Soil carbon sequestration in rangelands: a critical review of the impacts of major management strategies
Beverley Henry A * , Diane Allen B , Warwick Badgery C , Steven Bray D , John Carter B , Ram C. Dalal E , Wayne Hall F , Matthew Tom Harrison G , Sarah E. McDonald H and Hayley McMillan DA
B
C
D
E
F
G
H
Abstract
The agronomic benefits of soil organic matter have been studied for centuries, but contemporary focus has expanded to ask how increasing long-term storage of soil organic carbon (SOC) can contribute to mitigation of climate change. Understanding the potential for SOC sequestration in the vast rangelands is crucial for climate change policy, agricultural land management and carbon market opportunities. In this review, we evaluate the evidence from published field trials and modelling studies for sequestration in Australian rangeland soils managed for livestock grazing. We found few long-term studies with high quality SOC stock change data linked to new management, and our analysis was constrained by data limitations, conflicting results between studies, and highly variable climate, soil and landscape conditions across production systems. Rainfall and soil properties are dominant determinants of variation in SOC stocks in rangelands, and it was difficult to detect management impacts in these environments. However, there was consistent evidence that: (1) Sowing more productive grasses or legumes in existing grass pastures generally increases SOC stocks; (2) Prolonged high stocking is associated with net SOC loss; (3) Destocking or exclusion of grazing results in small SOC increases, especially in degraded soils; (4) Conversion from cropping to permanent pasture results in sequestration, influenced by management history; (5) Rotational grazing strategies show negligible impact on SOC stocks relative to continuous grazing; and (6) Waterponding increased SOC stocks initially but persistence has not been demonstrated. We discuss possible opportunities for SOC sequestration in rangelands in the context of uncertainties and associated benefits and trade-offs for livestock production, and make recommendations to improve the evidence-base for major management strategies.
Keywords: Australia, carbon credits, climate change mitigation, grazing management, greenhouse gas emissions, pasture improvement, rainfall variability, sequestration, soil carbon.
Introduction
The potential for increasing storage of organic carbon (C) in forest and agricultural soils to help achieve climate change mitigation goals has received growing attention since the Paris Agreement at the Conference of Parties (COP) 21 of the United Nations Framework Convention on Climate Change in 2015. At COP 21, introduction of the ‘4 per 1000 Initiative’, with an aspiration to increase soil organic carbon (SOC) storage in agricultural and forestry lands annually by 0.4%, provided added focus to the role of soil organic carbon sequestration (SCS) (Soussana et al. 2019), and more recently the Intergovernmental Panel on Climate Change Sixth Assessment Report stated that natural climate solutions, notably permanent sequestration of C in trees and soils, are critical to restricting global warming to 1.5°C (IPCC 2023; Matthews et al. 2023; Zickfeld et al. 2023). Despite enhanced research investment over almost a decade since 2015, the magnitude and value of climate change mitigation possible through implementing practices that foster SOC storage is still debated. Estimates of the proportion of anthropogenic greenhouse gas (GHG) emissions that could be offset by SCS vary from over 20% (Minasny et al. 2017) to 4% or less (Schlesinger and Amundson 2019; Henderson et al. 2022; Janzen et al. 2022). Factors contributing to differences between estimates include: (i) Assumptions about the multiple biophysical, economic, and socio-cultural influences on net SOC storage in landscapes; (ii) evolving understanding of the complexities of SOC dynamics; and (iii) the technical challenges of reliably detecting and quantifying persistent change in SOC stocks (Lavallee et al. 2020; Begill et al. 2023; Cotrufo et al. 2023; Moinet et al. 2023). The extent of global rangelands raises expectations that they represent a large contribution to the global potential for SCS but, to date, agreement on achievable increases in SOC storage through management has proven elusive.
Published estimates of the proportion of global SCS expected in ‘rangelands’ are confounded by unclear definitions of land areas classified as rangelands as opposed to grasslands. Grasslands, usually defined as grass-dominated communities occurring both naturally and as cultured landscapes (Squires et al. 2018), have been attributed with around a quarter of the global SCS potential (Mahanta et al. 2020). In contrast, rangelands exclude highly modified pastures and grassy landscapes that encompass areas of relatively high productivity. Rangelands are commonly described as lands that are grazed, or have the potential to be grazed, by livestock and wildlife, with vegetation dominated by grasses, grass-like plants, forbs or shrubs, although they may also contain trees as in grazed woodlands and savannas (ILRI et al. 2021). This definition that covers approximately 50% of global terrestrial surface area, includes land estimated to hold as much as a third of the global stock of 1500 Gt SOC to a depth of 1 m (Schuman et al. 2002; ILRI et al. 2021). Even a small percentage change in this SOC stock would represent a large C sink but the realistic opportunity for achieving SCS through changes in management in livestock production systems is poorly understood (Khalil et al. 2019).
Many areas of rangeland have low or unreliable rainfall, infertile soils and low productivity, which limit organic material, primarily plant biomass, available to enter the soil organic matter (SOM) pool (Conant et al. 2001, 2017; Puche et al. 2019; Bartley et al. 2023; Dondini et al. 2023). Nevertheless, they have substantial ecological, social, economic and cultural value, and are the home and primary source of food for millions of people (Alexandratos and Bruinsma 2012; Ghosh and Mahanta 2014). Compared to more productive agricultural land types, there is limited understanding of how rangeland management practices affect the dynamics of soil C and nutrients and their relationship to ecological functioning, land condition and productivity (Sanderman et al. 2017; Khalil et al. 2019). Addressing these knowledge and data gaps requires improved capacity to monitor SOC stock changes over multi-decadal time periods to quantify SCS. Accurate and cost-effective measurement and modelling capacity is also crucial for reporting climate change mitigation against Paris Agreement targets, and for estimating C offsets with integrity across regions and properties that are often very large and diverse. However, standardised protocols have been developed relatively recently with promise for long-term monitoring to document permanent (century or longer timescales) CO2 removals. The most used method, based on repeat field sampling and laboratory analysis to estimate SOC stock changes makes quantifying SCS more costly than point-in-time measurements of C concentration in surface soil, which have traditionally informed agronomic decisions (Lal et al. 2018; Poulton et al. 2018; Henderson et al. 2022; Dondini et al. 2023; Moinet et al. 2023; Powlson and Galdos 2023). In the large areas of spatially diverse landscapes, heterogeneous soils and variable climates that characterise the world’s rangelands, the challenges of accurate and cost-effective measurement are exacerbated, further contributing to the difficulty in estimating the potential for SCS.
The objective of this review was to analyse SCS data from published studies in Australian rangelands that applied methods consistent with internationally accepted science and protocols for reporting GHG removals or SOC offsets (Paustian et al. 2019; Smith et al. 2020). The criteria applied to determine inclusion of data largely align with SOC offset accounting methods in the Australian Government voluntary soil C crediting scheme, known as the Australian Carbon Credit Unit (ACCU) Scheme and formerly as the Emissions Reduction Fund (Australian Government 2021). Management strategies considered were those compatible with productive rangeland grazing systems, and analysis of opportunities for SCS were restricted to management options that are economically feasible and practical in low input grazing systems. We examine the challenges of measurement of SOC stock changes and attribution to management rather than exogenous SOC drivers, and briefly discuss the influence of associated co-benefits, trade-offs and risks on adoption and maintenance of management changes in livestock production systems. Finally, we provide recommendations for investment in research and field trials to improve data and address knowledge gaps, with the aim of enabling more accurate assessment of the potential for SCS in rangelands using location-specific management strategies.
Methods
Scope of the review
We analysed data for Australian rangelands delineated according to the Australian Rangeland Society (ARS 2023) as areas used for grazing that have not been intensively developed for primary production, consistent with the global definition of ILRI et al. (2021). This definition allows that clearing of woody vegetation and naturalisation of exotic grasses and other forages has occurred in some regions. The scope of our review is broadly consistent with use in other Australian rangeland assessments (ACRIS 2008; Foran et al. 2019), and considers grazing management in savannas and grasslands but excludes highly modified pastures. Much of the area defined as rangeland (Fig. 1) is in semi-arid and arid zones, but it extends into eastern Australia to include regions with higher annual rainfall, such as tropical savannas, where other limitations restrict agricultural use to grazing in the natural landscape (ACRIS 2008; Bastin et al. 2009).
Land classified as rangeland spans over 75% of the total Australian land area (769 Mha), with areas subject to domesticated livestock grazing of native vegetation occupying 283 Mha. Data sources: ABARES (2021), ACRIS (2008).
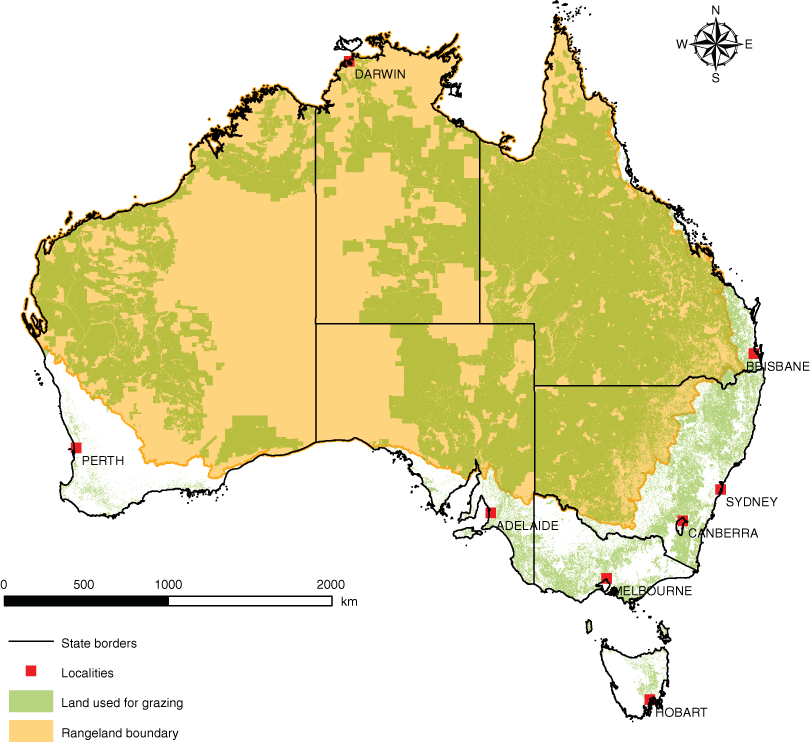
Conduct of the review
The review is based on a literature search to identify and assess SOC data relevant to analysis of the impact of management practices on SCS in Australian rangelands where extensive grazing of ruminant livestock (beef cattle, sheep, goats) is the primary land use (Fig. 1).
Both a traditional search of peer-reviewed articles and a systematic Web of Science search were used. The search terms used were TS = (rangeland* OR grassland* OR *arid) AND TS = (carbon) AND TS = (soil) AND TS = (Australia* OR Queensland OR “New South Wales” OR “Northern Territory” OR “Western Australia” OR Tasmania OR Victoria OR “South Australia” OR NSW OR QLD), which returned 626 articles. Published reports held by government and industry institutions were accessed through databases, online lists, or personal libraries of authors from these institutions. Australian results were placed in a global context using an overview of global measurement and novel quantification methods and conducting a review of relevant international publications on the SOC response to livestock and pasture management strategies in rangelands. Published reviews and original technical articles were used to provide an overview, but a comprehensive analysis of global research was out of scope for the present review.
Papers and reports were included in the review of Australian research if the study met data quality criteria (given below), and the reported results compared rangeland-relevant livestock production management strategies. Quantitative and qualitative data on soil C and its response to grazing and/or land management were extracted with accompanying information related to methodological requirements, including sampling depth, density and frequency, bulk density measurements, analysis method, period of monitoring, model calibration and verification, and any reported assumptions made in estimates of change in SOC stocks or SCS. Values of SCS were taken directly from publications, where reported, or calculated from published SOC stock data using either: (i) Initial or baseline measurement before implementation of a new management strategy; or (ii) Control and treatment measurements in paired-site or fenceline comparison studies. The latter assumes equal SOC in control and treatment sites prior to implementation, which inevitably introduces uncertainty. SCS (t C ha−1 year−1) attributable to the new management regime was estimated by dividing the difference in SOC stocks (t C ha−1) by years since implementation, recognising the constraints on these estimates due to the nonlinear pattern of change in stocks in response to management. Metadata, including study location, treatments, data collection methods and details such as duration of the study, protocols for soil carbon sampling, measurement, or modelling, were recorded.
Studies were grouped according to management strategy, observation period, data ‘quality’ (using criteria such as sampling density and depth) to determine whether SOC measurements allowed estimates of sequestration. Three fundamental requirements were used as the primary determinants for inclusion of data from field studies in the SCS analysis: (i) credible measurement or verified data enabling SCS to be quantified; (ii) adequate description of a rangeland-relevant management change; and (iii) monitoring over a sufficient period to indicate a persistent change in SOC stocks, with ≥10 years preferred although a limited amount of flexibility was accepted. Management strategies were analysed under three categories that are accepted as having a mechanistic link to SOC stock change: grazing management; pasture improvement; and land conversion (Fig. 2, CER 2021). However, causality between a specific management intervention and SOC stock change is difficult to establish in field studies due to the number of interacting factors that potentially influence SOM inputs and loss (Pringle et al. 2016).
Management and nonmanagement factors potentially affecting soil organic carbon (SOC) inputs to, and loss from, grazed rangelands. Underlined management strategies are those for which published studies provided data on the potential for SOC sequestration in Australian grazing systems analysed in this review. GPP, gross primary productivity; OM, organic matter.
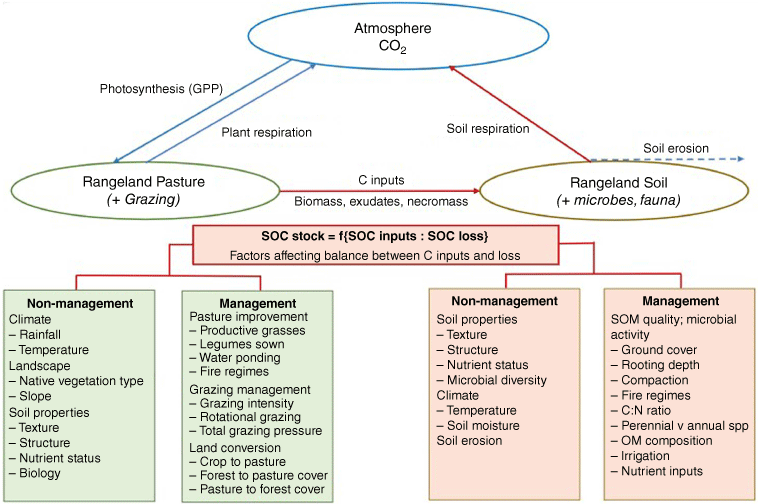
Preliminary examination of the initial dataset revealed that information provided in papers and reports was often insufficient to meet accepted minimum standards to quantify SCS for climate change mitigation accounting. This sometimes reflected the objectives of older trials or arose because data acquisition was realistically limited by scope and/or research budgets. Working within these constraints, the following criteria were used to determine data that could be included in the analysis of long-term SCS: (1) Soil sampling to a depth of ≥30 cm with unbiased spatial sampling strategy; (2) Analysis of SOC concentration using either dry combustion, other accredited laboratory analysis method, or in situ, proximal or remote sensing methods, such as VIS-NIR-MIR spectrometry, accompanied by adequate description to show proper calibration and validation; (3) Calculation of SOC stocks for measurement or calibration sites using measured soil bulk density to convert concentration to stocks (preferably on an equivalent soil mass basis, ESM); (4) SOC stock change using either at least two measurement rounds – baseline and remeasurement over a period sufficient to indicate persistence (at least a decade but noting longer is needed to demonstrate permanence), or space-for-time measurements of appropriately selected ‘control’ and ‘management change’ sites such as paired-site or fenceline comparisons; (5) A modelling approach with justification of process model functionality and capacity to simulate the management under analysis combined with demonstration of adequate calibration and validation data for the site and conditions; and (6) Description and implementation date for change in a management strategy relevant to rangeland livestock production.
Lack of clarity and consistency in the use of terms to describe grazing management strategies, particularly with reference to strategies based on the manipulation of the timing or duration of livestock access to pasture and periods of pasture rest in grazing rotations confounded analysis of the data. Due to the small number of studies and lack of clear delineation between more and less intensive stock densities and movements, we grouped all grazing rotation studies under the general term, ‘rotational grazing’, whether originally described here as rotational, cell or time-controlled. Hence, in our analysis, strategies were aligned according to the grazing practice descriptions given by Allen et al. (2013) but with (b) and (c) combined:
Continuous stocking: Pastures are never or rarely spelled; with grazing over most of the year.
Rotational grazing: Stock are moved between paddocks so that a period of grazing is followed by a period of resting (‘spelling’) a paddock. The spelling period usually depends on the condition and growth of pasture and predicted growing season rainfall.
Cell or time-controlled grazing: These are forms of intensive rotational grazing that use many small paddocks that are heavily stocked for a short time, followed by a long spelling period.
Destocking or Exclosures: These terms refer to, respectively, removal of livestock grazing (with grazing by native or feral animals still possible) or exclusion of livestock and other herbivores such as kangaroos. In some publications reviewed it was not clear whether non-domestic animals were contributing to the total grazing pressure.
Results and discussion
After screening for relevance and data quality, the results from 23 publications were assessed as providing reliable data on the impacts of management strategies on SCS in Australian rangelands (Table 1). Because the dataset was small, a further 15 publications were examined to expand and test information on the trend in SOC stocks for the same management strategies, despite not having data fully compliant with our listed criteria for quantification of SCS (Appendix Table A1).
Study reference | Study region | Av. Ann. rainfall (mm) | Management change | Monitoring period (years) | Sampling depth (cm) | Initial (I) or Control (C) SOC (t C ha−1) | SCS (t C ha−1 year−1) | |
---|---|---|---|---|---|---|---|---|
Grazing Management strategies | ||||||||
Grazing intensity | ||||||||
Bray et al. (2014)A | Charters Towers region, QLD | 640 | Moderate vs high | 16 | 0–30 | 19.2 (C) | 0.087 | |
Pringle et al. (2014)A | Julia Creek region, QLD | 429 | Low vs mod/high | 26 | 0–30 | 13.32 (C) | 0.004–0.035 (n.s.) | |
Rotational vs continuous grazing | ||||||||
Allen et al. (2013)A | QLD rangelands | 256–1138 | Rotation/cell vs continuous | ~10D | 0–30 | 4.79–75.49 (C) | 0 (n.s.)C | |
Schatz et al. (2020)A | Northern NT | 1209 | Intensive rotation vs continuous | 5 | 0–30 | 14.08–19.69 (I) | −0.03 (n.s.) | |
Sanderman et al. (2015)A | Upper, mid-north SA | 310–570 | Rotation vs continuous | 7+D | 0–30 | 20–80 (C) | −0.07 | |
Orgill et al. (2017)A | Brewarrina, NSW | 292 | Rotation vs continuous | 8+D | 0–30 | 13.54–13.97 (C) | −0.11 to 0.01 (n.s.) | |
Destocking or excluding grazing | ||||||||
Allen et al. (2013)A | QLD rangelands | 256–1138 | Exclosure vs grazed | ~10D | 0–30 | 4.79–75.49 (C) | 1.68 | |
Carter et al. (2006) | Charleville region, QLD | 483 | Exclosure vs grazed | 24 | 0–30 | 51.6 (C) | 0.28B | |
Daryanto et al. (2013)A | Enngonia region, NSW | 312 | Exclosure vs grazed | 20 | 0–30 | 30.06 (C) | 0.27–0.38B | |
Hunt (2014)F | Kidman Springs, NT | 667 | Destocked vs grazed | 58 | 0–30 | 32.51 (C) | 0.05 | |
Pringle et al. (2014)A | Julia Creek region, QLD | 429 | Destocked vs grazed | 26 | 0–30 | 13.48 (C) | 0.006–0.041 (n.s.) | |
Sanderman et al. (2015)A | Upper, mid-north SA | 310–570 | No stock vs grazed | 7+D | 0–30 | 20–80 (C) | 0.17–0.24 (n.s.) | |
Witt et al (2011)A | South-west QLD | 150–500 | Exclosure vs grazed | 13–43 | 0–30 | 22.0 (C) | ≤0.05–0.13B | |
Pasture management strategies | ||||||||
Sowing more productive grasses into grass pastures | ||||||||
Chan et al. (2010)E | Central-southern NSW | 600–800 | Introduced vs native pastures | ≥10 | 0–30 | 42.8 (C) | 0.02 (n.s.) | |
Clewett (2015)F | Condamine, QLD | 672 | Sown vs native pastures | 50 | 0–30 | 31–52 (C) | 0.11 | |
Sowing legumes into grass pastures | ||||||||
Conrad et al. (2017) | Gayndah region, QLD | 691 | Leucaena-grass vs native pastures | 40 | 0–30 | 43.7–51.7 (C) | 0.28 | |
Wochesländer et al. (2016) | South-west WA | 498 | Tagasaste vs native grass | 22 | 0–30 | 18.6 ± 0.6 (C) | 0.72 | |
Clewett (2015)F | Condamine, QLD | 672 | Sown grass-legume vs native pastures | 50 | 0–30 | 31–52 (C) | 0.38 | |
Waterponding in scald areas | ||||||||
Read et al. (2012) | Central-west catchment, NSW | 400 | Waterponding vs scalded | 20–25 | 0–30 | 18.7 (I) | 0.28 | |
Managing fire regimes in grazed savannas | ||||||||
Hunt (2014)F | Kidman Springs, NT | 667 | Early season burn (2,4 yearly) vs unmanaged fire regime | 58 | 0–30 | 32.51 (C) | −0.03 | |
Hunt (2014)F | Kidman Springs, NT | 667 | Late season burn (4,6 yearly) vs unmanaged fire regime | 58 | 0–30 | 32.51 (C) | −0.04 | |
Land conversion strategies | ||||||||
Conversion from cropland to permanent pastures | ||||||||
Badgery et al. (2020) | Condobolin, NSW | 424 | Perennial pasture vs cropping | 15 | 0–30 | 23.2 (C) | 0.48 | |
Jones et al. (2016) | South-west QLD | 583 | Cropping to grass pasture | 20 | 0–30 | 27.9 (I) | 0.18 | |
Wilson et al. (2011) | North-west NSW | 690–880 | Cultivation to pasture | 15–20 | 0–30 | 46–121 (C) | 0.06–0.15 | |
Conversion from forest cover to grassland | ||||||||
Dalal et al. (2005) | Mulga View, SW QLD | 516 | Mulga woodland to sown pasture | 20 | 0–30 | 27 (C) | 0.12 | |
Dalal et al. (2011) | Brigalow Catchment Study, QLD | 720 | Brigalow forest to sown pasture | 23 | 0–40 | 84 (C) | 0 (n.s.) | |
Dalal et al. (2021) | Brigalow Catchment Study, QLD | 720 | Brigalow forest to sown pasture | 33 | 0–30 | 54.8 (C) | −0.05 (n.s.) | |
Harms et al. (2005) | Central-Southern QLD rangelands | 600–800 | Forest/woodland to buffel grass/native pasture | <11–31 | 0–30 | 19.89–36.09 (C) | −0.22 (n.s.- Eucalypt, Brigalow;B Mulga) | |
Wilson et al. (2011) | North-west NSW | 690–880 | Woodland to native pasture | 20–50 | 0–30 | 46–121 (C) | −1.76 | |
Wilson et al. (2011) | North-west NSW | 690–880 | Native woodland to sown pasture | 20–30 | 0–30 | 46–121 (C) | −2.07 | |
Allen et al. (2016) | Brigalow Belt, QLD (45 sites) | NR | Brigalow forest to pasture | 15–73 | 0–30 | 27–116 (C) | 0.72 | |
Conversion from grassland to forest cover | ||||||||
Allen et al. (2016) | Brigalow Belt, QLD | NR | Pasture to brigalow regrowth | 16–76 | 0–30 | 16–76 (C) | 0 (n.s.) |
The studies spanned each of the mainland states and territories with rangeland used for grazing livestock: New South Wales (NSW), Northern Territory (NT), Queensland (QLD), South Australia (SA), Western Australia (WA). Studies were included in the analysis if they reported estimates of SCS or data on SOC stocks and period of monitoring that enabled calculation of SCS consistent with international GHG accounting protocols and method requirements in carbon crediting schemes.
Av. Ann. Rainfall, average annual rainfall; NR, not reported.
The review revealed marked differences between studies in the quality of data and information provided on the specific management intervention, which hampered comparisons and efforts to link management strategies to SCS. In summary, analysis results have high uncertainty due to the small number of long-term studies, non-standard methods for quantifying SCS, and inconsistencies in the implementation of grazing management strategies. We discuss the published results for each management intervention and possible reasons for conflicting outcomes to explain the value and the limitations of the analysis. The identified gaps in data and knowledge informed guidance for improved soil sampling and measurement and recommendations for priority research to support evidence-based policy and land management.
Analysis of management impacts on SCS
Grazing affects SOC in a range of ways that include: (1) herbivory removes plant biomass that could otherwise be incorporated in SOM; (2) altering the allocation to below ground (root) biomass; (3) changing plant growth rates and species composition; (4) trampling affects on soil compaction and the rate of litter breakdown; (5) modifying C and nutrient cycling, including through adding dung and urine; and (6) exposing soil to accelerated respiratory loss and increased risk of erosion (particularly at prolonged high stocking rates). The impacts on net SOC storage and stability, mediated primarily by microbial activity, are altered by livestock management decisions on stocking rates and the timing of grazing and rest periods. However, in Australian rangelands more than half the total grazing pressure may be largely unmanaged, depending on populations of feral animals, including goats, and native herbivores, such as kangaroos, although livestock management may indirectly alter feral and native herbivore numbers through fencing and access to watering points. In combination with drought and other climate factors the presence of non-domestic grazing can constrain the capacity of producers to predictably foster SCS through livestock management strategies (Waters et al. 2019).
Of the 32 publications identified that reported impacts of grazing management on SOC in Australian rangelands, 10 had data to ≥30 cm and measured the effects of management over a sufficient time period to indicate persistence, mostly for a decade or longer (Table 1). An additional seven publications were considered as having data indicative of extensive low-input grazing management impacts on SOC storage (0–30 cm) (Table A1) but were either outside the boundary adopted for rangelands (Fig. 1) or relied on unvalidated modelling (Clewett 2015). The variation in SOC stock change estimated for studies in this expanded dataset was higher than in the more reliably quantified SCS (Table 1) but broadly confirmed both the direction and uncertainty in the response for each management strategy analysed (last column, Table A1). For the 10 studies in Table 1, the results for SOC stock change, estimated from either an initial/baseline or a ‘control’ measurement, provide the following indicative SCS response to management.
Grazing intensity is commonly described as ‘light’, ‘moderate’ or ‘heavy’, terms sometimes translated to light, moderate or high stocking rate relative to a regional recommended stocking. The impact on SOC stocks of low to moderate grazing intensity was reported as non-significant or small (<0.1 t C ha−1 year−1) (Table 1; Bray et al. 2014; Pringle et al. 2014). This is supported by indicative trends in the expanded dataset (Table A1), and is consistent with results of a meta-analysis of stocking rate trials in Australia (McDonald et al. 2023) and research in other countries (e.g. Chen et al. 2015). In contrast with conservative stocking, high grazing pressure in rangelands has been associated with a decline in SOC reflecting net SOM loss resulting from lower inputs as a result of herbivory and higher rates of mineralisation and/or erosion due to higher disturbance and exposed soil surface (Fig. 2).
Specific stocking rate management practices, landscape characteristics and climate have been shown to affect the balance between SOM inputs and loss differently, and to lead to conflicting results (Waters et al. 2017). Temperature and vapour pressure deficit can account for >80% of differences in SOC stocks (Allen et al. 2013; Rabbi et al. 2015), and Allen et al. (2013) concluded that only after detrending of climate effects could the influence of stocking rate on SOC stocks be detected as a weak negative association in measured data from a north-eastern Australian survey. Baseline soil condition and SOC stocks, together with legacy management, may also contribute to variations (Muleke et al. 2023), and together these interacting influences confound the impact of stocking rate. Overall, the preliminary evidence indicates that high grazing intensity is likely to result in a small, negative SCS effect but low to moderate stocking rates appear to have no or negligible impact. Targeted studies are needed to improve attribution of changes in SCS under different grazing intensities to management versus climate and legacy effects.
Of the four studies assessing the response of SCS to implementation of grazing rotation strategies, none showed a significant impact of managing the timing of grazing and rest periods on SOC stocks (Table 1; Allen et al. 2013; Sanderman et al. 2015; Orgill et al. 2017 Schatz et al. 2020). A survey of 98 sites across a rainfall and temperature gradient in tropical and subtropical Queensland (28 continuously grazed; 60 rotationally grazed; 10 exclosures) by Allen et al. (2013) found that, after detrending of climate effects, the cell grazing form of grazing rotations was associated with a non-significant decline in SOC stocks compared to continuous grazing. Similarly, a field trial in tropical savannas (Northern Territory, Australia) that compared high-intensity rotational grazing with continuous grazing at either set or variable stocking rate, found no significant difference in SCS between any of the grazing systems (Schatz et al. 2020). However, the monitoring period was less than 6 years and there is uncertainty regarding longer-term changes. In all but one of the five additional studies with indicative data (Table A1), there was similarly no significant difference in continuous vs rotational grazing. This is consistent with findings of review publications for Australian and international rangelands (Hawkins et al. 2022; Henry 2023; McDonald et al. 2023).
Studies comparing SOC stocks in areas that were grazed or ungrazed by domestic livestock (Table 1) presented inconsistent results, but an overall trend for a small, positive rate of SCS for destocked and/or exclosure sites relative to areas grazed by livestock. Results ranged from −0.05 to 0.1 t C ha−1 year−1 (Carter et al. 2006; Witt et al. 2011; Daryanto et al. 2013; Hunt 2014; Pringle et al. 2014). Excluding livestock and reducing grazing pressure may provide an option for SCS while simultaneously improving land condition in non-productive or degraded parts of a grazing property, but is unlikely to be economically attractive in land managed for profitable production. Additionally, to manage the negative consequences of C farming displacing food production on agricultural land initially managed for livestock grazing, destocking is not eligible to earn C credits in schemes such as the Australian government scheme (Australian Government 2021, refer to Clauses 10(3) and 11(2)).
No studies were found that specifically examined the impact of the type of domestic ruminant animal on SOC stocks in rangelands, but it has been suggested that over long timeframes different species (cattle, sheep or goat) may result in plant diversity changes (e.g. Olff and Ritchie 1998; Tóth et al. 2018) relative to sites grazed by native herbivores (macropods) alone (Eldridge et al. 2017). Additionally, grazing patterns are known to differ between species, e.g. sheep, cattle, and kangaroos are able to travel 3, 6 and 8 km, respectively, from water (Fensham and Fairfax 2008; Pahl 2019). However, although it is conceivable that there could be an impact on SOC stocks over the long term, via either the quality of SOM and microbial diversity or patterns of grazing pressure, more research is needed to evaluate a possible species-linked management impact on SCS.
This review of published studies with reliable data for Australian rangelands indicates limited potential for SCS (<0.1 t C ha−1 year−1) for grazing exclusion or change from high to conservative grazing intensity. Uncertainty is high because no studies had dynamic baseline monitoring across decadal time periods, and almost all studies reviewed lacked an initial SOC stock measurement before implementation of the new management practice. The reliance on space-for-time measurement data to estimate SOC stock change and the dearth of information on the legacy of historic management also constrain confidence in projections of the timeframe and magnitude of SCS response to new management. Additionally, the location- and condition-specific potential for SCS cannot be determined due to insufficient data for different management systems across the biogeographical coverage of rangelands.
Climate, soil, landscape and vegetation characteristics are the predominant determinants of Net Primary Production and, hence, SOM inputs from above- and below-ground plant biomass. Management of pastures interacts with these natural factors to affect SOC stocks and the proportion of SOC that is stabilised (Sanderman et al. 2010; Janzen et al. 2022). In extensive grazing enterprises high costs (financial, time and resources) for implementation of practices such as fertilisation or irrigation act as a barrier to adoption although they may be feasible on more intensive properties. In the rangelands, there is a limited suite of practical and economic management options. A decision to implement a management change for voluntary participation in C farming must also consider the cost of SOC measurement in large and diverse landscapes, and the greater risks to permanency of SCS in the variable and extreme climates that are common in rangelands (White 2022). As a result, field studies evaluating pasture management strategies have traditionally focused on the production and land condition benefits, with limited collection of long-term SOC stock change data. The available data were analysed for four pasture management strategies (described below and in Fig. 2): (1) sowing more productive grasses; (2) sowing legumes into grass pastures; (3) use of waterponding to rehabilitate scalds; and (4) changing fire regimes to improve forage quality (an option of most relevance in tropical savannas). Six publications were found with credible SCS data (Table 1).
The practice of improving the productivity of livestock grazing by sowing more productive grasses into existing grass pastures is more relevant to temperate southern areas of Australia and only two publications, one measurement and one modelling, with SCS data relevant to rangelands were found. In the northern rangelands, investment in sowing is more likely to occur following clearing of woodlands and savannas (Dalal et al. 2005; Livesley et al. 2021) and these studies are reviewed with land conversion strategies.
Higher grass biomass is expected to increase SOM inputs and SCS, but low and variable soil moisture and poor nutrient levels make achieving persistent SOC gains in rangelands challenging. If sowing were accompanied by initial application of nutrients to promote growth, higher SOC accumulation may occur in the initial years, but longer-term results are more uncertain, even in no-rangeland pastures (Badgery et al. 2020). Measurements in central-west New South Wales showed that gains in SOC stocks after sowing improved grass species did not persist, and after a decade, SOC stocks (0–30 cm) were not significantly higher under introduced compared to native grasses (Chan et al. 2010). This study site was just outside the boundary but climatically comparable to rangeland regions where sowing grasses is a viable option. Longer-term monitoring is needed to confirm this impact for different rangeland conditions. A decline in SCS over time after sowing was also found in a modelling study for the Condamine region of southern Queensland. Confidence in these simulations is low because they were not verified by in-field measurements, but the study found SOC stocks under sown grass increased at 0.5 t C ha−1 year−1 compared to native pastures over the first 10 years but over 50 years averaged only 0.11 t C ha−1 year−1 (Clewett 2015). For this strategy, no field data for SCS monitored over a decade or longer were found for Australia’s northern or arid rangelands.
In periods where growth is not constrained by soil moisture, low soil nitrogen (N) limits productivity of grass pastures in large parts of the Australian rangelands. Legume–grass pastures are attractive to livestock producers because they can extend forage availability in dry periods and provide higher feed quality through N fixation, thus reducing the risk of land degradation while also improving animal growth rates (Radrizzani et al. 2011; Wochesländer et al. 2016). Higher input and quality of SOM from N-fixing legumes is also beneficial for soil microbial activity and the production of microbial necromass C, which comprises 2–5% of total soil C, can enhance SCS in rangelands (Kumar et al. 2018; Kästner et al. 2021).
Measurements over 40 years following planting of the leguminous shrub Leucaena leucocephala (Lam.) de Wit ssp. glabrata (Rose Zarate) into grasses in southern Queensland showed a consistent increase in SOC stocks (0–30 cm) equivalent to a SCS rate of 0.28 t C ha−1 year−1 (P < 0.05) (Conrad et al. 2017). In other studies where SOC was measured or modelled with various forage legumes and time periods, there was a consistent trend for increase in stocks, with estimated SCS rates of 0.14–0.72 t C ha−1 year−1 (0–30 cm) (Clewett 2015; Harrison et al. 2015; Wochesländer et al. 2016). Other publications with SOC stock measurements, although not consistent with sequestration requirements (Table A1), provide additional confidence, with SOC stock increases of 0.08–0.78 t C ha−1 year−1 to a depth of 15 cm measured in leucaena–grass pastures relative to adjacent grass-only pastures (Radrizzani et al. 2011). These authors reported a correlation between the rate of increase in SOC and the degree of soil N limitation prior to sowing, but more detailed studies are needed to understand the dependence on initial soil N status and the dynamics and stabilisation of C in rangeland soils. Other legumes planted in rangeland grass pastures for their productivity and environmental benefits include Stylosanthes spp. and Desmanthus spp. (Gardiner et al. 2013; Kumar et al. 2018). There has been less research on their impacts on SOC stocks (Clewett 2015) but, in regions where plantings are already extensive, such as for Stylosanthes spp. in parts of Queensland, ‘additionality’ may be difficult to establish for C credit eligibility (CER 2021).
In the semiarid rangelands of Australia, waterponding has been used to rehabilitate claypan or scalded areas that are eroded and sealed (Read et al. 2016). Because scalded land is characteristically degraded and depleted of SOC stocks, e.g. <20 t C ha−1 in the top 30 cm (Read et al. 2012), the possibility exists for waterponding to have an associated SCS benefit. In a study in the Central-West Catchment of New South Wales where there is extensive scalding, rates of SCS were found to be as high as 1.5 t C ha−1 year−1 over the first 5 years before stabilising (Read et al. 2012). Research is needed on the long-term SOC dynamics following waterponding and on the level of any GHG emissions (N2O, CH4) associated with the strategy and subsequent land management to understand the net climate change mitigation potential.
Although several publications have examined the impacts of fire as a management strategy for rejuvenating pastures in tropical and subtropical rangelands of Australia, very few studies have monitored SOC stocks and associated GHG emissions to enable quantification of SCS. The intensity and frequency of wildfire in tropical savannas are naturally highly variable, reflecting fluctuations with climate and ignition events over interannual climate patterns such as the El Nino Southern Oscillation, and longer decadal monsoonal cycles (Hunt 2014). Analysing the potential for SCS due to management actions to reduce the intensity or frequency of burning is complex and attribution of changes in SOC stocks is difficult when multiple practices are implemented. Whereas changes in fire regimes have long-term impacts on SOM inputs from above- and below-ground biomass, there are also more immediate effects of fire on plant litter and plant growth. Additionally, a decision to manage fire may be accompanied by activities to improve pasture production by sowing of favoured grass or legume species and possibly irrigation and fertilisation (Livesley et al. 2021). The inherent variability together with the absence of initial SOC stock value or a dynamic baseline, likely contributed to the absence of a detectable management impact on SCS in measurement studies in the long-term Kidman Springs fire experiment in the Northern Territory, Australia (16°05′S, 130°57′E) (Allen et al. 2021), despite modelling showing a modest amount of additional storage in soil (Hunt 2014). Overall, the reviewed publications indicated the potential for a small increase in SOC stocks with reduction in the frequency and intensity of savanna burning. The increase was typically lower than the additional sequestration of C in woody biomass that also followed the change in burning regime. The additional tree biomass can result in a productivity trade-off as pasture growth suffers from increased competition from tree cover, and this acts as a barrier to adoption of fire management for SCS goals.
There is evidence that certain pasture improvement strategies, notably sowing more productive grasses or legumes in grass pastures, have SCS potential. However, the number of studies with reliable data was small and coverage across the extent of rangelands was restricted to less arid regions. More research is needed to quantify the impact and its persistence in different locations and soil conditions. There is also a need to better understand the economic, social and environmental impacts of each prospective practice, including costs of implementation and any trade-offs, such as drought tolerance and the risk of weediness of introduced pasture species. The literature search did not provide sufficient publications to adequately assess the SCS potential for strategies such as changing fire regime and implementing waterponding, but there is some indication of value in more research on these options for applicable rangeland regions. For all strategies reviewed, significant data and knowledge gaps exist, notably in relation to time course and upper limits on accumulation of stable soil carbon in different soil types, permanency of SOC stock changes, and magnitude of any GHG emissions associated with implementation of pasture improvement activities (Tomkins et al. 2019; CER 2021).
We reviewed published studies with SOC stock data for three categories of land conversion that may occur in areas of rangeland livestock production: (1) Conversion from cropping to perennial pastures; (2) Conversion from forest or woodland cover to grassland; and (3) Conversion from grassland to forest cover. Historically, most land conversion activity in rangelands was due to livestock producers’ strategic management of the tree–grass balance, often involving removing woody vegetation to optimise forage production and grazing sustainability (Burrows et al. 2002; Hall et al. 2020). Land-use change studies, particularly those conducted more than three decades ago, rarely took baseline soil samples for C analysis, and most estimates of SOC stock change are based on space-for-time analyses (Guo and Gifford 2002; Dalal et al. 2005; Harms et al. 2005; Laganiere et al. 2010; Don et al. 2011; Bray et al. 2016). In a specific rangeland property or region, the potential for SCS will depend not only on biophysical factors but on a range of socio-economic aspects that affect the rate of adoption of prospective management strategies. Specifically, land conversion may be restricted by legislation governing vegetation management, permitted land use categories or access to water. Therefore, in addition to accurate biophysical data, understanding is needed of any policies affecting conversion, and constraints, including implementation barriers and opportunity costs, for a realistic assessment of the potential for SCS (Thamo and Pannell 2016; White 2022).
Three rangeland studies with credible SCS data were found for this category. They showed that, after periods of 15–20 years, perennial pasture soils stored more SOC than cropping soils, with an inferred rate of SCS (0–30 cm) attributed to conversion ranging from 0.06 to 0.48 t C ha−1 year−1 (Table 1, Wilson et al. 2011; Jones et al. 2016; Badgery et al. 2020). The range is comparable to other estimates for Australian rangelands (Table A1, Sanderman et al. 2010), but towards the lower bound of published results for grasslands globally (Poeplau et al. 2011; Conant et al. 2017; Sanderman et al. 2017). This is not unexpected since the global analyses include studies in higher rainfall, more productive conditions than those that characterise Australian rangelands (Liu et al. 2017).
A study in the semi-arid, sub-tropical rangelands of south-west Queensland that measured an increase in SOC stocks after conversion of land from cropping to perennial pasture of 0.09 t C ha−1 year−1 (0–30 cm) over 20 years (Jones et al. 2016) provides insights into constraints on SCS and recovery of SOC and soil function in these environments. After 20 years of cropping following clearing of virgin forest, SOC stocks in the top 30 cm had declined by 9.97 t C ha−1 (P < 0.01) from an initial level averaging 33.7 t C ha−1. After conversion to pasture, recovery of SOC stocks varied, with greater residual loss after 20 years in sites that had more years of cropping. Measurements also showed that the decline in soil N that occurred under cropping was only partially restored after 20 years of perennial pasture and the authors linked lower soil fertility as indicated by aggregation and mineralisable N to more years under cropping. The study demonstrates that long-term cropping limits the system’s resilience, capacity to recover soil fertility, and to sequester SOC. This legacy effect suggests that conversion to perennial pasture after long-term cropping may not be sufficient to restore soil health and productivity. SCS cannot be assumed to occur at a rate necessary for recovery of the amount of SOC lost under previous degrading management practices, at least over time periods of a couple of decades without addressing problems such as the fertility deficit, represented by N.
Six published studies with SOC data following conversion from native forest or woodlands to native pasture or sown grasses provided conflicting results for SCS, ranging from −2.42 to 0.12 t C ha−1 year−1, with most changes being not significant (Table 1, Table A1). Global publications for this land conversion category have also been inconsistent, reporting an increase or decrease or no change in SOC stocks (Post and Kwon 2000; Guo and Gifford 2002). The studies for Australian rangelands cover a range of climate, soil properties, land types and monitoring periods, and significant differences in management following removal of trees, all of which can contribute to conflicting results. The largest measured losses of SOC were for sites across soil type and land use gradients in the variable rainfall rangelands of north-west NSW (Table 1, Wilson et al. 2011). The extended set of indicative rangeland data (Table A1) confirmed the lack of consistency and confounding effects of postclearing management. For example, Livesley et al. (2021) reported SCS (0–30 cm) over a 28-year period of 0.34 t C ha−1 year−1 in the Northern Territory, reflecting fertilisation and planting of improved pastures followed clearing of woody vegetation. The range in outcomes demonstrate the need for additional information and the risks of extrapolation of results across sites or regions. Reliable predictions of SCS need regional vegetation, soil and climate data, including rainfall patterns as well as averages, and details of post-conversion management.
Management to establish trees on previously cleared land through either planting or assisted regeneration, such as not re-clearing woody regrowth, is widely recognised as a method of sequestering C in above- and below-ground biomass, but there are very limited data on associated changes in SOC. Based on a single study on retention of brigalow regrowth on degraded pastures in Queensland rangelands, no significant SCS follows regeneration of forest cover in rangeland situations (Allen et al. 2016). Results of additional studies (Table A1) that modelled (Paul et al. 2022) or measured (Guo et al. 2008) tree plantation establishment on grassland were inconsistent, ranging from small increases in SOC stocks to a substantial loss. In summary, there were too few published data from rangeland sites to estimate SCS for land conversion from permanent pasture to forest cover, and investment in research is needed due to a growing interest in the prospects for SOC credits from establishing trees on areas of grazing land to add to opportunities for biomass C credits, ecosystem services payments and other possible environmental benefits.
Sources of inconsistent results between studies
Our review highlights a marked inconsistency between studies in the reported impacts on SCS of different management strategies in rangelands (Fig. 3). Understanding the reasons for conflicting outcomes is fundamental to interpreting the results and informing future research and action (Dynarski et al. 2020).
Estimated SCS (t C ha−1 year−1) for each of the rangeland management strategies described in Table 1, illustrating the variation between studies (blue dots) and the median value (yellow diamonds). Where a range was reported, the average has been plotted. Differences between studies reflect both known factors based on the published information (e.g. + or – nutrient additions for sown pasture species), and unknown causes, which may include site characteristics, legacy management effects, and measurement quality (accuracy and representativeness).
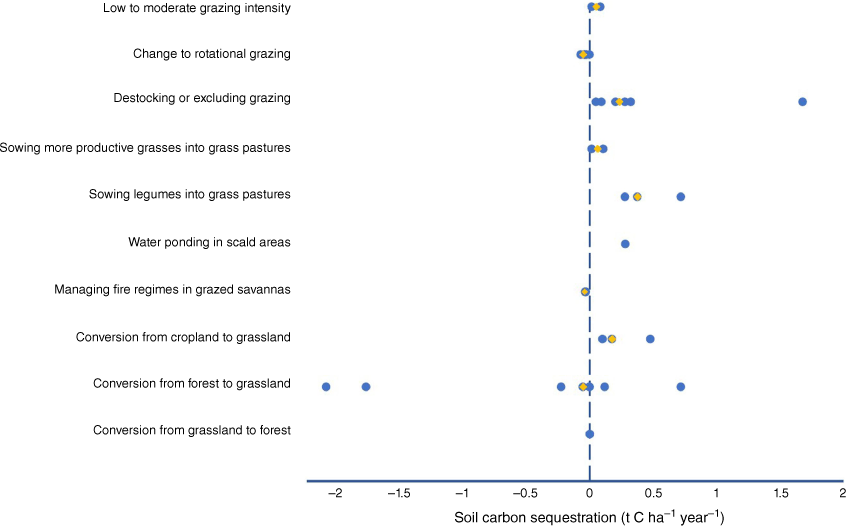
The diversity in climate, soil and landscape characteristics as well as in land use history and livestock production systems across Australia’s rangelands, mean that differences between locations in SOC storage will remain a challenge for predicting the potential for SCS (Bastin et al. 2009; Rabbi et al. 2015). However, unless attention is given to identifying and addressing reasons for different outcomes, including differences in protocols for quantifying SCS and variations in the implementation of management strategies in trials evaluating impacts on SOC, the capacity to develop evidence-based policy and make informed land management decisions related to SCS for climate change mitigation will be limited (Conant and Paustian 2004). Major sources of inconsistency are summarised in Table 2 and the discussion that follows seeks to explain the approach taken in evaluating evidence for SCS in this review, provide context for interpreting the results for global rangelands, and support a set of recommendations for improvements in future estimates of the potential for SCS.
Issue for SCS data | Elements for SCS data quality | Requirement for robust data and improved consistency across studies | |
---|---|---|---|
Definition of sequestration | Long-term increase in mass of stable SOC | For climate change mitigation and C credits, assess change in SOC stocks to ≥30 cm depth over multidecadal time periods | |
Sampling SOC | SOC stock change | Sample to ≥30 cm; Remove gravel, SOM > 2 mm, inorganic C; Measure bulk density; Estimate SOC stock change on ESM basis | |
Sampling design | Unbiased sampling (e.g. stratified random); sampling density that accounts for heterogeneity, Assess MDD across sampling depth and area of interest | ||
Sampling frequency | Period between measurement (usually ≥5 years) depending on rate of change, precision of analysis method, MDD | ||
Analysis of SOC stock change | Lab measurement: Dry combustion | Laboratory dry combustion for baseline and change period analysis in an accredited (preferably same) laboratory | |
Spectrometric measurement | Calibration data from appropriate sampling and laboratory dry combustion; Sampling density based on variance of estimates | ||
Remote sensing | Calibration data from appropriate sampling and laboratory dry combustion at scale; In-field verification; Not a reliable predictor of SOC at depth | ||
Flux measurements | Calibration using in-field data; Experienced expert data analysis; Monitoring over multiyear period over climate variability cycles | ||
Model predictions | Calibration and validation using independent in-field data; Verification using in-field data to ‘true-up’ model over multidecadal times; Model functionality for SOC dynamics |
Abbreviations: SOC, soil organic carbon; SCS, soil carbon sequestration; SOM, soil organic matter; MDD, minimum detectable difference; ESM, equivalent soil mass.
Over recent decades, ‘soil carbon sequestration’ has entered common usage in technical, policy and public interest literature, but the intent and interpretation of the term has not been consistent. Sequestration of organic carbon in soil is the process by which carbon is removed from the atmosphere as CO2 via photosynthesis and retained long-term in SOM (Baveye et al. 2023). In contrast, SOC storage does not have the same emphasis on stabilisation and persistence of additional SOC, a concept encompassed in the additionality requirement and permanence obligations of C crediting schemes (CER 2021). Stabilisation occurs over time when organic C that enters soil as organic matter, mainly plant residues, manure, root exudates and soil fauna and microbial necromass (Dynarski et al. 2020), becomes more physically or chemically protected from microbial breakdown and forms stable organo-mineral complexes able to persist for centuries or millennia (Moinet et al. 2023). For climate change mitigation and C offsets, sequestration is internationally accepted as meaning storage in the order of 100 years, although shorter periods (with discounted credit issuance) are permitted in some schemes and accounting methods, e.g. 25 years in the Australian government’s C crediting scheme (CER 2021). A high proportion of SOM, perhaps 90% or more, breaks down within a decade (Cotrufo and Lavallee 2022) releasing CO2 back to the atmosphere through the process of respiration mediated primarily by soil microbes. Importantly, although not all SOM counts as mitigating climate change, increasing SOM, labile or persistent, is fundamental to maintaining or improving soil condition and fertility (Lal et al. 2018; Cotrufo et al. 2019). The release of nutrients through rapidly cycling SOM is critical for plant growth, and especially in low productivity rangeland soils where application of chemical fertilisers is uncommon.
SCS is quantified as a long-term increase in the stock of organic carbon (t C ha−1 year−1) in soil. Carbon farming schemes issue a C credit for each 1 t CO2e sequestered after adjusting for any GHG emissions resulting from implementation of a new SOC-positive practice and according to any scheme-specific rules. The new practice must go beyond business-as-usual management to meet the ‘additionality’ requirement, which aims to ensure that only genuine climate change abatement is credited. Schemes may stipulate a list of practices that are eligible to earn SOC credits, which should be based on evidence of a positive link between the activity and SOC accumulation. However, establishing this evidence, and particularly causality, is very difficult due to the large number of interacting influences on SOC stocks.
In rangelands the dominant drivers of SOC change are generally climate and soil variables, and the smaller response to management can be difficult to detect and measure accurately (Allen et al. 2013; Rabbi et al. 2015; Baveye et al. 2023). Field studies established before climate change became a focus of the land management research commonly did not make an initial measurement of SOC stocks and did not establish a procedure to monitor a counterfactual (without practice change) baseline as part of the trial. In field trials, a dynamic baseline, ideally monitored over several decades, is now recognised as ‘best practice’ to quantify SCS attributable to a new agricultural management practice (Guan et al. 2023). A small number of surveys designed to compare practices in rangelands have sought to isolate the management influence by detrending SOC data for rainfall and other natural drivers of SCS (Allen et al. 2013).
Several recent reviews (Maillard et al. 2017; Paustian et al. 2019; Smith et al. 2020; Guan et al. 2023; Stanley et al. 2023) have discussed in detail SCS quantification methods and their limitations. The challenges of measuring small and variable changes in SOC stocks, which typically can be reliably measured only over periods of five or more years, are exacerbated by the vast and diverse nature of rangeland grazing systems (Sanderman et al. 2010; Lal 2019). Despite some promising research there are, as yet, no widely accepted measurement methods or verifiable modelling tools that are cost-effective, practical and accurate for routine use in these landscapes. The procedure considered most accurate is direct measurement using traditional in-field sampling and laboratory analysis of SOC content by the dry combustion technique, and this can be prohibitively expensive at the scale of rangeland monitoring. Methods currently used for estimating SCS in field studies each have significant uncertainty, and there is often limited capacity to analyse the confidence level in reported rates of SCS.
In-field soil sampling is usually the greatest cost in generating data for SCS measurement or for model calibration and verification, with average sampling costs typically one order of magnitude greater than laboratory analysis charges (de Gruijter et al. 2016). This cost can act as a barrier to establishing an effective sampling design that properly considers spatial and temporal diversity, depth, density and timing within a research budget for reliable estimation of SOC stock change.
Internationally established protocols for reporting SCS require sampling to at least 30 cm and to sufficient depth to enable SOC stock change to be expressed on an ESM basis. Where deeper sampling is possible, this may give more complete understanding of SCS, with recent research finding that approximately 20% of the impact of a management change on SOC stocks occurred below 30 cm (Skadell et al. 2023). For consistent comparisons in this review, data for 0–30 cm were selected wherever possible (Table 1), noting that, in rangeland sites, there may be physical constraints on sampling, such as rocky or steep terrain.
Although some C farming methods (e.g. Australian Government 2021) allow for a set number of samples across different managed land types, it is preferable that the density is adjusted based on spatial variation, and rate of change relative to the minimum detectable difference of the measurement method (FAO 2019; Stanley et al. 2023). Higher sampling error can occur in rangeland soils due to spatial heterogeneity resulting from features such as diverse topography, uneven rockiness, non-uniform soil horizons, patchy fertility and vegetation, and effects of uneven grazing and manure deposition, many of which are difficult to predict. Based on an analysis using linear mixed modelling for a long-term cattle stocking rate trial in north-east Australia (Wambiana Station, 20°34′S, 146°07′E), Pringle et al. (2011) recommended steps to calculate the density of sampling needed for measurement of mean SOC stocks (0–30 cm) to within 20% of the true mean in the tropical rangelands. Firstly, the property or project area is divided into units of apparently uniform soil type and grazing management (strata), before using stratified simple random sampling to distribute ≥25 sampling points in each unit, ensuring at least two samples in each stratum.
Minimum detectable difference provides a basis for the appropriate frequency of re-measurement, which is generally ≥5 years in rangelands (Chen et al. 2004). Samples should be taken as close as possible to the same time of year in repeat measurements. In a rangeland sampling strategy, monitoring should be continued for at least 20 years (Allen et al. 2010; Stanley et al. 2023), due to the impacts of interannual to decadal scale climate variations that affect rainfall and plant growth (Stafford Smith et al. 2007), or for the period required in scheme rules for a C farming project.
Laboratory analysis using dry combustion is widely accepted as the most accurate and precise method for quantifying SOC but, even in this method, there are small errors and interlaboratory differences that contribute to the total uncertainty in SCS data (Paustian et al. 2019). Although typically less than sampling costs, for an extensive rangeland project with adequate sampling density, dry combustion analysis costs ($10–20 AUD/sample, Singh et al. 2013) can be excessive in typical research budgets. Focused investment over more than one decade has sought to develop alternative approaches with adequate accuracy but taking candidates such as bench-top laboratory-based, in-situ field-based, and proximal and remote-sensing techniques through to routine SCS data acquisition with sufficiently low error and high confidence has proved difficult (Nayak et al. 2019; Orton et al. 2023). Although showing some success for rapid and feasible mapping of soil C at a site to regional scale (Nayak et al. 2019), the error in these novel methods remains greater than for the dry combustion method (Morgan and Ackerson 2022). Techniques such as eddy covariance have promise for extended monitoring over >10 years when combined with SOC stock measurements, and several options claim to provide value in calibrating modelling approaches, which may then be used to derive SCS (Smith et al. 2020).
The rate of change in SOC stocks relative to the baseline or initial level is not linear but varies with initial soil status and tends to decline over time since implementation of a new management practice. For example, an initial rapid increase in SOC in degraded land following improved management is commonly not sustained (Sanderman et al. 2017; Muleke et al. 2023), and these patterns of change in SCS likely contribute to apparent inconsistencies in SCS between studies (Table 1, Fig. 3). The inconsistences are more difficult to interpret because most data for SCS in Australian rangelands have been estimated from space-for-time studies, and even with close matching of paired sites, there can be error due to fine scale site variations in vegetation, soil clay content, slope and other characteristics shown to influence SOC stock change. The lack of information on management history and absence of a dynamic baseline contribute significantly to uncertainty and conflicting results (Pringle et al. 2011; Allen et al. 2016; Orgill et al. 2017; Muleke et al. 2023; Viscarra Rossel et al. 2023).
Process-based models offer potential to predict SCS due to adoption of new management (Stockmann et al. 2013; Hunt 2014; Smith et al. 2020; Albanito et al. 2022). However, their reliability for predicting SOC stock change is currently limited by availability of data for calibration and verification and by insufficient model functionality to fully represent SOM dynamics (Paustian et al. 2019; Smith et al. 2020). Data constraints are of particular significance in arid and tropical regions. A recent review found that 71% of models used for SOC simulations were not validated and, of the remainder, validation was limited in its coverage (Garsia et al. 2023). Factors affecting SOC dynamics and management strategies in rangelands have been demonstrated to be poorly represented in commonly used crop and pasture models (Badgery et al. 2020), and although development of functionality along with expansion of parameterisation data is occurring, it is likely that modelling approaches will initially have a greater role in larger scale estimates of SCS than at project scale (Smith et al. 2020).
Estimation of the climate change benefits of SCS-positive management strategies must account for any associated GHG emissions. Whether this adjustment is applied, and how accurately, affects the comparability of results from studies that report SCS, but is not always clearly reported. Sources of emissions in rangeland livestock systems may include N2O from synthetic fertiliser application or legumes and emissions associated with savanna burning, but the largest contribution is likely to be enteric CH4 if ruminant livestock numbers are increased to take advantage of higher carrying capacity, e.g. with pasture improvement strategies (Harrison et al. 2016; Tomkins et al. 2019; Rumpel et al. 2023). Schemes that credit SCS provide rules for estimating these emissions (e.g. Australian Government 2021).
Potential for SCS in global rangelands
Published studies for Australian rangelands provide evidence that, within the constraints of climate and soil drivers, certain management strategies may increase SOC storage in livestock production systems. The climate change mitigation benefit is likely constrained across much of the area, but it is nonetheless important to understand the potential for SCS for policy and land management decisions. From this review, the paucity in long-term data and variable or conflicting results provide insufficient evidence to predict statistically significant responses to management changes over multidecadal periods or to infer the potential for SCS across the extent of diverse Australian or global rangelands. Multiple location-specific factors can interact to influence the dynamics of SOC (Luo et al. 2017). The legacy effect of historic management together with fine scale variations in soil properties, rainfall and other climate factors determine the SOC response to implementation of a new management strategy. As for Australia, global data from longer term monitoring with robust baseline measurements and estimates from surveys detrended for dominant climate and soil influences are needed to quantify the SCS potential (Allen et al. 2013; Rabbi et al. 2015; Paustian et al. 2019).
Management strategies with consistent outcomes for increasing SOC storage include: (i) Sowing more productive grasses or legumes in existing grass pastures increases SOC stocks; (ii) Conversion of cropping land to permanent pasture results in SCS, influenced by management history and baseline soil condition; and (iii) prolonged high stocking rates are associated with net SOC loss relative to conservative stocking. Where credible Australian measurements are available for rotational grazing strategies, they support global data (e.g. Hawkins et al. 2022) indicating negligible impact on SOC stocks relative to continuous grazing. For rotational grazing and other strategies lacking adequate long-term monitoring, including waterponding or fire regime management, further research is recommended in field trials with controlled and treatment sites having robust baseline measurements and historical management data. Similar limitations in data and consistency of results have been identified in other Australian analyses (Sanderman et al. 2010; McDonald et al. 2023) and global publications (Derner and Schuman 2007; Dondini et al. 2023), with broadly consistent SCS trends reported for extensive livestock production systems (Conant et al. 2017; Sanderman et al. 2017; Bossio et al. 2020; McKenna et al. 2022; Moinet et al. 2023). Uncertainty remains regarding: (i) the maximum SOC storage capacity of different soils, and dynamics of change towards this projected saturation level; (ii) the permanency of sequestered SOC; and (iii) levels of associated GHG emissions, and these factors affect confidence in the potential for climate change mitigation and the integrity of SOC offsets that may be earned in rangeland C farming projects. They highlight the need for caution in extrapolating results between sites to estimate the potential for SCS (Janzen et al. 2022).
Co-benefits, trade-offs and risks for potential rangeland SCS
Although available evidence from rangelands indicates that the prospects for permanent SCS per hectare is constrained by soil and climate factors, the fundamental benefits of SOM for soil condition and fertility, and for strong plant growth are well accepted (Kopittke et al. 2022). Descriptions of co-benefits for practices that are promoted to foster enhanced levels of SOM (and SOC) include improved ecosystem services (Bossio et al. 2020) and agricultural production (Paustian et al. 2016), mediated by better soil quality and soil function (Rumpel and Chabbi 2021). Co-benefits that may make uptake of management strategies attractive to rangeland livestock producers despite the uncertainty of SCS and C farming prospects include enhanced soil quality, sustainable production, and more climate resilient ecosystems and grazing businesses (Henry et al. 2018; Baveye et al. 2020; Hoffland et al. 2020; Page et al. 2020). Targeting degraded sites depleted of SOC due to historic mismanagement can increase these co-benefits as well as enhancing the prospects for SCS.
Before adopting a management strategy for SCS, consideration should also be given to possible biophysical and socio-economic trade-offs (Rumpel et al. 2023). Practices that foster long-term storage of stabilised SOC provide climate change abatement, but stoichiometric constraints result in immobilisation of N and other nutrients associated with the soil fertility benefits (van Groenigen et al. 2017; Soussana et al. 2019; Schlesinger 2022). In the decomposition of more labile SOM, these nutrients are released while returning CO2 to the atmosphere (Kirkby et al. 2011; Kirkby et al. 2013). The extent of the possible trade-off between SCS and productivity remains unresolved, with ongoing research on the optimisation of agricultural land management for multiple objectives (Janzen 2006; Peck et al. 2011; Ma et al. 2023; Rumpel et al. 2023). Rangeland soils, such as those in Australia, are typically low in nutrients, notably N, (e.g. Conrad et al. 2017), and targeted research is needed in these landscapes to understand potential trade-offs and pathways for stabilisation of SOC across soil types.
In addition to biophysical impacts, social and economic co-benefits and trade-offs are possible due to changes in management practices in livestock production systems (Baumber et al. 2020). Implementing a new management strategy may involve substantial time and resource allocation, and loss of flexibility occurs if the producer enters into a C credit contract with a permanency obligation that may be for 25 or 100 years (Australian Government 2021). Socio-economic concerns are known to affect uptake of SCS-positive strategies and participation in C crediting schemes (Macintosh et al. 2019; Barbato and Strong 2023; Henry et al. 2023). As C markets have continued to evolve alongside expectations of corporate climate disclosure and ecosystem services markets (Kreibich and Hermwille 2021; Cotton and Witt 2024), there has also been increasing focus on integrated approaches that consider the multiple interests, benefits and trade-offs of climate change mitigation actions. Of relevance to SCS in rangelands, are policies and frameworks that offer financial incentives additional to C offset market opportunities for positive environmental and social outcomes delivered by C farming projects (Sonter et al. 2020). Premiums are generally based on accounting standards such as those developed by some Australian state governments (Department of Agriculture and Food WA 2022; Queensland Government 2023) and in similar accounting and payment schemes internationally. As for SCS, the associated benefits and trade-offs are context-specific, variable and difficult to quantify and verify. The possibility of a change in management providing multiple economic benefits for a livestock production enterprise through ecosystem services credits and price premiums for products labelled as ‘environmentally sustainable’ may foster adoption but could challenge eligibility for C credits. Most C farming schemes (e.g. Australian Government 2021) seek to ensure the integrity of C offsets by requiring credits to be issued only for sequestration that is ‘additional’ to business-as-usual and would not occur in the absence of the incentive provided by the C farming scheme. Additionality standards generally allow for productivity or income from co-benefits associated with a new management strategy, but the extent to which these become common practice for economic advantage in farm business may lead to future changes.
Understanding of co-benefits and trade-offs associated with management for enhanced SCS is evolving (Harrison et al. 2021; Rumpel et al. 2023; Viscarra Rossel et al. 2023), in association with protocols to account for SCS within frameworks that also credit multiple ecosystem services (Bossio et al. 2020; Sonter et al. 2020). Of critical importance is ensuring the integrity of C credits and managing the level of financial or legal risk for scheme participants. An overarching risk to the potential for SCS is the impact of climate change. Rising temperatures and increasingly unreliable and extreme rainfall have been identified as threats to both ongoing accumulation and maintenance of existing SOC stocks (Roxburgh et al. 2020), and especially in rangelands, which are predicted to experience amongst the most severe climate changes (ILRI et al. 2021; IPCC 2023).
Conclusions and recommendations
Using Australian rangelands as a case study, this review sought to evaluate the evidence for implementation of changes in management strategies in extensive livestock production systems to result in SCS in rangelands. Understanding the potential for SCS in these vast landscapes is crucial for effective climate change, agricultural and natural resource policies, and for land managers’ decisions relating to livestock production systems. However, the paucity of data from long-term field studies or verified model simulations combined with the diversity of rangelands and complexity of SOC dynamics constrained conclusions from this analysis. Overarching observations were that: (i) very few publications provide data consistent with internationally accepted protocols for quantifying SCS; and (ii) available results were often conflicting, due in part to the range of interacting factors that influence SOC levels and persistence.
Despite climate, soil, and landscape factors being the dominant determinants of SOC stocks in rangelands (Allen et al. 2013), there was evidence from published Australian data for location-specific management effects. Strategies that were more consistently positive for SCS include sowing more productive grasses or legumes within existing grass pastures and maintaining conservative stocking rates to avoid net SOC loss under prolonged high intensity grazing. Excluding livestock or all grazing may increase SOC stocks while improving land condition, but the effect appears small and is detected most often in degraded areas. In the studies reviewed there was no significant difference in SCS between rotational grazing (managing timing of grazing and rest periods) and continuous grazing strategies. Less well-studied strategies, such as waterponding, for which there is some evidence of significant benefits for SCS in the initial years from implementation, warrant further research to determine longer term impacts.
As in our review, other Australian (McDonald et al. 2023) and international (Khalil et al. 2019; Hawkins et al. 2022) analyses have reported high uncertainty, demonstrating that current scientific evidence is insufficient to provide confidence in the SCS potential in rangelands or to ensure the integrity of SOC offsets for credible net-zero claims and effective C markets. There is high uncertainty in the permanency of sequestration in rangelands, and this is exacerbated by the risk to both accumulation and maintenance of SOC stocks due to projected anthropogenic climate change impacts (Roxburgh et al. 2020).
To help address areas of uncertainty in the potential for SCS in rangelands, investment in addressing data and knowledge gaps is needed with targeted research on the threats to the integrity of SCS for climate change mitigation. Priority should be given to continuing, and expanding, long-term trials with regionally relevant management practices. Survey techniques and resampling of past measurement sites can also supplement existing data in the short term. To meet the needs of multiple stakeholders and increase the transparency of SCS actions and accounting, partnerships should be fostered to improve data-sharing arrangements and educational material providing access to factual information for rangeland systems and realistic assessments of challenges and opportunities for SCS in livestock production systems. The sources of inconsistency in published studies discussed in this review provide guidance on ensuring more reliable and accurate data on SOC stock changes and the potential for SCS with different management strategies.
Three specific recommendations to increase the value of field studies and improve the capacity to predict and quantify SCS, will support rangeland management decisions and policy development into the future:
Invest in improved understanding of the spatial and temporal variability in rangelands as a basis for designing sampling protocols and multidecadal monitoring programs that can provide high-quality data on SOC stocks as a resource for accurate SCS modelling approaches linked to locally relevant management strategies.
Design and maintain long-term field studies that incorporate a dynamic baseline and monitoring programs to facilitate statistical analysis and attribution of change in SOC stocks between management and exogenous (climate, soil, landscape) drivers to provide a stronger evidence base for assessing additionality and the benefits of adoption of specific practices.
Support innovation for cost-effective and accurate quantification of small changes in SOC stocks, including improved capacity of process models and statistical analytics to more accurately simulate the dynamics of SOC in response to natural and management factors by integrating data from multiple sources and promising technological advances in spectrometry, remote sensing and machine learning.
Conflicts of interest
Two authors (SB and SMcD) are Associate Editors of The Rangeland Journal. To mitigate this potential conflicts of interest they had no editor-level access to this manuscript during peer review. The authors have no further conflicts of interest to declare.
Acknowledgements
The support of Queensland Department of Agriculture and Fisheries, Australia for the literature review relating to northern Australian rangelands is gratefully acknowledged. We thank anonymous reviewers for very helpful comments on the manuscript.
References
ABARES (2021) ‘Snapshot of Australian Agriculture 2021.’ (Australian Bureau of Agricultural and Resource Economics and Sciences: Canberra) 10.25814/rxjx-3g23 [accessed 14 April 2023]
ACRIS (2008) Taking the Pulse. National Land and Water Resources Audit, Canberra, ACT. Bastin, G. and the ACRIS Management Committee. Australian Collaborative Rangeland Information System. Available at https://www.dcceew.gov.au/environment/land/publications/acris-rangelands-2008-taking-pulse [accessed 14 April 2023]
Albanito F, McBey D, Harrison M, Smith P, Ehrhardt F, Bhatia A, et al. (2022) How modelers model: the overlooked social and human dimensions in model intercomparison studies. Environmental Science & Technology 56, 13485-13498.
| Crossref | Google Scholar | PubMed |
Alexandratos N, Bruinsma J (2012) ‘World agriculture towards 2030/2050: the 2012 revision’. ESA Working paper No. 12-03. (Food and Agriculture Organization: Rome) Available at https://www.fao.org/3/ap106e/ap106e.pdf [accessed 14 August 2023]
Allen DE, Pringle MJ, Page KL, Dalal RC (2010) A review of sampling designs for the measurement of soil organic carbon in Australian grazing lands. The Rangeland Journal 32, 227-246.
| Crossref | Google Scholar |
Allen DE, Pringle MJ, Bray S, Hall TJ, O’Reagain PO, Phelps D, Cobon DH, Bloesch PM, Dalal RC (2013) What determines soil organic carbon stocks in the grazing lands of north-eastern Australia? Soil Research 51, 695-706.
| Crossref | Google Scholar |
Allen DE, Pringle MJ, Butler DW, Henry BK, Bishop TFA, Bray SG, et al. (2016) Effects of land-use change and management on soil carbon and nitrogen in the Brigalow Belt, Australia: I. Overview and inventory. The Rangeland Journal 38, 443-452.
| Crossref | Google Scholar |
Allen DE, Bloesch PM, Cowley RA, Orton TG, Payne JE, Dalal RC (2021) Impacts of fire on soil organic carbon stocks in a grazed semi-arid tropical Australian savanna: accounting for landscape variability. The Rangeland Journal 43(4), 281-282.
| Crossref | Google Scholar |
ARS (2023) Australian Rangeland Society. Available at https://austrangesoc.com.au/ [accessed 12 May 2023]
Badgery WB, Simmons AT, Murphy BW, Rawson A, Andersson KO, Lonergan VE (2014) The influence of land use and management on soil carbon levels for crop-pasture systems in Central New South Wales, Australia. Agriculture, Ecosystems and Environment 196, 147-157.
| Crossref | Google Scholar |
Badgery WB, Mwendwa JM, Anwar MR, Simmons AT, Broadfoot KM, Rohan M, Singh BP (2020) Unexpected increases in soil carbon eventually fell in low rainfall farming systems. Journal of Environmental Management 261, 110192.
| Crossref | Google Scholar | PubMed |
Badgery W, Murphy B, Cowie A, Orgill S, Rawson A, Simmons A, Crean J (2021) Soil carbon market-based instrument pilot–the sequestration of soil organic carbon for the purpose of obtaining carbon credits. Soil Research 59, 12-23.
| Crossref | Google Scholar |
Barbato CT, Strong AL (2023) Farmer perspectives on carbon markets incentivizing agricultural soil carbon sequestration. npj Climate Action 2, 26.
| Crossref | Google Scholar |
Bartley R, Abbott BN, Ghahramani A, Ali A, Kerr R, Roth CH, Kinsey-Henderson A (2023) Do regenerative grazing management practices improve vegetation and soil health in grazed rangelands? Preliminary insights from a space-for-time study in the Great Barrier Reef catchments, Australia. The Rangeland Journal 44, 221-246.
| Crossref | Google Scholar |
Bastin GN, Smith DS, Watson IW, Fisher A (2009) The Australian Collaborative Rangelands Information System: preparing for a climate of change. The Rangeland Journal 31, 111-125.
| Crossref | Google Scholar |
Baumber A, Waters C, Cross R, Metternicht G, Simpson M (2020) Carbon farming for resilient rangelands: people, paddocks and policy. The Rangeland Journal 42, 293-307.
| Crossref | Google Scholar |
Baveye PC, Schnee LS, Boivin P, Laba M, Radulovich R (2020) Soil organic matter research and climate change: merely re-storing carbon versus restoring soil functions. Frontiers in Environmental Science 8, 579904.
| Crossref | Google Scholar |
Baveye PC, Berthelin J, Tessier D, Lemaire G (2023) Storage of soil carbon is not sequestration: straightforward graphical visualization of their basic differences. European Journal of Soil Science 74, e13380.
| Crossref | Google Scholar |
Begill N, Don A, Poeplau C (2023) No detectable upper limit of mineral‐associated organic carbon in temperate agricultural soils. Global Change Biology 29, 4662-4669.
| Crossref | Google Scholar | PubMed |
Bossio DA, Cook-Patton SC, Ellis PW, Fargione J, Sanderman J, Smith P, Wood S, Zomer R J, von Unger M, Emmer IM, Griscom BW (2020) The role of soil carbon in natural climate solutions. Nature Sustainability 3, 391-398.
| Crossref | Google Scholar |
Bray S, Doran-Browne N, O’Reagain P (2014) Northern Australian pasture and beef systems. 1. Net carbon position. Animal Production Science 54, 1988-1994.
| Crossref | Google Scholar |
Bray SG, Allen DE, Harms BP, Reid DJ, Fraser GW, Dalal RC, Walsh D, Phelps DG, Gunther R (2016) Is land condition a useful indicator of soil organic carbon stock in Australia’s northern grazing land?. The Rangeland Journal 38, 229-243.
| Crossref | Google Scholar |
Burrows WH, Henry BK, Back PV, Hoffmann MB, Tait LJ, Anderson ER, Menke N, Danaher T, Carter JO, McKeon GM (2002) Growth and carbon stock change in eucalypt woodlands in northeast Australia: ecological and greenhouse sink implications. Global Change Biology 8, 769-784.
| Crossref | Google Scholar |
CER (2021) Understanding your soil carbon project: Emissions Reduction Fund simple method guide for soil carbon projects registered under the Carbon Credits (Carbon Farming Initiative – Estimation of Soil Organic Carbon Sequestration using Measurement and Models) Methodology Determination 2021. Available at https://cer.gov.au/document/understanding-your-soil-carbon-project-simple-method-guide [accessed 26 July 2022]
Chan KY, Oates A, Li GD, Conyers MK, Prangnell RJ, Poile G, et al. (2010) Soil carbon stocks under different pastures and pasture management in the higher rainfall areas of south-eastern Australia. Soil Research 48, 7-15.
| Crossref | Google Scholar |
Chan KY, Conyers MK, Li GD, Helyar KR, Poile G, Oates A, Barchia IM (2011) Soil carbon dynamics under different cropping and pasture management in temperate Australia: results of three long-term experiments. Soil Research 49, 320-328.
| Crossref | Google Scholar |
Chen X, Eamus D, Hutley LB (2004) Seasonal patterns of fine-root productivity and turnover in a tropical savanna of northern Australia. Journal of Tropical Ecology 20, 221-224.
| Crossref | Google Scholar |
Chen W, Huang D, Liu N, Zhang Y, Badgery WB, Wang X, Shen Y (2015) Improved grazing management may increase soil carbon sequestration in temperate steppe. Scientific Reports 5, 10892.
| Crossref | Google Scholar | PubMed |
Conant RT, Paustian K (2004) Grassland management activity data: current sources and future needs. Environmental Management 33, 467-473.
| Crossref | Google Scholar | PubMed |
Clewett JF (2015) Pasture measurements and bio-economic analyses to assess the effects of climate, grazing pressure and pasture rundown on soil carbon and returns from legume-based sown pastures in the Condamine region of Southern Queensland. 74 pp. Final Report to Condamine Alliance on project AOTGR1-137 Increasing Soil Carbon in Degraded Cropping and Grazing Land. (Agroclim Australia: Toowoomba, Qld)
Conant RT, Paustian K, Elliott ET (2001) Grassland management and conversion into grassland: effects on soil carbon. Ecological Applications 11, 343-355.
| Crossref | Google Scholar |
Conant RT, Cerri CE, Osborne BB, Paustian K (2017) Grassland management impacts on soil carbon stocks: a new synthesis. Ecological Applications 27, 662-668.
| Crossref | Google Scholar | PubMed |
Conrad KA, Dalal RC, Dalzell SA, Allen DE, Menzies NW (2017) The sequestration and turnover of soil organic carbon in subtropical leucaena-grass pastures. Agriculture, Ecosystems and Environment 248, 38-47.
| Crossref | Google Scholar |
Cotrufo MF, Ranalli MG, Haddix ML, Six J, Lugato E (2019) Soil carbon storage informed by particulate and mineral-associated organic matter. Nature Geoscience 12, 989-994.
| Crossref | Google Scholar |
Cotrufo MF, Lavallee JM, Six J, Lugato E (2023) The robust concept of mineral‐associated organic matter saturation: a letter to Begill et al., 2023. Global Change Biology 29, 5986-5987.
| Crossref | Google Scholar | PubMed |
Cotton R, Witt B (2024) Carbon and ecosystem service markets in rangelands and grazing systems are a wicked problem: multi-stakeholder partnership or roundtable as a vehicle forward? The Rangeland Journal 46, RJ23029.
| Crossref | Google Scholar |
Cowie AL, Lonergan VE, Rabbi SF, Fornasier F, Macdonald C, Harden S, Kawasaki A, Singh BK (2013) Impact of carbon farming practices on soil carbon in northern New South Wales. Soil Research 51, 707-718.
| Crossref | Google Scholar |
Dalal RC, Harms B, Krull E, Wang W (2005) Total soil organic matter and its labile pools following Mulga (Acacia aneura) clearing for pasture development and cropping 1. Total and labile carbon. Australian Journal of Soil Research 43, 13-20.
| Crossref | Google Scholar |
Dalal RC, Cowie BA, Allen DE, Yo SA (2011) Assessing carbon lability of particulate organic matter from δ13C changes following land-use change from C3 native vegetation to C4 pasture. Soil Research 49, 98-103.
| Crossref | Google Scholar |
Dalal RC, Thornton CM, Allen DE, Owens JS, Kopittke PM (2021) Long-term land use change in Australia from native forest decreases all fractions of soil organic carbon, including resistant organic carbon, for cropping but not sown pasture. Agriculture, Ecosystems and Environment 311, 107326.
| Crossref | Google Scholar |
Daryanto S, Eldridge DJ, Throop HL (2013) Managing semi-arid woodlands for carbon storage: grazing and shrub effects on above-and belowground carbon. Agriculture, Ecosystems and Environment 169, 1-11.
| Crossref | Google Scholar |
de Gruijter JJ, McBratney AB, Minasny B, Wheeler I, Malone BP, Stockmann U (2016) Farm-scale soil carbon auditing. Geoderma 265, 120-130.
| Crossref | Google Scholar |
Department of Agriculture and Food WA (2022) WA Carbon Farming and Land Restoration Program. ACCU Plus Guidelines, August 2022. Available at www.agric.wa.gov.au/CF-LRP [accessed 28 January 2024].
Derner JD, Schuman GE (2007) Carbon sequestration and rangelands: a synthesis of land management and precipitation effects. Journal of Soil and Water Conservation 62, 77-85.
| Google Scholar |
Don A, Schumacher J, Freibauer A (2011) Impact of tropical land‐use change on soil organic carbon stocks–a meta‐analysis. Global Change Biology 17, 1658-1670.
| Crossref | Google Scholar |
Dondini M, Martin M, De Camillis C, Uwizeye A, Soussana J-F, Robinson T, Steinfeld H (2023) Global assessment of soil carbon in grasslands – From current stock estimates to sequestration potential. FAO Animal Production and Health Paper No. 187. (Food and Agriculture Organization: Rome, Italy) 10.4060/cc3981en [accessed 14 August 2023]
Dynarski KA, Bossio DA, Scow KM (2020) Dynamic stability of soil carbon: reassessing the “permanence” of soil carbon sequestration. Frontiers in Environmental Science 8, 514701.
| Crossref | Google Scholar |
Eldridge DJ, Delgado‐Baquerizo M, Travers SK, Val J, Oliver I (2017) Do grazing intensity and herbivore type affect soil health? Insights from a semi‐arid productivity gradient. Journal of Applied Ecology 54, 976-985.
| Crossref | Google Scholar |
Fensham R, Fairfax R (2008) Water-remoteness for grazing relief in Australian arid-lands. Biological Conservation 141, 1447-1460.
| Crossref | Google Scholar |
Foran B, Smith MS, Burnside D, Andrew M, Blesing D, Forrest K, Taylor J (2019) Australian rangeland futures: time now for systemic responses to interconnected challenges. The Rangeland Journal 41, 271-292.
| Crossref | Google Scholar |
Gardiner C, Kempe N, Hannah I, McDonald J (2013) PROGARDES: a legume for tropical/subtropical semi-arid clay soils. Tropical Grasslands-Forrajes Tropicales 1, 78-80.
| Crossref | Google Scholar |
Garsia A, Moinet A, Vazquez C, Creamer RE, Moinet GYK (2023) The challenge of selecting an appropriate soil organic carbon simulation model: a comprehensive global review and validation assessment. Global Change Biology 29, 5760-5774.
| Crossref | Google Scholar | PubMed |
Ghosh PK, Mahanta SK (2014) Carbon sequestration in grassland systems. Range Management and Agroforestry 35, 173-181.
| Google Scholar |
Grover SPP, Livesley SJ, Hutley LB, Jamali H, Fest B, Beringer J, et al. (2012) Land use change and the impact on greenhouse gas exchange in north Australian savanna soils. Biogeosciences 9, 423-437.
| Crossref | Google Scholar |
Guan K, Jin Z, Peng B, Tang J, DeLucia EH, West PC, et al. (2023) A scalable framework for quantifying field-level agricultural carbon outcomes. Earth-Science Reviews 243, 104462.
| Crossref | Google Scholar |
Guo LB, Gifford RM (2002) Soil carbon stocks and land use change: a meta analysis. Global Change Biology 8, 345-360.
| Crossref | Google Scholar |
Guo LB, Cowie AL, Montagu KD, Gifford RM (2008) Carbon and nitrogen stocks in a native pasture and an adjacent 16-year-old Pinus radiata D. Don. plantation in Australia. Agriculture, Ecosystems and Environment 124, 205-218.
| Crossref | Google Scholar |
Hall TJ, Silcock RG, Mayer DG (2020) Grazing pressure and tree competition affect cattle performance and native pastures in Eucalypt woodlands of Queensland, north-eastern Australia. Animal Production Science 60, 953-966.
| Crossref | Google Scholar |
Harms BP, Dalal RC, Cramp AP (2005) Changes in soil carbon and soil nitrogen after tree clearing in the semi-arid rangelands of Queensland. Australian Journal of Botany 53, 639-650.
| Crossref | Google Scholar |
Harrison MT, McSweeney C, Tomkins NW, Eckard RJ (2015) Improving greenhouse gas emissions intensities of subtropical and tropical beef farming systems using Leucaena leucocephala. Agricultural Systems 136, 138-146.
| Crossref | Google Scholar |
Harrison MT, Cullen BR, Tomkins NW, McSweeney C, Cohn P, Eckard RJ (2016) The concordance between greenhouse gas emissions, livestock production and profitability of extensive beef farming systems. Animal Production Science 56, 370-384.
| Crossref | Google Scholar |
Harrison MT, Cullen BR, Mayberry DE, Cowie AL, Bilotto F, Badgery WB, Liu K, Davison T, Christie KM, Muleke A, Eckard RJ (2021) Carbon myopia: the urgent need for integrated social, economic and environmental action in the livestock sector. Global Change Biology 27, 5726-5761.
| Crossref | Google Scholar | PubMed |
Hawkins HJ, Venter ZS, Cramer MD (2022) A holistic view of Holistic Management: what do farm-scale, carbon, and social studies tell us? Agriculture, Ecosystems & Environment 323, 107702.
| Crossref | Google Scholar |
Henry B (2023) ‘Potential for soil carbon sequestration in Northern Australian grazing lands: A review of the evidence.’ (Department of Agriculture and Fisheries: Qld) Available at www.futurebeef.com.au [accessed 14 August 2023]
Henry B, Murphy B, Cowie A (2018) ‘Sustainable Land Management for Environmental Benefits and Food Security. A Synthesis Report for the Global Environment Fund.’ (United Nations Environment Program) Available at www.unep.org/
Hoffland E, Kuyper TW, Comans RN, Creamer RE (2020) Eco-functionality of organic matter in soils. Plant and Soil 455, 1-22.
| Crossref | Google Scholar |
Hunt LP (2014) Aboveground and belowground carbon dynamics in response to fire regimes in the grazed rangelands of northern Australia: initial results from field studies and modelling. The Rangeland Journal 36, 347-358.
| Crossref | Google Scholar |
ILRI IUCN FAO WWF UNEP ILC (2021) ‘Rangelands Atlas.’ (Nairobi, Kenya: ILRI) International Livestock Research Institute. Available at https://www.rangelandsdata.org/atlas/ [accessed 14 August 2023]
IPCC (2023) Climate Change 2023: Synthesis Report. A Report of the Intergovernmental Panel on Climate Change. In ‘Contribution of Working Groups I, II and III to the Sixth Assessment Report of the Intergovernmental Panel on Climate Change’. (Core Writing Team, Eds H Lee, J Romero) (Intergovernmental Panel on Climate Change: Geneva, Switzerland)
Janzen HH (2006) The soil carbon dilemma: shall we hoard it or use it? Soil Biology and Biochemistry 38, 419-424.
| Crossref | Google Scholar |
Janzen HH, van Groenigen KJ, Powlson DS, Schwinghamer T, van Groenigen JW (2022) Photosynthetic limits on carbon sequestration in croplands. Geoderma 416, 115810.
| Crossref | Google Scholar |
Jones AR, Orton TG, Dalal RC (2016) The legacy of cropping history reduces the recovery of soil carbon and nitrogen after conversion from continuous cropping to permanent pasture. Agriculture, Ecosystems and Environment 216, 166-176.
| Crossref | Google Scholar |
Kästner M, Miltner A, Thiele-Bruhn S, Liang C (2021) Microbial necromass in soils—linking microbes to soil processes and carbon turnover. Frontiers in Environmental Science 9, 756378.
| Crossref | Google Scholar |
Khalil MI, Francaviglia R, Henry B, Klumpp K, Koncz P, Llorente M, Madari BE, Muñoz-Rojas M, Nerger R (2019) Strategic management of grazing grassland systems to maintain and increase organic carbon in soils. In ‘CO2 Sequestration’. (Eds LA Frazão, AM Silva Olaya, J Cota) pp. 45–65. (IntechOpen: London, UK)
Kirkby CA, Kirkegaard JA, Richardson AE, Wade LJ, Blanchard C, Batten G (2011) Stable soil organic matter: a comparison of C:N:P:S ratios in Australian and other world soils. Geoderma 163, 197-208.
| Crossref | Google Scholar |
Kirkby CA, Richardson AE, Wade LJ, Batten GD, Blanchard C, Kirkegaard JA (2013) Carbon-nutrient stoichiometry to increase soil carbon sequestration. Soil Biology and Biochemistry 60, 77-86.
| Crossref | Google Scholar |
Kopittke PM, Berhe AA, Carrillo Y, Cavagnaro TR, Chen D, Chen QL, et al. (2022) Ensuring planetary survival: the centrality of organic carbon in balancing the multifunctional nature of soils. Critical Reviews in Environmental Science and Technology 52, 4308-4324.
| Crossref | Google Scholar |
Kreibich N, Hermwille L (2021) Caught in between: credibility and feasibility of the voluntary carbon market post-2020. Climate Policy 21, 939-957.
| Crossref | Google Scholar |
Laganiere J, Angers DA, Pare D (2010) Carbon accumulation in agricultural soils after afforestation: a meta‐analysis. Global Change Biology 16, 439-453.
| Crossref | Google Scholar |
Lal R (2019) Carbon cycling in global drylands. Current Climate Change Reports 5, 221-232.
| Crossref | Google Scholar |
Lal R, Smith P, Jungkunst HF, Mitsch WJ, Lehmann J, Nair PR, McBratney AB, de Moraes Sá JC, Schneider J, Zinn YL, Skorupa AL (2018) The carbon sequestration potential of terrestrial ecosystems. Journal of Soil and Water Conservation 73, 145A-152A.
| Crossref | Google Scholar |
Lavallee JM, Soong JL, Cotrufo MF (2020) Conceptualizing soil organic matter into particulate and mineral-associated forms to address global change in the 21st century. Global Change Biology 26, 261-273.
| Crossref | Google Scholar | PubMed |
Liu X, Zhang B, Henry B, Zhang J, Grace P (2017) Assessing the impact of historical and future climate change on potential natural vegetation types and net primary productivity in Australian grazing lands. The Rangeland Journal 39, 387-400.
| Crossref | Google Scholar |
Livesley SJ, Bristow M, Grover SP, Beringer J, Arndt SK, Hutley LB (2021) Soil carbon density can increase when Australian savanna is converted to pasture, but may not change under intense cropping systems. Agriculture, Ecosystems and Environment 319, 107527.
| Crossref | Google Scholar |
Luo Z, Feng W, Luo Y, Baldock J, Wang E (2017) Soil organic carbon dynamics jointly controlled by climate, carbon inputs, soil properties and soil carbon fractions. Global Change Biology 23, 4430-4439.
| Crossref | Google Scholar | PubMed |
Ma Y, Woolf D, Fan M, Qiao L, Li R, Lehmann J (2023) Global crop production increase by soil organic carbon. Nature Geoscience 16, 1159-1165.
| Crossref | Google Scholar |
Macintosh A, Roberts G, Buchan S (2019) ‘Improving carbon markets to increase farmer participation.’ AgriFutures Australia Publication No. 19-026. (Commonwealth of Australia) Available at https://agrifutures.com.au/product/improving-carbon-markets-participation/ [accessed 20 August 2023]
Maillard É, McConkey BG, Angers DA (2017) Increased uncertainty in soil carbon stock measurement with spatial scale and sampling profile depth in world grasslands: a systematic analysis. Agriculture, Ecosystems and Environment 236, 268-276.
| Crossref | Google Scholar |
Matthews HD, Zickfeld K, Koch A, Luers A (2023) Accounting for the climate benefit of temporary carbon storage in nature. Nature Communications 14, 5485.
| Crossref | Google Scholar | PubMed |
McDonald SE, Badgery W, Clarendon S, Orgill S, Sinclair K, Meyer R, Butchart DB, Eckard R, Rowlings D, Grace P, Doran-Browne N, Harden S, Macdonald A, Wellington M, Pachas A, Eisner R, Amidy M, Harrison MT (2023) Grazing management for soil carbon in Australia: a review. Journal of Environmental Management 347, 119146.
| Crossref | Google Scholar | PubMed |
McKenna MD, Grams SE, Barasha M, Antoninka AJ, Johnson NC (2022) Organic and inorganic soil carbon in a semi-arid rangeland is primarily related to abiotic factors and not livestock grazing. Geoderma 419, 115844.
| Crossref | Google Scholar |
Minasny B, Malone BP, McBratney AB, Angers DA, Arrouays D, Chambers A, Chaplot V, Chen ZS, Cheng K, Das BS, Field DJ (2017) Soil carbon 4 per mille. Geoderma 292, 59-86.
| Crossref | Google Scholar |
Moinet GYK, Hijbeek R, van Vuuren DP, Giller KE (2023) Carbon for soils, not soils for carbon. Global Change Biology 29, 2384-2398.
| Crossref | Google Scholar | PubMed |
Morgan CL, Ackerson JP (2022) Sampling design for quantifying soil organic carbon stock in production Ag fields. Crops & Soils 55, 28-33.
| Crossref | Google Scholar |
Muleke A, Harrison MT, Eisner R, Yanotti M, de Voil P, Fahad S, et al. (2023) Clarifying confusions over carbon conclusions: antecedent soil carbon drives gains realised following intervention. Global Environmental Change Advances 1, 100001.
| Crossref | Google Scholar |
Nayak AK, Rahman MM, Naidu R, Dhal B, Swain CK, Nayak AD, et al. (2019) Current and emerging methodologies for estimating carbon sequestration in agricultural soils: a review. Science of The Total Environment 665, 890-912.
| Crossref | Google Scholar | PubMed |
Olff H, Ritchie ME (1998) Effects of herbivores on grassland plant diversity. Trends in Ecology & Evolution 13, 261-265.
| Crossref | Google Scholar | PubMed |
Orgill SE, Condon JR, Conyers MK, Greene RSB, Morris SG, Murphy BW (2014) Sensitivity of soil carbon to management and environmental factors within Australian perennial pasture systems. Geoderma 214, 70-79.
| Crossref | Google Scholar |
Orgill SE, Waters CM, Melville G, Toole I, Alemseged Y, Smith W (2017) Sensitivity of soil organic carbon to grazing management in the semi-arid rangelands of south-eastern Australia. The Rangeland Journal 39, 153-167.
| Crossref | Google Scholar |
Orgill SE, Condon JR, Conyers MK, Morris SG, Alcock DJ, Murphy BW, Greene RSB (2018) Removing grazing pressure from a native pasture decreases soil organic carbon in southern New South Wales, Australia. Land Degradation and Development 29, 274-283.
| Crossref | Google Scholar |
Orton TG, Thornton CM, Page KL, Dalal RC, Allen DE, Dang YP (2023) Evaluation of remotely sensed imagery to monitor temporal changes in soil organic carbon at a long-term grazed pasture trial. Ecological Indicators 154, 110614.
| Crossref | Google Scholar |
Page KL, Dang YP, Dalal RC (2020) The ability of conservation agriculture to conserve soil organic carbon and the subsequent impact on soil physical, chemical, and biological properties and yield. Frontiers in Sustainable Food Systems 4, 31.
| Crossref | Google Scholar |
Pahl L (2019) Macropods, feral goats, sheep and cattle. 2. Equivalency in what and where they eat. The Rangeland Journal 41, 519-533.
| Crossref | Google Scholar |
Paul KI, Polglase PJ, Nyakuengama JG, Khanna PK (2002) Change in soil carbon following afforestation. Forest Ecology and Management 168, 241-257.
| Crossref | Google Scholar |
Paul KI, England JR, Roxburgh SH (2022) Carbon dynamics in tree plantings: how changes in woody biomass impact litter and soil carbon. Forest Ecology and Management 521, 120406.
| Crossref | Google Scholar |
Paustian K, Lehmann J, Ogle S, Reay D, Robertson GP, Smith P (2016) Climate-smart soils. Nature 532, 49-57.
| Crossref | Google Scholar | PubMed |
Paustian K, Collier S, Baldock J, Burgess R, Creque J, DeLonge M, Dungait J, Ellert B, Frank S, Goddard T, Govaerts B (2019) Quantifying carbon for agricultural soil management: from the current status toward a global soil information system. Carbon Management 10, 567-587.
| Crossref | Google Scholar |
Peck G, Buck S, Hoffman A, Holloway C, Johnson B, Lawrence D, Paton C (2011) ‘Review of productivity decline in sown grass pastures B.NBP.0624.’ (Meat and Livestock Australia Limited: North Sydney, NSW) Available at https://era.daf.qld.gov.au/id/eprint/5037/1/B.NBP.0624_MLA_Final_Report% 20% 281% 29.pdf
Poeplau C, Don A, Vesterdal L, Leifeld J, Van Wesemael BAS, Schumacher J, Gensior A (2011) Temporal dynamics of soil organic carbon after land‐use change in the temperate zone–carbon response functions as a model approach. Global Change Biology 17, 2415-2427.
| Crossref | Google Scholar |
Post WM, Kwon KC (2000) Soil carbon sequestration and land‐use change: processes and potential. Global Change Biology 6, 317-327.
| Crossref | Google Scholar |
Poulton P, Johnston J, Macdonald A, White R, Powlson D (2018) Major limitations to achieving “4 per 1000” increases in soil organic carbon stock in temperate regions: evidence from long‐term experiments at Rothamsted Research, United Kingdom. Global Change Biology 24, 2563-2584.
| Crossref | Google Scholar | PubMed |
Powlson DS, Galdos MV (2023) Challenging claimed benefits of soil carbon sequestration for mitigating climate change and increasing crop yields: heresy or sober realism? Global Change Biology 29, 2381-2383.
| Crossref | Google Scholar | PubMed |
Pringle MJ, Allen DE, Dalal RC, Payne JE, Mayer DG, O’Reagain P, Marchant BP (2011) Soil carbon stock in the tropical rangelands of Australia: effects of soil type and grazing pressure, and determination of sampling requirement. Geoderma 167, 261-273.
| Crossref | Google Scholar |
Pringle MJ, Allen DE, Phelps DG, Bray SG, Orton TG, Dalal RC (2014) The effect of pasture utilization rate on stocks of soil organic carbon and total nitrogen in a semi-arid tropical grassland. Agriculture, Ecosystems and Environment 195, 83-90.
| Crossref | Google Scholar |
Pringle MJ, Allen DE, Orton TG, Bishop TFA, Butler DW, Henry BK, Dalal RC (2016) Effects of land-use change and management on soil carbon and nitrogen in the Brigalow Belt, Australia: II. Statistical models to unravel the climate-soil-management interaction. The Rangelands Journal 38, 453-466.
| Crossref | Google Scholar |
Puche N, Senapati N, Flechard CR, Klumpp K, Kirschbaum MUF, Chabbi A (2019) Modelling carbon and water fluxes of managed grasslands: comparing flux variability and net carbon budgets between grazed and mowed systems. Agronomy 9, 183.
| Crossref | Google Scholar |
Queensland Government (2023) The Land Restoration Fund Co-benefits Standard Version 1. Available at https://www.qld.gov.au/__data/assets/pdf_file/0025/116548/lrf-co-benefits-standard.pdf [accessed 28 January 2024]
Rabbi SMF, Tighe M, Delgado-Baquerizo M, Cowie A, Robertson F, Dalal R, Page K, Crawford D, Wilson BR, Schwenke G, Mcleod M, Badgery W, Dang YP, Bell M, O'Leary G, Liu DL, Baldock J (2015) Climate and soil properties limit the positive effects of land use reversion on carbon storage in Eastern Australia. Scientific Reports 5, 17866.
| Crossref | Google Scholar | PubMed |
Radrizzani A, Shelton HM, Dalzell SA, Kirchhof G (2011) Soil organic carbon and total nitrogen under Leucaena leucocephala pastures in Queensland. Crop & Pasture Science 62, 337-345.
| Crossref | Google Scholar |
Read ZJ, King H P, Tongway DJ, Ogilvy S, Greene RSB, Hand G (2016) Landscape function analysis to assess soil processes on farms following ecological restoration and changes in grazing management. European Journal of Soil Science 67, 409-420.
| Crossref | Google Scholar |
Rumpel C, Chabbi A (2021) Managing soil organic carbon for mitigating climate change and increasing food security. Agronomy 11, 1553-1558.
| Crossref | Google Scholar |
Sanderman J, Reseigh J, Wurst M, Young MA, Austin J (2015) Impacts of rotational grazing on soil carbon in native grass-based pastures in southern Australia. PLoS One 10, e0136157.
| Crossref | Google Scholar | PubMed |
Sanderman J, Hengl T, Fiske GJ (2017) Soil carbon debt of 12,000 years of human land use. Proceedings of the National Academy of Sciences of the United States of America 114, 9575-9580.
| Crossref | Google Scholar | PubMed |
Schatz T, Ffoulkes D, Shotton P, Hearnden M (2020) Effect of high-intensity rotational grazing on the growth of cattle grazing buffel pasture in the Northern Territory and on soil carbon sequestration. Animal Production Science 60, 1814-1821.
| Crossref | Google Scholar |
Schlesinger WH (2022) Biogeochemical constraints on climate change mitigation through regenerative farming. Biogeochemistry 161, 9-17.
| Crossref | Google Scholar |
Schlesinger WH, Amundson R (2019) Managing for soil carbon sequestration: let’s get realistic. Global Change Biology 25, 386-389.
| Crossref | Google Scholar | PubMed |
Schuman GE, Janzen HH, Herrick JE (2002) Soil carbon dynamics and potential carbon sequestration by rangelands. Environmental Pollution 116, 391-396.
| Crossref | Google Scholar | PubMed |
Singh K, Murphy BW, Marchant BP (2013) Towards cost-effective estimation of soil carbon stocks at the field scale. Soil Research 50, 672-684.
| Google Scholar |
Skadell LE, Schneider F, Gocke MI, Guigue J, Amelung W, Bauke SL, et al. (2023) Twenty percent of agricultural management effects on organic carbon stocks occur in subsoils–Results of ten long-term experiments. Agriculture, Ecosystems and Environment 356, 108619.
| Crossref | Google Scholar |
Skjemstad JO, Catchpoole VR, LeFeuvre RP (1994) Carbon dynamics in Vertisols under several crops as assessed by natural abundance 13C. Soil Research 32, 311-321.
| Crossref | Google Scholar |
Smith P, Soussana JF, Angers D, Schipper L, Chenu C, Rasse DP, Batjes NH, Van Egmond F, McNeill S, Kuhnert M, Arias-Navarro C, Olesen JE, Chirinda N, Fornara D, Wollenberg E, Álvaro-Fuentes J, Sanz-Cobena A, Klumpp K (2020) How to measure, report and verify soil carbon change to realize the potential of soil carbon sequestration for atmospheric greenhouse gas removal. Global Change Biology 26, 219-241.
| Crossref | Google Scholar | PubMed |
Sonter LJ, Gordon A, Archibald C, Simmonds JS, Ward M, Metzger JP, et al. (2020) Offsetting impacts of development on biodiversity and ecosystem services. Ambio 49, 892-902.
| Crossref | Google Scholar | PubMed |
Soussana J-F, Lutfalla S, Ehrhardt F, Rosenstock T, Lamanna C, Havlík P, et al. (2019) Matching policy and science: rationale for the ‘4 per 1000-soils for food security and climate’ initiative. Soil and Tillage Research 188, 3-15.
| Crossref | Google Scholar |
Stafford Smith DM, McKeon GM, Watson IW, Henry BK, Stone GS, Hall WB, Howden SM (2007) Learning from episodes of degradation and recovery in variable Australian rangelands. Proceedings of the National Academy of Sciences of the United States of America 104, 20690-20695.
| Crossref | Google Scholar | PubMed |
Stanley P, Spertus J, Chiartas J, Stark PB, Bowles T (2023) Valid inferences about soil carbon in heterogeneous landscapes. Geoderma 430, 116323.
| Crossref | Google Scholar |
Stockmann U, Adams MA, Crawford JW, Field DJ, Henakaarchchi N, et al. (2013) The knowns, known unknowns and unknowns of sequestration of soil organic carbon. Agriculture, Ecosystems and Environment 164, 80-99.
| Crossref | Google Scholar |
Thamo T, Pannell DJ (2016) Challenges in developing effective policy for soil carbon sequestration: perspectives on additionality, leakage, and permanence. Climate Policy 16, 973-992.
| Crossref | Google Scholar |
Tomkins N, Harrison M, McSweeney CS, Denman S, Charmley E, Lambrides CJ, Dalal R (2019) Greenhouse gas implications of leucaena-based pastures. Can we develop an emissions reduction methodology for the beef industry? Tropical Grasslands-Forrajes Tropicales 7, 267-272.
| Crossref | Google Scholar |
Tóth E, Deák B, Valkó O, Kelemen A, Miglécz T, Tóthmérész B, Török P (2018) Livestock type is more crucial than grazing intensity: traditional cattle and sheep grazing in short‐grass steppes. Land Degradation and Development 29, 231-239.
| Crossref | Google Scholar |
van Groenigen JW, Van Kessel C, Hungate BA, Oenema O, Powlson DS, Van Groenigen KJ (2017) Sequestering soil organic carbon: a nitrogen dilemma. Environmental Science & Technology 51, 4738-4739.
| Crossref | Google Scholar | PubMed |
Viscarra Rossel RA, Webster R, Zhang M, Shen Z, Dixon K, Wang Y-P, Walden L (2023) How much organic carbon could the soil store? The carbon sequestration potential of Australian soil. Global Change Biology 30, e17053.
| Crossref | Google Scholar | PubMed |
Waters CM, Orgill SE, Melville GJ, Toole ID, Smith WJ (2017) Management of grazing intensity in the semi‐arid rangelands of Southern Australia: effects on soil and biodiversity. Land Degradation and Development 28, 1363-1375.
| Crossref | Google Scholar |
Waters CM, McDonald SE, Reseigh J, Grant R, Burnside DG (2019) Insights on the relationship between total grazing pressure management and sustainable land management: key indicators to verify impacts. The Rangeland Journal 41, 535-556.
| Crossref | Google Scholar |
White RE (2022) The role of soil carbon sequestration as a climate change mitigation strategy: an Australian case study. Soil Systems 6, 46.
| Crossref | Google Scholar |
Wilson BR, Koen TB, Barnes P, Ghosh S, King D (2011) Soil carbon and related soil properties along a soil type and land‐use intensity gradient, New South Wales, Australia. Soil Use and Management 27, 437-447.
| Crossref | Google Scholar |
Witt GB, Noël MV, Bird MI, Beeton RB, Menzies NW (2011) Carbon sequestration and biodiversity restoration potential of semi-arid mulga lands of Australia interpreted from long-term grazing exclosures. Agriculture, Ecosystems and Environment 141, 108-118.
| Crossref | Google Scholar |
Wochesländer R, Harper RJ, Sochacki SR, Ward PR, Revell C (2016) Tagasaste (Cytisus proliferus Link.) reforestation as an option for carbon mitigation in dryland farming systems. Ecological Engineering 97, 610-618.
| Crossref | Google Scholar |
Young RR, Wilson B, Harden S, Bernardi A (2009) Accumulation of soil carbon under zero tillage cropping and perennial vegetation on the Liverpool Plains, eastern Australia. Soil Research 47, 273-285.
| Crossref | Google Scholar |
Young R, Cowie A, Harden S, McLeod R (2016) Soil carbon and inferred net primary production in high- and low-intensity grazing systems on the New England Tableland, eastern Australia. Soil Research 54, 824-839.
| Crossref | Google Scholar |
Zickfeld K, MacIsaac AJ, Canadell JG, Fuss S, Jackson RB, Jones CD, et al. (2023) Net-zero approaches must consider Earth system impacts to achieve climate goals. Nature Climate Change 13, 1298-1305.
| Crossref | Google Scholar |
Appendix
Study reference | Study region | Av. Ann. rainfall (mm) | Management change | Monitoring period (years) | Sampling depth (cm) | SOC stock change (t C ha−1 year−1) | Indicative SCS | |
---|---|---|---|---|---|---|---|---|
Grazing Management strategies | ||||||||
Grazing intensity | ||||||||
Bray et al. (2014) A | Charters Towers region, QLD | 640 | Moderate vs high | 16 | 0–30 | 0.087 | + | |
Pringle et al. (2014) A | Julia Creek region, QLD | 429 | Low vs mod/high | 26 | 0–30 | 0.004–0.035 (n.s.) | 0 | |
Clewett (2015) F | Condamine, QLD | 668 | Low vs mod/high | 18 | 0–30 | 0.03–0.13 | + | |
Clewett (2015) F | Condamine, QLD | 668 | Low vs mod/high | 10 | 0–30 | 0.5–0.9 | + | |
Young et al. (2016) | Walcha region, NSW | 900–1200 | Low vs High | >20 | 0–50 | 0.1 (n.s.) | 0 | |
Rotational vs continuous grazing | ||||||||
Allen et al. (2013) A | QLD rangelands | 256–1138 | Rotation/cell vs continuous | ~10 C | 0–30 | 0 (n.s.) C | + | |
Schatz et al. (2020) A | Northern NT | 1209 | Intensive rotation vs continuous | 5 | 0–30 | −0.03 (n.s.) | 0 | |
Sanderman et al. (2015) A | Upper, mid-north SA | 310–570 | Rotation vs continuous | 7+ D | 0–30 | −0.07 | 0 | |
Orgill et al. (2017) A | Brewarrina, NSW | 292 | Rotation vs continuous | 8+ D | 0–30 | −0.11 to 0.01 (n.s.) | 0 | |
Badgery et al. (2014) | Central West Slopes and Plains, NSW | 300–650 | Increasing intensity of rotational grazing | Various | 0–30 | NR | 0 | |
Chan et al. (2010) | Central & South NSW, North-East VIC | 600–800 | Rotational vs traditional | >10 D | 0–30 | −0.07 (n.s.) A | 0 | |
Cowie et al. (2013) | Northern Tablelands, NSW | 792 | Rotational vs continuous | >5 | 0–30 | −1.36 (n.s.) A | 0 | |
Orgill et al. (2018) | South-east NSW (Berridale region) | 582 | Rotational vs tactical (set-stock) | 4 | 0–40 | −0.85 (n.s.) | 0 | |
Orgill et al. (2014) | South-east NSW (Boorowa Region) | 610 | Rotational vs continuous | 15 D | 0–70 | −0.28 A, B | – | |
Destocking or excluding grazing | ||||||||
Allen et al. (2013) A | QLD rangelands | 256–1138 | Exclosure vs grazed | ~10 D | 0–30 | 1.68 | + | |
Carter et al. (2006) | Charleville region, QLD | 483 | Exclosure vs grazed | 24 | 0–30 | 0.28 B | ++ | |
Daryanto et al. (2013) A | Enngonia region, NSW | 312 | Exclosure vs grazed | 20 | 0–30 | 0.27–0.38 B | ++ | |
Hunt (2014) F | Kidman Springs, NT | 667 | Detocked vs grazed | 58 | 0–30 | 0.05 | + | |
Pringle et al. (2014) A | Julia Creek region, QLD | 429 | Destocked vs grazed | 26 | 0–30 | 0.006–0.041 (n.s.) | 0 | |
Sanderman et al. (2015) A | Upper, mid-north SA | 310–570 | No stock vs grazed | 7+ D | 0–30 | 0.17–0.24 (n.s.) | ++ | |
Witt et al (2011) A | South-west QLD | 150–500 | Exclosure vs grazed | 13–43 | 0–30 | ≤0.05–0.13 B | 0, ++ | |
Orgill et al. (2018) | South-east NSW (Berridale) | 582 | Ungrazed vs grazed | 4 | 0–40 | 0.98–1.83 B | ++ | |
Orgill et al. (2017) A | Cobar North, NSW | 336 | No stock/High TGP vs grazed | 8+ D | 0–30 | −0.08 to 0.21 (flat) (n.s.) −1.04 (ridges) | 0 | |
Orgill et al. (2014) | South-east NSW (Boorowa) | 610 | Ungrazed (remnant) vs grazed | 30 D | 0–70 | −0.01 to 0.14 (n.s.) A | 0 | |
Pasture management strategies | ||||||||
Sowing more productive grasses into grass pastures | ||||||||
Chan et al. (2010) E | Central-southern NSW | 600–800 | Introduced vs native pastures | ≥10 | 0–30 | 0.02 (n.s.) | 0 | |
Clewett (2015) F | Condamine, QLD | 672 | Sown vs native pastures | 50 | 0–30 | 0.11 | + | |
Chan et al. (2010) | Central-southern NSW | 600–800 | Perennial vs annual pasture | ≥10 | 0–30 | 0.4 (n.s.) | 0 | |
Chan et al. (2011) | Wagga Wagga NSW | 650 | Perennial vs annual pasture | 13 | 0–30 | 0.00 (n.s.) | 0 | |
Sowing legumes into grass pastures | ||||||||
Conrad et al. (2017) | Gayndah, QLD | 691 | Leucaena-grass vs native pastures | 40 | 0–30 | 0.28 (n.s.) | + | |
Wochesländer et al. (2016) | South-west WA | 498 | Tagasaste vs native grass | 22 | 0–30 | 0.72 | ++ | |
Clewett (2015) F | Condamine, QLD | 672 | Sown grass-legume vs native pastures | 50 | 0–30 | 0.38 | + | |
Harrison et al. (2015) | Tropical rangelands, QLD | 600–800 | Leucaena-grass vs grass pastures | ~10 | 0–30 | 0.27 ± 0.12 | + | |
Radrizzani et al. (2011) | Gayndah, QLD | 691 | Leucaena-grass vs native pastures | 20–38 | 0–15 | 0.08–0.27 | + | |
Radrizzani et al. (2011) | Banana, QLD | 667 | Leucaena-grass vs crop | 14 | 0–15 | 0.76 | ++ | |
Waterponding in scald areas | ||||||||
Read et al. (2012) | Central-west catchment, NSW | 400 | Waterponding vs scalded | 20–25 | 0–30 | 0.28 | ++ | |
Managing fire regimes in grazed savannas | ||||||||
Hunt (2014) F | Kidman Springs, NT | 667 | Early season burn (2,4 yearly) vs unmanaged fire regime | 58 | 0–30 | −0.03 | - | |
Hunt (2014) F | Kidman Springs, NT | 667 | Late season burn (4,6 yearly) vs unmanaged fire regime | 58 | 0–30 | −0.04 | - | |
Allen et al. (2021) | Kidman Springs, NT | 667 | Early or late season burn (2,4,6 yearly) vs unmanaged/unburnt fire | 20 | 0–30 | 0 (n.s.) | - | |
Land conversion strategies | ||||||||
Conversion from cropland to permanent pastures | ||||||||
Badgery et al. (2020) | Condobolin, NSW | 424 | Perennial pasture vs cropping | 15 | 0–30 | 0.48 | ++ | |
Jones et al. (2016) | South-west QLD | 583 | Cropping to grass pasture | 20 | 0–30 | 0.18 | ++ | |
Wilson et al. (2011) | North-west NSW | 690–880 | Cultivation to pasture | 15–20 | 0–30 | 0.06–0.15 | + | |
Badgery et al. (2021) | Central-west NSW | ~600 | Reduced-till cropping vs pasture | 5 | 0–30 | 0.92 | ++ | |
Skjemstad et al. (1994) | Narayan, S QLD | 716 | Cropping to perennial pasture | 11 | 0–15 | 0.21–0.44 | – | |
Young et al. (2009) | Liverpool Plains, NSW | 684 | Zero-till cropping to perennial pasture | 7 | 0–20 | 0.17 | - | |
Conversion from forest cover to grassland | ||||||||
Dalal et al. (2005) | Mulga View, SW QLD | 516 | Mulga woodland to sown pasture | 20 | 0–30 | 0.12 | + | |
Dalal et al. (2011) | Brigalow Catchment Study, QLD | 720 | Brigalow forest to sown pasture | 23 | 0–40 | 0 (n.s.) | 0 | |
Dalal et al. (2021) | Brigalow Catchment Study, QLD | 720 | Brigalow forest to sown pasture | 33 | 0–30 | −0.05 (n.s.) | 0 | |
Harms et al. (2005) | Central-Southern QLD rangelands | 600–800 | Forest/woodland to buffel grass/native pasture | <11–31 | 0–30 | −0.22 (n.s. Eucalypt; B Mulga) | 0/+ | |
Wilson et al. (2011) | North-west NSW | 690–880 | Woodland to native pasture | 20–50 | 0–30 | −1.76 | – | |
Wilson et al. (2011) | North-west NSW | 690–880 | Native woodland to sown pasture | 20–30 | 0–30 | −2.07 | – | |
Allen et al. (2016) | Brigalow Belt, QLD (45 sites) | NR | Brigalow forest to pasture | 15–73 | 0–30 | 0.72 | - | |
Grover et al. (2012) H | Douglas-Daly River Catchment, NT | 1057–1180 | Savanna woodland to pasture | 25–30 | NR | −1.1 | – | |
Livesley et al. (2021) | Douglas-Daly River Catchment, NT | 1057–1180 | Savanna woodland to improved pasture | 28 | 0–30 | 0.34 | + | |
Conversion from grassland to forest cover | ||||||||
Allen et al. (2016) | Brigalow Belt, QLD | NR | Pasture to brigalow regrowth | 16–76 | 0–30 | 0 (n.s.) | 0 | |
Paul et al. (2002) G | Australia | NR | Pasture to planted forest | 25 | 0–30 | 0.07–0.40 | + | |
Paul et al. (2002) G | Australia | NR | Pasture to plantation trees | 30 | 0–30 | 0.14 | + | |
Guo et al. (2008) | Billy Billy, ACT | 623 | Native pasture to conifer plantation | 16 | 0–100 | −1.02 | – |
NR, not reported; TGP, total grazing pressure.