Climate change impacts on northern Australian rangeland livestock carrying capacity: a review of issues
G. M. McKeon A I J , G. S. Stone A , J. I. Syktus A , J. O. Carter A , N. R. Flood A , D. G. Ahrens A , D. N. Bruget A , C. R. Chilcott B , D. H. Cobon C , R. A. Cowley D , S. J. Crimp E , G. W. Fraser A , S. M. Howden E , P. W. Johnston F , J. G. Ryan G , C. J. Stokes H and K. A. Day AA Queensland Climate Change Centre of Excellence (QCCCE), Environmental Protection Agency, Climate Building, 80 Meiers Road, Indooroopilly, Qld 4068, Australia.
B Department of Agriculture and Food Western Australia, 3 Baron-Hay Court, South Perth, WA 6151, Australia.
C Queensland Climate Change Centre of Excellence (QCCCE), Environmental Protection Agency, Climate Building, PO Box 102, Toowoomba, Qld 4350, Australia.
D NT Department of Regional Development, Primary Industry, Fisheries and Resources, PO Box 1346, Katherine, NT 0851, Australia.
E CSIRO Climate Adaptation Flagship, GPO Box 284, Canberra, ACT 2601, Australia.
F Queensland Department of Primary Industries and Fisheries, Animal Research Institute, 665 Fairfield Road, Yeerongpilly, Qld 4105, Australia.
G Centre for Remote Sensing and Spatial Information Science, The University of Queensland, St Lucia, Qld 4072, Australia.
H CSIRO Climate Adaptation Flagship, PMB PO, Aitkenvale, Qld 4814, Australia.
I Land & Water Australia Senior Research Fellowship.
J Corresponding author. Email: greg.mckeon@climatechange.qld.gov.au
The Rangeland Journal 31(1) 1-29 https://doi.org/10.1071/RJ08068
Submitted: 3 December 2008 Accepted: 6 February 2009 Published: 26 March 2009
Abstract
Grazing is a major land use in Australia’s rangelands. The ‘safe’ livestock carrying capacity (LCC) required to maintain resource condition is strongly dependent on climate. We reviewed: the approaches for quantifying LCC; current trends in climate and their effect on components of the grazing system; implications of the ‘best estimates’ of climate change projections for LCC; the agreement and disagreement between the current trends and projections; and the adequacy of current models of forage production in simulating the impact of climate change. We report the results of a sensitivity study of climate change impacts on forage production across the rangelands, and we discuss the more general issues facing grazing enterprises associated with climate change, such as ‘known uncertainties’ and adaptation responses (e.g. use of climate risk assessment).
We found that the method of quantifying LCC from a combination of estimates (simulations) of long-term (>30 years) forage production and successful grazier experience has been well tested across northern Australian rangelands with different climatic regions. This methodology provides a sound base for the assessment of climate change impacts, even though there are many identified gaps in knowledge. The evaluation of current trends indicated substantial differences in the trends of annual rainfall (and simulated forage production) across Australian rangelands with general increases in most of western Australian rangelands (including northern regions of the Northern Territory) and decreases in eastern Australian rangelands and south-western Western Australia.
Some of the projected changes in rainfall and temperature appear small compared with year-to-year variability. Nevertheless, the impacts on rangeland production systems are expected to be important in terms of required managerial and enterprise adaptations.
Some important aspects of climate systems science remain unresolved, and we suggest that a risk-averse approach to rangeland management, based on the ‘best estimate’ projections, in combination with appropriate responses to short-term (1–5 years) climate variability, would reduce the risk of resource degradation.
Climate change projections – including changes in rainfall, temperature, carbon dioxide and other climatic variables – if realised, are likely to affect forage and animal production, and ecosystem functioning. The major known uncertainties in quantifying climate change impacts are: (i) carbon dioxide effects on forage production, quality, nutrient cycling and competition between life forms (e.g. grass, shrubs and trees); and (ii) the future role of woody plants including effects of fire, climatic extremes and management for carbon storage.
In a simple example of simulating climate change impacts on forage production, we found that increased temperature (3°C) was likely to result in a decrease in forage production for most rangeland locations (e.g. –21% calculated as an unweighted average across 90 locations). The increase in temperature exacerbated or reduced the effects of a 10% decrease/increase in rainfall respectively (–33% or –9%). Estimates of the beneficial effects of increased CO2 (from 350 to 650 ppm) on forage production and water use efficiency indicated enhanced forage production (+26%). The increase was approximately equivalent to the decline in forage production associated with a 3°C temperature increase. The large magnitude of these opposing effects emphasised the importance of the uncertainties in quantifying the impacts of these components of climate change.
We anticipate decreases in LCC given that the ‘best estimate’ of climate change across the rangelands is for a decline (or little change) in rainfall and an increase in temperature. As a consequence, we suggest that public policy have regard for: the implications for livestock enterprises, regional communities, potential resource damage, animal welfare and human distress. However, the capability to quantify these warnings is yet to be developed and this important task remains as a challenge for rangeland and climate systems science.
Additional keywords: El Niño Southern Oscillation, forage production, grazing, land and pasture degradation, potential evapotranspiration, seasonal climate forecasting.
Introduction
Climate change has been identified as a major issue for the world’s rangelands (e.g. Harle et al. 2007; Henry et al. 2007; Howden et al. 2008; Wei et al. 2008). The interim Garnaut Climate Change Review (Garnaut 2008a , p. 22) highlighted the risk that Australia, with a climate that is ‘already hot, dry and variable’, faces under global warming. Grazing with domestic livestock (mainly sheep and cattle) is the major land use of Australian rangelands. The number of animals that can be sustained without irreversible damage to the soil and vegetation resource is strongly controlled by climate, particularly rainfall and its seasonal distribution (Wilson and Harrington 1984). To achieve sustainable use, state agencies have developed quantitative approaches to estimate grazing capacity using combinations of grazier experience, land-type attributes, historical climate data and simulation of forage production (e.g. Condon 1968; Johnston et al. 1996a ).
IPCC (2007, p. 8) states that warming of the global system is occurring and that further warming and other climate changes (increase in drought) are likely to occur as human-induced greenhouse emissions increase. Thus, the assessment of current climatic trends/fluctuations and future climate change projections is an important challenge for graziers, public policy and rangeland science in addressing issues of current and future rangeland use by livestock.
In this paper, we have adopted the following approach in reviewing the challenge, concentrating on northern Australia. We first review the definitions and procedures of modelling forage production developed since the early 1990s (after Scanlan et al. 1994) to define and calculate livestock carrying capacity (LCC). We then re-evaluate the climate-driven components of LCC from the viewpoint of how they are likely to be influenced by climate change. To quantify climate change impacts on forage production and LCC, we consider two questions: what has happened in the recent past (i.e. last 15–30 years) and what is likely to happen in the future? Hence, in this paper we: (i) document current changes concentrating on important components of the grazing system (soil moisture, forage production, surface cover and hydrology); (ii) briefly describe current projections; and (iii) compare current trends and future projections.
In northern Australian rangelands, simulation models of the grazing system are being used to assess likely impacts of climate change (e.g. Hall et al. 1998) including the effects of increased CO2 on forage production and water use. We review some of the known uncertainties that need to be addressed to better quantify climate change impacts on the rangeland grazing system. We present a simple example of simulating combinations of climate change (rainfall, temperature and CO2) on forage production, demonstrating the importance of quantifying the net impacts of factors operating in opposing directions (i.e. increase or decrease in forage production).
Graziers, their advisers and public policy face a difficult task in adapting to climate change. We briefly discuss a wide range of issues that are likely to influence decisions on the number of livestock grazed at an enterprise level. In particular, we discuss the importance of current developments in climate systems science and the use of climate risk assessment (and seasonal climate forecasting) in the adaptation process to achieve sustainable rangeland use.
The aims of this paper are to:
-
review the importance of LCC and calculation procedures in northern Australia;
-
evaluate current trends in climate variables and simulated components of the grazing system using the AussieGRASS model;
-
compare trends with climate change projections;
-
review the implication of climate change projections for LCC;
-
review modelling capability to simulate forage production; and
-
discuss major management issues affecting grazing enterprises resulting from climate change.
The importance of livestock carrying capacity and calculation procedures in northern Australia
Grazing is an extensive land use in the rangelands (Bastin et al. 2008). Despite high year-to-year variability in rainfall, many grazing enterprises are based on a nucleus of self-replacing herds and flocks. The concept of a safe LCC was developed (e.g. Condon 1968; Scanlan et al. 1994; Johnston et al. 1996a , 2000; Johnston and Garrad 1999) to estimate the capacity of the pasture resource to sustainably carry livestock (and other herbivores) in the long-term (>30 years).
Implicit in the meaning of the words ‘long-term’ is the responsibility of resource managers to achieve sustainable resource use over much greater time intervals (thousands of generations) than the duration of individual manager’s life. Many of the degradation processes (e.g. soil erosion, woodland thickening) which are accelerated by over-utilisation operate episodically or inexorably (e.g. Pringle et al. 2006) and, hence, managerial vigilance is required to reduce the risk of degradation. The nominated timeframe of ‘>30 years’ matches the period of responsibility of an individual manager (i.e. one generation) and the challenge of managing multi-decadal variability in rainfall. Throughout this paper, we use the term ‘long-term’ to refer to a period of time >30 years.
While some graziers maintain relatively constant property stock numbers (e.g. Stone et al. 2003), others vary stocking rates from year-to-year as part of livestock management in response to drought or above-average conditions (e.g. Scanlan et al. 1994). Based on successful experience of managing climate and resource variability, many graziers were prepared to document a ‘safe’ LCC for individual land units (and land-types), and these were used to formulate objective systems to calculate LCC (e.g. Johnston et al. 1996a , 1996b ). These calculations allow extrapolation of the successful ‘solutions’ to the problem of highly variable climates and land resources to other grazing enterprises or new managers. In the following section, we will show that this systems approach also provides a basis for calculating climate change impacts.
Johnston et al. (1996a), p. 246; after Scanlan et al. 1994) defined the ‘safe’ grazing capacity (LCC) as the number of animals [e.g. dry sheep equivalents (DSE) or beef equivalents] that ‘can be carried on a land system, paddock or property in the long-term without any decrease in pasture condition and without accelerated soil erosion.’ Johnston et al. (1996a) indicated that LCC is a strategic, i.e. long-term, estimate of stock numbers.
Mathematically, Johnston et al. (1996a) indicated that a ‘safe’ grazing capacity (i.e. LCC) can be represented as:
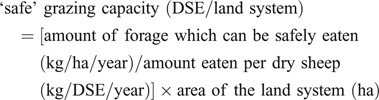
where,

Thus, the calculation of LCC involves estimates of forage production and the safe level of forage utilisation. These components of LCC will be discussed later. The above terms and formulae are not to be confused with the procedures for the calculation of short-term (<1 year) stocking rates based on appropriate utilisation of standing forage elsewhere described as the ‘responsive’ strategy to grazing management (e.g. McKeon et al. 2000).
In the rangelands, LCC is a major determinant of the gross value of production (i.e. dollars per ha from mainly cattle and sheep, McKeon et al. 2008a ), resource condition (Friedel et al. 1990; McKeon et al. 2004) and enterprise viability (Foran and Stafford Smith 1991). At the large spatial scale of Statistical Divisions (58 in Australia), the gross value of production from sheep, cattle and wool was highly correlated with livestock carried (ABS census 2001, r 2 = 0.83). Similarly, for rangeland and adjacent Statistical Divisions with relatively short growing periods (% growth index days <30, McKeon et al. 2008a ), there was also high correlation between gross value of production and LCC (r 2 = 0.80, n = 18). Many studies have indicated that spatial variation in LCC is strongly related to interactions of land-type attributes (e.g. soil properties and tree density) and climatic variation (Condon 1968; Johnston et al. 1996a ). Climate factors (and CO2) interact with land-type attributes to affect forage (including shrub) production and woody plant competition. Similarly, land-type and climate factors affect many components of the grazing system, potential forage utilisation, animal production per head (liveweight gain and wool cut), choice of breed, enterprise type, animal husbandry and supplementation requirement, calendar of operations, and the impact of grazing on resource components such as cover, soil erosion, fire frequency and pasture species composition.
The 11 Statistical Divisions that constitute the arid and semi-arid interior of Australia (72% of the area of national rural holdings), carry 28% of Australia’s sheep and cattle (expressed as beef equivalents, BE) and generate 20% of the ‘gross dollar value of production’ attributed to sheep, cattle and wool (McKeon et al. 2008a , p. 6). LCC is also important in terms of greenhouse gas emissions. Methane from sheep and cattle (excluding dairy) was 9% of Australia’s greenhouse gas emissions in 2006 (National Greenhouse Gas Inventory 2008). Tree clearing which is conducted mainly for maintaining or improving pastoral production was 11% of Australia’s greenhouse gas emissions (interim estimate for 2006, National Greenhouse Gas Inventory 2008). From 1990 to 2006, there has been a substantial reduction (54%) in emissions from the land-use change sector (National Greenhouse Gas Inventory 2008). Woodland ‘thickening’ has also been a major process in grazed woodlands (Anon. 1969; Burrows et al. 2002). Burrows et al. (2002) estimated that the change in carbon stocks in Queensland’s (Qld) grazed woodlands (over an average 14 years timeframe), as a result of woody vegetation thickening, was equivalent in magnitude to ~25% of total estimated national net emissions in 1999.
Importance of estimating safe livestock carrying capacity for resource condition
Over the last 150 years of rangeland use, graziers and public policy have had difficulty determining a LCC at a property scale. This difficulty has had important implications for the resource condition in rangelands (e.g. Tothill and Gillies 1992). McKeon et al. (2004, p. 34) listed 17 degradation episodes that have been documented in Australia’s rangelands since the 1880s. A detailed analysis was carried out for eight of these episodes. The episodes comprised a general sequence of degradation and partial recovery in which: (i) the population of livestock and other herbivores (i.e. rabbits, goats and macropods) built up during sequences of years with above-average rainfall; (ii) resource damage occurred due to the high herbivore numbers and intermittent drought; (iii) rapid decline in commodity prices for beef and wool occurred devaluing herds and flocks and increasing the difficulty of destocking; (iv) severe and extended drought led to heavy utilisation of forage and further resource damage; and (v) partial resource recovery did not occur until a sequence of above-average rainfall years, which was sometimes decades after the degradation episode (McKeon 2006, p. 17).
After the initial expansion of grazing in rangelands in the mid–late 1800s, it took many decades to develop the skill of managing for climatic variability that is required to estimate long-term LCCs. Graziers at the time (1880s and ’90s) did not have the advantage of long historical records of rainfall or experience in the variability in forage production of the rangeland resource. In fact, it is only with the benefit of over 100 years hindsight (and historical rainfall records) that we are able to define the late 1880 to the early 1890s as ‘above average’. It could be argued that rangeland producers and public policy are now (2009) in a similar state of uncertainty about future climate (particularly rainfall) as those graziers of the late 19th century. Furthermore, in some regions (e.g. western Qld) property managers may have a relatively short experience (e.g. 10–15 years) at a particular location (e.g. Reynolds and Carter 1993) and, hence, have not experienced the range of historical variability in rainfall. If the LCC of the resource is over-estimated or economic conditions prevent appropriate destocking during drought, then resource damage is likely to occur with loss of future productivity and downstream effects (e.g. McKergow et al. 2005). Thus, it is important to assess climate change projections in terms of their implications for future LCC and the risk of resource degradation.
Climate drivers of recovery from drought-degradation episodes
Drought/degradation episodes have been followed by periods of potential recovery. For the degradation episodes in eastern and central Australia that occurred in the 1890s, 1920s and 1940s, partial recovery took place during the above-average years of the 1950s and ’70s (e.g. Pickard 1993). These above-average rainfall years were associated with La Niña episodes (i.e. cool water anomalies in the eastern and central equatorial Pacific Ocean), in combination with the cool phase of the Pacific Decadal Oscillation (PDO) (Mantua et al. 1997; Folland et al. 1998; Power et al. 1999; McKeon et al. 2004). The resilience of the resource has also been attributed to many factors (e.g. soil and vegetation properties, property development, better grazing and pest management, as well as above-average rainfall, Condon 1986). Nevertheless, the future behaviour of La Niña and PDO (e.g. Cai and Whetton 2000, 2001, p. 3353) has important implications for the resilience of rangelands in eastern and central Australia (and some smaller regions of western and northern Australia). Furthermore, for these regions, Cai and Whetton (2000, p. 2579) suggested that a more ‘El Niño-like warming pattern’ was likely to develop through the 21st century. However, models vary considerably in their capability to simulate changes in ENSO amplitude and frequency (in the 21st century, CSIRO-BoM 2007, p. 106) and, hence, this emerging but currently uncertain component of climate research will have important implications for rangeland management.
Calculation of livestock carrying capacity and forage production and utilisation
During the 1960s and ’70s, objective approaches to estimating LCC were developed to account for climate and land-type factors based on: (i) grazier experiences, (ii) land resource assessments (Condon et al. 1969), and (iii) formal field experiments (termed grazing trials, e.g. Roe and Allen 1993). With the development of ecosystem simulation models, feed year planning and field experiments in the 1960–70s, it was hypothesised that spatial variation in LCC should be directly related to forage production, which determines the flow of edible dry matter in the grazing system.
The term ‘percentage utilisation’ has been commonly but loosely used since the 1970s (see review by Hunt 2008). However, for calculating LCC, it is used here to indicate the proportion of forage production that can be consumed in the long-term. Safe long-term utilisation rates were derived by comparing LCCs reported by expert graziers for different land-types and average forage production calculated from forage production models (Johnston et al. 1996a ; Hall et al. 1998).
For Qld’s native pastures, estimates of forage production accounted for a high (>80%) proportion of the variation in LCC across a wide range of land-types, properties and regions (south-west, north-east and south-east Qld, Hall et al. 1998).
Forage production has been calculated for different pasture communities from: (i) simple functions of rainfall (Foran 1984; Robertson 1987); (ii) rainfall use efficiencies (e.g. Scanlan et al. 1994; Johnston et al. 1996a ); or (iii) soil water – forage production models such as GRASP (e.g. Day et al. 1997a , 1997b ; McKeon et al. 2000; Rickert et al. 2000) which account for year-to-year climatic variability, soil attributes and tree density. Forage production can be calculated from historical rainfall as: ‘average’ (e.g. Johnston et al. 1996a ; Hall et al. 1998); ‘median’ (e.g. Cowley et al. 2008; C. Chilcott, pers. comm.); or ‘Decile 3’ (i.e. 30% percentile of forage production, Scanlan et al. 1994).
Safe utilisation rates across Qld range from 15 to 25% of average annual forage production, with vegetation communities that are located on more fertile (and/or resilient) landscapes or with a more continuous growing season, having higher values (for example, fertile Mitchell grasslands, Gidgee pastures, Johnston et al. 1996a , p. 249; and coastal Qld native pastures, Hall et al. 1998, p. 192). This approach has also been tested in the Victoria River District (VRD) of the Northern Territory (NT) by Cobiac (2007), Cowley et al. (2008) and Hamilton et al. (2008). In this region, it was important to correctly account for the area grazed based on watering points. Cowley et al. (2008) found that for 12 cattle properties in the VRD, utilisation averaged 18 and 25% when grazed areas from water points <5 and <3 km were considered, respectively. The consistency of safe utilisation rates across such a wide range of climatic environments suggests that this approach can also be used to calculate an impact of climate change through effects on forage production.
Forage production models for calculating livestock carrying capacity from climatic factors
The GRASP model (McKeon et al. 2000; Rickert et al. 2000) has also been used to simulate year-to-year variation in forage production in a wide range of rangeland and sown pasture environments (e.g. dry monsoonal in the VRD district of the NT, Dyer et al. 2001; Cobiac 2007; Cowley et al. 2008; semi-arid pastures in central Australia, western NSW, Richards et al. 2001; sown pastures in central NSW, Tupper et al. 2001; native and sown pastures in Qld, Day et al. 1997a ; McKeon et al. 1998a ). In a study on Qld native pastures, rainfall accounted for 41% of variation in forage production, while simulated growth accounted for 72% (Day et al. 1997a , p. 41).
In addition, the AussieGRASS model (Carter et al. 2000, 2003) has been developed as a spatial (5 × 5 km) implementation of GRASP including tree densities and soil attributes (available water range) with a comprehensive parameterisation of 185 pasture communities across Australia’s grazing lands. For rangeland Statistical Local Areas (SLA, i.e. local government areas), average forage production simulated by AussieGRASS was compared with reported livestock numbers (SLA-LCC). The simulation of forage production greatly improved the variation explained in SLA-LCC (r 2 = 0.64, n = 118), compared with annual rainfall (r 2 = 0.07, n = 118). The analysis indicated lower utilisation rates (i.e. ratio of LCC to forage production) in the NT, WA, SA and western Qld. The lower utilisation rates were likely to be due to a range of factors, such as less intense property development (e.g. Bastin et al. 2008), lack of availability of preferred fertile land units and dryness of the winter–spring period. Thus, the GRASP model and AussieGRASS parameters provide a well tested explanation of the climatically-induced variability in forage production across the rangelands and hence are suitable for assessing some of the relative effects of the impact of current trends and future climate change projections.
In summary, grazier estimates of LCC integrate a wide range of factors such as management of climatic variability, land-type fragility and resilience, and animal nutrition. The modelling approach we use in a later section is based on: (i) the high correlation between forage production and grazier-derived LCC; and (ii) a mechanistic understanding of the impact of heavy utilisation derived from grazing trials and supporting models. The approach has been used at the different spatial scales: individual benchmark properties (Johnston et al. 1996a ); grazier surveys (Scanlan et al. 1994); expert knowledge (Day et al. 1997b ); regional estimates (Hall et al. 1998); and census statistics (Crimp et al. 2002; McKeon et al. 2008a ).
Evaluation of current trends in climate variables
Before considering the effects of current and future climate change on forage production, we review the current climate trends and projections of climatic variables of particular importance to Australia’s rangelands. In the arid and semi-arid rangelands of inland Australia, rainfall is, by definition, a major limitation for forage production and LCC. Forage production is determined by soil moisture and fertility, temperature and solar radiation with the rate and efficiency of transpiration determined by evaporative demand (vapour pressure deficit, potential evapotranspiration). In particular, potential evapotranspiration is regarded as a key climatic variable in determining the frequency, duration and intensity of drought (e.g. EPA 2008a ; Hennessy et al. 2008).
Major changes in the climate and economic forces that affect grazing enterprises have been occurring since 1970 and, hence, trends in rainfall from this time are particularly important. Since 1970, the major human-induced climate forcings have been greenhouse gas emissions, stratospheric ozone depletion, Asian aerosols and land cover change in Australia, (Pittock 2003; Henry et al. 2007). The major economic developments have been in property infrastructure, higher livestock numbers in some regions, and increased economic pressures (declining prices and increasing costs) on enterprises.
We evaluated recent changes/trends in rainfall using alternative analytical approaches:
-
historical trends from 1970 to 2007 (Bureau of Meteorology 2008a ); and
-
change in rainfall for the period 1991–2007 (17 years) expressed as a % of the base 1961–90 (30 years).
With the exception of the gulf region of Qld, the two approaches were similar, and in the following analysis we concentrate on the latter approach. However, the statistical issue of how best to identify and quantify trends in highly variable climates with large multi-decadal variability (White et al. 2003b ) is unresolved.
The 30 year period 1961–90 has been used as a common base for many studies on climate change (e.g. CSIRO 2001) and was used here to provide a measure of change since 1990 that can be compared with rainfall projections (e.g. CSIRO-BoM 2007, p. 67, 71). For temperature, the base period 1980–99 was used for ‘IPCC global warming best estimates’ and in constructing temperature projections (CSIRO-BoM 2007, p. 51).
Trends in rainfall
Trends in rainfall have been analysed across Australia by the Bureau of Meteorology (2008a ).
Over the last 38 years (1970–2007) there have been strongly contrasting trends in rainfall across the continent with substantial declines (e.g. 50 mm/decade in north-eastern Qld) and increases (50 mm/decade) in north-western Australia. In eastern Australia, and some regions of WA, the severity of the 2001–06 drought (in terms of rainfall deficit and high temperatures) has emphasised the decline in rainfall from the wetter decades of the 1950s and ’70s (McKeon 2006; EPA 2008a , p. 132; Productivity Commission 2008, p. 65). However, in the context of the last 100 years, there is an important difference in the interpretation of these contrasting trends. In eastern Australia, the decades of the 1950s and ’70s were anomalous compared with period from 1900 to 2007. Furthermore, across eastern Australia as a whole, average rainfall (from 1978 to 2007) has been close to the long-term average (from 1900 to 2007). However, in the case of north-western Australia, the recent anomalous wetter period (1995–2001) was greater than previous historical variability (compared with the period 1900–94). Thus, care has to be taken when interpreting current trends given the impact of the starting year on analysis and historical rainfall variability.
The changes/fluctuations in rainfall have affected pasture and shrub growth (e.g. Watson et al. 2007), and, hence, LCC (e.g. McKeon et al. 2004; Bastin et al. 2008; EPA 2008a , p. 127). The above-average periods of the early 1950s and early ’70s may have biased expectation of LCC in central and eastern Australia. In eastern Australian rangelands, rainfall since 1977 has been less favourable (including severe drought periods of the early 1990s and early 2000s) and has placed potential ecological (Stone et al. 2003; EPA 2008a ) and economic hardship on grazing enterprises (e.g. wool industry in western Qld, Hall 1996).
The major feature of the spatial patterns of seasonal rainfall trends since 1970 and the percentage changes since 1990 are the strongly contrasting patterns of increasing rainfall in the western Australian rangelands and decreasing rainfall in the central and eastern Australian rangelands. Because of the strongly contrasting patterns of trends in rainfall across the continent, we use the general terms ‘western Australian rangelands’ to refer to inland WA and northern or ‘top end’ of the NT; and ‘eastern Australian rangelands’ for central and eastern Qld, western NSW and north-eastern SA.
For western Australian rangelands, rainfall has increased in summer and autumn. This increasing trend in warm season rainfall also occurred in the winter-dominant rainfall zone of the southern rangelands of WA. In contrast, winter and spring rainfall in the latter zone declined. In eastern Australian rangelands and central Australia, rainfall has declined in summer and autumn over much of these regions, particularly coastal Qld. The spatial pattern of decline was more general in autumn than in summer. Increases in winter rainfall have occurred in some regions of eastern Australia (NSW, northern and inland Qld). The directions of trends in spring rainfall have been spatially variable across Australia.
For seasons and locations, two analytical approaches (trend and percentage change patterns) were broadly consistent in indicating the direction of change. However, spring was an exception with trends since 1970 indicating extensive areas of decrease, while the percentage change patterns indicated increased rainfall since 1990 throughout much of inland Australia due to wetter conditions in the late 1990s.
Trends in temperature
In general terms, low temperatures limit plant growth in winter, while high temperatures restrict growth in summer in association with high vapour pressure deficits.
Over the whole continent, average annual temperatures warmed at 0.16°C per decade since 1950 (CSIRO-BoM 2007, p. 17). However, as with rainfall trends, there were contrasting patterns of temperature change between western and eastern Australian rangelands. From 1970 to 2007, annual minimum temperatures in central and north-eastern Australia increased by 0.35 to 0.50°C per decade, while maximum temperatures increased at slower rates (from 0.25 to 0.35°C per decade). In western Australian rangelands, the rate of temperature change was lower. For annual minimum temperatures, rates ranged from decreases (–0.125°C per decade) in north-western Australia to increases (+0.125°C per decade) in south-western Australian rangelands. Maximum temperature increases ranged from +0.075°C per decade for north-western regions to +0.25°C per decade in south-western Australian rangelands.
In terms of seasonal trends in temperature, there was considerable spatial variation in direction (increase and decrease) across seasons. The strongly contrasting directions of summer rainfall trends were also apparent in summer maximum temperature, with decreases in western rangelands and increases in eastern regions. In winter, minimum temperatures increased (0.10–0.50°C per decade) across most of eastern and southern rangelands, but decreased (–0.175 to –0.025°C per decade) in central WA, northern Australia and western NSW.
Trends in potential evapotranspiration
Potential evapotranspiration (PE) is an important (but often underrated) climate variable determining the rate and efficiency of water use in pastures and crops in arid and semi-arid environments (e.g Fischer 1979; Hammer et al. 1987). In general terms, the ratio of rainfall (or actual evapotranspiration) to pan evaporation has provided a useful index of moisture availability: (i) delineating the rangelands (Fitzpatrick and Nix 1970); and (ii) ranking current periods (e.g. 2001–06 drought) relative to historical variability (EPA 2008a , p. 132).
PE is currently measured as Class A Pan evaporation. Trends in annual pan evaporation since 1970 (Bureau of Meteorology 2008a ) indicated increases of up to 7.5 mm/decade in central Australia and central Qld. In contrast, over most of western Australian rangelands, western NSW and northern Australia, trends in pan evaporation were constant or declined (by up to 7.5 mm/decade). The regions of declining pan in the western Australian rangelands were consistent with increased rainfall (more cloud cover) and decreasing maximum temperature in summer and autumn. However, in eastern Australian rangelands, where annual rainfall declined and maximum temperatures increased, there were contrasting regions of increasing (e.g. central Qld) and decreasing pan evaporation (inland NSW).
PE is the result of a complex interaction of different climatic elements: solar radiation, vapour pressure deficit (VPD) and wind run (e.g. Roderick et al. 2007). VPD is a function of minimum and maximum temperature and humidity. Generally, as maximum temperature increases without associated major increases in dew point temperature, VPD and PE would be expected to increase and pasture/crop transpiration efficiency (i.e. growth kg/ha per mm) to decrease (Tanner and Sinclair 1983). However, decreases in wind speed in some regions of Australia have reduced PE despite increases in temperature (Rayner 2007).
McVicar et al. (2008) analysed trends in wind speed data since 1975 and found a general decrease (termed ‘stilling’, Roderick et al. 2007) across most (88%) of the continent. Although the trend was small (~0.5% per year), Rayner (2007) and Roderick et al. (2007) nevertheless found that much of the decline in pan evaporation was due to declining wind speed. Central Australia was an important exception where there was an increase. However, if the analysis was commenced in 1980, there was no change in wind speed.
The explanation of regional trends in pan evaporation in the rangelands will require further research and improved networks of instruments, especially with regard to trends in solar radiation and wind run. Without this understanding, expectations of a warmer and drier climate (e.g. Hennessy et al. 2008, p. 12) may not necessarily imply increasing evaporative demand across the rangelands.
Trends in wind speed and dust storms
The decline in wind speed over much of Australia has implications for frequency of dust storms and risk of wind-driven soil erosion in the rangelands. Time series of dust storm frequency for selected rangeland locations (data from G. McTainsh, reported by McKeon et al. 2004, p. 79) indicated reduced frequencies since the widespread drought periods of the mid 1960s and early ’80s. Declining wind speeds are likely to reduce the frequency of wind-driven soil erosion, although the risk has remained high during the extended drought period commencing in 2001 (Leys et al. 2008). Major events (e.g. 23 October 2002 in eastern Australia) have occurred during this period (McTainsh et al. 2005). For western Qld’s rangelands (EPA 2008b , p. 104), a comparison of the period from 2002 to 2006 and the previous drought period from 1992 to 1995 suggested an overall small decline. A dust storm index developed by McTainsh and Tews (2002) declined from 0.91 to 0.73 over this period.
In summary, important but spatially variable and complex trends have occurred in the climate variables that affect forage production and other rangeland ecosystem processes. However, as described below, the implications of changes in different climate variables are best assessed through monitoring vegetation response (Bastin et al. 2008; Donohue et al. 2008; EPA 2008a ) and simulation models such as AussieGRASS (Carter et al. 2003).
Trends in simulated forage production and other components of the grazing system using the AussieGRASS model
AussieGRASS includes several information layers which affect forage production and other variables (soil available water range, pasture community attributes such as temperature response, pasture fertility, tree density). The impact of climate trends (rainfall and other climatic variability) was assessed for the variables: soil moisture, forage production indices, surface cover and ‘flow-to-stream’ (i.e. hill-slope runoff and drainage) using the AussieGRASS model and parameter set (Carter et al. 2000, 2003; Fig. 1). The ‘recent’ period 1991–2007 was compared with a commonly used base period 1961–90 (Hall et al. 1998; CSIRO 2001; CSIRO-BoM 2007).
![]() |
Soil moisture and forage growth index
AussieGRASS simulates soil moisture at a daily time step and hence, integrates changes in daily rainfall distribution, intensity and pan evaporation. In eastern Australia where rainfall has declined (–20 to –5%), the decline in average available soil moisture was greater (–40 to –10%). In northern Australia, and much of WA, where rainfall has increased, the percentage increases in soil-water were of similar magnitude to percentage changes in rainfall. The forage growth index (after Fitzpatrick and Nix 1970; McKeon et al. 1980) provided a simple index integrating effects of rainfall, temperature, solar radiation, and pan evaporation with spatial variability in pasture communities, tree density and soil attributes. The growth index was mainly a climatic construct including the effects of tree water use and soil texture and depth. However, it did not include the effects of soil fertility limitation on forage production. Patterns of changes in the forage growth index were similar to rainfall changes in many regions with substantial exacerbation of relative rainfall decline in inland SA and south-west Qld. For regions with increasing rainfall (most of inland WA, the top end of the NT and north-western Qld), percentage changes in growth index were similar to percentage rainfall changes. In northern Australia, the growth index did not increase as much as rainfall, reflecting the overriding constraints of the limited duration of the wet season in this region. Further simulation studies are required to evaluate effects of individual climate variables.
Forage production
Simulated forage production included the effects of spatial variation in soil fertility. For some of the regions where reductions in rainfall have occurred (Fig. 1a ), the percentage declines in forage production were larger, (i.e. exacerbated 2-fold). For arid regions of WA where rainfall increases have occurred, there was also some amplification in terms of increases of forage production. However, in regions where soil nutrients were likely to limit forage production, percentage changes in forage production were smaller than rainfall changes.
Surface cover
The simulation of changes in surface cover was not possible in northern Australia as spatially complete remotely-sensed records of savannah burning were only available since 1997. However, in other regions where fire has not been a widespread or frequently used management tool, simulated cover has been in close agreement with observed or remotely sensed cover (EPA 2008a , p. 136). For inland areas (south-west Qld, western NSW, eastern SA and central coastal Qld), the relative reductions in pasture cover were greater than the relative decline in rainfall.
Flow-to-stream
AussieGRASS calculated ‘flow-to-stream’ as the addition of daily hill-slope runoff and through-drainage. Flow-to-stream is a function of surface cover, antecedent soil moisture, rainfall intensity (including seasonal factors) and available water range, and was validated at a drainage basin level (Carter et al. 2003, p. 156). Across the continent, the magnitude of percentage changes in flow-to-stream (–60 to +80%) were substantially greater than the changes in rainfall. In central coastal Qld, simulated flow-to-stream increased in contrast to the decrease in rainfall. Thus, a likely consequence of declining rainfall with the retention of grazing pressure is reduced cover and increased hill-slope runoff when rainfall has occurred (Scanlan et al. 1996; Fraser and Waters 2004; McKergow et al. 2005), with increased downstream risk of sediment transport to the Great Barrier Reef Lagoon (McKergow et al. 2005; Cobon et al. 2007).
In conclusion, the AussieGRASS simulations of forage production, surface cover, and flow-to-stream indicated the amplification or exacerbation of the rainfall changes that were likely to have occurred since 1990. The simulations indicated that even relatively small percentage changes in rainfall (e.g. –10 to 0%) have had greater impacts on forage production (and, hence, LCC). In particular, those rangeland regions with a 5–20% decline in rainfall (south-east Australia and coastal WA) had a much greater reduction (<–40 to –10%) in simulated forage production. The pasture resource is likely to have been more heavily utilised where grazing pressure from livestock and other herbivores (macropods and feral animals) has been maintained (e.g. EPA 2008a , p. 134) and as indicated by observations (EPA 2008a , p. 138).
Climate change projections
CSIRO-BoM (2007) have documented in detail projections in rainfall, temperature and other climatic variables that have been derived from the analysis of 23 global circulation models (GCMs) considering different greenhouse gas emissions scenarios over the 21st century as well as other human-induced and natural climate forcings (CSIRO-BoM 2007, p. 40). However, there is considerable variation between the models in terms of the natural and human-induced forcings (in addition to greenhouse gases) that were included in the simulations. The projections were derived by weighting individual GCMs based on performance relative to current patterns of precipitation, surface air temperature and sea level pressure (CSIRO-BoM 2007, p. 51). However, as discussed in greater detail below, GCMs had a wide range of projected rainfall for Australia’s rangelands (CSIRO-BoM 2007, p. 71; Hennessy et al. 2008; Preston and Jones 2008). Variation between models had a greater effect than variation between emissions scenarios even later in the 21st Century (e.g. 2050; CSIRO-BoM 2007, p. 71). The main variation in modelled rainfall results from the uncertainty in simulating small scale (<50 km scale) convective rainfall, precipitation in tropical regions, as well as large scale phenomena such as Madden Julian Oscillation (MJO), ENSO, and quasi and inter-decadal variability in ocean and atmospheric processes (e.g. Preston and Jones 2008, p. 760). Approaches using different criteria to select GCMs with better performance (e.g. Hennessy et al. 2008) or higher resolution models (Preston and Jones 2008, p. 758) are yet to demonstrate reduced uncertainty in rainfall projections.
Climate change projections for Australian rangelands
CSIRO-BoM (2007, p. 67) reported ‘best estimates’ (median) of projected changes in Australian precipitation for 2030 as a percentage of rainfall from 1961 to 1990. For medium emissions scenario A1B, the ‘best estimate’ changes are smaller for summer and autumn (varying from –7.5 to +2.5% across the continent) compared with the larger projected changes (–10 to +1%) for winter and spring. For the high emissions scenario (A1FI), the ‘best estimate’ of changes in annual rainfall for 2070 vary spatially from little change (±2%) in northern Australia, to a 20% decrease in central and WA.
The range in rainfall projections is large. For example, for the high emissions scenario in 2050, the 10th and 90th percentiles of projected changes range from –20 to +15% for much of Australia’s rangelands. This large range provides an indication of the variability in simulating rainfall in GCMs.
The ‘best estimate’ of annual warming over Australia is ≈1.0°C for 2030 (compared with 1990). Later in the 21st century, projected warming is more dependent on greenhouse gas emissions scenarios. For example, by 2070, temperature is expected to increase by 1.0 to 2.5°C for low emissions scenario and 2.2 to 5.0°C for high emissions scenario (CSIRO-BoM 2007, p. 9). By 2070, the risk of exceeding 4.0°C is greater than 30% over inland Australia under the high emissions scenario (A1FI, CSIRO-BoM 2007, p. 57).
Climate change projections suggest small changes in solar radiation, relative humidity and wind (CSIRO-BoM 2007, pp. 76–79). In the case of PE (CSIRO-BoM 2007, p. 81), for high emissions scenario A1FI in 2070, ‘best estimate’ projections are for a 8–12% increase in northern and eastern rangelands, and a 4–8% increase for the remainder of the continent. The projected increases would exacerbate any decline in rainfall, increasing moisture stress for rangeland ecosystems. For example, a 10% decline in rainfall in combination with a 10% increase in PE would result in an 18% decline in a simple moisture index based on the ratio of rainfall to PE (EPA 2008a , p. 132).
In rangelands, daily rainfall intensity has been identified as an important component of hill-slope runoff and hence, soil erosion (Scanlan et al. 1996; Fraser and Waters 2004). Rainfall intensity is expected to increase as temperature and moisture content of the atmosphere increase (CSIRO-BoM 2007, fig. 5.25). Preliminary simulations (G. Fraser, unpublished data) indicate that increased rainfall intensity could become an important component of climate change impacts and pose a major risk of increased soil erosion in rangeland landscapes.
Comparison of trends with climate change projections
There is some agreement between the direction of current trends and projected rainfall changes in eastern Australian rangelands for summer and particularly for autumn. However, in western and north-western Australian rangelands, there are substantial differences between current increasing rainfall trends and the ‘best estimate’ projections of decreases or little change in northern Australia.
For winter and spring, the projections are for relatively large declines in rainfall across eastern and western rangelands, and particularly the Gascoyne region of WA. In some regions of WA and south-east Qld, there is some agreement between the directions of ‘best estimate’ projections and current changes. However, over much of inland Qld, NSW and SA, average rainfall for spring from 1991 to 2007 has been greater than for the period from 1961 to 1990.
The analysis by CSIRO-BoM (2007, p. 71) indicates less range in estimates of rainfall change for southern latitudes of the continent compared with the tropics. However, this latitudinal gradient in the ‘reliability’ of projections contrasts with the longitudinal difference in lack of agreement between current and projected changes indicated above (with the western rangelands increasing and eastern rangelands declining in rainfall).
In addition to greenhouse gas emissions, other human-induced forcings that could be contributing to current climate change (Burroughs 2003; Pittock 2003) are stratospheric ozone depletion (Syktus 2005; Cai 2006), Asian aerosols (Cai and Cowan 2007; Rotstayn et al. 2007) and regional land cover change (McAlpine et al. 2007). Natural variability at quasi-decadal and inter-decadal time scales, and changes in solar radiation and volcanic dust can also contribute to variability in the climate system. Global climate models provide an experimental basis for evaluating contributing effects.
Of particular importance for understanding the increase in summer/autumn rainfall in western Australian rangelands is the simulation study by Rotstayn et al. (2007). They suggested that Asian anthropogenic aerosols have contributed to increased meridional temperature gradient between Asia and Australia, and as a consequence, increased rainfall in north-western Australia. However, subsequent analyses (Shi et al. 2007) have indicated some of the difficulties in simulating northern Australian rainfall with GCMs and stated that ‘the impact of aerosols on Australian rainfall remains an open question’.
The lack of agreement between current trends of increasing summer/autumn rainfall and ‘best estimate’ projections of declining rainfall in western Australian rangelands suggest that current trends in rainfall cannot be depended on to continue. Appropriate conservative management responses will be required should rainfall trends change in the direction of ‘best estimate’ projections.
General interpretation of climate trends and projections for rangelands
We have used three main approaches to interpret climate change projections:
-
qualitative assessment derived from the existing effects of spatial climatic variation and historical variability (e.g. McKeon et al. 1988);
-
a formal risk management approach involving detailed consideration of individual climate factors and their impacts on components of the grazing system (Cobon et al. 2009, this issue); and
-
simulation of impacts with biophysical models (Hall et al. 1998; Crimp et al. 2002; Carter et al. 2004; McKeon et al. 2008a ).
Estimating climate change impacts using current experience in space and time
A major challenge in communicating the importance of the projected changes in temperature and rainfall is how to relate them to the experience of rangeland managers. Although projected temperature changes of the order of 2.5−5°C (e.g. for 2070 with high emissions scenario) are small compared with daily or seasonal changes, nevertheless, they are large when the spatial range in climatological averages across the continent is considered. For example, for locations with similar annual rainfall, average temperature at Charters Towers (north-east Qld) is 23.8°C, compared with 27.2°C at Kidman Springs (VRD, NT). These spatial differences are associated with substantial differences in climatic effects on vegetation, animals, and humans (e.g. McKeon et al. 1988). Howden et al. (1999c) calculated heat stress using a temperature-humidity index across the continent. Compared with north-eastern Qld, north-western Australia had a substantially higher proportion of days with heat stress (65 compared with 35%). Field measurements with cattle in north-western Australia (Petty 1997) have indicated the importance of heat stress in limiting potential animal production when high quality pastures were available. Similarly, studies with sheep have indicated that high temperatures reduce growth (Thomas et al. 2008) and reproductive performance (Hopkins et al. 1978).
For rainfall, projected decreases of the order of 10% also appear ‘low’ when compared with year-to-year variability, or even the difference between average of El Niño and La Niña years (e.g. ~–20 to +20%, respectively, in eastern Australian rangelands, McKeon et al. 2004, p. 48). However, when the historical range of variation in 20-year moving average of rainfall is considered (Fig. 2), then these projected changes are relatively high. For example, the 20-year moving average of rainfall across Qld’s grazing lands has fluctuated between –10 and +7% since 1890. The extended periods of lower (20-year average) rainfall have been associated with extensive droughts and degradation episodes (Gibbs and Maher 1967; Day et al. 2003a , p. 144; McKeon et al. 2004). Fig. 2 also demonstrated the substantial variability (± 35%) at a quasi-decadal timescale (5-year moving average, White et al. 2003b ). The combination of long-term rainfall decline and continued quasi-decadal variability (CSIRO-BoM 2007, p. 69) is likely to episodically place severe stress on rangeland ecosystems, grazing enterprises and rural communities.
![]() |
The extent to which current trends in rainfall can be attributed to increases in greenhouse gas concentration is the subject of debate. Nevertheless, changes in landscape processes in response to anomalous rainfall/drought episodes provide examples of likely impacts. The increased summer and autumn rainfall in most rangeland regions of western and central Australia is associated with, or causing, substantial changes in landscape hydrology, vegetation and stocking rate, for example:
-
widespread increase in perennial plant vegetation cover has been measured by remote sensing (Donohue et al. 2008) and site monitoring (Watson et al. 2007);
-
buffel grass has been colonising and invading other pasture communities (Friedel et al. 2008);
-
winter and spring annual grasses which are highly favoured by stock for their nutritional value are being replaced by summer-growing perennial grasses, resulting in a reduction in forage quantity and quality. Clarke et al. (2005) showed buffel grass was directly influencing native grasses by reducing winter growth response, as well as the summer growth response of forbs;
-
stocking rates have been increasing in some regions (Bastin et al. 2008); and
-
increased gully erosion rates are also reported (Pringle and Tinley 2003, Pringle et al. 2006), although it is not clear whether this is due to more rainfall or an increase in forage utilisation.
Implications of climate change projections for livestock carrying capacity
The LCCs developed by property owners represent the result of years of experience in dealing with economic, climatic and ecological variability. Average annual forage production explained a high proportion of variation between properties and regions, and in a later section, we evaluate the climate change projections in terms of relative changes in simulated forage production. However, estimates of the likely climate change impacts on LCC also need to consider components other than forage production.
The levels of forage utilisation associated with LCC result in several beneficial factors (financial, animal and resource) by:
-
allowing enterprise ‘survival’ of multi-year droughts (e.g. Foran and Stafford Smith 1991; Stone 2004);
-
minimising the nutritional impact of minor (e.g. single year) droughts (Lauder 2008, p. 67) in maintaining perennial vegetation that responds to small (<10 mm) falls of rain (Foran 1984, p. 304);
-
reducing the need for forced sales and the need to ‘take’ low prices (White 1978; Stone 2004; Lauder 2008, p. 131);
-
minimising nutritional stress in seasonally dry conditions when high quality feed is limited (Hall et al. 1998) and, hence, less supplementation is required;
-
allowing animals to achieve higher weight gains and reproductive performance (Gillard and Moneypenny 1990; O’Reagain et al. 2008, 2009); and
-
producing better resource condition in terms of soil surface cover, ‘desired species’ composition, forage production, frequency of pasture burning, opportunities for pasture spelling, and grazing pressure on preferred land-types (e.g. Purvis 1986; Landsberg et al. 1998).
The above analysis of the components of LCC allows qualitative assessment of important climate change impacts (summarised in Table 1). The ‘best estimate’ projections are for declining rainfall (with the exception of far northern Australia) and the majority of climate change impacts listed in Table 1 are likely to be detrimental to LCC. Thus, adaptive responses to reduce animal numbers, in line with declining forage production, will be required.
![]() |
The assessment of property LCC has important implications for economic viability and in some cases, property values. The financial viability of properties has generally been assessed as the number of animals (sheep or cattle) that are required to maintain a family (i.e. a ‘living area’).
Long-term declining trends in forage production due to declining rainfall would create problems as graziers attempt to maintain livestock numbers under declining LCC. The benefits of safe utilisation described above will be, reduced and the risk of financial difficulties and resource damage increased. So, the implications of the projected climate changes, which result in lower forage production, are for lower LCC, increased living area requirement, and in some cases, lower property market values. In these circumstances, major challenges for graziers (and their advisers) will be to develop and implement better management options to compensate for the reduced LCC. Cobon et al. (2009, this issue) review the adaptations available to graziers and procedures for assessment of enterprise vulnerability. Similarly, public policy should have regard for the risk of: (i) enterprise failure with implications for regional communities (Drought Policy Review Expert Social Panel 2008; Productivity Commission 2008); (ii) potential resource damage (e.g. Stone et al. 2003; McKeon et al. 2004); and (iii) human distress involving the risk of increased mortalities (including suicides) in rural communities (Miller and Burns 2008; Sartore et al. 2008).
The long-term stability of perennial grasslands is strongly dependent on maintaining grass basal cover of desirable perennial species (Hodgkinson and Cook 1995; Ash et al. 2002). Grass basal cover is greatly reduced under conditions of high utilisation and dry growing conditions (McKeon et al. 2004, p. 70). For example, analysis of a 10-year grazing trial in black speargrass communities (south-east Qld, Scattini 1973) indicated that damage to perennial grass basal cover occurred when utilisation exceeded 30% of forage in ‘dry’ growing seasons (McKeon et al. 1990, p. 365). Similarly, grazing trials in north-eastern Qld (Ash et al. 2002, p. 21, 25) showed that composition of preferred ‘3P’ grasses (perennial, productive and palatable) could be maintained or increased (even in dry years) by adjusting stock numbers to maintain a constant level of forage utilisation (25%) independent of climatic variability.
Average low utilisation rates allows relatively constant stock numbers to be run (e.g. Stone 2004) despite high year-to-year variability in rainfall. Constant stocking rate results in heavy utilisation of perennial grasses during inevitable drought periods. Nevertheless, the proportion of years in which damage to perennial grass basal cover occurs has been less than the proportion of years in which recovery can occur (e.g. McKeon et al. 1990; Stone 2004). For example, a simulation of a native pasture at Charters Towers (north-east Qld), grazed at a constant stocking rate equivalent to 20% average annual forage utilisation, showed that the likely threshold for damage was exceeded in only 30% of years. Thus, in modelling LCC, Scanlan et al. (1994) used Decile 3 rainfall rather than average or median rainfall to calculate forage production. Stone (2004) analysed detailed historical (>70 years) livestock records for two properties in good condition in western Qld and concluded that, for both properties, there were low (~30%) proportions of years when resource damage was likely to occur.
Thus, a major challenge in adapting to climate change will be to manage for the changing frequency of damaging and recovery events. However, climate change projections have so far concentrated on changes in the ‘mean climate system’ and hence, do not yet provide information on projected changes in inter-annual and decadal variability (CSIRO-BoM 2007, p. 69; Preston and Jones 2008, p. 773).
The above review of the interaction of climate and components of LCC indicates that climate variables such as decadal variability, severity of drought, daily rainfall distribution and rainfall intensity (Table 1) are important for assessing climate change impacts. In a later section we report example simulation case studies of impacts on forage production.
Review of modelling capability to simulate forage production and known uncertainties
A risk management framework is being developed (Cobon et al. 2009, this issue) to assess the potential impact of climate change on the grazing industry at a regional scale. This will address the issue of planning for climate change including the uncertainties associated with projected rainfall. A difficulty in risk assessment is that climate factors such as increasing temperature can have both beneficial and detrimental effects on components of the grazing system (Table 2). For example, at locations where there is winter rainfall or occurrence of frosts, higher temperatures can increase the length of the growing season and improve pasture/diet quality (Wilson and Mannetje 1978). In contrast, increasing temperatures in the growing season can increase evaporative demand (depending on changes in wind speed), so reducing forage yield and decreasing forage digestibility (Wilson 1982).
![]() |
The development of simulation models
Simulation models have been developed to estimate the effects of climate variability and projected climate change on forage production and other components of the grazing system. These models represent an important distillation of rangeland science conducted since the 1930s through forage production studies (e.g. Elderslie Station in north-western Qld in 1935, Davies et al. 1938) and grazing trials (e.g. Gilruth Plains Station in south-western Qld in the 1940s, Roe and Allen 1993). A perceived strength of the models is their ability to explain much of the current spatial and temporal variation in key attributes, particularly LCC (Crimp et al. 2002). However, a weakness is that many of the climate change projections involve climate factors and CO2 concentrations outside current experience leading to uncertainties in predicting how different species of plants and animals will respond to future unprecedented climate change and CO2 concentrations. For example, potential climate extremes of moisture deficit are likely to cause the death of long-lived perennial plants (trees and perennial grasses) as demonstrated by recent episodes in northern Australian rangelands (Fensham 1998; Fensham and Holman 1999; Orr and Phelps 2006).
Nevertheless, the fact that these models explain to some extent the effects of spatial variation in climate suggests that they can be used to quantify some of the effects of climate change, particularly change in forage production.
There are many uncertainties (‘known uncertainties’, Table 3) that limit the ability to predict the impacts of a given climate change on the grazing system. Major uncertainties exist regarding the effect of CO2 on plant growth, diet quality, and tree-grass balance.
![]() |
Furthermore, the potential variation in plant species response in native grasslands provides a major challenge in predicting the impact of climate change, especially given the very limited number of measurements on the effects of increased CO2 (Stokes et al. 2005, 2008a , 2008b ) and the large number of plant species across Australia’s rangelands (e.g. Tothill and Gillies 1992).
CO2 effects
The effects of current and future increases in CO2 represent a particularly difficult problem. The general physiological effects of reduced transpiration rate, slower decline in soil moisture, increased deep drainage, and increased transpiration and radiation use efficiencies are well known (Howden et al. 1998, 1999a ; Morgan et al. 2004). Field trials using the FACE methodology (e.g. Stokes et al. 2005, 2008a ) suggest that other effects are: (i) greater relative increase in forage production in years with some moisture stress compared with years when moisture is not limiting; (ii) little benefit under extreme drought conditions; (iii) dilution of leaf nitrogen and reduced grass digestibility; (iv) greater benefit in temperate compared with tropical pastures; and (v) likely relative benefits to woody species over grasses. However, unlike other climate variables such as temperature and rainfall, there are no spatial examples that allow the effects to be quantified at large grazing trial or property scales.
Although CO2 has increased from 280 to 385 ppm since 1880 (IPCC 2007), the expected effects on dry matter production over this period are within the bounds of error in observed measurements of vegetation response, as well as year-to-year and decadal variability in rainfall (Campbell et al. 2000). Furthermore, the past effects of increased CO2 are difficult to separate from other climatic changes (temperature, pan evaporation, rainfall) and changes in grazing management (frequency of burning, increased livestock stocking rates, change in livestock species).
CO2 effects are also likely to vary between plant species and appear to be more variable under defoliation (Stokes et al. 2008a ). Field trials also suggest that the beneficial effect of CO2 in stimulating plant production may decline over time due to reduced photosynthesis (as a result of re-allocation of nitrogen by the plant away from photosynthetic enzymes) and progressive nitrogen limitation. However, such declines in CO2 effects may be an experimental artefact of the ‘shock effect’ of a sudden experimental step increase in CO2 temporarily tying up inorganic nitrogen in organic form (until the carbon and nitrogen cycles return to equilibrium). The secondary effects of reduced transpiration rate on increasing plant canopy temperatures may also need to be considered at larger regional scales (Pollard and Thompson 1995; McAlpine et al. 2007).
Change in woody plant cover
There is uncertainty in attributing causes to historically-observed changes in perennial land cover (trees and shrubs) given the number of factors involved (e.g. climate variability and trends, increasing CO2, grazing management, frequency of pasture burning and wild fires, and severity of intermittent drought). Although many of the climatic and managerial effects on rangelands have been well documented (Archer et al. 1995; Noble 1997a , 1997b ; Scholes and Archer 1997; Dyer et al. 2001), the effects of increasing CO2 on tree/grass balance are just beginning to be studied, particularly for the tropical C4 dominated grasslands of northern Australia (e.g. Stokes et al. 2008a ). If C3 woody plant species are favoured over C4 grasses (in the absence of fire) then increasing CO2 would be expected to contribute to woodland thickening and an increase in perennial land cover (Morgan et al. 2007).
However, in the case of the open woodlands of northern Australia, the perceived reduction in pasture burning has also been regarded as the major factor contributing to woodland thickening (Dyer et al. 2001; Burrows et al. 2002). The frequency of burning is dependent on the interaction of climate variability and grazing pressure. For example, increased rainfall at decadal timescales has supported increased livestock numbers over time, reducing forage yields, opportunities for pasture burning and the control of woody plants (Anon. 1969; Noble 1997a ; Burrows et al. 2002; McKeon et al. 2004). Climate changes that result in lower rainfall and lower forage yields would also be expected to reduce burning opportunities.
The major effect of climate change on the role of woody plants in rangelands may in fact result from policy decisions. For example, Garnaut (2008b , p. 557) indicated that Australia had the largest area of woodlands and forest per capita among OECD countries and a high potential for ‘carbon removal’. However, the short-term benefits of promoting broad-scale increases in woody vegetation across Australia’s rangelands need to be carefully considered in association with several trade-offs and risks.
An increase in the woody plant component in the landscape can benefit rangeland production and sustainability through greater water retention reducing downstream impacts (Ryan 2007), and positive feedback effects on regional climate. The latter may ameliorate the impact of climate change through reduced surface temperature, surface wind and evaporative demand, and potentially increased rainfall (McAlpine et al. 2007). Some studies assessing the role of tree strips (shelter belts) in southern Qld have indicated that the retention of trees (e.g. 20% of area) involved relatively less loss in forage production (5–10% reduction, e.g. McKeon et al. 2008b ). Thus, it is possible that the short-term economic, ecological and social benefits of increasing tree density strategically in the landscape may outweigh the reduction in LCC due to increased competition for forage production (Burrows et al. 1990) and reduced stream flow (Huxman et al. 2005). However, long-term impacts, including the effects of climate change on potential woody plant density and increased competition under drier conditions (Burrows et al. 1990), will need to be considered in any evaluation of alternatives in landscape design.
Known uncertainties
From the detailed model development of grazing systems conducted over the last 40 years, an example list of known uncertainties of climate change impacts has been developed (Table 3). The major unknowns relate to the effects of CO2 increase on pasture species and the likely response of trees to climate change and CO2. The investment of time and money into research of these unknowns will be determined by their perceived relative importance in aiding decision-making for graziers and policy makers. As yet, a detailed sensitivity study has not been conducted but the process described by Cobon et al. (2009, this issue) is beginning to indicate the major research needs from graziers’ perspectives.
A major issue relates to the most likely effect of increasing temperature on the severity of drought. As discussed earlier, increases in temperature are not necessarily linked to increases in PE. However, other hypotheses of how soil evaporation occurs (Rose 1968) indicate that increased rates of soil drying are likely to occur with higher temperatures. We suggest that this is an important area of research (e.g. Hennessy et al. 2008), given that increases in temperature are very likely to occur and have potentially large impacts across the rangelands. The severity of drought could well influence other major uncertainties, namely the survival of long-lived perennial tree and pasture species (Fensham 1998; Orr and Phelps 2006) and soil fauna which are important for infiltration and other aspects of ‘soil health’ (e.g. Hunt and Dawes-Gromadzki 2008).
A significant conclusion from the analysis of uncertainties is that rangeland science will have to monitor and understand the effects of climate change as they occur if the community wishes to anticipate rather than just respond to changes. As part of this process, there will be a need to attribute the observed impacts on biophysical systems (e.g. death of long-lived perennial plants) between climate variability, climate change and management. To meet this requirement, ongoing eco-physiological research will be required to understand the observed changes and make future projections. This information will support the risk management approach leading to improved adaptation responses (Cobon et al. 2009, this issue).
Simulation of climate change impacts on forage production
In the previous sections, we have highlighted the importance of forage production as a determinant of LCC and as an integrator of the consequences of changes in different climate variables (e.g. rainfall, temperature and PE). In the following case study, we evaluate general climate change impacts on forage production across the rangelands.
Pasture parameters in the GRASP model include forage production response to temperature, potential growth rates, transpiration efficiency, and minimum forage nitrogen concentration for plant growth. In the AussieGRASS implementation of GRASP (Carter et al. 2000, 2003), these parameters were derived by calibrating the model output with observed time series of remotely-sensed cover and estimates of standing dry matter using the rapid mobile data collection method developed by Hassett et al. (2000, 2006). Crimp et al. (2002) found that some pasture community parameters could be related to climate elements.
We describe below approaches to modelling the following major uncertainties associated with temperature increase: (i) the possible changes in species composition with associated plant attributes (e.g. temperature limitations, frost sensitivity, nutrient dilution); and (ii) the effects on PE.
Simulating climate effects on pasture community temperature responses
Australian rangelands cover a very wide range of climatic zones including ‘summer dominant with dry winter’, ‘winter dominant and dry summer’, ‘arid’ and ‘uniform’ regions with rainfall occurring at any time of the year (Bureau of Meteorology 2008b ). Thus, there is a very wide seasonal distribution in the timing of the main growing season between southern to northern rangeland regions. A key pasture species attribute that determines the response to rainfall is the threshold temperature required for plant growth (Fitzpatrick and Nix 1970). In parameterising the AussieGRASS model, Carter et al. (2003) used satellite measurements of green cover (NDVI time series from 1981 to 1996) to determine the threshold temperature for each pasture community. Crimp et al. (2002) found that across pasture communities, threshold temperatures for growth were highly correlated with minimum temperature of the region suggesting adaptation of existing vegetation to long-term climate attributes. We hypothesise that as rainfall and temperature regimes change, there will be a change in pasture composition towards species/ecotypes better adapted to the new climate (e.g. Howden et al. 1999b ).
Other factors such as CO2 effects on different species, climatic interactions with soil attributes (texture, depth, fertility), variable tree density, and climatic effects on nutrient availability (Moore 1970) also have a major role in determining vegetation response. In this study, we have simulated forage production without woody vegetation and with average soil attributes, in order to solely evaluate the spatial effects of climate variation on forage production.
Simulating climate change effects on PE
Following the approaches by McKeon et al. (1998a), Hall et al. (1998) and Rayner (2007), daily PE (i.e. pan evaporation, mm/day) was calculated as a function of VPD (VPD, hPa) and solar radiation (MJ/m2/day). This approach assumes that the effects of temperature increase on PE under climate change will be consistent with the existing (1980–99) average relationship between these climatic variables. However, the effects of climate change on wind speed will need to be considered in future sensitivity studies and representation of climate change projections (Rayner 2007). We have used a general equation derived from locations across Australia: pan evaporation = min (0, –1.378 + 0.1647VPD + 0.2180solar radiation). VPD was recalculated for each temperature scenario, assuming that the relative humidity associated with minimum temperature remained constant. This approach calculated similar increases in PE to those indicated by CSIRO-BoM (2007, p. 81); for example, an increase in temperature of 3°C resulted in a +5 to +10% increase in pan evaporation, depending on location.
Simulating the impact of increasing CO2
The representation of CO2 in forage production models such as GRASP involves several uncertainties:
-
the lack of field data, particularly in Australian rangelands, to develop and validate models;
-
the number of processes affected by increased CO2 (described above) and interactions with other climate variables such as temperature; and
-
the possible variation in response between ecotypes, species and life forms.
In this simulation example we used previous estimates of plant response to increased CO2 (Hall et al. 1998; Howden et al. 1999a , 1999b ; Crimp et al. 2002). For CO2 increase, pasture parameters (radiation use efficiency, potential regrowth, transpiration use efficiency, yield for 50% cover and nitrogen uptake per 100 mm of transpiration) were changed for C3 and C4 pasture communities. The main effects of doubling CO2 (700 ppm) were to increase transpiration efficiency and reduce potential transpiration for C3 and C4 pastures by 32 and 40%, respectively. However, we add the caveat that model development to include more recent experimental work relevant to the rangelands is yet to be carried out (e.g. Morgan et al. 2004; Stokes et al. 2005, 2008a ; Morgan et al. 2007).
Simulation of climate change impacts on forage production across Australian rangelands
We regard the following simulation study as a ‘sensitivity’ test, not as an evaluation of climate change projections. Furthermore, in evaluating projections it is important to recognise that climate variables such as rainfall, temperature, radiation, humidity and pan evaporation need to be linked consistently (CSIRO-BoM 2007, p. 114). However, in the following case we have concentrated on evaluating interactions of combinations of CO2, temperature and rainfall.
We evaluated the combination of a temperature increase of 3°C and increased CO2 (650 ppm) to simply represent a high emissions scenario. We also examined a range of rainfall changes including –10 or +10% as well as the larger range (–30% and +20%) as indicated by CSIRO-BoM (2007, p. 70). Our aim was not to represent the regional variation in climate change projections, but rather to evaluate the sensitivity of forage production, across the rangelands as a whole, to the same combination of climate changes in CO2, temperature and rainfall.
Several locations (192) were simulated including two locations for each Statistical Division across Australia, as well as an additional 76 locations in the 11 arid/semi-arid Statistical Divisions. The effects on forage production of single factors such as doubling CO2 (from 350 to 650 ppm), increasing temperature by 3°C and changing rainfall by –10 or +10% are shown in Fig. 3a–d . The combination of these factors, i.e. doubling CO2, temperature increase of 3°C and changes in rainfall, including the wide range indicated in projections (–30 to +20%, CSIRO-BoM 2007, p. 69) are given in Fig. 3e–h .
![]() |
Doubling CO2 (from 350 to 650 ppm) resulted in a ~25% increase in forage production across most of the arid and semi-arid zone. The response was smaller (10–20%) in higher rainfall areas, and there was little response in south-east Australia where native pasture production was simulated to be limited by soil fertility.
Increasing temperature by 3°C resulted in (i) beneficial effects in forage production response to temperature; and (ii) detrimental effects of decreasing transpiration efficiency and increasing pan evaporation. The net response was an overall decrease of 10 to >20% in forage production across much of the arid and semi-arid zones and dry monsoonal regions. In south-eastern Australia, where temperature was likely to limit growth (Fitzpatrick and Nix 1970), there was a net benefit (0–10%) of increasing temperature on forage production. Across most of the arid and semi-arid zones, temperature increase exacerbated or reduced the –10 or +10% changes in rainfall, respectively. There was less effect of temperature increase in the wetter south-eastern and northern regions where native pasture production was simulated to be limited by soil fertility (after Moore 1970; Cobiac 2007).
Increasing temperature by 3°C, doubling CO2 and rainfall increase by 10% increased forage production by 15–30% over most of Australia’s rangelands. There was less effect (0–10%) in the dry monsoonal regions of northern Australia, where soil fertility would be expected to limit forage production (Cobiac 2007).
For increasing temperature by 3°C, doubling CO2 and rainfall decrease by 10%, the decline in forage production was slightly greater than the decrease in rainfall over much of inland Australia. Thus, the effects of increased temperature and decreased rainfall generally outweighed the likely benefits of increasing CO2.
Increasing temperature by 3°C, doubling CO2 and rainfall increase by 20% substantially amplified forage production (compared with rainfall) in the very dry inland of Australia, with less amplification over much of the rangelands.
For increasing temperature by 3°C, doubling CO2 and rainfall decrease by 30%, there was a greater decrease over most of Australia’s rangelands (–50 to –30%). There was greater impact on forage production in the drier regions of central Australia (SA, western NSW and south-west Qld).
Simulations of forage production (Table 4) were averaged (unweighted) for the 90 rangeland locations where average annual forage production was less than 2500 kg/ha/year (i.e. where nutrient availability was less likely to limit production). The percentage changes in average forage production for individual components were: doubling CO2 +26%; 3°C temperature increase –21%; 10% increase in rainfall +15%; and 10% decrease in rainfall –15%. The combination of increases in CO2 and temperature resulted in no net change. The combinations of the increases in CO2 and temperature with changes in rainfall (–10 or +10%, –30 and +20%), were similar to the effects of changes in rainfall alone. Without the beneficial effects of CO2, increased temperature exacerbated the decrease in rainfall, or reduced the potential beneficial effect of increased rainfall (Table 4).
![]() |
This simulation study, as well as previous studies (Hall et al. 1998; McKeon et al. 1998a ; Crimp et al. 2002), has highlighted the importance of uncertainties in: (i) CO2 effects, particularly where nutrient availability limits forage production; (ii) changes in temperature and associated PE; and (iii) daily and seasonal rainfall distribution in calculating impacts on forage and animal production. However, at this stage of model development, it is not possible to indicate the relative importance of these uncertainties.
Major management issues affecting grazing enterprises resulting from climate change
The effects of climate change on LCC need to be considered within the broader context of issues associated with climate change. The major issues for the rangeland community that have to be addressed under climate change are: (i) mitigation to contribute to the nation’s international commitments; (ii) the assessment of climate change impacts on the grazed resource, production, biodiversity and communities; and (iii) the development of adaptation options and responses, and analysis of vulnerabilities and possible policy actions.
Mitigation
Current research projects that address mitigation are aimed at reducing emissions and quantifying potential changes in carbon stocks from grazing and fire management options. The reduction in methane emissions from animals is being addressed in terms of understanding the fundamental mechanisms of methane production in sheep and cattle, and comparing animal breeds and the impact of nutrition (e.g. MLA 2008). Current research on quantifying rangeland carbon stocks includes: the role of fire in tree/grass balance; the use of trees/shrubs in pastures; and changes in soil carbon due to grazing and fire management (e.g. Howden et al. 1994; Howden et al. 2003; Bray and Golden 2008).
Garnaut (2008b , p. 168) indicated that the ratio of permit costs to the value of production (in 2005) was highest for beef cattle and sheep, compared with other agricultural and industry sectors. The future role of grazing in rangelands as a source and possible sink for greenhouse gas emissions (and implication for LCC) is a subject of current debate. The position of agriculture with regard to the Carbon Pollution Reduction Scheme will be reviewed in 2013 (Commonwealth of Australia 2008; MLA 2008).
Monitoring the impact of climate change on forage production
A range of proven field options are available to measure the impact of climate change, such as long-term grazing trials, producer demonstrations and on-property monitoring. Of these options, grazing trials, which include measuring forage and animal production using defined experimental procedures (e.g. O’Reagain et al. 2008, 2009), are important to separate the different impacts of climate variability (e.g. year-to-year rainfall) and management (stocking rate). Simulation models developed from grazing trial data are allowing the effects of climate and management to be separated. In several cases, grazing trials have also been supported by forage production measurements across northern Australia using a similar field methodology (GUNSYNpD, McKeon et al. 1990; SWIFTSYNpD, Day et al. 1997a ). This methodology concentrates on the measurement of above-ground net primary production, pasture water use and nitrogen uptake, and hence, if conducted over time could reveal the impacts of increasing CO2 concentration and temperature on soil water balance, forage production and quality, and species composition. However, a major challenge for rangeland science is the long-term continuity of field research, so as to monitor, understand and anticipate impacts.
Although this approach concentrates on impacts on production, other approaches will be required to measure impacts on resource condition, biodiversity and population dynamics of important plant species (Orr and Phelps 2006; Watson et al. 2007). Nevertheless, the major scientific challenge of separating climate and management effects will remain.
Developing adaptation options
The historical success of agricultural science has been due to the development of a wide range of options for graziers to improve grazing management, forage production, and animal survival and production (Howden et al. 2008). Many previous solutions to technical and managerial problems have been the result of combining grazier and scientific expertise (Leslie 1993; Johnston et al. 1996a , 1996b ). Some examples involving substantial changes to the grazing industry of the rangelands over the last hundred years are: calculation of LCC, change in cattle breed from Bos taurus to Bos indicus, animal supplementation including medicated delivery through water supply, early weaning, development of pest and disease prevention, and the introduction of improved pasture species such as buffel grass and pasture legumes (McKeon et al. 1988; Hall et al. 1998). However, some of these changes have resulted in detrimental impacts on the rangeland resource and hence, they highlight the need for care in making substantial changes to grazing management and production systems.
Current projects anticipating climate change include research on animal genetics, choice of breeds (e.g. sheep meat animals), new pasture species, agroforestry, use of browse/leucaena, monitoring and control of weeds, and emerging response plans for disease and pest outbreaks.
A key component of adoption of new technologies and practices is the accessibility and transparency of new information. In this regard, extension processes such as ClimEd (George et al. 2007), Grazing Land Management Education Package (MLA 2003) and on-property demonstration trials play a necessary role.
Assessing vulnerability and response
The most likely impacts of current and future climate change across most of the rangelands are: (i) a reduction in LCC for individual grazing properties; and (ii) increased severity of drought. These changes may be beyond the capacity of adaptation for individual graziers resulting in financial and human distress (Miller and Burns 2008; Sartore et al. 2008) and long-term damage to the resource (McKeon et al. 2004). Depending on markets and costs of production, the viability of existing properties is likely to be reduced. Furthermore, some studies (e.g. Hennessy et al. 2008) suggest that droughts are likely to be more frequent and warmer (i.e. by implication more severe impacts on plants, animals and people), increasing the need for tactical responses from graziers and public policy when enterprises are pushed to breaking point. Public policy can play some part in reducing the vulnerability by supporting communities in property amalgamation (e.g. South West Strategy in Qld, Hewitt and Murray 1999, and Gascoyne Murchison Strategy in WA, WADA 2004) and providing support during extreme drought episodes (Botterill 2003; Day et al. 2005; Drought Policy Review Expert Social Panel 2008; Sartore et al. 2008). How this support should be delivered in a changing climate is a subject of current review (Productivity Commission 2008).
Thus, in addressing current and future climate change, there is an increasing emphasis on graziers being ‘expert’ at sustainably managing the resource, as well as maintaining forage and animal production. To achieve these goals, graziers will need to be supported by a sound scientific, educational and technological effort to provide the options, knowledge and skills necessary for adaptation to climate change (George et al. 2007; Howden et al. 2008; Nelson et al. 2008). With regard to the uncertainty of the direction of future rainfall, it will be important for managers, and the agencies that support them, to maintain a range of options that can be used for a range of rainfall conditions.
Managing uncertainty of future rainfall using seasonal climate risk assessment
A major limitation to forecasting the impacts of climate change on rangeland production and management is the wide range in direction of rainfall projections (e.g. –30 to +20% by 2070 for a high emissions scenario, CSIRO-BoM 2007, p. 69). Two approaches to address this uncertainty include:
-
the construction of climate change scenarios based on a shared understanding of the climate system; and
-
a ‘cut-through’ subjective year-by-year response based on a risk-averse approach to grazing management.
We discuss the basis of these emerging approaches below.
The above analysis of climate trends and projections has indicated some major issues for rangeland managers namely: in western Australian rangeland regions, the current trends (after 1990) in rainfall are not aligned with the direction of ‘best estimate’ of projections. There are several human-induced forcings as well as natural variability that could explain current trends, and the scientific understanding of the climate system is still rapidly changing (Henry et al. 2007; Table 5). For example, when the first climate change scenarios were developed in the late 1980s (e.g. Pittock 1988), the best described source of year-to-year variability in Australia’s rangelands was El Niño-Southern Oscillation (ENSO, e.g. Pittock 1975; Allan 1985). Variability at longer timescales was regarded as random fluctuations. Since that time, the understanding of the sources of climate variability and climate change has increased rapidly (Table 5).
However, the interpretation of current trends and future projections is just beginning to bridge the gap in public understanding of how these climate changes relate to the new findings on the climate processes that affect rangeland rainfall (e.g. Syktus 2005; McAlpine et al. 2007; Cai and Cowan 2008). Thus, rangeland managers and scientists are working in a complex information and managerial environment where climate change and increasing scientific understanding are rapidly occurring at the same time.
Agricultural extension agencies have developed various education programs to communicate the developing understanding of climate systems science to rangeland managers (e.g. Clewett et al. 1994, 2000, 2003; MLA 2003; Cobon et al. 2005; Meinke and Stone 2005; Henry 2006; Clewett and Clarkson 2007; George et al. 2007; Cobon et al. 2008). Various surveys have indicated that this information is used in management, (e.g. Agriculture Fisheries and Forestry Australia 2003; Keogh et al. 2004; Keogh et al. 2005; Cobon et al. 2008). For example, Marshall (2008, p. 53) surveyed 100 graziers in Dalrymple Shire (north-east Qld). Fifty-two percent of graziers agreed that they made ‘good use of the Southern Oscillation Index’ for grazing and business management. Similarly, an innovative grazier, R. Landsberg (QCL 2008) has detailed how he uses climate forecasting tools in a large number of management decisions. However, it has taken 20 years of agricultural agency extension to rangeland communities (e.g. Clewett et al. 1988, 1994, 2000; McKeon and White 1992; Stone et al. 1996b ; Meinke and Stone 2005; Ash et al. 2007; Clewett and Clarkson 2007; George et al. 2007) to communicate and achieve this level of use of seasonal climate forecasting information. Given the rapidly changing understanding of the climate system, as well as climate fluctuations and trends at longer timescales, there remains a major challenge to communicate this complexity to rangeland managers and to continue to develop ‘climate literacy’ in the rangeland community, particularly for new managers. The understanding of climate variability and climate change will be fundamental to underpin a new philosophy for management and public policy in the rangelands (e.g. Productivity Commission 2008).
One adaptation to managing uncertainty with regard to the direction of future rainfall is to link management decisions to seasonal climate risk assessment (McKeon et al. 1993; Stokes et al. 2008b ). Over the last 20 years, various statistical systems based on historical rainfall data have been developed and are operational for different rangeland regions (e.g. McBride and Nicholls 1983; Coughlan 1988; Clewett et al. 1994, 2003; Stone et al. 1996b ; Cobon et al. 2005). A simpler more compatible approach with public information is to consider the likelihood of El Niño or La Niña events occurring (Henry et al. 2004, 2007). Following the work of Allan et al. (1996) and Power and Smith (2007), we have used the SOI from June to November to classify historical years. From 1876 to 1977, there were an equal number of El Niño and La Niña events (26). However, since 1978 there have been ten El Niño events (1982, 1987, 1991, 1992, 1993, 1994, 1997, 2002, 2004, 2006) compared with five La Niña events (1988, 1996, 1998, 2000, 2008). The 2007–08 La Niña event did not develop until summer and is not included in this classification. This difference between the number of El Niño and La Niña events is anomalous in terms of the last 100 years, although the ‘values are within the bounds of what could be expected from random variation alone’ (Power and Smith 2007, p. 4). ENSO has global impacts contributing to rainfall variability in other rangeland regions across the world (e.g. Stone et al. 1996b , southern Africa, Day et al. 2003b ). Thus, the approaches in using ENSO information in the management of Australian rangelands may have wider global application.
In Australia, the SOI and other aspects of the ENSO system have been publicly available since the mid 1980s (McKeon and White 1992) and information regarding the effects of ENSO on local rainfall have been publicly extended to the rural community in Qld since 1991 (e.g. Clewett et al. 2000). This information has been supported by weekly media reports. Thus, linking management decisions involving expectations of seasonal rainfall to ENSO development would have led to a more realistic view of likely rainfall in the rangelands regions affected by ENSO (McBride and Nicholls 1983; Clewett et al. 1994; Henry 2006). The change in frequency of El Niño and La Niña, in combination with other Pacific Ocean indices, explains to some extent, the declining rainfall across Qld’s grazing lands which has occurred since the mid-1970s (Crimp and Day 2003).
One issue that needs to be resolved for the future use of ENSO information, is the stability of the relationship between ENSO phases and rainfall (McBride and Nicholls 1983; McKeon et al. 1998b ; Risbey et al. 2009). Recent research has indicated that components of the climate system operating at different timescales (ENSO and decadal) interact affecting rainfall across Australia’s rangelands (Power et al. 1999; Crimp and Day 2003; White et al. 2003b ). An operational system for summer rainfall in north-eastern Australia has been developed to include this additional understanding, using year-by-year indicators of both ENSO and the Inter-decadal Pacific Oscillation (SPOTA-1, Day et al. 2000, 2007; Crimp and Day 2003). Such developments may allow more effective ‘tracking’ of climate change.
Use of global climate models in seasonal climate forecasting
A major question with regard to the future use of seasonal forecasting is whether historical relationships between sea surface temperature/atmospheric indices and rainfall are likely to continue as global temperatures increase and human-induced forcings change climate over the next 30–50 years. One approach to address these issues has been the development of GCMs coupled with regional climate models (RCMs) to provide ‘real-time’ forecasts of rainfall using current and predicted sea surface temperatures on a seasonal to annual timescale (e.g. Goddard et al. 2003; Syktus et al. 2003). Forecasts at present concentrate on eastern Australian rangelands where ENSO is the major source of year-to-year variation, and, hence, there is a need to also provide forecasts in regions and seasons where/when ENSO is not the major source of rainfall variability or trends (Nicholls 2009; Ummenhofer et al. 2009).
In summary, we suggest that both statistical (using historical data) and GCM/RCM approaches will continue to provide year-by-year climate risk assessment which may allow adaptation of rangeland management to occur, despite the uncertainties of the future direction of rainfall. However, a major challenge is to develop a similar capability for rangeland regions including western and central Australia, and in neutral ENSO years for eastern Australian rangelands (McKeon et al. 2004).
A risk-averse approach in using climate change projections
The ‘best estimate’ projections indicate lower rainfall for most of the rangelands with little change indicated for monsoonal regions of northern Australia. Thus, the projections support a risk-averse view (i.e. expectation of reduced forage production and LCC), where rainfall has been declining in summer/autumn in eastern Australian rangelands, and in winter in southern rangelands of WA.
However, the wide range in rainfall projections (e.g. –30 to +20% for 2070 high emissions scenario), and the lack of agreement in western Australian rangelands between current wetter trends (in summer and autumn rainfall since the 1990s) and drier ‘best estimate’ projections, are unresolved scientific issues. Hence, future planning in the rangelands, especially in estimating current and future LCC, should continue to closely monitor scientific developments in these areas.
Improved scientific understanding and capacity to simulate the climate system, and the past and future impact of other human-induced climate forcings is occurring in parallel with current climate changes and fluctuations (Table 5). Thus, a major challenge for climate systems science is to provide greater understanding of recent trends (e.g. Nicholls 2009) and to update regional projections (as has occurred over the last 20 years, e.g. Pittock 1988, CSIRO-BoM 2007). Similarly, eco-physiological rangeland science faces the task of predicting and detecting the impact of increasing CO2 and temperature increases on rangeland ecosystems, in particular, forage production.
Despite these uncertainties, the ‘best estimate’ projections should be carefully considered in resource planning as they represent the well researched review of a global scientific endeavour (IPCC 2007). A risk-averse approach would be based on a generally drier (or little change in far northern Australia) expectation of future rainfall during the 21st century, depending on the region. In the case of western Australian rangelands, this approach would assume that the current wetter trends in summer and autumn will not continue indefinitely, and that in fact, they may reverse.
In general terms, planning should consider the range of climate change scenarios including the need for monitoring natural resource condition and the findings of an improving climate systems science capability. Planning should aim to maintain flexibility (e.g. resource in good condition, sufficient financial reserves, and an ability to diversify production systems), to allow the necessary responses to drier conditions should, or when, they occur. The detailed risk management procedures described by Cobon et al. (2009) provide a more formal approach in managing for the uncertainties of, and assessing the vulnerabilities resulting from, the future direction of rainfall trends.
Conclusion
We conclude that:
-
The method of quantifying LCC from a combination of estimated long-term (>30 years) forage production and successful grazier experience has been well tested across northern Australian rangelands covering a range of climatic regions. Hence, the method provides a sound base to estimate the impact of climate change. We suggest that implicit in this approach has been the successful management of year-to-year and decade-to-decade rainfall and forage production variability. However, likely changes in these climate variability components are yet to be quantified in climate change projections and the required changes in modelling LCC are yet to be addressed.
-
The evaluation of current trends (i.e. change since 1961–90) indicated substantial spatial differences in the direction and magnitude of trends in rainfall (and simulated forage production) across Australian rangelands with general increases in most of western Australian rangelands (including northern regions of the NT) and decreases in eastern Australian rangelands and south-western WA.
-
The ‘best estimate’ projections recently released by CSIRO-BoM (2007, p. 57, 70) are for hotter and drier (e.g. 2.5 to 5°C, –20 to –5% for 2070 and high emissions scenario) conditions across most of the Australian rangelands with the exception of the monsoonal regions of northern Australia where little change is projected. However, there is a wide range (e.g. –30 to +20% for 2070 with high emissions scenarios) in rainfall projections derived from the 23 global climate models (GCMs) used to develop the projections.
-
Although some of the projected changes in rainfall and temperature appear small compared with year-to-year variability, nevertheless when spatial and temporal analogues (e.g. 20-year moving averages) are considered, then the changes can be regarded as large and the consequent impacts on rangeland production systems will require important managerial and enterprise adaptations (e.g. responses to increasing frequency and intensity of drought).
-
Studies using GCMs have suggested that other human-induced forcings (stratospheric ozone depletion, Asian anthropogenic aerosols, land cover change) may have contributed to the recent differences in rainfall and climate trends across the continent. The future influence of these forcings is uncertain and should be a high priority for climate systems science in developing projections, particularly up to 2030. This is especially the case for rangeland regions where current trends are not in agreement with ‘best estimate’ projections.
-
In lieu of scientific understanding of the disagreement between trends and projections in regions of western Australian rangelands, and the large range in rainfall projections across the rangelands as a whole, we suggest that a risk-averse approach be taken. This approach involves estimating LCC based on the ‘best estimate’ projections, in combination with appropriate responses to short-term (1–5 years) climate variability, and would reduce the risk of resource degradation.
-
Climate change projections, including changes in rainfall, temperature, carbon dioxide and other climatic variables, are likely to affect forage and animal production, and ecosystem functioning. The major known uncertainties in quantifying climate change impacts are: (i) carbon dioxide effects on forage production, quality, nutrient cycling and competition between vegetation forms (e.g. grass, shrubs and trees); and (ii) the future role of woody plants including effects of fire, climatic extremes and management of carbon storage.
-
Simple spatial and temporal examples of climatic effects indicated the potential importance of projected changes such as a 3°C increase in temperature or 5–10% decline in average rainfall. In a simple example of simulating climate change impacts on forage production, we found that increased temperature (3°C) was likely to result in a decrease in forage production for most rangeland locations (–21%, unweighted average across 90 locations). Changes of – or +10% in rainfall were exacerbated/amplified to – or +15%. The change in temperature exacerbated or reduced the effects of a 10% decrease/increase in rainfall respectively (–33 or –9%, respectively). Estimates of the beneficial effects of increased CO2 on forage production and water availability indicated enhanced forage production (+26%) with CO2 increasing to 650 ppm. The increase was approximately equivalent to the decline in forage production associated with a 3°C temperature increase. The large magnitude of these opposing effects emphasises the importance of the uncertainties in quantifying the impacts of these components of climate change.
We also briefly reviewed a broader range of issues affecting the impacts of climate change on LCC such as the need for mitigation of greenhouse emissions from rangelands; the difficulty of monitoring and detecting climate change effects in variable rangeland climates; and the emerging importance of seasonal climate risk assessment as a useful management tool in some rangeland regions.
Given that the ‘best estimate’ of climate change across the rangelands is for a decline in rainfall and an increase in temperature, we anticipate decreases in LCC. As a consequence, we suggest that public policy have regard for: (i) the risk of enterprise failure with implications for regional communities; (ii) potential resource damage; and (iii) human distress including the risk of increased mortality. However, the capability to quantify these warnings is yet to be developed and this important task remains as a challenge for rangeland and climate systems science.
Acknowledgements
We gratefully acknowledge the support of A. Peacock and T. Van Bruggen in preparing the document. We also appreciated the comments of L. Pahl, A. Panjkov, P. Pinjuh, N. Treloar, N. Toombs, G. Whish, and B. Zhang. The important insights of J. Clewett, J. Scanlan, A. Pressland, A. Lauder and several anonymous referees were extremely valuable in dealing with the complexities of the paper. The senior author gratefully acknowledges the support a Land & Water Australia Senior Research Fellowship.
Archer S.,
Schimel D. S., Holland E. A.
(1995) Mechanisms of shrubland expansion: land use, climate or CO2? Climatic Change 29, 91–99.
| Crossref | GoogleScholarGoogle Scholar |
(Accessed 16 March 2009).
Condon R. W.,
Newman J. C., Cunningham G. M.
(1969) Soil erosion and pasture degeneration in Central Australia: Part III – the assessment of grazing capacity. Journal of the Soil Conservation Service of New South Wales 25, 25–50.
Daly J. J.
(1984) Cattle need shade trees. Queensland Agricultural Journal 110, 21–24.
Davies J. G.,
Scott A. E., Kennedy J. F.
(1938) The yield and composition of a Mitchell grass pasture for a period of twelve months. Journal of the Council for Scientific and Industrial Research 11, 127–139.
|
CAS |
Donald A.,
Meinke H.,
Power B.,
Maia A.,
de H. N.,
Wheeler M. C.,
White N.,
Stone R., Ribbe J.
(2006) Near-global impact of the Madden–Julian Oscillation on rainfall. Geophysical Research Letters 33, L09704.
| Crossref | GoogleScholarGoogle Scholar |
Donnelly J. R.,
Moore A. D., Freer M.
(1997) GRAZPLAN: Decision support systems for Australian grazing enterprises – I. Overview of the GRAZPLAN project, and a description of the MetAccess and LambAlive DSS. Agricultural Systems 54, 57–76.
| Crossref | GoogleScholarGoogle Scholar |
Donohue R. J.,
McVicar T. R., Roderick M. L.
(2008) Climate-related changes in Australian vegetation cover as inferred from satellite observations for 1981–2006. Global Change Biology (in press). ,
Fensham R. J.
(1998) The influence of cattle grazing on tree mortality after drought in savanna woodland in north Queensland. Australian Journal of Ecology 23, 405–407.
| Crossref | GoogleScholarGoogle Scholar |
Fensham R. J., Holman J. E.
(1999) Temporal and spatial patterns in drought related tree dieback in Australian savanna. Journal of Applied Ecology 36, 1035–1050.
| Crossref | GoogleScholarGoogle Scholar |
Fischer R. A.
(1979) Growth and water limitation to dryland wheat yield in Australia: a physiological framework. The Journal of the Australian Institute of Agricultural Science 45, 83–94.
Foran B. D., Stafford Smith D. M.
(1991) Risk, biology and drought management strategies for cattle stations in central Australia. Journal of Environmental Management 33, 17–33.
| Crossref | GoogleScholarGoogle Scholar |
Friedel M. H.,
Foran B. D., Stafford Smith D. M.
(1990) Where the creeks run dry or ten feet high: pastoral management in arid Australia. Proceedings of the Ecological Society of Australia 16, 185–194.
George D. A.,
Clewett J. F.,
Wright A.,
Birch C., Allen W.
(2007) Improving farmer knowledge and skills to better manage climate variability and climate change. Journal of International Agricultural and Extension Education 14, 5–19.
Gillard P., Moneypenny R.
(1990) A decision support model to evaluate the effects of drought and stocking rate on beef cattle properties in northern Australia. Agricultural Systems 34, 37–52.
| Crossref | GoogleScholarGoogle Scholar |
Goddard L.,
Barnston A. G., Mason S. J.
(2003) Evaluation of the IRI’S ‘net assessment seasonal climate forecasts: 1997–2001. American Meteorological Society 84, 1761–1781.
| Crossref | GoogleScholarGoogle Scholar |
Hall W. B.,
McKeon G. M.,
Carter J. O.,
Day K. A.,
Howden S. M.,
Scanlan J. C.,
Johnston P. W., Burrows W. H.
(1998) Climate Change and Queensland’s grazing lands: II. An assessment of the impact on animal production from native pastures. The Rangeland Journal 20, 177–205.
| Crossref | GoogleScholarGoogle Scholar |
Hamilton J. S.,
Chilcott C. R., Savage D. B.
(2008) Contemporary livestock carrying capacities for pastoral properties in Northern Australia: a methodology for integrating objective data on forage production and condition. Australian Journal of Experimental Agriculture 48, 735–740.
| Crossref | GoogleScholarGoogle Scholar |
Hammer G. L.,
Woodruff D. R., Robinson J. B.
(1987) Effects of climatic variability and possible climatic change on reliability of wheat cropping – a modelling approach. Agricultural and Forest Meteorology 41, 123–142.
| Crossref | GoogleScholarGoogle Scholar |
Harle K. J.,
Howden S. M.,
Hunt L. P., Dunlop M.
(2007) The potential impact of climate change on the Australian wool industry by 2030. Agricultural Systems 93, 61–89.
| Crossref | GoogleScholarGoogle Scholar |
Hassett R. C.,
Wood H. L.,
Carter J. O., Danaher T. J.
(2000) A field method for statewide ground-truthing of a spatial model. Australian Journal of Experimental Agriculture 40, 1069–1079.
| Crossref | GoogleScholarGoogle Scholar |
Hendy E. J.,
Gagan M. K., Lough J. M.
(2003) Chronological control of coral records using luminescent lines and evidence for non-stationary ENSO teleconnections in northeast Australia. The Holocene 13, 187–199.
| Crossref | GoogleScholarGoogle Scholar |
Hopkins P. S.,
Knights G. I., Le Feuvre A. S.
(1978) Studies of the environmental physiology of tropical merinos. Australian Journal of Agricultural Research 29, 161–171.
| Crossref | GoogleScholarGoogle Scholar |
Howden S. M.,
Crimp S. J., Stokes C. J.
(2008) Climate change and its effect on Australian livestock systems. Australian Journal of Experimental Agriculture 48, 780–788.
| Crossref | GoogleScholarGoogle Scholar |
Howden S. M.,
McKeon G. M.,
Walker L.,
Carter J. O.,
Conroy J. P.,
Day K. A.,
Hall W. B.,
Ash A. J., Ghannoum O.
(1999a) Global change impacts on native pastures in south-east Queensland, Australia. Environmental Modelling & Software 14, 307–316.
| Crossref | GoogleScholarGoogle Scholar |
Howden S. M.,
White D. H.,
McKeon G. M.,
Scanlan J. C., Carter J. O.
(1994) Methods for exploring management options to reduce greenhouse gas emissions from tropical pastures. Climatic Change 27, 49–70.
| Crossref | GoogleScholarGoogle Scholar |
CAS |
Hunt L. P.
(2008) Safe pasture utilisation rates as a grazing management tool in extensively grazed tropical savannas of northern Australia. The Rangeland Journal 30, 305–315.
| Crossref | GoogleScholarGoogle Scholar |
Huxman T. E.,
Bradford P. W.,
Breshears D. D.,
Scott R. L.,
Snyder K. A.,
Small E. E.,
Hultine K.,
Pockman W. T., Jackson R. B.
(2005) Ecohydrological implications of woody plant encroachment. Ecology 86, 308–319.
| Crossref | GoogleScholarGoogle Scholar |
Ivory D. A., Whiteman P. C.
(1978) Effect of temperature on growth of five subtropical grasses. Effect of day and night temperature on growth and morphological development. Australian Journal of Plant Physiology 5, 131–148.
Johnston P. W.,
McKeon G. M., Day K. A.
(1996a) Objective ‘safe’ grazing capacities for south-west Queensland Australia: development of a model for individual properties. The Rangeland Journal 18, 244–258.
| Crossref | GoogleScholarGoogle Scholar |
Johnston P. W.,
Tannock P. R., Beale I. F.
(1996b) Objective ‘safe’ grazing capacities for south–west Queensland, Australia: model application and evaluation. The Rangeland Journal 18, 259–269.
| Crossref | GoogleScholarGoogle Scholar |
Keogh D. U.,
Bell K. L.,
Park J. N., Cobon D. H.
(2004) Formative evaluation to benchmark and improve climate-based decision support for graziers in western Queensland. Australian Journal of Experimental Agriculture 44, 233–246.
| Crossref | GoogleScholarGoogle Scholar |
Keogh D. U.,
Watson I. W.,
Bell K. L.,
Cobon D. H., Dutta S. C.
(2005) Climate information needs of Gascoyne Murchison pastoralists: a representative study of the Western Australian grazing industry. Australian Journal of Experimental Agriculture 45, 1613–1625.
| Crossref | GoogleScholarGoogle Scholar |
Landsberg R. G.,
Ash A. J.,
Shepherd R. K., McKeon G. M.
(1998) Learning from history to survive the future: management evolution on Trafalgar Station, north-east Queensland. The Rangeland Journal 20, 104–118.
| Crossref | GoogleScholarGoogle Scholar |
Leslie J. K.
(1993) Systems, stakeholders and state support. Agricultural Science 6, 421–430.
Leys J.,
McTainsh G.,
Strong C.,
Heidenreich S., Biesaga K.
(2008) DustWatch: using community networks to improve wind erosion monitoring in Australia. Earth Surface Processes and Landforms 33, 1912–1926.
| Crossref | GoogleScholarGoogle Scholar |
Mantua N. J.,
Hare S. R.,
Zhang Y.,
Wallace J. M., Francis R. C.
(1997) A Pacific inter-decadal climate oscillation with impacts on salmon production. Bulletin of the American Meteorological Society 78, 1069–1079.
| Crossref | GoogleScholarGoogle Scholar |
Marshall G. J.
(2003) Trends in the Southern Annular Mode from observations and reanalyses. Journal of Climate 16, 4134–4143.
| Crossref | GoogleScholarGoogle Scholar |
McAlpine C. A.,
Syktus J.,
Deo R. C.,
Lawrence P. J.,
McGowan H. A.,
Watterson I. G., Phinn S.
(2007) Modelling the impact of historical land cover change on Australia’s regional climate. Geophysical Research Letters 34, L22711.
| Crossref | GoogleScholarGoogle Scholar |
McBride J. L., Nicholls N.
(1983) Seasonal relationships between Australian rainfall and the Southern Oscillation. Monthly Weather Review 111, 1998–2004.
| Crossref | GoogleScholarGoogle Scholar |
McKeon G. M.,
Day K. A.,
Howden S. M.,
Mott J. J.,
Orr D. M.,
Scattini W. J., Weston E. J.
(1990) Northern Australian savannas: management for pastoral production. Journal of Biogeography 17, 355–372.
| Crossref | GoogleScholarGoogle Scholar |
McKeon G. M.,
Hall W. B.,
Crimp S. J.,
Howden S. M.,
Stone R. C., Jones D. A.
(1998b) Climate change and Queensland’s grazing lands: I. Approaches and climatic trends. The Rangeland Journal 20, 151–176.
| Crossref | GoogleScholarGoogle Scholar |
McKeon G. M., White D. H.
(1992) El Nino and better land management. Search 23, 197–200.
McKergow L. A.,
Prosser I. P.,
Hughes A. O., Brodie J.
(2005) Sources of sediment to the Great Barrier Reef World Heritage Area. Marine Pollution Bulletin 51, 200–211.
| Crossref | GoogleScholarGoogle Scholar |
CAS |
PubMed |
McPhaden M. J., Zhang D.
(2002) Slowdown of the meridional overturning circulation in the upper Pacific Ocean. Nature 415, 603–608.
| Crossref | GoogleScholarGoogle Scholar |
CAS |
PubMed |
McTainsh G. H.,
Chan Y. C.,
McGowan H. A.,
Leys J. F., Tews E. K.
(2005) The 23rd October, 2002 dust storm in eastern Australia: characteristics and meteorological conditions. Atmospheric Environment 39, 1227–1236.
| Crossref | GoogleScholarGoogle Scholar |
CAS |
McVicar T. R.,
Van Niel T. G.,
Li L. T.,
Roderick M. L.,
Rayner D. P.,
Ricciardulli L., Donohue R. J.
(2008) Wind speed climatology and trends for Australia, 1975–2006: capturing the stilling phenomenon and comparison with near-surface reanalysis output. Geophysical Research Letters 35, L20403.
| Crossref | GoogleScholarGoogle Scholar |
Meinke H.,
deVoil P.,
Hammer G. L.,
Power S.,
Allan R.,
Stone R. C.,
Folland C., Potgieter A.
(2005) Rainfall variability at decadal and longer time scales: signal or noise? Journal of Climate 18, 89–96.
| Crossref | GoogleScholarGoogle Scholar |
Meinke H., Stone R. C.
(2005) Seasonal and inter-annual climate forecasting: the new tool for increasing preparedness to climate variability and change in agricultural planning and operations. Climatic Change 70, 221–253.
| Crossref | GoogleScholarGoogle Scholar |
Miller K., Burns C.
(2008) Suicides on farms in South Australia, 1997–2001. Australian Journal of Rural Health 16, 327–331.
| Crossref | GoogleScholarGoogle Scholar | PubMed |
Morgan J. A.,
Milchunas D. G.,
LeCain D. R.,
West M., Mosier A. R.
(2007) Carbon dioxide enrichment alters plant growth community structure and accelerates shrub growth in the shortgrass steppe. Proceedings of the National Academy of Sciences of USA
, 14724–14729.
| Crossref | GoogleScholarGoogle Scholar |
CAS |
Morgan J. A.,
Pataki D. E.,
Korner C.,
Clark H., Del Grosso S. J. ,
et al
.
(2004) Water relations in grassland and desert ecosystems exposed to elevated atmospheric CO2. Oecologia 140, 11–25.
| Crossref | GoogleScholarGoogle Scholar |
CAS |
PubMed |
Nelson R.,
Howden S. M., Stafford Smith M.
(2008) Using adaptive governance to rethink the way science supports Australian drought policy. Environmental Science & Policy 11, 588–601.
| Crossref | GoogleScholarGoogle Scholar |
Nicholls N.
(1988) More on early ENSOs: evidence from Australian documentary sources. Bulletin of the American Meteorological Society 69, 4–6.
| Crossref | GoogleScholarGoogle Scholar |
Nicholls N.
(1991) The El Nino/Southern Oscillation and Australian vegetation. Vegetation 91, 23–36.
| Crossref | GoogleScholarGoogle Scholar |
Nicholls N.
(2006) Detecting and attributing Australian climate change: a review. Australian Meteorological Magazine 55, 199–211.
Nicholls N.
(2009) Local and remote causes of the southern Australian autumn-winter rainfall decline, 1958–2007. Climate Dynamics ,
| Crossref | GoogleScholarGoogle Scholar |
Noble J. C., Vines R. G.
(1993) Fire studies in mallee (Eucalyptus spp.) communities of western New South Wales; grass fuel dynamics and associated weather patterns. The Rangeland Journal 15, 270–297.
| Crossref | GoogleScholarGoogle Scholar |
O’Reagain P. J.,
Bushell J. J.,
Holloway C. H., Reid A.
(2009) Managing for rainfall variability: effect of grazing strategy on cattle production in a dry tropical savanna. Animal Production Science 49, 85–99.
| Crossref | GoogleScholarGoogle Scholar |
Pickard J.
(1993) Western New South Wales – increased rainfall, not a miracle, leads to recovery. Australian Journal of Soil and Water Conservation 6, 4–9.
Pittock A. B.
(1975) Climatic change and the patterns of variation in Australia’s rainfall. Search 6, 498–504.
Pollard D., Thompson S. L.
(1995) Use of a land-surface-transfer scheme (LSX) in a global climate model: the response to doubling stomatal resistance. Global and Planetary Change 10, 129–161.
| Crossref | GoogleScholarGoogle Scholar |
Power S.,
Casey T.,
Folland C.,
Colman A., Mehta V.
(1999) Inter-decadal modulation of the impact of ENSO on Australia. Climate Dynamics 15, 319–324.
| Crossref | GoogleScholarGoogle Scholar |
Power S. B., Smith I. N.
(2007) Weakening of the Walker circulation and apparent dominance of El Niño both reach record levels, but has ENSO really changed? Geophysical Research Letters 34, L18702.
| Crossref | GoogleScholarGoogle Scholar |
Preston B. L., Jones R. N.
(2008) Evaluating sources of uncertainty in Australian runoff projections. Advances in Water Resources 31, 758–775.
| Crossref | GoogleScholarGoogle Scholar |
Pringle H. J. R., Tinley K. L.
(2003) Are we overlooking critical geomorphic determinants of landscape change in Australian rangelands? Ecological Management & Restoration 4, 180–186.
| Crossref | GoogleScholarGoogle Scholar |
Pringle H. J. R.,
Watson I. W., Tinley K. L.
(2006) Landscape improvement, or ongoing degradation: reconciling apparent contradictions from the arid rangelands of Western Australia. Landscape Ecology 21, 1267–1279.
| Crossref | GoogleScholarGoogle Scholar |
Purvis J. R.
(1986) Nurture the land: My philosophies of pastoral management in central Australia. Australian Rangeland Journal 8, 110–117.
| Crossref | GoogleScholarGoogle Scholar |
QCL
(2008) Forecasts aid planning. Profit in a changing climate. Queensland Country Life July, 10.
Rayner D. P.
(2007) Wind run changes are the dominant factor affecting pan evaporation trends in Australia. Journal of Climate 20, 3379–3394.
| Crossref | GoogleScholarGoogle Scholar |
Risbey J. S.,
Pook M. J.,
McIntosh P. C.,
Wheeler M. C., Hendon H. H.
(2009) On the remote drivers of rainfall variability in Australia. Monthly Weather Review in press. ,
Robertson G.
(1987) Effect of drought and high summer rainfall on biomass and composition of grazed pastures in western New South Wales. Australian Rangeland Journal 9, 79–85.
| Crossref | GoogleScholarGoogle Scholar |
Roderick M. L.,
Rotstayn L. D.,
Farquhar G. D., Hobbins M. T.
(2007) On the attribution of changing pan evaporation. Geophysical Research Letters 34, L17403.
| Crossref | GoogleScholarGoogle Scholar |
Roe R., Allen G. H.
(1993) Studies on the Mitchell grass association in south-western Queensland. 3. Pasture and wool production under different rates of stocking and continuous or rotational grazing. The Rangeland Journal 15, 302–319.
| Crossref | GoogleScholarGoogle Scholar |
Rose C. W.
(1968) Water transport in soil with a daily temperature wave. I. Theory and experiment. Australian Journal of Soil Research 6, 31–44.
| Crossref | GoogleScholarGoogle Scholar |
Rotstayn L. D.,
Cai W.,
Dix M. R.,
Farquhar G. D., Feng Y. ,
et al
.
(2007) Have Australian rainfall and cloudiness increased due to the remote effects of Asian anthropogenic aerosols? Journal of Geophysical Research 112, D09202.
| Crossref | GoogleScholarGoogle Scholar |
Saji N. H.,
Goswami B. N.,
Vinayachandran P. N., Yamagata T.
(1999) A dipole mode in the tropical Indian Ocean. Nature 401, 360–363.
| Crossref | GoogleScholarGoogle Scholar |
CAS |
PubMed |
Sartore G.-M.,
Kelly B.,
Stain H.,
Albrecht G., Higginbotham N.
(2008) Control, uncertainty, and expectations for the future: a qualitative study of the impact of drought on a rural Australian community. Rural and Remote Health 8, 950.
| PubMed |
Scanlan J. C.,
McKeon G. M.,
Day K. A.,
Mott J. J., Hinton A. W.
(1994) Estimating safe carrying capacities of extensive cattle grazing properties within tropical semi-arid woodlands of north-eastern Australia. The Rangeland Journal 16, 64–76.
| Crossref | GoogleScholarGoogle Scholar |
Scanlan J. C.,
Pressland A. J., Myles D. J.
(1996) Runoff and soil movement on mid-slopes in north–east Queensland grazed woodlands. The Rangeland Journal 18, 33–46.
| Crossref | GoogleScholarGoogle Scholar |
Scholes R. J., Archer S. R.
(1997) Tree–grass interactions in savannas. Annual Review of Ecology and Systematics 28, 517–544.
| Crossref | GoogleScholarGoogle Scholar |
Shi G.,
Cai W.,
Cowan T.,
Ribbe J.,
Rotstayn L., Dix M.
(2007) Variability and trend of north west Australia rainfall: observations and coupled climate modelling. American Meteorological Journal ,
| Crossref | GoogleScholarGoogle Scholar |
Stokes C. J.,
Ash A. J.,
Tibbett M., Holtum J.
(2005) OzFACE: the Australian savanna free air CO2 enrichment facility and its relevance to carbon-cycling issues in a tropical savanna. Australian Journal of Botany 53, 677–687.
| Crossref | GoogleScholarGoogle Scholar |
Stone R.,
Hammer L., Marcussen T.
(1996b) Prediction of global rainfall probabilities using phases of the Southern Oscillation Index. Nature 384, 252–255.
| Crossref | GoogleScholarGoogle Scholar |
CAS |
Stone R.,
Nicholls N., Hammer G.
(1996a) Frost in NE Australia: trends and influence of phases of the Southern Oscillation. Journal of Climate 9, 1896–1909.
| Crossref | GoogleScholarGoogle Scholar |
Syktus J. I.
(2005) Reasons for decline in eastern Australia’s rainfall. Bulletin of the American Meteorological Society 86, 624.
Thomas D. T.,
Wilmot M. G.,
Alchin M., Masters D. G.
(2008) Preliminary indications that merino sheep graze different areas on cooler days in the southern rangelands of Western Australia. Australian Journal of Experimental Agriculture 48, 889–892.
| Crossref | GoogleScholarGoogle Scholar |
Ummenhofer C. C.,
England M. H.,
McIntosh P. C.,
Meyers G. A.,
Pook M. J.,
Risbey J. S.,
Gupta S. A., Taschetto A. S.
(2009) What causes Southeast Australia’s worst droughts? Geophysical Research Letters 36,
| Crossref | GoogleScholarGoogle Scholar |
Watson I. W.,
Thomas P. W. E., Fletcher W. J.
(2007) The first assessment, using a monitoring system, of change in shrub and tree populations across the arid shrublands of Western Australia. The Rangeland Journal 29, 25–37.
| Crossref | GoogleScholarGoogle Scholar |
Webeck K., Pearson S.
(2005) Stick-nest rat middens and a late-Holocene record of White Range, central Australia. The Holocene 15, 466–471.
| Crossref | GoogleScholarGoogle Scholar |
White N.,
Sutherst R.,
Hall R., Whish-Wilson P.
(2003a) The vulnerability of the Australian beef industry to impacts of the cattle tick (Boophilus microplus) under climate change. Climatic Change 61, 157–190.
| Crossref | GoogleScholarGoogle Scholar |
White W. B.,
McKeon G. M., Syktus J. I.
(2003b) Australian drought: the interference of multi-spectral global standing modes and travelling waves. International Journal of Climatology 23, 631–662.
| Crossref | GoogleScholarGoogle Scholar |
Williams A. A. J., Stone R. C.
(2008) An assessment of relationships between the Australian subtropical ridge, rainfall variability, and high-latitude circulation patterns. International Journal of Climatology (in press). ,
| Crossref | GoogleScholarGoogle Scholar |
Wilson J. R., Mannetje L’ t.
(1978) Senescence, digestibility and carbohydrate content of buffel grass and green panic leaves in swards. Australian Journal of Agricultural Research 29, 503–516.
| Crossref | GoogleScholarGoogle Scholar |
CAS |