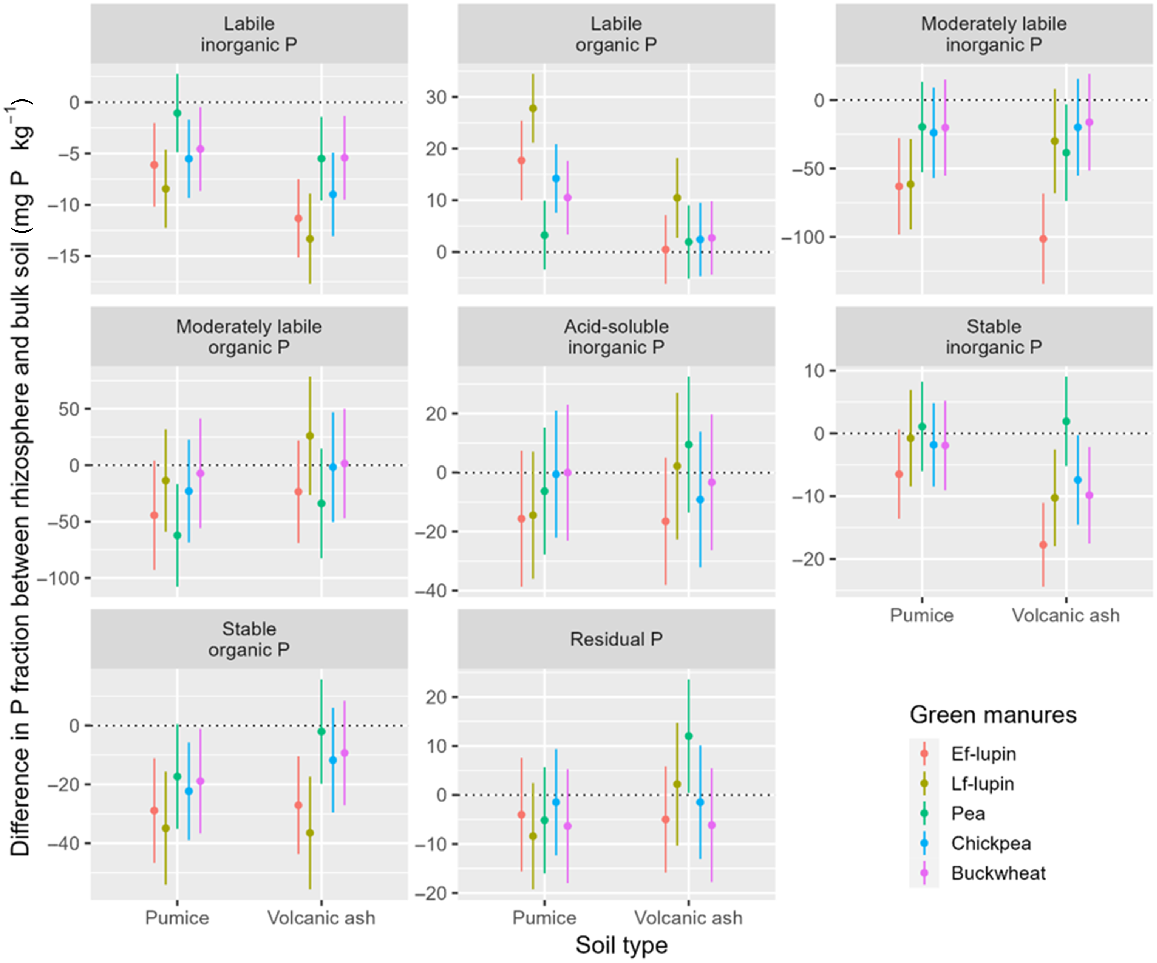
The overall means of P depletion in the rhizosphere for each soil type averaged across plant species show labile and stable inorganic P pools were more depleted in the volcanic ash soil compared with the pumice soil (Table 4). Across soil types, the overall means of P depletion or accumulation in the rhizosphere indicate that the highest depletion of labile and moderately inorganic P occurred under lupin species, especially Ef-lupin (Table 5). Lf-lupin also accumulated higher labile organic P than others (Table 5). While Ef-lupin depleted stable inorganic P, pea slightly accumulated this P pool, causing a significant difference between two plant species in terms of the change of the P pool (Table 5). Stable organic P was depleted significantly higher under Lf-lupin compared with pea (Table 5).
P fractions | Pumice | Volcanic ash | P-value | |
---|---|---|---|---|
mg P kg−1 | ||||
Labile inorganic P | −5.13 ± 0.61b | −8.85 ± 1.25a | 0.008 | |
Labile organic P | 14.60 ± 1.80a | 3.31 ± 1.88b | <0.0001 | |
Moderately labile inorganic P | −37.40 ± 4.37a | −43.20 ± 11.70a | 0.633 | |
Moderately labile organic P | −30.37 ± 8.42a | −7.75 ± 12.70a | 0.136 | |
Acid-soluble inorganic P | −7.37 ± 2.92a | −3.99 ± 6.56a | 0.630 | |
Stable inorganic P | −2.01 ± 0.90b | −8.85 ± 2.30a | 0.007 | |
Stable organic P | −24.10 ± 1.70a | −17.07 ± 5.52a | 0.228 | |
Residual P | −5.07 ± 0.96a | 0.12 ± 3.58a | 0.152 |
P fractions | Ef-lupin | Lf-lupin | Pea | Chickpea | Buckwheat | P-value | |
---|---|---|---|---|---|---|---|
mg P kg−1 | |||||||
Labile inorganic P | −8.89 ± 1.32a | −10.53 ± 1.57a | −3.13 ± 1.06b | −7.14 ± 1.92ab | −4.99 ± 1.30ab | 0.005 | |
Labile organic P | 7.85 ± 3.78b | 20.36 ± 3.33a | 2.63 ± 2.09b | 8.71 ± 2.72b | 6.62 ± 2.57b | 0.001 | |
Moderately labile inorganic P | −83.47 ± 10.65a | −48.00 ± 17.66ab | −28.42 ± 11.29b | −22.04 ± 10.18b | −18.18 ± 10.08b | 0.001 | |
Moderately labile organic P | −33.30 ± 20.24a | 3.36 ± 19.81a | −49.09 ± 12.71a | −13.07 ± 15.40a | −2.89 ± 13.09a | 0.154 | |
Acid-soluble inorganic P | −16.10 ± 9.15a | −7.32 ± 7.85a | 1.08 ± 7.42a | −4.57 ± 8.22a | −1.66 ± 5.84a | 0.577 | |
Stable inorganic P | −12.49 ± 2.92a | −5.51 ± 2.75ab | 1.50 ± 3.65b | −4.43 ± 1.58ab | −5.58 ± 1.89ab | 0.010 | |
Stable organic P | −27.93 ± 9.43ab | −35.68 ± 4.16a | −9.68 ± 5.94b | −17.39 ± 2.45ab | −14.10 ± 5.74ab | 0.033 | |
Residual P | −4.53 ± 2.66a | −3.85 ± 3.05a | 2.86 ± 6.81a | −1.47 ± 2.09a | −6.24 ± 3.69a | 0.555 |
Organic anions
Total organic anions were not only affected by green manures and soil types, but also by the interaction between them (Table 2). Total organic anion exudation varied greatly between plant species (0.010–0.100 μmol cm−2 h−1) (Fig. 3). The greatest concentrations were found in the chickpea and late flowering lupin in both soils. However, significant differences were only found between chickpea and other plant species for the pumice soil, and between chickpea and buckwheat for the volcanic ash soil. The relative percentages of different organic anions are presented in Table 6 and show that multiple organic anions were starkly different among plant species and soil types. Formate, lactate, malate, malonate, and pyruvate were affected by interaction between soil type and plant species. Lactate (12–71%, average 45%), citrate (7–24%, average 14%), and malate (4–30%, average 14%) were the most abundant anions detected. The combination of lactate, citrate, and malate accounted for over 70% of total organic anions found in exudates from early and late flowering lupin, pea and buckwheat for both soils, while relatively high concentrations of malonate (21–51%) were present in chickpea exudates. There were no significant correlations observed between total organic anion exudate concentrations at the rhizoplane and total soil P depletion in the rhizosphere across all soil–plant combinations (r = −0.01 and P = 0.88). However, the concentration of malate in exudates was correlated to the depletion of moderately labile inorganic P (r = 0.33, P = 0.012) and stable inorganic P (r = 0.36, P = 0.007).
Mean total root organic anion exudation (μmol cm−2 h−1) determined for five green manure plant species at the rhizoplane of the pumice and volcanic soils (for each soil type, values for different plant species without a common letter are significantly different at α = 0.05). Ef, early flowering; Lf, late flowering.
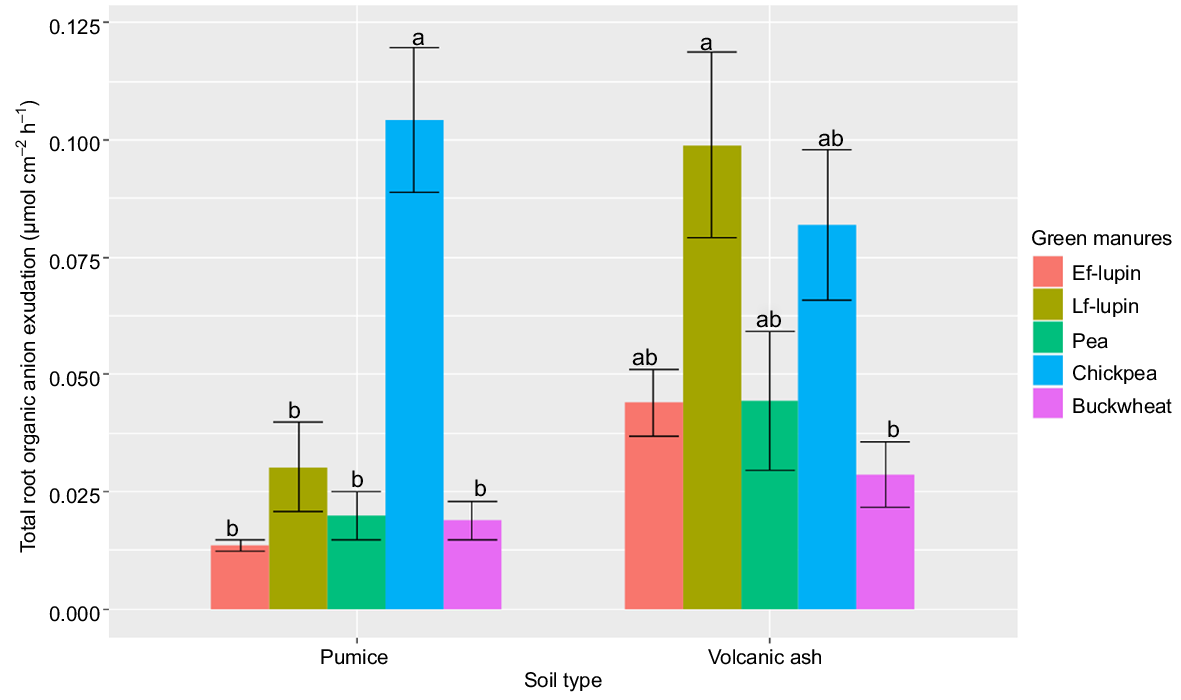
Soil type | Plant species | Acetate | Citrate | Formate | Lactate | Malate | Malonate | Pyruvate | |
---|---|---|---|---|---|---|---|---|---|
% of total | |||||||||
Pumice | Ef-lupin | 0.6 (0.2–1.7) ab | 11.5 (6.3–20.1) a | 15.3 (10.6–21.4) bc | 42.0 (28.6–56.8) abc | 29.7 (21.5–39.5) b | 0.4 (0.2–1.2) a | 2.2 (1.3–3.8) ab | |
Lf-lupin | 7.0 (3.0–15.5) c | 10.5 (5.6–18.8) a | 18.3 (13.2–24.8) c | 44.2 (30.5–58.9) bc | 17.9 (11.6–26.5) ab | 4.2 (1.7–9.9) bc | 1.8 (1.0–3.2) ab | ||
Pea | 0.4 (0.2–1.2) a | 12.0 (6.7–20.8) a | 5.6 (3.1–9.8) ab | 44.6 (30.8–59,2) bc | 14.6 (9.0–22.8) ab | 0.2 (0.1–0.6) a | 13.1 (10.4–16.3) d | ||
Chickpea | 7.3 (3.2–16.1) c | 23.8 (15.6–34.5) a | 2.2 (1.0–4.8) a | 12.2 (5.9–23.5) a | 9.2 (5.1–16.1) a | 50.5 (38.0–62.9) d | 1.4 (0.7–2.7) a | ||
Buckwheat | 14.1 (7.1–26.1) c | 7.1 (3.4–14.0) a | 9.6 (6.1–14.9) abc | 62.9 (48.0–75.6) bc | 5.6 (2.8–11.2) a | 0.3 (0.1–0.9) a | 5.3 (3.7–7.6) bc | ||
Ef-lupin | 4.9 (2.0–11.7) bc | 24.2 (16.0–35.0) a | 14.2 (9.820.2) bc | 42.4 (28.9–57.1) bc | 13.7 (8.3–21.7) ab | 0.8 (0.3–2.3) ab | 2.6 (1.6–4.3) ab | ||
Lf-lupin | 9.7 (4.4–19.8) c | 12.0 (6.7–20.8) a | 8.9 (5.5–14.0) abc | 34.9 (22.6–49.8) ab | 29.4 (21.2–39.2) b | 6.5 (2.9–13.9) bc | 3.0 (1.8–4.8) abc | ||
Volcanic ash | Pea | 0.6 (0.2–1.7) ab | 8.1 (4.0–15.5) a | 6.3 (3.6–10.8) ab | 71.2 (56.6–82.4) c | 4.3 (2.0–9.2) a | 0.2 (0.1–0.6) a | 7.1 (5.1–9.6) cd | |
Chickpea | 5.2 (2.1–12.2) bc | 15.8 (9.4–25.4) a | 5.6 (3.1–9.8) ab | 45.9 (32.0–60.5) bc | 6.3 (3.2–12.2) a | 21.0 (12.7–32.7) c | 3.1 (1.9–5.0) abc | ||
Buckwheat | 16.4 (8.6–29.0) c | 11.9 (6.6–20.7) a | 6.3 (3.6–10.7) ab | 51.6 (37.1–65.8) bc | 8.6 (4.7–15.4) a | 0.3 (0.1–0.9) a | 5.0 (3.4–7.2) bc | ||
P-value | |||||||||
Soil type | 0.732 | 0.239 | 0.213 | 0.263 | 0.346 | 0.971 | 0.816 | ||
Plant species | <0.0001 | 0.016 | <0.0001 | <0.0001 | <0.0001 | <0.0001 | <0.0001 | ||
Plant species × soil type | 0.101 | 0.076 | 0.027 | 0.0005 | 0.0006 | 0.030 | 0.003 |
Values here represent the cell means (95% confident interval) based on beta regression models.
Ef, early flowering; Lf, late flowering.
Discussion
It has been acknowledged that the inclusion of green manures in crop rotations has the potential to alter P cycling, improve P use efficiency, and thereby reduce the quantity of P inputs required to maintain production (Condron et al. 2013; Hallama et al. 2019). It is therefore important to investigate and quantify the impact of different green manure plant species on the acquisition of P from soil (Pearse et al. 2007; Mat Hassan et al. 2012; Hallama et al. 2019), including soils that contain significant quantities of legacy P.
The mechanisms of P mobilisation by plants can be ascribed to differences and interactions between the root exudation of organic anions, rhizosphere microbial activity, root morphology, and the availability of soil P. As this experiment was designed to determine the removal of soil legacy P, individual effects of each factor mentioned above were not investigated in detail. Instead we focus this discussion on the changes in soil P and the relative effects of root organic anions on rhizosphere soil P concentrations and forms that occurred.
Dry matter yield and P uptake varied under different plant species and soil types in this study. This was consistent with the study of Chen et al. (2002) that biomass and P uptake were affected by plant species and soil types. In the present study, the higher P uptake by pea and buckwheat in the volcanic ash soil could be due to the higher available P compared with that in the pumice soil. Consequently, dry matter yield increased in the volcanic ash soil. By contrast, this did not occur for other plant species. It seems that the different root structure and the constraint volume of rhizosphere study containers impacted the exploration of roots to the soils, leading to the different results in dry matter yield and P uptake as compared with other plant species.
Labile P concentrations were, on average, different between the two soil types irrespective of plant species, while both concentrations of labile and moderately labile P were, on average, different under the plant species across the soil types (Tables 4 and 5). Changes of labile and stable P pools in the rhizosphere were affected by both plant species and soil type, while moderately labile P was only affected by plant species. The initial labile P concentration of the soil may relate to P depletion by plants (McDowell and Condron 2004; Dodd et al. 2012). In high P status soils, the concentrations of bioavailable P need to be substantially reduced by repeated biomass removal with no P inputs (Dodd et al. 2012, 2013; Boitt et al. 2018b; McDowell et al. 2020) to reach agronomic optimum P concentration (McDowell et al. 2020), before the use of alternative plant species to further utilise legacy P, improve P use efficiency, and reduce risk of P loss in drainage can be expected to be a viable option.
The enhanced mobilisation of inorganic P compared with organic P in the pumice soil is consistent with findings from other soil P depletion experiments (Perrott 1992; McDowell et al. 2016; Boitt et al. 2018c; Chen et al. 2019). Although no effect of the interaction between soil types and plant species on P depletion was observed, grouping soil types or plant species shows some differences in terms of P changes in the rhizosphere. Lupin species seemed to deplete more P than other species across the soils. This could be due to differences in rhizoplane root mass and morphology between plant species. Depletion of extractable inorganic P by both lupin varieties was mainly due to substantial decreases in the moderately labile fraction, which was also observed by Boitt et al. (2018c) and Chen et al. (2019). The observed depletion of P in both soils was not related to plant yield (r = −0.23, P = 0.18 for the pumice soil, and r = −0.23, P = 0.20 for the volcanic ash soil) nor P uptake (r = −0.22, P = 0.20 for the pumice soil and r = −0.13 and P = 0.47 for the volcanic ash soil), which was as expected given that impact assessment was based on differences in soil P determined at and adjacent to the rhizoplane created by the nylon mesh (Chen et al. 2002). P depletion at the rhizosphere constituted a very small percentage for P uptake that mainly relied on root biomass exploring the soil in the upper container. Root structure and morphology were different between plant species, leading to differences in areas of root–soil interface for various P uptake.
The depletion of organic P by early flowering lupin and pea were due to decreases in moderately labile and stable P, although for lupin this was countered by a concomitant increase in labile organic P. The increase in labile organic P could be caused by immobilisation of inorganic P into organic P forms by microbes in the rhizosphere under early flowering lupin (Armstrong and Helyar 1992; Grayston et al. 1997; Mat Hassan et al. 2012). Secondly, increases in labile organic P could have been derived from breakdown of more stable forms of organic P linked to a combination of increased organic anions and enhanced microbial activity in the rhizosphere. The absence of labile organic P accretion in the rhizosphere of pea may indicate enhanced mineralisation of this form of organic P compared with early flowering lupin (Armstrong and Helyar 1992). The contrasting extent of inorganic P depletion and pattern of organic P mobilisation suggests that the mechanisms involved were different for lupin compared with pea. In general, lupin species depleted more P than other species across both soils. This could be due to differences in rhizoplane root mass and morphology between the plant species. Moreover, the difference regarding P mobilisation could be partly explained by the symbiosis of different mycorrhizae associated with roots (Chen et al. 2002).
Consideration of the potential role of root exudate low molecular weight organic anions in rhizosphere P dynamics and bioavailability was included in the current study to investigate the ‘unbutton’ model of soil P acquisition proposed by Clarholm et al. (2015). According to this model, organic anions produced by plant roots and microorganisms play a crucial role in the release of inorganic and organic P from mineral surfaces and organic matter by chelation of binding metal ions such as iron and aluminium. Investigating the role and function of organic anions is acknowledged to be extremely challenging due to a combination of factors including separating roots from soil, spatial and temporal variations in exudate production, and the labile nature of these compounds in the rhizosphere environment (Jones et al. 2004; Shi et al. 2013; Oburger and Jones 2018). Nonetheless, it is possible to capture exudate organic anions at the plant–soil interface using an anion exchange membrane (Schefe et al. 2008; Shi et al. 2011a), and the composition of organic anions present has been shown to have a major impact on microbial activity and diversity (Shi et al. 2011b).
The mass and morphology of the root mat formed at the nylon mesh in the root study container probably varied between plant species and possibly soil type, which in turn may influence organic anion capture by the attached exchange membrane. This may at least partly account for the high degree of variability in the total concentrations of organic anions determined for the different plant species in both soils. Sampling organic anions at a single point in time at the end of the period may not accurately reflect the organic anion production of the roots during the whole period, which may explain the lack of correlation between most organic anions and P depletion. Nonetheless, comparison between the relative proportions of the seven organic anions detected at the rhizoplane of both lupin varieties and pea in the pumice soil revealed that formate and malate were greater for lupin compared with acetate and pyruvate for pea. Given that Shi et al. (2013) demonstrated that individual organic anions influenced the activity of specific soil bacterial communities, it is possible that different organic anions may be involved in the release of P from different metal complexes in soil. Thus malate, a dicarboxylate, may react differently with soil cations compared with monocarboxylates such as acetate (Clarholm et al. 2015). Accordingly, exudation of malate by lupin may have played a role in mobilising soil inorganic P. This, in turn, could at least partly account for the significant correlation observed between depletion of moderately labile and stable forms of inorganic P and the concentration of malate in root exudates.
The interaction between plant species and soil types affected the exudation of rhizoplane organic anions. This could be attributed to composition of organic anions. For example, chickpea released more malonate in the pumice soil than that in the volcanic ash soil, while other plant species exuded more lactate in both soil types. Egle et al. (2003) also found that organic anion exudation was different between plant species and between soil P levels. Similarly, the composition and proportion of root organic anions varied with soil type in the study by Richardson et al. (2009), yielding different exudation rates of total organic anions across soils. Further detailed investigation is warranted for how organic anions are involved in mobilisation of inorganic and organic P in the rhizosphere of different plants (e.g. lupin species, pea) including the complementary role of phosphatase enzyme activity.
Conclusions
The short-term study of various green manure species demonstrated no significant relationship between P uptake and rhizosphere P depletion. However, P uptake, dry matter yield, and rhizoplane organic anions were affected by the interaction between soil type and plant species. Labile and stable P pools were affected by both soil type and plant species, while moderately labile P was merely affected by plant species. Ef-lupin depleted more moderately labile inorganic P than other plant species across the soil types. High variability in total organic anion exudation at the rhizoplane was found between plant species in both soil types. However, the quality and proportion of organic anions (e.g. malate) could play an important role in P mobilisation. For early flowering blue lupin and pea, there appeared to be a difference in P mobilisation mechanisms. Further study is, therefore, required to investigate detailed mechanisms responsible for enhanced soil P depletion under green manures.
Data availability
The data that support this study are available in Figshare at https://doi.org/10.6084/m9.figshare.21707528.
Conflicts of interest
The authors declare no conflicts of interest. Leo Condron is an Associate Editor for the Journal.
Acknowledgements
Lincoln University provided funding for this study and Ministry of Agriculture and Rural Development of the Socialist Republic of Viet Nam provided PhD Scholarship. The authors would like to thank Neil Smith for his help with setting up the experiment, Zach Simpson for advice on statistics, and two anonymous reviewers for helpful comments.
References
Alamgir M, McNeill A, Tang C, Marschner P (2012) Changes in soil P pools during legume residue decomposition. Soil Biology and Biochemistry 49, 70-77.
| Crossref | Google Scholar |
Anderson KA (1996) Micro-digestion and ICP-AES analysis for the determination of macro and micro elements in plant tissues. Atomic Spectroscopy 17, 30-33.
| Google Scholar |
Armstrong RD, Helyar KR (1992) Changes in soil phosphate fractions in the rhizosphere of semi-arid pasture grasses. Soil Research 30, 131-143.
| Crossref | Google Scholar |
Boitt G, Tian J, Black A, Wakelin SA, Condron LM (2018a) Effects of long-term irrigation on soil phosphorus under temperate grazed pasture. European Journal of Soil Science 69, 95-102.
| Crossref | Google Scholar |
Boitt G, Simpson ZP, Tian J, Black A, Wakelin SA, Condron LM (2018b) Plant biomass management impacts on short-term soil phosphorus dynamics in a temperate grassland. Biology and Fertility of Soils 54, 397-409.
| Crossref | Google Scholar |
Boitt G, Black A, Wakelin SA, McDowell RW, Condron LM (2018c) Impacts of long-term plant biomass management on soil phosphorus under temperate grassland. Plant and Soil 427, 163-174.
| Crossref | Google Scholar |
Calabi-Floody M, Medina J, Rumpel C, Condron LM, Hernandez M, Dumont M, de la Luz Mora M (2018) Smart fertilizers as a strategy for sustainable agriculture. In ‘Advances in agronomy, Vol. 147’. (Ed. DL Sparks) pp. 119–157. doi:10.1016/bs.agron.2017.10.003
Cheesman AW, Turner BL, Reddy KR (2010) Interaction of phosphorus compounds with anion-exchange membranes: implications for soil analysis. Soil Science Society of America Journal 74, 1607-1612.
| Crossref | Google Scholar |
Chen CR, Condron LM, Davis MR, Sherlock RR (2000) Effects of afforestation on phosphorus dynamics and biological properties in a New Zealand grassland soil. Plant and Soil 220, 151-163.
| Crossref | Google Scholar |
Chen CR, Condron LM, Davis MR, Sherlock RR (2002) Phosphorus dynamics in the rhizosphere of perennial ryegrass (Lolium perenne L.) and radiata pine (Pinus radiata D. Don.). Soil Biology and Biochemistry 34, 487-499.
| Crossref | Google Scholar |
Chen X, Jiang N, Condron LM, Dunfield KE, Chen Z, Wang J, Chen L (2019) Soil alkaline phosphatase activity and bacterial phoD gene abundance and diversity under long-term nitrogen and manure inputs. Geoderma 349, 36-44.
| Crossref | Google Scholar |
Clarholm M, Skyllberg U, Rosling A (2015) Organic acid induced release of nutrients from metal-stabilized soil organic matter – the unbutton model. Soil Biology and Biochemistry 84, 168-176.
| Crossref | Google Scholar |
Condron LM, Newman S (2011) Revisiting the fundamentals of phosphorus fractionation of sediments and soils. Journal of Soils and Sediments 11, 830-840.
| Crossref | Google Scholar |
Condron LM, Spears BM, Haygarth PM, Turner BL, Richardson AE (2013) Role of legacy phosphorus in improving global phosphorus-use efficiency. Environmental Development 8, 147-148.
| Crossref | Google Scholar |
Dabney SM, Delgado JA, Reeves DW (2001) Using winter cover crops to improve soil and water quality. Communications in Soil Science and Plant Analysis 32, 1221-1250.
| Crossref | Google Scholar |
Dapaah HK, Vyn TJ (1998) Nitrogen fertilization and cover crop effects on soil structural stability and corn performance. Communications in Soil Science and Plant Analysis 29, 2557-2569.
| Crossref | Google Scholar |
Deguchi S, Uozumi S, Touno E, Uchino H, Kaneko M, Tawaraya K (2017) White clover living mulch reduces the need for phosphorus fertilizer application to corn. European Journal of Agronomy 86, 87-92.
| Crossref | Google Scholar |
Dick WA, Tabatabai MA (1977) Determination of orthophosphate in aqueous solutions containing labile organic and inorganic phosphorus compounds. Journal of Environmental Quality 6, 82-85.
| Crossref | Google Scholar |
Dodd RJ, McDowell RW, Condron LM (2012) Predicting the changes in environmentally and agronomically significant phosphorus forms following the cessation of phosphorus fertilizer applications to grassland. Soil Use and Management 28, 135-147.
| Crossref | Google Scholar |
Dodd RJ, McDowell RW, Condron LM (2013) Changes in soil phosphorus availability and potential phosphorus loss following cessation of phosphorus fertiliser inputs. Soil Research 51, 427-436.
| Crossref | Google Scholar |
Egle K, Römer W, Keller H (2003) Exudation of low molecular weight organic acids by Lupinus albus L., Lupinus angustifolius L. and Lupinus luteus L. as affected by phosphorus supply. Agronomie 23, 511-518.
| Crossref | Google Scholar |
Eichler-Löbermann B, Köhne S, Kowalski B, Schnug E (2008) Effect of catch cropping on phosphorus bioavailability in comparison to organic and inorganic fertilization. Journal of Plant Nutrition 31, 659-676.
| Crossref | Google Scholar |
Eisenreich SJ, Bannerman RT, Armstrong DE (1975) A simplified phosphorus analysis technique. Environmental Letters 9, 43-53.
| Crossref | Google Scholar |
Faucon M-P, Houben D, Reynoird J-P, Mercadal-Dulaurent A-M, Armand R, Lambers H (2015) Advances and perspectives to improve the phosphorus availability in cropping systems for agroecological phosphorus management. In ‘Advances in agronomy, Vol. 134’. (Ed. DL Sparks) pp. 51–79. doi:10.1016/bs.agron.2015.06.003
Fowler CJE, Condron LM, McLenaghen RD (2004) Effects of green manures on nitrogen loss and availability in an organic cropping system. New Zealand Journal of Agricultural Research 47, 95-100.
| Crossref | Google Scholar |
Grayston SJ, Vaughan D, Jones D (1997) Rhizosphere carbon flow in trees, in comparison with annual plants: the importance of root exudation and its impact on microbial activity and nutrient availability. Applied Soil Ecology 5, 29-56.
| Crossref | Google Scholar |
Hallama M, Pekrun C, Lambers H, Kandeler E (2019) Hidden miners – the roles of cover crops and soil microorganisms in phosphorus cycling through agroecosystems. Plant and Soil 434, 7-45.
| Crossref | Google Scholar |
Haygarth PM, Bardgett RD, Condron LM (2013) Nitrogen and phosphorus cycles and their management. In ‘Soil conditions and plant growth’. (Eds PJ Gregory, S Nortcliff) pp. 132–159. doi:10.1002/9781118337295.ch5)
Haynes RJ (1992) Relative ability of a range of crop species to use phosphate rock and monocalcium phosphate as P sources when grown in soil. Journal of the Science of Food and Agriculture 60, 205-211.
| Crossref | Google Scholar |
He Z, Honeycutt CW (2005) A modified molybdenum blue method for orthophosphate determination suitable for investigating enzymatic hydrolysis of organic phosphates. Communications in Soil Science and Plant Analysis 36, 1373-1383.
| Crossref | Google Scholar |
Hedley MJ, Nye PH, White RE (1983) Plant-induced changes in the rhizosphere of rape (Brassica napus Var. Emerald) seedlings. New Phytologist 95, 69-82.
| Crossref | Google Scholar |
Hoffland E (1992) Quantitative evaluation of the role of organic acid exudation in the mobilization of rock phosphate by rape. Plant and Soil 140, 279-289.
| Crossref | Google Scholar |
Jones DL, Hodge A, Kuzyakov Y (2004) Plant and mycorrhizal regulation of rhizodeposition. New Phytologist 163, 459-480.
| Crossref | Google Scholar | PubMed |
Loehr RC (1974) Characteristics and comparative magnitude of non-point sources. Journal (Water Pollution Control Federation) 46, 1849-1872.
| Google Scholar |
Lu C, Tian H (2017) Global nitrogen and phosphorus fertilizer use for agriculture production in the past half century: shifted hot spots and nutrient imbalance. Earth System Science Data 9, 181-192.
| Crossref | Google Scholar |
Marschner P, Solaiman Z, Rengel Z (2007) Brassica genotypes differ in growth, phosphorus uptake and rhizosphere properties under P-limiting conditions. Soil Biology and Biochemistry 39, 87-98.
| Crossref | Google Scholar |
Mat Hassan H, Marschner P, McNeill A, Tang C (2012) Growth, P uptake in grain legumes and changes in rhizosphere soil P pools. Biology and Fertility of Soils 48, 151-159.
| Crossref | Google Scholar |
McDowell RW, Condron LM (2004) Estimating phosphorus loss from New Zealand grassland soils. New Zealand Journal of Agricultural Research 47, 137-145.
| Crossref | Google Scholar |
McDowell RW, Condron LM, Stewart I (2016) Variation in environmentally- and agronomically-significant soil phosphorus concentrations with time since stopping the application of phosphorus fertilisers. Geoderma 280, 67-72.
| Crossref | Google Scholar |
McDowell R, Dodd R, Pletnyakov P, Noble A (2020) The ability to reduce soil legacy phosphorus at a country scale. Frontiers in Environmental Science 8, 6.
| Crossref | Google Scholar |
Menezes-Blackburn D, Giles C, Darch T, George TS, Blackwell M, Stutter M, Shand C, Lumsdon D, Cooper P, Wendler R, Brown L, Almeida DS, Wearing C, Zhang H, Haygarth PM (2018) Opportunities for mobilizing recalcitrant phosphorus from agricultural soils: a review. Plant and Soil 427, 5-16.
| Crossref | Google Scholar | PubMed |
Murphy J, Riley JP (1962) A modified single solution method for the determination of phosphate in natural waters. Analytica Chimica Acta 27, 31-36.
| Crossref | Google Scholar |
Nash DM, Haygarth PM, Turner BL, Condron LM, McDowell RW, Richardson AE, Watkins M, Heaven MW (2014) Using organic phosphorus to sustain pasture productivity: a perspective. Geoderma 221–222, 11-19.
| Crossref | Google Scholar |
Nuruzzaman M, Lambers H, Bolland MDA, Veneklaas EJ (2006) Distribution of carboxylates and acid phosphatase and depletion of different phosphorus fractions in the rhizosphere of a cereal and three grain legumes. Plant and Soil 281, 109-120.
| Crossref | Google Scholar |
Oburger E, Jones DL (2018) Sampling root exudates – mission impossible? Rhizosphere 6, 116-133.
| Crossref | Google Scholar |
Pearse SJ, Veneklaas EJ, Cawthray G, Bolland MDA, Lambers H (2007) Carboxylate composition of root exudates does not relate consistently to a crop species’ ability to use phosphorus from aluminium, iron or calcium phosphate sources. New Phytologist 173, 181-190.
| Crossref | Google Scholar | PubMed |
Perrott KW (1992) Utilisation of inorganic and organic soil phosphorus in a hill country soil. Proceedings of the New Zealand Grassland Association 54, 65-69.
| Crossref | Google Scholar |
Possinger AR, Byrne LB, Breen NE (2013) Effect of buckwheat (Fagopyrum esculentum) on soil-phosphorus availability and organic acids. Journal of Plant Nutrition and Soil Science 176, 16-18.
| Crossref | Google Scholar |
Randhawa PS, Condron LM, Di HJ, Sinaj S, McLenaghen RD (2005) Effect of green manure addition on soil organic phosphorus mineralisation. Nutrient Cycling in Agroecosystems 73, 181-189.
| Crossref | Google Scholar |
Raymond NS, Gómez-Muñoz B, van der Bom FJT, Nybroe O, Jensen LS, Müller-Stöver DS, Oberson A, Richardson AE (2021) Phosphate-solubilising microorganisms for improved crop productivity: a critical assessment. New Phytologist 229, 1268-1277.
| Crossref | Google Scholar | PubMed |
Richardson AE, Hocking PJ, Simpson RJ, George TS (2009) Plant mechanisms to optimise access to soil phosphorus. Crop & Pasture Science 60, 124-143.
| Crossref | Google Scholar |
Sattari SZ, Bouwman AF, Giller KE, van Ittersum MK (2012) Residual soil phosphorus as the missing piece in the global phosphorus crisis puzzle. Proceedings of the National Academy of Sciences 109, 6348-6353.
| Crossref | Google Scholar |
Schefe CR, Watt M, Slattery WJ, Mele PM (2008) Organic anions in the rhizosphere of Al-tolerant and Al-sensitive wheat lines grown in an acid soil in controlled and field environments. Soil Research 46, 257-264.
| Crossref | Google Scholar |
Shi S, Condron L, Larsen S, Richardson AE, Jones E, Jiao J, O’Callaghan M, Stewart A (2011a) In situ sampling of low molecular weight organic anions from rhizosphere of radiata pine (Pinus radiata) grown in a rhizotron system. Environmental and Experimental Botany 70, 131-142.
| Crossref | Google Scholar |
Shi S, Richardson AE, O’Callaghan M, DeAngelis KM, Jones EE, Stewart A, Firestone MK, Condron LM (2011b) Effects of selected root exudate components on soil bacterial communities. FEMS Microbiology Ecology 77, 600-610.
| Crossref | Google Scholar | PubMed |
Shi S, Richardson AE, O’Callaghan M, Firestone M, Condron L (2013) Challenges in assessing links between root exudates and the structure and function of soil microbial communities. In ‘Molecular microbial ecology of the rhizosphere, Vol. 1’. (Ed. FJ de Bruijn) pp. 125–135. doi:10.1002/9781118297674.ch11
Teboh JM, Franzen DW (2011) Buckwheat (Fagopyrum esculentum Moench) potential to contribute solubilized soil phosphorus to subsequent crops. Communications in Soil Science and Plant Analysis 42, 1544-1550.
| Crossref | Google Scholar |
Veneklaas EJ, Stevens J, Cawthray GR, Turner S, Grigg AM, Lambers H (2003) Chickpea and white lupin rhizosphere carboxylates vary with soil properties and enhance phosphorus uptake. Plant and Soil 248, 187-197.
| Crossref | Google Scholar |
Vu DT, Tang C, Armstrong RD (2008) Changes and availability of P fractions following 65 years of P application to a calcareous soil in a Mediterranean climate. Plant and Soil 304, 21-33.
| Crossref | Google Scholar |
White JGH, Jarvis P, Lucas RJ (1995) Fertiliser requirements of Russell lupins. Proceedings of the Agronomy Society of New Zealand 25, 87-90.
| Google Scholar |
Wouterlood M, Cawthray GR, Scanlon TT, Lambers H, Veneklaas EJ (2004) Carboxylate concentrations in the rhizosphere of lateral roots of chickpea (Cicer arietinum) increase during plant development, but are not correlated with phosphorus status of soil or plants. New Phytologist 162, 745-753.
| Crossref | Google Scholar | PubMed |
Zhang FS, Ma J, Cao YP (1997) Phosphorus deficiency enhances root exudation of low-molecular weight organic acids and utilization of sparingly soluble inorganic phosphates by radish (Raghanus satiuvs L.) and rape (Brassica napus L.) plants. Plant and Soil 196, 261-264.
| Crossref | Google Scholar |