Effects of varying levels of nutrient inputs to coastal marine systems: a case study of a semi-enclosed bay influenced by a large urban population
Gregory P. Jenkins
A
B
C
Abstract
Port Phillip Bay (PPB) is the largest marine bay on the Australian coast and is the site of Australia’s second largest city, Melbourne. A major environmental study in the 1990s recommended a reduction in the nitrogen (N) input to the bay. Subsequently, improvements to sewage treatment efficiency in the 2000s coincided with the longest and most severe drought in recorded history, resulting in N inputs dropping by more than half in the 2000s compared with the 1990s. Here we review studies conducted over the past 30 years to understand the effects of varying nutrient levels on the ecology of PPB. Studies showed that PPB is an N limited system both in time and space. Biological productivity in PPB was markedly affected by reduced N loads during the drought, resulting in declines in seagrass and kelp cover, as well as benthic fish biomass. Overall, research has shown that while setting conservative limits on N input will effectively negate the risk of widespread eutrophication, there will likely be a tradeoff in reduced bay productivity. Managers will need to consider both sides of this equation when managing the load of N entering PPB, and potentially other marine systems around the world.
Keywords: denitrification, drought, ecology, eutrophication, nitrogen, nutrients, Port Phillip Bay, productivity.
Introduction
The input of nutrients to the coastal zone is a major factor influencing the characteristics of marine ecosystems (Cloern et al. 2016). Factors that can influence the input of nutrients to coastal zones include droughts and floods; changes in treated water quality or quantity from sewage treatment plants (STPs); alterations to catchment use including agricultural runoff, urbanisation and climate change; and changes to atmospheric deposition caused by human activities (Glibert 2017; Malone and Newton 2020; Rozemeijer et al. 2021; Zhao et al. 2021).
Anthropogenic changes to nutrient inputs to coastal systems is a major issue worldwide, particularly in relation to increasing nutrient inputs and eutrophication of the coastal zone (Howarth 2008; Lemley et al. 2019; Malone and Newton 2020). An excess of nutrients, such as nitrogen (N) and phosphorus (P) added to the system by activities such as agriculture and discharge of treated water from STPs, can lead to increased phytoplankton productivity, which in turn results in hypoxia that can lead to anoxia and associated environmental degradation (Whitall et al. 2007; Howarth 2008). It is generally agreed that N is the primary cause of eutrophication in marine systems (Howarth 2008), and anthropogenic production of dissolved inorganic nitrogen (DIN) is expected to double by 2050 compared with the 1990s (Malone and Newton 2020).
Conversely, some anthropogenic activities can lead to decreases in nutrient loads to coastal systems, such as dam construction and water diversion that leads to nutrient trapping and reduced freshwater flows (Humborg et al. 2000; Oczkowski et al. 2009; Glibert 2017; Broadley et al. 2020; Maavara et al. 2020b), and improved sewage treatment (Oviatt et al. 2017; Shim and Yoon 2021). In contrast to the situation of excess nutrients and eutrophication, there can also be negative consequences of too few nutrients entering coastal systems in relation to supporting biological productivity, including important industries such as fisheries and aquaculture (Oczkowski et al. 2009; Broadley et al. 2020). An example of this was the highly productive coastal Mediterranean fishery off the Nile River delta that collapsed after the completion of the Aswan High Dam on the Nile which led a dramatic reduction in nutrient inputs to the oligotrophic coastal system (Oczkowski et al. 2009).
In this review, we undertake a case study of the effect of varying nutrient levels on the ecology of Port Phillip Bay (PPB), the largest marine bay on the Australian coast. PPB is largely isolated from coastal waters with a water residence time of over 12 months, and the bay receives most of the treated water from the wastewater treatment plant that services approximately half (~2.5 million people) of the city of Melbourne. PPB supports important fisheries and aquaculture industries as well as providing amenity for the population of Melbourne.
A major environmental study was conducted by the Commonwealth Scientific and Industrial Research Organisation (CSIRO) in the early to mid-1990s (Harris et al. 1996) which led to the recommendation of a reduction in N input. This led to sewage treatment upgrades in the early 2000s which coincided with the Millennium Drought (1997–2009). This was the longest and most severe drought on record in southern Australia (Ummenhofer et al. 2009). Thus, there have been major changes to nutrient inputs to PPB on a decadal scale over the past 30 years. Here we review the body of research undertaken over this period that illustrates the profound effects changes to nutrient inputs can have on an important marine system. Based on the review, we make recommendations to managers in regard to the effect of varying nutrient levels on the bay ecosystem and how this may be managed into the future. The outcomes of the review also have strong relevance to other coastal marine systems subject to varying nutrient regimes.
System description
Physical setting
Port Phillip Bay has a surface area of 1930 km2, volume of 2.63 × 1010 m3, an average depth of 14 m, and a maximum depth in the central basin of 24 m (Fig. 1). The narrow entrance at Port Phillip Heads and a large area of shallow channelised sand banks (The Great Sands) essentially isolate the ocean from the inner bay basin (Fig. 1) resulting in long residence times of around 12–16 months (Harris et al. 1996) and a narrow tidal range of less than 1 m. Medium-sized sand dominates over the large sand banks near the entrance, while soft mud predominates in the 18–24 m deep central region. Water temperature varies from approximately 11°C in winter up to 23°C in summer. Salinity is typically around ocean values of 35–36, but cycles at decadal time scales from hyposaline to hypersaline due to slow shifts in the evaporation and rainfall balance (Lee et al. 2012). The major city of Melbourne (5.2 million people) is situated around the lower reaches of the Yarra River in the north-east of the bay (Fig. 1).
Hydrodynamics
Port Phillip Bay is highly variable in a hydrodynamic sense, consisting of three broad hydrodynamic zones (Black et al. 1993). The narrow entrance is characterised by fast tidal currents that create an ebb and flood tidal jet each half tidal cycle. Over the sands region in the southern segment of the bay, tidal currents remain strong (up to 1 m s−1) over the banks and channels. Wind-driven circulation in this region is relatively unimportant, and the low-frequency circulation consists primarily of the tidal cycle residual currents and the low-frequency currents associated with barometric pressure driven oscillations, wind set-up, and coastal trapped waves in Bass Strait entering Port Phillip Bay (Black et al. 1993). Beyond the sands, tidal currents in Port Phillip Bay are weak and net circulation is primarily induced by direct local wind-forcing. Thus, there is wide variability in the absolute current strengths and in the dominant mechanisms forcing the circulation. Currents also show considerable vertical stratification, especially where currents are wind-driven rather than tidal (Black et al. 1993). Corio Bay and Swan Bay (Fig. 1) are relatively isolated from the main bay in relation to hydrodynamics.
Nutrient sources
Phosphorus has been shown to not be a limiting nutrient in PPB (Harris et al. 1996) and is therefore not considered further in this review. The dominant sources of nutrients entering the bay are the surface water catchment inputs and the major sewage treatment plant for the city of Melbourne, the Western Treatment Plant (WTP), on the north-western coast of the bay (Fig. 1). In the 1990s over 85% of the N entering PPB was estimated to come from these two sources (Harris et al. 1996). Over 70% of the surface water catchment inputs emanate from the Yarra River (Harris et al. 1996) that enters the bay in the north-east (Fig. 1). In the mid-1990s, the WTP was estimated to contribute about 55% of all N inputs into PPB (Harris et al. 1996), although this contribution has declined to about 45% due to improved wastewater treatment (Hirst et al. 2016). Another nutrient of ecological importance in PPB is silicon (Murray and Parslow 1999). In contrast to the situation with N, the input of dissolved silicon is higher from the catchment than the WTP (Murray and Parslow 1999).
Construction of the WTP moved a major N discharge to a different part of PPB where it is diffused and circulated in a very different way to the Yarra River, the major catchment input (Jenkins et al. 2015; Jenkins and Black 2021). The Yarra River plume and the WTP footprint are quite spatially distinct and influence different habitats and processes within the bay (Jenkins et al. 2015; Hirst et al. 2016; Jenkins and Black 2021). Based on measurements of N isotope ratios in benthic macroalgae deployed at sites throughout PPB, Parry and Black (2023) estimated that the footprint of N sourced from the WTP extends along the north-western shoreline of PPB and for approximately 10–15 km offshore. This distribution was similar to the distribution of DIN originating from the WTP estimated by hydrodynamic and dispersal modelling (Parry and Black 2023).
Other sources of N entering PPB include atmospheric inputs (wet and dry deposition of NOx and N-fixation), groundwater inputs and seasonal influxes from Bass Strait (Harris et al. 1996; Cook et al. 2015; Hirst et al. 2016; Wong et al. 2022; Parry and Black 2023). Atmospheric deposition is ~1000 t N per year, while groundwater inputs are estimated to be 25–82 t N per year (Harris et al. 1996), mostly from the Werribee region (Wong et al. 2022). There have been no estimates of total inputs to PPB from N-fixation; however, scaling the average N-fixation rates measured within seagrass beds of 23 μmol m−2 h−1 to the total area of seagrass within the bay of ~68 km2 (Blake and Ball 2001; Cook et al. 2015) gives a first order estimate of 200 t N per year. In the south of PPB, isotope tracing suggests that a variable but potentially significant fraction of N in seagrass is derived from exchange with Bass Strait (Hirst et al. 2016). However, the net exchange with Bass Strait is an export of ~1000 t N per year (Harris et al. 1996; Wong et al. 2022).
The input of nutrients into PPB is highly variable over time, particularly in relation to periods of drought and flood. For example, from 1997 to 2009, southern Australia experienced the longest and most severe drought on record, the Millennium Drought (Ummenhofer et al. 2009; Hirst et al. 2016). Freshwater flows from the catchment declined substantially from 1997 onwards (Fig. 2), and this coincided with reduced N loads to PPB (Hirst et al. 2016). Reduced freshwater flows during the drought also led to a substantially lower N load entering PPB from the WTP (Fig. 2) (Jenkins et al. 2015). Overall, N inputs to PPB declined 56% for the period 1998–2009 compared with 1991–1997 (Jenkins et al. 2015; Hirst et al. 2016).
Annual total N contribution to Port Phillip Bay from WTP and catchments from 1990 to 2009. Source: Melbourne Water and EPA E2Port catchment model (from Jenkins et al. 2015).
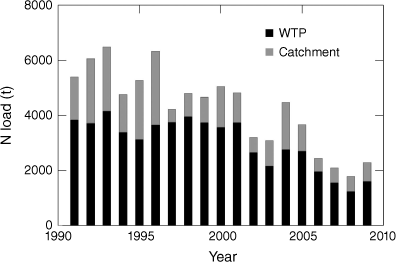
Habitats
There is a range of habitats that are of high importance to ecological processes and fauna. The deeper central basin of PPB is characterised by muds and fine silts and has a high diversity of benthic invertebrates (Wilson et al. 1999). Seagrass beds occur in shallower areas that are sheltered from the predominant south-westerly winds (Fig. 1), including the Geelong Arm, Corio Bay and Swan Bay (Hirst et al. 2017). Rocky reefs predominantly occur on the coastal fringe of PPB, mainly in the north and east, and rocky reef is the dominant habitat in the entrance area (Fig. 1). Attached macroalgae grows on rocky reefs and other hard substrates but historically there have also been significant areas of unattached ‘drift’ macroalgae, particularly in the Geelong Arm (Chidgey and Edmunds 1997).
History
The Yarra catchment has been dammed with extensive water extraction (approximately 50%) since European settlement in the mid-1800s and currently supplies approximately 70% of the drinking water for the greater city of Melbourne. Water diversion is likely to have had a marked effect on the nutrient inputs over that period, potentially reducing the input of N and silicon to PPB (Maavara et al. 2020a; Shim and Yoon 2021). Over the same period, increasing use of agricultural fertilisers would have been expected to add to the level of N entering PPB. However, it should be noted that most of the Yarra catchment is ‘protected’, that is, densely forested rather than agricultural farmland.
The sewage system for the city of Melbourne was built at the end of the 1800s and was connected to the ‘farm’ at Werribee for land filtration. Beginning in the mid-1930s, lagoon treatment has now replaced land filtration. After recommendations from a major environmental study of PPB in the mid-1990s (Harris et al. 1996), a major upgrade to the WTP was completed in 2004 to meet limits set on N input to the bay of 3100 t per year from the plant (EPA 2021).
At the time of colonial settlement around PPB in the early 1800s the seabed in the bay was covered with extensive shellfish reefs, primarily composed of native flat oysters, Ostrea angasi, and blue mussel, Mytilus edulis galloprovincialis (Ford and Hamer 2016). However, today these beds have largely disappeared, primarily due to destructive dredge fishing practices and long periods of large-scale removal of biomass (overharvesting) (Ford and Hamer 2016).
Effects of varying nutrient levels on key values
Nutrient recycling and denitrification
A critical process that allows PPB to receive significant quantities of N from the catchment and the WTP and yet maintain an oligotrophic to mesotrophic environment without tipping into eutrophic conditions is denitrification (Harris et al. 1996). Denitrification is a process whereby sediment microbes in aerobic conditions convert remineralised ammonia to nitrite and then nitrate, followed by the conversion of nitrate to N2 gas under anaerobic conditions in a tightly coupled process (Harris et al. 1996; Berelson et al. 1998; Marshall et al. 2021, 2023a, 2023b)
Denitrification efficiency is the percentage of the total inorganic N released from the sediments as di-nitrogen gas (N2). Average bay-wide denitrification efficiency (DE) estimates for PPB of greater than 60% are comparatively high by global standards (Berelson et al. 1998; Eyre and Ferguson 2009). Physical factors attributed to the high DE within PPB include the high benthic area to total volume ratio, the large proportion of muddy fine-grained sediments, a relatively low external carbon input, the slow exchange rate with the open ocean, high sediment-water mixing, and bio irrigation (Harris et al. 1996; Berelson et al. 1998, 1999; Murray and Parslow 1999; Holdgate et al. 2001). A key factor is long residence time (more than 400 days) in PPB as denitrification increases with increasing residence time (Seitzinger et al. 2006). This is because the longer a N atom spends cycling between the sediment and water column, the more chance there is of it being denitrified. In PPB the entire water column is predicted to cycle through the surface sediments at least 1−2 times before the water is exchanged with the open ocean (Harris et al. 1996; Berelson et al. 1999).
There is a dome-shaped relationship between sediment N loading and DE (Harris et al. 1996). At very low organic N loads, oxygen consumption is low, the oxygenated zone is deep, and most organic matter is remineralised in the upper part of the oxygenated zone. Most of the resulting ammonia is converted to nitrate, but very little of this nitrate encounters low enough oxygen levels for denitrification to occur. DE is therefore low, and inorganic N is recycled to the water column in the form of nitrate with high efficiency. At very high organic loads, the oxygenated zone becomes extremely thin, and the bottom water itself may become anoxic. There is little or no chance for ammonia released by remineralisation to be nitrified, and almost all is returned to the water column as ammonia. Denitrification cannot occur because nitrate is not produced.
Denitrification is considered to be a key process in Port Phillip Bay and was monitored throughout the Millennium Drought and the subsequent high rainfall and flooding at the end of the drought associated with the La Niña climate phase in 2010–2011 (Longmore and Nicholson 2012). The total N entering PPB from the catchment and WTP ranged between approximately 2000 and 6000 t per year over the period (Longmore and Nicholson 2012; Jenkins et al. 2015). Throughout the period, DE remained high, indicating that DE is robust over this range of variability in N loading (Longmore and Nicholson 2012). However, modelling has indicated that if the annual N load to PPB were to increase to 14,000–17,000 t per year then DE would be reduced to the point where it ceased to be effective in controlling eutrophication (Harris et al. 1996). Conversely, if the N load were to drop to very low levels, then DE would also be expected to decline (Harris et al. 1996; Longmore and Nicholson 2012). However, in this case the reduction in DE would be beneficial, because the return of N to the water column would help support biological production in the face of low nutrient concentrations.
It was estimated that in the early 1990s, when total N load was about 6000 t per year (WTP contribution approximately 4000 t per year), that denitrification efficiency was near its peak or slightly beyond the peak (Harris et al. 1996). This led to a recommendation to management that the overall annual N load to PPB be reduced by 1000 t per year (Harris et al. 1996).
Phytoplankton (including blooms)
Phytoplankton make the largest contribution to primary production in PPB (approximately 60%), with turnover times of about 3 days (Murray and Parslow 1999). Phytoplankton blooms are not common in PPB, and for those blooms that do occur, it is the diatom species that are typically responsible (Jenkins and Black 2019). The most abundant and potentially bloom-forming diatoms in PPB are Skeletonema costatum, Chaetoceros spp., and Pseudo-nitzschia spp. (Harris et al. 1996; Jenkins and Black 2019). Blooms of S. costatum tend to occur over summer in the north-east of PPB following high rainfall events that deliver nutrients from the Yarra catchment (Jenkins and Black 2021). For example, an increase in phytoplankton blooms, predominantly related to diatoms in the north-east of PPB, was recorded at the end of the Millennium Drought when heavy rainfall events occurred over summer (EPA 2012). The localisation of diatom blooms to this area of PPB would partly relate to the higher concentrations of dissolved silicon in the Yarra catchment compared with the outfalls of the WTP (Black et al. 2016b). Silicon can become the limiting nutrient in blooms, even though N concentrations are much less in PPB waters, because of the relatively slow re-mineralisation and dissolution of silicate (Black et al. 2016b).
Scenarios of changes to nutrient inputs to PPB have been simulated using an Nutrient Phytoplankton Zooplankton (NPZ) model (Jenkins and Black 2021). Scenario modelling was based on the 2016–2017 fiscal year (1 July to 30 June), a year of close to average rainfall, when the N load from the WTP was approximately 2850 t. One scenario addressed a 50% increase (4275 t), and another a 50% decrease (1425 t) in N load from the WTP, while keeping catchment N loads stable and both catchment and WTP freshwater flows unchanged.
It was hypothesised that the increased and decreased N scenarios would have had a minimal effect on the summer diatom bloom, because diatom blooms are primarily limited by the amount of dissolved silicon coming from the catchment (Jenkins and Black 2021). The effect of the two scenarios was larger for flagellates (including dinoflagellates), with increases in some peaks in flagellate abundance of up to 100% under the higher N scenario, and decreases in flagellate abundance of up to 50% under the lower N scenario (Jenkins and Black 2021). The change in flagellates led to a corresponding change in the zooplankton that are the preferred prey for larvae of a key fishery species, snapper, Chrysophrys auratus (Black et al. 2016a; Jenkins and Black 2021), potentially benefiting PPB fisheries in the higher N scenario. Conversely, many of the toxic phytoplankton species in PPB are flagellates and dinoflagellates, and the higher N scenario could have an impact on the shellfish aquaculture industry in PPB through greater exceedances of concentration limits for harmful species (EPA 2012).
Microphytobenthos
In PPB, the total amount of N contained in the microphytobenthos, dominated by diatoms, is about twice that contained in the phytoplankton (Harris et al. 1996), and makes a substantial contribution to primary production, amounting to about half of the phytoplankton production (Murray and Parslow 1999). Microphytobenthos have been suggested to have both positive and negative impacts on denitrification. It has been shown that microphytobenthos reduce the rates of denitrification by competing with denitrifying bacteria for N (Risgaard-Petersen 2003; Risgaard-Petersen et al. 2004). By contrast, Harris et al. (1996) concluded that microphytobenthos can increase the effective DE of the sediment in PPB by intercepting DIN which would otherwise escape from the sediment, and converting it into organic matter where it re-enters the sediment microbial loop. Modelling indicates that the activity of microphytobenthos in PPB may increase DE by a factor of two, and that increasing N loads could see this benefit quickly lost due to the shading effects of the phytoplankton blooms (Harris et al. 1996). Unfortunately, long-term monitoring of microphytobenthos has not been conducted in PPB so there is no empirical data about temporal variability in relation to N inputs and how this feeds back into DE.
Attached macroalgae
Aerial photographs since the 1930s and field studies since the 1960s have shown that there has been a major decline in the cover of kelp, Ecklonia radiatia, on the reefs of northern PPB since the 1980s (Carnell and Keough 2019). The declines were correlated with increased water temperature and decreased rainfall (Carnell and Keough 2019). Low nutrients associated with decreased rainfall would likely be a key factor as kelp productivity has a dome-shaped relationship with nutrient concentrations (Dayton et al. 1999), with low nutrient levels, such as occurred in the Millennium Drought, leading to reduced algal productivity (Carnell and Keough 2019).
Recent studies have supported the hypothesis that kelp growth in PPB is N limited. Experimental studies indicated that nutrients were not limiting growth at a site near the Yarra mouth (Kriegisch et al. 2016), but were limiting growth at a site on the south-east coast of the bay (Kriegisch et al. 2019). Furthermore, exclusion of grazers from plots at a number of reef sites showed the kelp growth rates were higher towards the north of PPB where nutrient levels were higher (Kriegisch et al. 2020). Overall, the results indicated that growth of kelp becomes increasingly N limited with distance from the major nutrient inputs.
Other factors are also likely to have influenced the decline in kelp. After the initial loss of kelp from the 1980s to the early 2000s, there was a major increase in urchins, Heliocidaris erythrogramma, in the mid-2000s (see below). A further factor potentially contributing to the decline in native kelp is competition with Undaria pinnatifida, the non-native kelp introduced to PPB in the 1980s (Carnell and Keough 2014).
Drift macroalgae
A significant area of live, unattached (drift) macroalgae occurs in the north of PPB, particularly along the north and west coast (Light and Woelkerling 1992; Chidgey and Edmunds 1997; Valero-Rodríguez et al. 2024). Community composition in shallower areas tends to be a mixture of green, red and brown algal species, while deeper areas (10–15 m) are dominated by red algae, and in particular, monospecific stands of Botryocladia sonderi (Chidgey and Edmunds 1997; Valero-Rodríguez et al. 2024). Analysis of stable isotopes of N indicate that drift algae have a strong dependence on N sourced from the WTP (Valero-Rodríguez 2019). In contrast, the percentage N composition of drift algae was not related to proximity to nutrient inputs (Valero-Rodríguez et al. 2024). In the 20 years between the studies of the same sampling sites by Chidgey and Edmunds (1997) and Valero-Rodríguez et al. (2024) there was a major decline in the drift algae (Valero-Rodríguez et al. 2024). This decline may be linked to reduced N inputs to the bay over this period, particularly in relation to the Millennium Drought that occurred in the intervening period (Valero-Rodríguez et al. 2024). Other contributing factors may have been upgrades to sewage treatment that led to decreased ammonia in the treated water discharge, and also grazing by urchins, Heliocidaris erythrogramma, that increased markedly in abundance between the two survey periods (Valero-Rodríguez et al. 2024).
The accumulation of drift algae on sediments has been shown to have a strong effect on the N cycle and this is likely to vary temporally as macroalgae accumulate over spring and summer and then decay over autumn. Indeed, the reported effects of drift algae on denitrification are highly variable, and this most likely depends on the growth state of the algae. In the accumulation phase or perennial scenarios, macroalgae are a strong sink for N and are likely to dominate sediment N uptake over denitrification (Dalsgaard 2003) and high rates of denitrification have been observed (Chen et al. 2021). Indeed, within PPB, it has been shown that the presence of drift algae generally leads to an increase in denitrification efficiency when live drift algae are added to the sediment (Valero-Rodríguez 2019). As macroalgae begins to decay, it is likely that absolute rates of denitrification are stimulated by an increase in N availability. However, denitrification rates are likely to be low compared with ammonia release from the sediment (Viaroli et al. 1996; Trimmer et al. 2000; García-Robledo et al. 2013) due to low localised oxygen concentrations which reduce denitrification efficiency as discussed previously. Within permeable sediments it has been shown that drift algae can also stimulate denitrification as well as dissimilatory nitrate reduction to ammonium, potentially enhancing both the recycling and removal of N (Bourke et al. 2014; Wong et al. 2021). On this basis, we speculate that during periods of accumulation or where there is a perennial presence of drift algae, it acts as a strong sink for N, and possibly stimulates denitrification. Where excessive growth accumulates as wrack in nearshore areas, it is likely that there will be a large release of N and limited denitrification. This is consistent with observations of very high ammonium and anoxia in areas of nearshore drift algae accumulation (P. L. M. Cook unpubl. data).
Seagrass
The total N pool and productivity of seagrass in PPB is low compared with the plant groups considered above. However, seagrass provides important ecosystem services, such as reduced erosion and improved water quality, as well as providing a nursery habitat for a range of fish and invertebrate species (Barbier 2017; Gaylard et al. 2020).
Seagrass predominantly occurs on the western side of PPB where the coast is protected from the dominant south-westerly winds (Hirst et al. 2017). Seagrasses in PPB utilise a number of sources of N depending on their location (Hirst et al. 2016). Stable isotope studies showed that seagrass beds along the north-west coast of PPB were dominated by sewage derived N; seagrass beds in Swan Bay and some parts of Corio Bay were dominated by N fixation and recycling; while seagrass beds in the central and southern areas of the main bay received N from a variety of sources, including the catchment (Hirst et al. 2016).
There was a major decline in seagrass in the central and southern parts of the main bay corresponding with the Millennium Drought that occurred after a long (decades) period of seagrass expansion (Ball et al. 2014) coinciding with a multi-decadal wet period (Figs 3, 4). Hirst et al. (2016) hypothesised that this decline related to a reduction in N input from the catchment over the period of the drought, affecting seagrass in areas that partly depended on N from this source. This hypothesis was supported by a field experiment showing that at a site in the main bay, seagrass growth was N limited while at sites in Swan Bay and near the WTP seagrass growth was not N limited (Hirst and Jenkins 2017). These results were consistent with earlier experimental studies showing that seagrass growth in central and southern PPB was N limited (Bulthuis et al. 1992).
Mean annual rainfall anomaly for Victoria 1935–2012 with changes in % maximum seagrass cover at three locations: Blairgowrie (blue line), St Leonards (red line) and Bellarine Bank (green line) overlaid. Dashed lines represent WWII drought (1937–1945) and Millennial Drought (1997–2009) (from Jenkins et al. 2015).
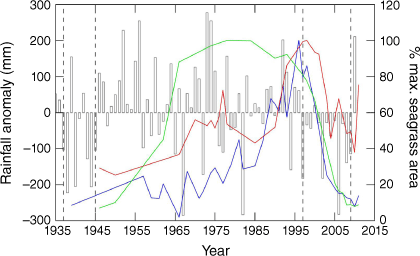
Historical sequence of aerial photography taken along a section of coastline on the Bellarine Bank (southern coast of the Geelong Arm) in 1946, 1978, 2000 and 2010 showing changes to seagrass cover (from Jenkins et al. 2015).
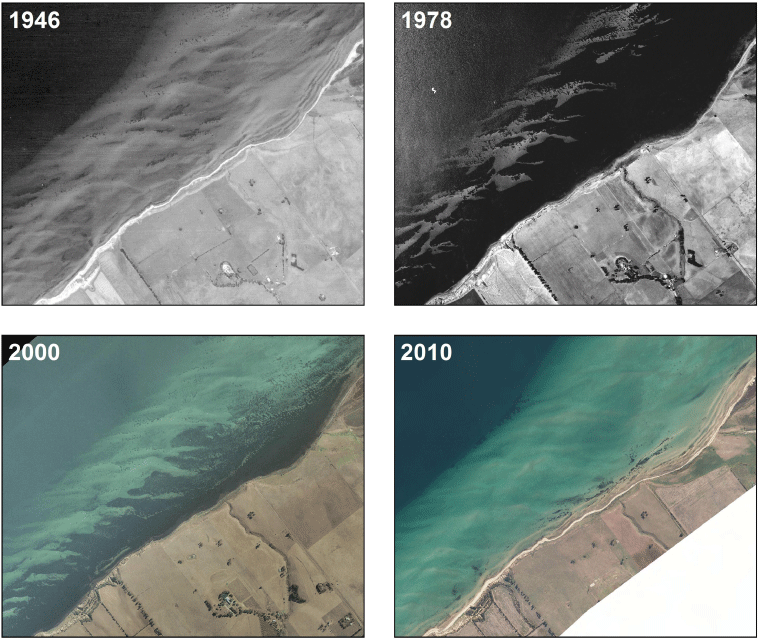
Overall, studies have shown that over a significant area of PPB, seagrass growth is N limited, and changes to seagrass cover are particularly sensitive to variation in N inputs from the catchment.
Urchins
The loss of kelp, Ecklonia radiata, from reefs in northern PPB from the 1980s to the early 2000s was most likely associated with increasing water temperature and decreasing nutrients (Carnell and Keough 2019). However, from the mid-2000s during the Millennium Drought, there was a rapid increase in sea urchin, Heliocidaris erythrogramma, populations, leading to overgrazing of kelp and the formation of urchin barrens (Carnell and Keough 2019). Grazing by urchins can lead to ‘an alternative state’ where reef habitat in PPB changes from kelp dominated to sediment-trapping turf algae dominated (Reeves et al. 2018).
Experimental studies have shown that there is a threshold density of urchins for overgrazing and barren formation to occur (Kriegisch et al. 2016), and above these densities, the grazing pressure from urchins overwhelms increased kelp productivity from the bottom-up effect of N supplementation (Kriegisch et al. 2019). In field studies, higher N levels led to higher kelp productivity in the north of PPB; however, these were also the areas of largest increase in urchin abundance, and the greatest impact of grazing on kelp (Kriegisch et al. 2020). Fragmentation of kelp beds in PPB has been found to reduce resistance to overgrazing by urchins, with patch extinction occurring when perimeter-area ratio exceeds a critical value of ~0.7 (Reeves et al. 2022)
Fish
A long-term trawl survey of benthic and demersal fish in PPB recorded a major decline in fish biomass over the period of the Millennium Drought (1997–2010), with a 69% decline in fish biomass in the deeper centre of the bay and a 50% decline in fish biomass in intermediate depths (Parry and Hirst 2016). Most of the decline was attributed to the cumulative effects of reduced productivity during the 12-year drought (Parry and Hirst 2016). The decline was particularly strong for the sand flathead, Platycephalus bassensis, an economically important fishery species (Parry and Hirst 2016). This species had the highest biomass of any fish species in the trawl surveys from 1990 to 2000, but by 2010 its biomass had declined by up to 90% across all regions of the bay (Parry and Hirst 2016). The decline was attributed to a prolonged period of weak recruitment coinciding with the drought, which was directly linked to reduced catchment inputs over this period (Hirst et al. 2014; Parry and Hirst 2016). These conditions were thought to reduce the survival of larvae and subsequent recruitment of sand flathead (Hirst et al. 2014; Parry and Hirst 2016).
In contrast to most of the fish species surveyed in the trawl sampling, for snapper, another economically important fish species in PPB, there was a positive effect of the Millennium Drought, with strong recruitment years in 2001, 2004 and 2005 that led to a significant increase in the catch rate (Jenkins 2010). Both field (Murphy et al. 2012, 2013) and modelling (Black et al. 2016a) studies show that interannual variability in abundance of snapper larvae is related to the availability of their preferred prey, calanoid copepods of the genus Paracalanus (Fig. 5). Modelling studies showed that recruitment success in terms of larval survival was related to increased dominance of flagellates (including dinoflagellates) relative to diatoms in the plankton at the time of snapper spawning in the early summer (Black et al. 2016a). Dominance of diatoms is detrimental to snapper recruitment because diatoms in the diet have a negative impact on Paracalanus abundances (Jenkins and Black 2019). In years of high rainfall where loads of N and silicon from the catchment are increased, the plankton community becomes dominated by diatoms earlier in the seasonal cycle, resulting in poor survival of larval snapper and correspondingly poor recruitment (Black et al. 2016a). Conversely, years of very low rainfall also had a negative effect on snapper recruitment due to a lack of nutrients resulting in low phytoplankton concentrations leading to low copepod prey availability for larvae (Black et al. 2016a). Highest snapper recruitment occurred in years of low to intermediate rainfall and nutrient inputs, particularly when pulses of nutrients occurred in the spring period prior to snapper spawning (Black et al. 2016a).
Model predicted Paracalanus versus the concentration of snapper larvae from field collections over seven snapper spawning seasons from 2004–2005 to 2010–2011 (from Black et al. 2016a).
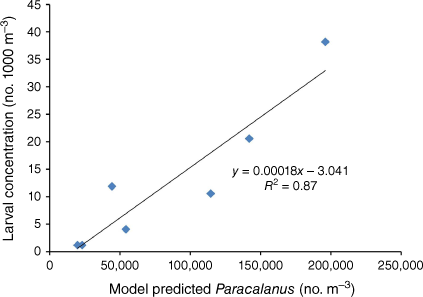
As mentioned above, scenario modelling indicated that a 50% increase in N load from the WTP (relative to 2016 levels) and no change to catchment N loads led to a greater ncrease in flagellates relative to diatoms, with a corresponding increase in Paracalanus abundance that could potentially benefit snapper recruitment (Black et al. 2016a; Jenkins and Black 2021). Conversely, a 50% reduction in N load from the WTP resulted in markedly lower concentrations of flagellates and in turn Paracalanus concentrations, with a potential negative effect on snapper recruitment (Black et al. 2016a; Jenkins and Black 2021). Moreover, under the low N load scenario, the peak in flagellate abundance shifted to after the snapper spawning season, potentially resulting in a mismatch between the presence of larvae and their Paracalanus prey (Jenkins and Black 2021).
Discussion
Ecological status under different levels of nutrient inputs
The improvements to sewage treatment in the early 2000s that resulted from recommendations of the PPB Environmental Study (Harris et al. 1996), together with the onset of the prolonged Millennium Drought in the late 1990s, saw a dramatic reduction in nutrient load from the WTP and catchment where the load approximately halved for a period of a decade compared with the 1990s (Jenkins et al. 2015; Hirst et al. 2016). The combined effects of better sewage treatment, increased water recycling and the Millennium Drought meant that the N load from the WTP dropped from approximately 4000 to 2000 t per annum (Fig. 2) between the two periods (Jenkins et al. 2015). This dramatic change provided the opportunity for researchers to document the effects of major changes in N load on the PPB ecosystem.
Research sampling prior to and during the Millennium Drought was able to document dramatic changes to the PPB ecosystem corresponding to the change in nutrient load, and indicated that the ecosystem of PPB was strongly N limited. The Millennium Drought period saw major declines in seagrass (Ball et al. 2014) and kelp (Carnell and Keough 2019) cover, together with a decline in demersal fish biomass (including a dramatic decline in sand flathead biomass) (Parry and Hirst 2016) and also an apparent major decline in drift algae in the Geelong Arm (Chidgey and Edmunds 1997; Valero-Rodríguez 2019). This strong N limitation is consistent with evidence of a spatial gradient of N limitation for seagrass (Hirst and Jenkins 2017) and kelp (Kriegisch et al. 2020) with distance from the main nutrient sources.
In contrast to the declines in seagrass, kelp, drift algae and demersal fish, there was a rapid increase in sea urchin populations that led to overgrazing of the remaining kelp and the formation of urchin barrens on reefs (Carnell and Keough 2019). One possible scenario is that before the drought, urchin populations were more dispersed and were using the extensive drift algal beds as a primary food resource. However, with the decline in drift algae associated with nutrient load reduction, urchins may have aggregated onto reefs to find food resources, leading to overgrazing of kelp.
Another species that showed an increase over the Millennium Drought was the snapper, where two strong recruitment years occurred that led to an increase in snapper abundance over the following years (Jenkins 2010). These years were likely associated with low to moderate inflows and nutrient inputs from the catchment, that resulted in the production of preferred copepod prey, and with the timing of plankton productivity coinciding with the snapper spawning season (Black et al. 2016a).
In summary, the reduction in nutrient load entering PPB associated with the Millennium Drought resulted in a marked decline in productivity of seagrass, kelp, drift algae and demersal fish in the bay due to strong N limitation. It also potentially resulted in the adverse effect of increasing urchin populations and formation of urchin barrens. One positive effect, however, was that some drought years were highly favourable for snapper recruitment.
Historical baseline
There have been no published estimates of pre-European N loads to Port Phillip Bay. The creation of dams and water extraction and diversion since European settlement would have reduced nutrient loads from the catchment through nutrient trapping and elimination, and reduced freshwater flows (Humborg et al. 2000; Glibert 2017; Maavara et al. 2020b). Conversely, clearing for agriculture and urbanisation in the lower catchment has most likely increased water runoff and nutrient loads. Runoff from urban-dominated areas have an N yield ~4.5 times that of forest/scrubland (Drewry et al. 2006). In the Lake Wellington catchment in eastern Victoria it has previously been estimated that current loads of total N are 1.8 times higher than prior to European arrival (Grayson et al. 2001). Overall, while dam construction and water diversion are likely to have led to a significant reduction in nutrient loads post-settlement, this would be unlikely to have offset increases in load from sewage treatment and urban runoff. Therefore, we consider the most likely scenario is that pre-settlement nutrient loads were lower than current loads, and conditions in PPB were more oligotrophic.
Without any historical data on nutrient recycling in PPB, we can only speculate how the system functioned prior to European arrival based on contemporary knowledge of habitat types. It seems likely that a large fraction of phytoplankton productivity in PPB was processed through the filter-feeding oyster and mussel reefs that previously were abundant in PPB (Ford and Hamer 2016), rather than the sediments as is presently the case. A recent meta-analysis of oyster impacts on sediment biogeochemistry showed that the presence of oysters significantly enhanced the recycling of ammonia, silicon and phosphorus, but that denitrification was also enhanced compared with bare sediments (Ayvazian et al. 2022). A re-analysis of their data for denitrification efficiency (where N2, NH4+ and NO3− fluxes were available) showed that denitrification efficiency in control sites was on average ~100%, compared with 50% at sites with oysters (P < 0.005, paired t test, log transformed data). In this scenario, more N would be returned to the water column, which is then taken up by phytoplankton, which are then rapidly removed again through filter feeding resulting in a lower phytoplankton biomass. This in turn would result in less sedimentation of phytoplankton to the sediment in the muddy centre of PPB which would lower sediment respiration and also denitrification rates and efficiency (as noted previously denitrification efficiency is highest at intermediate respiration rates). This would lead to a highly productive, but clearwater system driven by rapid nutrient recycling analogous to coral reefs. On this basis we speculate that PPB was able to maintain high oyster productivity, despite lower nutrient loads, and most likely subsidised by imported N from Bass Strait.
Speculating on a probable historical baseline for PPB becomes even more difficult considering that dam construction affects not only nutrient load, but also nutrient ratios (Humborg et al. 2000; Billen and Garnier 2007; Maavara et al. 2020a) and nutrient chemistry (Glibert 2017), both of which can affect the ecology of receiving waters. For example, more rapid depletion of Silicon over N in dams and reservoirs (Humborg et al. 2000; Billen and Garnier 2007) means that the phytoplankton in PPB before European settlement may have had a higher dominance of diatoms.
Future scenarios for nutrient inputs
Overall, climate models predict a decrease in rainfall and runoff for Victoria in the future, with most of the decrease occurring over the winter–spring period when most of the annual rainfall has typically occurred in the past (Chiew et al. 2017; Dey et al. 2019; Zheng et al. 2019; Rauniyar and Power 2020; Rauniyar and Power 2022). The mean value projected from climate models is a decline in mean annual runoff of 20% for far south-eastern Australia under a high emission scenario (Chiew et al. 2017; Zheng et al. 2019). These predictions come with significant uncertainty, however, with considerable divergence among different climate models (Chiew et al. 2017; Dey et al. 2019; Zheng et al. 2019; Rauniyar and Power 2020; Rauniyar and Power 2022).
Rainfall and runoff are also expected to be more variable under climate change in the future. Climate modelling indicates that there will be an increasing frequency of extreme El Niño events (Cai et al. 2014), which would correspond to an increased frequency of very dry weather events in south-eastern Australia. The frequency of extreme La Niña events is also expected to increase in the future under climate change (Cai et al. 2015), leading to more frequent high rainfall and flooding events in south-eastern Australia. Short duration extreme rainfall events are also expected to intensify (Dey et al. 2019; Zheng et al. 2019).
Apart from climate, the other major driver that could impact nutrient delivery to PPB in the future is population growth. Melbourne is projected to be the largest city in Australia by the mid-2030s (Australian Government 2023) with a projected population of between 9.3 and 13.8 million by 2071 (Australian Bureau of Statistics 2023). Significant development of sewage treatment infrastructure and efficiency will be required to maintain current nutrient loads from the WTP below the current load objective of 3100 t per year into the future based on these population projections. This need may be offset to some extent by diversion to other treatment plants that do not discharge into PPB, and also through the greater level of water recycling and reuse likely to occur in the future.
In summary, under climate change there may be a decrease in average runoff, although how this affects nutrient loads will also depend on trends in nutrient concentrations in the catchment. At the same time, nutrient input will become more variable, with more frequent swings between drought and flood. These changes will occur against a backdrop of increasing population in the greater Melbourne area, placing increasing pressure on sewage treatment infrastructure in terms of nutrient loads entering PPB from the WTP. Increasing population is also likely to lead to an expansion of urban boundaries that will affect nutrient inputs from the catchment.
Implications for managers
The PPB Environmental Study (PPBES) was conducted in the early- to mid-1990s with a primary focus on determining an acceptable level of nutrient inputs that would limit the risk of eutrophication (Harris et al. 1996). The study was conducted near the end of a relatively wet period that began in the late 1940s and did not focus on risks associated with the opposite issue: too few nutrients entering the bay to support productivity. At the time of the PPBES the annual N load entering PPB from the catchment and WTP was approximately 6000 t per year of which approximately 4000 t came from the WTP discharge (Fig. 2). The PPBES studies concluded that at that time the dentrification efficiency in PPB was near or slightly past the peak of a dome-shaped relationship with N load although modelling indicated that the annual N load to PPB would have to increase to 14,000–17,000 t for DE to be reduced to the point where it ceased to be effective in controlling eutrophication (Harris et al. 1996). To reduce the risk of eutrophication the PPBES recommendation to management was that the annual N load from the WTP be reduced by 1000 t per year (Harris et al. 1996), which led to the current annual limit of 3100 t for the WTP.
Upgrades to sewage treatment to reduce N load from the WTP in the early 2000s coincided with the Millennium Drought and resulted in a halving or more of N inputs to PPB. Research over the past three decades, including this drought period, has shown that there is strong N limitation of biological productivity in PPB, with declines in seagrass (Ball et al. 2014; Hirst et al. 2016; Hirst and Jenkins 2017), drift algae (Chidgey and Edmunds 1997; Valero-Rodríguez 2019), kelp (Carnell and Keough 2019) and benthic fish (Parry and Hirst 2016) productivity over the drought period. It is also possible that overgrazing by urchins on reefs and formation of barrens may have resulted from reduced nutrient inputs and associated plant productivity. Thus, setting a conservative limit on N input will reduce the risk of eutrophication in PPB. However, this needs to be done with the understanding that at very conservative levels there will be a consequence for the productivity of PPB, and also for the industries that depend on that productivity, such as fisheries and aquaculture.
The focus of this review has been on the bay-wide impacts of different nutrient levels on the ecology of PPB. Even when most of PPB is nutrient limited, there are likely to be localised eutrophic effects near the points of nutrient discharge. Thus, the question of scale is important in regard to management actions, which may differ depending on the scale considered. Furthermore, management actions considered appropriate for one scale may affect other scales differently. For example, management actions to reduce eutrophic conditions near nutrient sources through reduced N concentrations may also result in N limitation of productivity at a larger scale. Where there are recommendations relating to limiting nutrient concentrations at local scales to protect local values, managers need to be aware that these will have a broader impact as well, and this should be considered when setting local guidelines.
It may be tempting for managers to try to set a goal for nutrient inputs that is equal to the historical or ‘natural’ baseline. However, since European settlement the ecology of PPB has changed greatly, from a shellfish-dominated system to a phytoplankton-dominated system (Ford and Hamer 2016). Thus, although historical inputs of nutrients are likely to have been lower than today, it is also likely that biological productivity was high due to efficient recycling of nutrients by shellfish. The consequences of lower nutrient inputs will be different under today’s changed ecosystem conditions, potentially resulting in a greater loss of biological productivity compared with the historical baseline.
Conclusions
A major environmental study of PPB in the early- to mid-1990s focused on the risks of eutrophication and recommended a reduction in the N load from the WTP. Improved treatment efficiency at the WTP in the early 2000s as a result of the limit coincided with the decade-long Millennium Drought. The N load entering PPB was reduced by more than half compared with the wetter period in the 1990s. This provided a unique opportunity to study the ecological status of PPB under different nutrient regimes. Studies showed that PPB is an N limited system both in time and space. Biological productivity in PPB was markedly affected by reduced N loads during the drought with consequences for the important fisheries and aquaculture industries. Overall, research has shown that while setting conservative limits on N input will effectively negate the risk of widespread eutrophication, there will likely be a tradeoff in reduced bay productivity. Managers will need to consider both sides of this equation when managing the load of N entering PPB.
Data availability
Data sharing is not applicable as no new data were generated or analysed during this study.
Declaration of funding
We gratefully acknowledge the funding support from Melbourne Water, Australia.
Acknowledgements
We would like to thank Dr Catherine Rees and Dr Judy Blackbeard of Melbourne Water for supporting the development of this review.
References
Australian Bureau of Statistics (2023) Population Projections, Australia. Available at https://www.abs.gov.au/statistics/people/population/population-projections-australia/2022-base-2071
Australian Government (2023) Projections of population changes in our capital cities and rest-of-state areas. Available at https://population.gov.au/data-and-forecasts/dashboards/projections-population-changes-our-capital-cities-and-rest-state
Ayvazian SG, Ray NE, Gerber-Williams A, Grabbert S, Pimenta A, Hancock B, Cobb D, Strobel C, Fulweiler RW (2022) Evaluating connections between nitrogen cycling and the macrofauna in native oyster beds in a New England estuary. Estuaries and Coasts 45(1), 196-212.
| Crossref | Google Scholar | PubMed |
Ball D, Soto-Berelov M, Young P (2014) Historical seagrass mapping in Port Phillip Bay, Australia. Journal of Coastal Conservation 18(3), 257-272.
| Crossref | Google Scholar |
Barbier EB (2017) Marine ecosystem services. Current Biology 27(11), R507-R510.
| Crossref | Google Scholar | PubMed |
Berelson WM, Heggie D, Longmore A, Kilgore T, Nicholson G, Skyring G (1998) Benthic nutrient recycling in Port Phillip Bay, Australia. Estuarine, Coastal and Shelf Science 46, 917-934.
| Crossref | Google Scholar |
Berelson WM, Townsend T, Heggie DT, Ford P, Longmore AR, Skyring GW, Kilgore T, Nicholson GJ (1999) Modelling bio-irrigation rates in the sediments of Port Phillip Bay. Marine and Freshwater Research 50, 573-580.
| Crossref | Google Scholar |
Billen G, Garnier J (2007) River basin nutrient delivery to the coastal sea: Assessing its potential to sustain new production of non-siliceous algae. Marine Chemistry 106(1), 148-160.
| Crossref | Google Scholar |
Black KP, Hatton D, Rosenberg M (1993) Locally and externally-driven dynamics of a large semi-enclosed bay in southern Australia. Journal of Coastal Research 9, 509-538.
| Google Scholar |
Black KP, Longmore AR, Hamer PA, Lee R, Swearer SE, Jenkins GP (2016a) Linking nutrient inputs, phytoplankton composition, zooplankton dynamics and the recruitment of pink snapper, Chrysophrys auratus, in a temperate bay. Estuarine, Coastal and Shelf Science 183, 150-162.
| Crossref | Google Scholar |
Bourke MF, Kessler AJ, Cook PLM (2014) Influence of buried Ulva lactuca on denitrification in permeable sediments. Marine Ecology Progress Series 498, 85-94.
| Crossref | Google Scholar |
Broadley A, Stewart-Koster B, Kenyon RA, Burford MA, Brown CJ (2020) Impact of water development on river flows and the catch of a commercial marine fishery. Ecosphere 11(7), e03194.
| Crossref | Google Scholar |
Bulthuis DA, Axelrad DM, Mickelson MJ (1992) Growth of the seagrass Heterozostera tasmanica limited by nitrogen in Port Phillip Bay, Australia. Marine Ecology Progress Series 89, 269-277.
| Crossref | Google Scholar |
Cai W, Borlace S, Lengaigne M, van Rensch P, Collins M, Vecchi G, Timmermann A, Santoso A, McPhaden M, Wu L, England M, Wang G, Guilyardi E, Jin F-F (2014) Increasing frequency of extreme El Niño events due to greenhouse warming. Nature Climate Change 4(2), 111-116.
| Crossref | Google Scholar |
Cai W, Wang G, Santoso A, McPhaden MJ, Wu L, Jin F-F, Timmermann A, Collins M, Vecchi G, Lengaigne M, England MH, Dommenget D, Takahashi K, Guilyardi E (2015) Increased frequency of extreme La Niña events under greenhouse warming. Nature Climate Change 5(2), 132-137.
| Crossref | Google Scholar |
Carnell PE, Keough MJ (2014) Spatially variable synergistic effects of disturbance and additional nutrients on kelp recruitment and recovery. Oecologia 175(1), 409-416.
| Crossref | Google Scholar | PubMed |
Carnell PE, Keough MJ (2019) Reconstructing historical marine populations reveals major decline of a kelp forest ecosystem in Australia. Estuaries and Coasts 42(3), 765-778.
| Crossref | Google Scholar |
Chen JJ, Erler DV, Wells NS, Huang J, Welsh DT, Eyre BD (2021) Denitrification, anammox, and dissimilatory nitrate reduction to ammonium across a mosaic of estuarine benthic habitats. Limnology and Oceanography 66(4), 1281-1297.
| Crossref | Google Scholar |
Chiew FHS, Zheng H, Potter NJ, Ekstrom M, Grose MR, Kirono DGC, Zhang L, Vaze J (2017) Future runoff projections for Australia and science challenges in producing next generation projections. In ‘Proceedings - 22nd International Congress on Modelling and Simulation’, MODSIM 2017. pp. 1745–1751. (Modelling and Simulation Society of Australia and New Zealand) Available at https://mssanz.org.au/modsim2017/L16/chiew.pdf
Cloern JE, Abreu PC, Carstensen J, Chauvaud L, Elmgren R, Grall J, Greening H, Johansson JOR, Kahru M, Sherwood ET, Xu J, Yin K (2016) Human activities and climate variability drive fast-paced change across the world’s estuarine–coastal ecosystems. Global Change Biology 22(2), 513-529.
| Crossref | Google Scholar | PubMed |
Cook PLM, Evrard V, Woodland RJ (2015) Factors controlling nitrogen fixation in temperate seagrass beds. Marine Ecology Progress Series 525, 41-51.
| Crossref | Google Scholar |
Dalsgaard T (2003) Benthic primary production and nutrient cycling in sediments with benthic microalgae and transient accumulation of macroalgae. Limnology and Oceanography 48(6), 2138-2150.
| Crossref | Google Scholar |
Dayton PJ, Tegner MJ, Edwards PB, Riser KL (1999) Temporal and spatial scales of kelp demography: The role of oceanographic climate. Ecological Monographs 69(2), 219-250.
| Crossref | Google Scholar |
Dey R, Lewis SC, Arblaster JM, Abram NJ (2019) A review of past and projected changes in Australia’s rainfall. WIREs Climate Change 10(3), e577.
| Crossref | Google Scholar |
Drewry JJ, Newham LTH, Greene RSB, Jakeman AJ, Croke BFW (2006) A review of nitrogen and phosphorus export to waterways: context for catchment modelling. Marine and Freshwater Research 57(8), 757-774.
| Crossref | Google Scholar |
Eyre BD, Ferguson AJP (2009) Denitrification efficiency for defining critical loads of carbon in shallow coastal ecosystems. Hydrobiologia 629(1), 137-146.
| Crossref | Google Scholar |
Ford JR, Hamer P (2016) The forgotten shellfish reefs of coastal Victoria: documenting the loss of a marine ecosystem over 200 years since European settlement. Proceedings of the Royal Society of Victoria 128, 87-105.
| Crossref | Google Scholar |
García-Robledo E, Revsbech NP, Risgaard-Petersen N, Corzo A (2013) Changes in N cycling induced by Ulva detritus enrichment of sediments. Aquatic Microbial Ecology 69(2), 113-122.
| Crossref | Google Scholar |
Gaylard S, Waycott M, Lavery P (2020) Review of coast and marine ecosystems in temperate Australia demonstrates a wealth of ecosystem services. Frontiers in Marine Science 7, 453.
| Crossref | Google Scholar |
Glibert PM (2017) Eutrophication, harmful algae and biodiversity — Challenging paradigms in a world of complex nutrient changes. Marine Pollution Bulletin 124(2), 591-606.
| Crossref | Google Scholar | PubMed |
Hirst AJ, Jenkins GP (2017) Experimental test of N-limitation for Zostera nigricaulis seagrass at three sites reliant upon very different sources of N. Journal of Experimental Marine Biology and Ecology 486, 204-213.
| Crossref | Google Scholar |
Hirst AJ, Longmore AR, Ball D, Cook PLM, Jenkins GP (2016) Linking nitrogen sources utilised by seagrass in a temperate marine embayment to patterns of seagrass change during drought. Marine Ecology Progress Series 549, 79-88.
| Crossref | Google Scholar |
Hirst AJ, Giri K, Ball D, Lee RS (2017) Determination of the physical drivers of Zostera seagrass distribution using a spatial autoregressive lag model. Marine and Freshwater Research 68, 1752-1763.
| Crossref | Google Scholar |
Holdgate GR, Geurin B, Wallace MW, Gallagher SJ (2001) Marine geology of Port Phillip, Victoria. Australian Journal of Earth Sciences 48(3), 439-455.
| Crossref | Google Scholar |
Howarth RW (2008) Coastal nitrogen pollution: A review of sources and trends globally and regionally. Harmful Algae 8(1), 14-20.
| Crossref | Google Scholar |
Humborg C, Conley DJ, Rahm L, Wulff F, Adriana C, Ittekkot V (2000) Silicon Retention in River Basins: Far-Reaching Effects on Biogeochemistry and Aquatic Food Webs in Coastal Marine Environments. Ambio 29(1), 45-50.
| Crossref | Google Scholar |
Jenkins GP, Black KP (2019) Contrasting impact of diatoms on dominant calanoid and cyclopoid copepods in a temperate bay. Estuarine, Coastal and Shelf Science 217, 211-217.
| Crossref | Google Scholar |
Jenkins GP, Black KP (2021) Modelling the effects of changing loads of nitrogen discharged from a sewage treatment plant on plankton dynamics in a large, urbanised bay. Regional Studies in Marine Science 45, 101825.
| Crossref | Google Scholar |
Kriegisch N, Reeves S, Johnson CR, Ling SD (2016) Phase-shift dynamics of sea urchin overgrazing on nutrified reefs. PLoS One 11(12), e0168333.
| Crossref | Google Scholar | PubMed |
Kriegisch N, Reeves SE, Johnson CR, Ling SD (2019) Top-down sea urchin overgrazing overwhelms bottom-up stimulation of kelp beds despite sediment enhancement. Journal of Experimental Marine Biology and Ecology 514–515, 48-58.
| Crossref | Google Scholar |
Kriegisch N, Reeves SE, Johnson CR, Ling SD (2020) Sea urchin control of macroalgal communities across a productivity gradient. Journal of Experimental Marine Biology and Ecology 527, 151248.
| Crossref | Google Scholar |
Lee RS, Black KP, Bosserelle C, Greer D (2012) Present and future prolonged drought impacts on a temperate marine embayment. Ocean Dynamics 62, 907-922.
| Crossref | Google Scholar |
Lemley DA, Adams JB, Bornman TG, Campbell EE, Deyzel SHP (2019) Land-derived inorganic nutrient loading to coastal waters and potential implications for nearshore plankton dynamics. Continental Shelf Research 174, 1-11.
| Crossref | Google Scholar |
Maavara T, Akbarzadeh Z, Van Cappellen P (2020a) Global dam-driven changes to riverine N:P:Si ratios delivered to the coastal ocean. Geophysical Research Letters 47(15), e2020GL088288.
| Crossref | Google Scholar |
Maavara T, Chen Q, Van Meter K, Brown LE, Zhang J, Ni J, Zarfl C (2020b) River dam impacts on biogeochemical cycling. Nature Reviews Earth & Environment 1(2), 103-116.
| Crossref | Google Scholar |
Malone TC, Newton A (2020) The globalization of cultural eutrophication in the coastal ocean: causes and consequences. Frontiers in Marine Science 7, 670.
| Crossref | Google Scholar |
Marshall AJ, Longmore A, Phillips L, Tang C, Hayden HL, Heidelberg KB, Mele P (2021) Nitrogen cycling in coastal sediment microbial communities with seasonally variable benthic nutrient fluxes. Aquatic Microbial Ecology 86, 1-19.
| Crossref | Google Scholar |
Marshall AJ, Phillips L, Longmore A, Hayden HL, Heidelberg KB, Tang C, Mele P (2023a) Temporal profiling resolves the drivers of microbial nitrogen cycling variability in coastal sediments. Science of The Total Environment 856, 159057.
| Crossref | Google Scholar |
Marshall AJ, Phillips L, Longmore A, Hayden HL, Tang C, Heidelberg KB, Mele P (2023b) Using metatranscriptomics to better understand the role of microbial nitrogen cycling in coastal sediment benthic flux denitrification efficiency. Environmental Microbiology Reports 15(4), 308-323.
| Crossref | Google Scholar |
Murphy HM, Jenkins GP, Hamer PA, Swearer SE (2012) Interannual variation in larval survival of snapper (Chrysophrys auratus, Sparidae) is linked to diet breadth and prey availability. Canadian Journal of Fisheries and Aquatic Sciences 69, 1340-1351.
| Crossref | Google Scholar |
Murphy HM, Jenkins GP, Hamer PA, Swearer SE (2013) Interannual variation in larval abundance and growth in snapper Chrysophrys auratus (Sparidae) is related to prey availability and temperature. Marine Ecology Progress Series 487, 151-162.
| Crossref | Google Scholar |
Murray AG, Parslow JS (1999) Modelling of nutrient impacts in Port Phillip Bay — a semi-enclosed marine Australian ecosystem. Marine and Freshwater Research 50(6), 597-612.
| Crossref | Google Scholar |
Oczkowski AJ, Nixon SW, Granger SL, El-Sayed A-FM, McKinney RA (2009) Anthropogenic enhancement of Egypt’s Mediterranean fishery. Proceedings of the National Academy of Sciences 106(5), 1364-1367.
| Crossref | Google Scholar | PubMed |
Oviatt C, Smith L, Krumholz J, Coupland C, Stoffel H, Keller A, McManus MC, Reed L (2017) Managed nutrient reduction impacts on nutrient concentrations, water clarity, primary production, and hypoxia in a north temperate estuary. Estuarine, Coastal and Shelf Science 199, 25-34.
| Crossref | Google Scholar |
Parry GD, Black KP (2023) Sewage-derived nitrogen dispersal and N-fixation in Port Phillip Bay in south-eastern Australia. Marine and Freshwater Research 74(16), 1370-1387.
| Crossref | Google Scholar |
Parry GD, Hirst AD (2016) Decadal decline in demersal fish biomass coincident with a prolonged drought and the introduction of an exotic starfish. Marine Ecology Progress Series 544, 37-52.
| Crossref | Google Scholar |
Rauniyar SP, Power SB (2020) The Impact of anthropogenic forcing and natural processes on past, present, and future rainfall over Victoria, Australia. Journal of Climate 33(18), 8087-8106.
| Crossref | Google Scholar |
Rauniyar SP, Power SB (2022) Estimating future rainfall distributions in a changing climate for water resource planning: Victoria, Australia. Climate Dynamics 60, 527-547.
| Crossref | Google Scholar |
Reeves SE, Kriegisch N, Johnson CR, Ling SD (2018) Reduced resistance to sediment-trapping turfs with decline of native kelp and establishment of an exotic kelp. Oecologia 188(4), 1239-1251.
| Crossref | Google Scholar | PubMed |
Reeves SE, Kriegisch N, Johnson CR, Ling SD (2022) Kelp habitat fragmentation reduces resistance to overgrazing, invasion and collapse to turf dominance. Journal of Applied Ecology 59(6), 1619-1631.
| Crossref | Google Scholar |
Risgaard-Petersen N (2003) Coupled nitrification-denitrification in autotrophic and heterotrophic sediments: On the influence of benthic microalgae. Limnology and Oceanography 48, 93-105.
| Crossref | Google Scholar |
Risgaard-Petersen N, Nicolaisen MH, Revsbech NP, Lomstein BA (2004) Competition between ammonia-oxidizing bacteria and benthic microalgae. Applied and Environmental Microbiology 70(9), 5528-5537.
| Crossref | Google Scholar | PubMed |
Rozemeijer J, Noordhuis R, Ouwerkerk K, Dionisio Pires M, Blauw A, Hooijboer A, van Oldenborgh GJ (2021) Climate variability effects on eutrophication of groundwater, lakes, rivers, and coastal waters in the Netherlands. Science of The Total Environment 771, 145366.
| Crossref | Google Scholar | PubMed |
Seitzinger S, Harrison JA, Böhlke JK, Bouwman AF, Lowrance R, Peterson B, Tobias C, Van Drecht G (2006) Denitrification across landscapes and waterscapes: a synthesis. Ecological Applications 16(6), 2064-2090.
| Crossref | Google Scholar | PubMed |
Shim MJ, Yoon YY (2021) Long-term variation of nitrate in the East Sea, Korea. Environmental Monitoring and Assessment 193(11), 720.
| Crossref | Google Scholar | PubMed |
Trimmer M, Nedwell DB, Sivyer DB, Malcolm SJ (2000) Seasonal organic mineralisation and denitrification in intertidal sediments and their relationship to the abundance of Enteromorpha sp. and Ulva sp. Marine Ecology Progress Series 203, 67-80.
| Crossref | Google Scholar |
Ummenhofer CC, England MH, McIntosh PC, Meyers GA, Pook MJ, Risbey JS, Gupta AS, Taschetto AS (2009) What causes southeast Australia’s worst droughts? Geophysical Research Letters 36(4), L04706.
| Crossref | Google Scholar |
Valero-Rodríguez JM, Dempster T, Wong WW, Lewis JA, Cook PLM, Swearer SE (2024) Spatio-temporal patterns in the biomass, species composition and nitrogen content of drift macroalgae in an urbanised coastal embayment. Journal of Applied Phycology 36, 2369-2381.
| Crossref | Google Scholar |
Viaroli P, Bartoli M, Bondavalli C, Christian RR, Giordani G, Naldi M (1996) Macrophyte communities and their impact on benthic fluxes of oxygen, sulphide and nutrients in shallow eutrophic environments. Hydrobiologia 329(1–3), 105-119.
| Crossref | Google Scholar |
Whitall D, Bricker S, Ferreira J, Nobre AM, Simas T, Silva M (2007) Assessment of eutrophication in estuaries: pressure–state–response and nitrogen source apportionment. Environmental Management 40(4), 678-690.
| Crossref | Google Scholar | PubMed |
Wilson RS, Heislers S, Poore GCB (1999) Changes in benthic communities of Port Phillip Bay, Australia, between 1969 and 1995. Marine and Freshwater Research 49(8), 847-861.
| Crossref | Google Scholar |
Wong WW, Greening C, Shelley G, Lappan R, Leung PM, Kessler A, Winfrey B, Poh SC, Cook P (2021) Effects of drift algae accumulation and nitrate loading on nitrogen cycling in a eutrophic coastal sediment. Science of The Total Environment 790, 147749.
| Crossref | Google Scholar | PubMed |
Wong WW, Cartwright I, Poh SC, Cook P (2022) Sources and cycling of nitrogen revealed by stable isotopes in a highly populated large temperate coastal embayment. Science of The Total Environment 806, 150408.
| Crossref | Google Scholar | PubMed |
Zhao H, Yuan M, Strokal M, Wu HC, Liu X, Murk A, Kroeze C, Osinga R (2021) Impacts of nitrogen pollution on corals in the context of global climate change and potential strategies to conserve coral reefs. Science of The Total Environment 774, 145017.
| Crossref | Google Scholar |
Zheng H, Chiew FHS, Potter NJ, Kirono DGC (2019) Projections of water futures for Australia: An update. In ‘23rd International Congress on Modelling and Simulation - Supporting Evidence-Based Decision Making: The Role of Modelling and Simulation’, MODSIM 2019. pp. 1000–1006. (Modelling and Simulation Society of Australia and New Zealand) Available at https://mssanz.org.au/modsim2019/K7/zhengH.pdf