Integrating biobanking could produce significant cost benefits and minimise inbreeding for Australian amphibian captive breeding programs
Lachlan G. Howell


A School of Environmental and Life Sciences, University of Newcastle, Callaghan, NSW 2308, Australia.
B FAUNA Research Alliance, Kahibah, NSW 2290, Australia.
C Perth Zoo, Department of Biodiversity, Conservation and Attractions, PO Box 489, South Perth, WA 6951, Australia.
D Department of Biological Sciences, Macquarie University, Sydney, NSW 2019, Australia.
E Australian Museum, Sydney, NSW 2010, Australia.
F San Diego Zoo Institute for Conservation Research, San Pasqual Valley Road, Escondido, CA 92027, USA.
G Conservation Science Network, 24 Thomas Street, Mayfield, NSW 2304, Australia.
H Centre for Conservation Ecology and Genomics, Institute for Applied Ecology, University of Canberra, Bruce, ACT 2617, Australia.
I Corresponding author. Email: lachlan.howell@newcastle.edu.au
Reproduction, Fertility and Development 33(9) 573-587 https://doi.org/10.1071/RD21058
Submitted: 12 February 2021 Accepted: 23 March 2021 Published: 18 May 2021
Journal Compilation © CSIRO 2021 Open Access CC BY-NC
Abstract
Captive breeding is an important tool for amphibian conservation despite high economic costs and deleterious genetic effects of sustained captivity and unavoidably small colony sizes. Integration of biobanking and assisted reproductive technologies (ARTs) could provide solutions to these challenges, but is rarely used due to lack of recognition of the potential benefits and clear policy direction. Here we present compelling genetic and economic arguments to integrate biobanking and ARTs into captive breeding programs using modelled captive populations of two Australian threatened frogs, namely the orange-bellied frog Geocrinia vitellina and the white bellied frog Geocrinia alba. Back-crossing with frozen founder spermatozoa using ARTs every generation minimises rates of inbreeding and provides considerable reductions in colony size and program costs compared with conventional captive management. Biobanking could allow captive institutions to meet or exceed longstanding genetic retention targets (90% of source population heterozygosity over 100 years). We provide a broad policy direction that could make biobanking technology a practical reality across Australia’s ex situ management of amphibians in current and future holdings. Incorporating biobanking technology widely across this network could deliver outcomes by maintaining high levels of source population genetic diversity and freeing economic resources to develop ex situ programs for a greater number of threatened amphibian species.
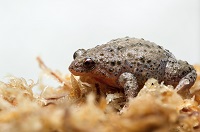
Keywords: anuran, artificial reproductive technologies, captive survival-assurance colonies, cryopreservation, frog, genome resource banking, IVF.
Introduction
Ex situ conservation tools, particularly captive breeding and assisted reproductive technologies (ARTs) are becoming increasingly important for amphibian species recovery (Bishop et al. 2012; Kouba et al. 2012; Clulow and Clulow 2016; Browne et al. 2019; Clulow et al. 2019). Australian animals face significant in situ declines across multiple taxa due to expanding and persistent anthropogenic threats, policy failings and funding neglect (Woinarski et al. 2017; Howell and Rodger 2018; Wintle et al. 2019). Threatening processes have driven some Australian species to an increasing reliance on ex situ management across the country’s network of institutions holding captive collections (Harley et al. 2018). This is especially true for amphibians, which face considerable in situ declines globally (Scheele et al. 2019) and domestically (Scheele et al. 2017) due to infectious fungal diseases (Bower et al. 2017). For example, the amphibian chytrid fungus Batrachochytrium dendrobatidis alone has resulted in the decline or extinction of more than 500 species in just a few decades (Scheele et al. 2019), with little that can be done to combat ongoing declines in the wild (although see Clulow et al. 2018a). Several Australian amphibian species are at immediate risk of extinction (Skerratt et al. 2016; Gillespie et al. 2020), some of which rely on ex situ conservation tools (particularly captive breeding) as a last line of defence (Skerratt et al. 2016; Harley et al. 2018).
Amphibians account for >27% of all native species held in historic and on-going captive breeding programs in Australia, representing 11 of the 40 species held in significant captive programs between 1980 and 2018, including six on-going amphibian programs (a modest number compared with the quantum of species facing in situ declines; Harley et al. 2018; Gillespie et al. 2020). The modest number of programs (particularly on-going programs) can be attributed to the logistical challenges and financial costs of captive breeding (Mawson and Lambert 2017; Harley et al. 2018), particularly the high average annual costs (>A$200 000) that are often required for on-going multiyear (or multidecade) programs (Harley et al. 2018). External multisectoral funding support is often required to meet these high costs (Mawson and Lambert 2017; Harley et al. 2018) and, in the absence of external funding, captive breeding is often self-funded by the institution responsible for the program (Harley et al. 2018). As a result of limited funding, it has been estimated that there are only resources to hold approximately 50 amphibian species globally in captive programs, which is in stark contrast to the at least 3000 amphibian species currently threatened with extinction (Gagliardo et al. 2008; Zippel et al. 2011; Bishop et al. 2012; Clulow et al. 2014; Murphy and Gratwicke 2017; González-del-Pliego et al. 2019).
Aside from economic challenges, captive breeding programs face significant challenges managing genetic diversity within captive collections. Captive breeding programs are criticised for the theorised or realised deleterious genetic effects associated with sustained captivity (Snyder et al. 1996), including domestication and adaptation to captivity (Frankham 2008), inbreeding depression (Ralls et al. 1988), reduced reproductive fitness and success (Farquharson et al. 2018) and loss of fitness when reintroduced or translocated (Robert 2009). There are various examples of these genetic problems occurring within Australian captive breeding programs across multiple taxa. Even some of Australia’s most well-resourced captive programs (e.g. Tasmanian devils Sarcophilus harrisii and orange-bellied parrots Neophema chrysogaster) have theorised or realised genetic issues (Farquharson et al. 2017; Grueber et al. 2017; Morrison et al. 2020). There is a clear focus within many programs on understanding and maximising genetic diversity (Hogg 2013), including for captive amphibians (Lees et al. 2013). Genetic diversity is consistently a key consideration and desired performance outcome when planning and running captive breeding programs (Harley et al. 2018).
A suggested global target for captive breeding programs is to retain 90% of source population heterozygosity (Ht/Ho) for 200 years (Soulé et al. 1986), later adjusted to 90% for 100 years (Frankham et al. 2010). However, it is unlikely this target has been met within any captive breeding program due to the large colony sizes and resources that would be required to avoid heterozygosity loss through inbreeding and genetic drift (Howell et al. 2020). Howell et al. (2020) provided a strong argument based on modelling that biobanking technology could address the challenges of high costs and genetics common to captive breeding programs and allow captive programs to meet (and surpass) the suggested genetic retention benchmark (<10% Ht/Ho loss after 100 years).
Biobanking technology (frozen living cell repositories combined with ARTs) has long been proposed as a complementary tool that may reduce space and holding requirements, as well as labour and other resources, required to run captive breeding programs (Holt et al. 1996; Clulow and Clulow 2016; Ananjeva et al. 2017; Silla and Byrne 2019). Howell et al. (2020) quantified the potential cost and genetic benefits of biobanking technology using genetic and economic modelling and demonstrated that supplementing captive-bred populations of Oregon spotted frogs Rana pretiosa with frozen founder spermatozoa through IVF every generation could result in massive short- and long-term cost reductions, minimise inbreeding and greatly reduce required live colony sizes. That modelling suggested that the reductions in inbreeding were potentially significant enough to exceed the genetic retention benchmark of 90% of source population heterozygosity within a captive amphibian population, and even allow 95% or 99% heterozygosity targets to be realistically achieved. Howell et al. (2020) proposed that amphibians are an ideal taxon to demonstrate the synergies and benefits of biobanking in captive breeding due to a combination of ideal life history traits (e.g. minimal post-fertilisation parental support, short generation length and high fecundity; Bloxam and Tonge 1995), comparatively low ex situ holding costs for individual animals (Conde et al. 2015) and recent advances in the underlying reproductive sciences in amphibians (Kouba and Vance 2009; Kouba et al. 2013; Clulow et al. 2014, 2018b, 2019; Clulow and Clulow 2016).
A consensus on the potential merits of biobanking technology and ARTs is not universal among the Australian conservation community (Skerratt et al. 2016), and uptake of the technology has been largely absent in Australia and elsewhere (Monfort 2014; Clulow et al. 2019). No examples yet exist of biobanking technology leading to measurable outcomes in an Australian amphibian captive breeding program, and there are few, if any, examples globally (Howell et al. 2020). Without a paradigm shift from practitioners, the benefits of biobanking will not be realised in Australian amphibian conservation despite clear economic and genetic fitness benefits. Nevertheless, stretched budgets for conservation, the challenges of captive breeding with standard husbandry and the persistent in situ decline of amphibians provide Australian ex situ conservation practitioners and institutions with a strong impetus to develop and adopt cost-effective biobanking and ART tools for the ex situ management of Australian amphibians.
This study provides support for integrating biobanking technology into Australian amphibian captive breeding programs by testing economic and genetic models targeted towards the network of Australian captive breeding institutions. Here, we model the end-stage cost benefits, reductions in required colony sizes and genetic diversity outcomes achieved with biobanking technology in modelled populations of orange-bellied frogs Geocrinia vitellina and white-bellied frogs Geocrinia alba using cost data from the Perth Zoo Native Species Breeding Program. As in Howell et al. (2020), we modelled the economic cost and required colony sizes to meet different heterozygosity (Ht/Ho) targets (90%, 95% and 99% of source population Ht/Ho) under scenarios of no biobanking and biobanked colonies of G. vitellina and G. alba where the captive populations are supplemented with frozen founder spermatozoa using existing or developing ARTs at every generation.
We also provide a roadmap to incorporate biobanking technology into existing and novel ex situ amphibian captive breeding programs in Australian institutions. We propose required inputs (through economic resources, strategic partnership generation, infrastructure usage and/or leverage from existing programs) across a set of actions for G. vitellina and G. alba that would also be applicable to other Australian amphibian species. We provide budgets and timelines for significant actions along the pathway, including closing species-specific knowledge gaps and biobanking protocol development for candidate amphibian species. Finally, we outline a new paradigm for the ex situ management of Australian amphibians under which biobanking technology could free economic resources to generate an ex situ network in Australia with the flexibility and resource capacity to allow the inclusion of a greater number of species in captive programs in response to the continued in situ declines of Australian amphibians.
Materials and methods
Study species
Australia’s orange-bellied frog G. vitellina and white-bellied frog G. alba are small, terrestrial breeding frogs endemic to a small area of south-west Western Australia (Clulow and Swan 2018). The two species are listed as vulnerable and critically endangered respectively under the International Union for the Conservation of Nature (IUCN) Red List (Hero and Roberts 2004; Roberts and Hero 2004), and Australia’s Environment Protection and Biodiversity Conservation (EPBC) Act 1999 Threatened Species List (https://www.environment.gov.au/cgi-bin/sprat/public/publicthreatenedlist.pl). Both species are facing combined persisting threats of disease (chytridiomycosis), land use and habitat alterations, climate change (Hoffmann et al. 2021) and invasive species (Wardell-Johnson et al. 1995; Roberts et al. 1999). Each species is reduced to small fragmented metapopulations within narrow home ranges, and both have documented in situ genetic issues (Driscoll 1997, 1998, 1999; Roberts et al. 1999; Conroy 2001). We selected G. vitellina and G. alba as case study species due to the availability of the primary data required for the modelling presented in Howell et al. (2020), G. vitellina and G. alba effective population sizes (Ne; Table 1; Driscoll 1997) and access to captive breeding costs and colony sizes from Perth Zoo’s Native Species Breeding Program (Supplementary Tables S1–S3). G. vitellina and G. alba are currently maintained as part of a successful population supplementation program in which spawn are collected from the wild, raised to metamorphosis with a high survival rate and returned to supplement (head-start) the wild source population (with only a small component of ex situ breeding). In the present study we use data for the cost of husbandry derived from these captive holdings, but model a more conventional captive breeding colony in which there is a single founder event from a source population and subsequent generations are derived from the F0 founders.
Models
We have adopted the methodologies presented in Howell et al. (2020) to model the potential cost and genetic benefits of incorporating biobanking technology into the captive breeding of G. vitellina and G. alba. We incorporate actual captive breeding program costs (2017–18 financial year breeding season of G. vitellina and G. alba within Perth Zoo’s Native Species Breeding Program) and genetic modelling (Howell et al. 2020) to model the long-term cost benefits and genetic outcomes achieved if biobanking were successfully implemented and could be incorporated with minimal additional infrastructure investment and labour costs into existing funded programs.
We modelled various 100-year captive breeding cost scenarios in which the size of live G. vitellina and G. alba captive colonies reflects the colony sizes required to maintain different proportions of the source population heterozygosity (90%, 95% and 99% Ht/Ho retention), with or without the use of biobanking technology, as proposed in other studies (Soulé et al. 1986; Frankham et al. 2010; Howell et al. 2020). For these hypothetical populations, we model biobanking strategies in which cryopreserved founder spermatozoa of G. vitellina and G. alba are used to reduce the size of live colonies while meeting genetic retention targets by the reintroduction of founder genes at each generation via IVF up to 100 years after the colony is established. We assume 4-year generational intervals for G. vitellina and G. alba (Table 2).
We adjusted the genetic back-crossing model presented in Howell et al. (2020) to account for the different life history traits, required back-cross frequency and Ne of G. vitellina and G. alba. Our model determines the census size (N) of the captive colony required to maintain various levels of genetic diversity (with heterozygosity values derived from inbreeding coefficients) under two scenarios: (1) non-back-crossed populations representing the minimum number of individuals required to meet heterozygosity targets in a conventional captive breeding program without biobanking; and (2) populations with back-crossing to founder genotypes every generation at 4-year intervals sourced from biobanked founder spermatozoa. For back-crossed populations, Ne was generated by random substitution into iterative genetic models until the 100-year Ht/Ho met desired genetic retention targets. We derived a range of colony size numbers (N) using various published and assumed Ne/N ratio estimates for G. vitellina and G. alba (Table 1). Modelled Ne/N ratios included: (1) a mean Ne/N ratio for captive vertebrate populations of 0.3 (presented in Mace 1986); (2) a median Ne/N value of 0.141 calculated using range of wild-derived temporal Ne/N estimates for amphibian species (Table 1; Frankham 1995; Frankham et al. 2019); and (3) species-specific wild-derived demographic Ne/N estimates for G. vitellina and G. alba of 1.17 and 1.21 respectively (Table 1; Driscoll 1999). The range of Ne/N values modelled here includes extremes of published values and assumptions that generate a wide range of census population sizes for captive G. vitellina and G. alba; we assume that true captive Ne/N values for G. vitellina and G. alba values would lie somewhere between the extremes modelled here. Derived N values were substituted into an economic costing model based on costs for the 2017–18 breeding season of G. vitellina and G. alba at Perth Zoo. This was used to estimate an economic ex situ holding cost per individual animal for each species and 100-year captive colony costs for each derived colony size (N) with or without biobanking technology in various modelling scenarios.
Cost modelling
All cost modelling was based on all fixed and variable program costs from Perth Zoo’s Native Species Breeding Program for the 2017–18 breeding seasons of G. vitellina and G. alba. We used a microcosting approach whereby all fixed and variable costs (expressed in nominal Australian dollars) associated with the captive breeding of G. vitellina and G. alba were calculated or estimated using face-to-face interviews with personnel as well as access to financial, logistical and operations reporting.
Year 1 economic ex situ holding cost per individual (C) is given by Eqn 1:

where all costs are in nominal 2018 Australian dollars, and where I represents facilities costs (set-up and recurring maintenance), G represents fixed founder collection costs (field collection costs including vehicles, accommodation and food for staff), E represents minor equipment and consumables costs, L represents labour costs (director and husbandry staff salaries), U represents utilities costs (electricity and water), F represents food costs, N is the captive colony size for G. vitellina and G. alba, and M represents fixed management costs (research and administration).
Year 1 program costs for non-back-crossed populations (Pc) and program costs for G. vitellina and G. alba populations incorporating biobanking technology (Pbc) are given by Eqns 2 and 3 respectively and were incorporated into iterative processes in Microsoft Excel 16.45 to model 100 years of captive management starting in 2019 (by adjusting 2017–18 values for inflation) based on Year 1 consolidated costs and assuming a 2.6% inflation rate (mean Australian inflation rate 1990–2016) going forward (https://tradingeconomics.com/australia/inflation-cpi):


where C represents Year 1 economic ex situ holding costs for either G. vitellina or G. alba (Eqn 1), B represents biobanking costs (fixed basic additional infrastructure costs and variable outsourced labour costs for recurring IVF and Year 1 cryopreservation, summarised below) and N represents the number of adult frogs in the colony. Most costs recurred annually, excluding facilities set-up costs, which occurred in Year 1 and then at 50-year intervals.
We present a low-cost biobanking scenario where biobanking-specific costs include replacement of frozen storage infrastructure of dewars and freezers at 10- and 15-year intervals respectively, based on asset deprecation rates (https://www.ato.gov.au/law/view/document?docid=TXR/TR20195/NAT/ATO/00003). Founder collection costs for G. vitellina and G. alba were modelled in Year 1 based on costs for the Perth Zoo Native Species Breeding Program annual collection of egg clutches. Costs were fixed and assumed to cover field collection costs (vehicles, accommodation and food) and did not recur after Year 1. Biobanking-specific labour costs were modelled as outsourced labour to a team of two reproductive biologists at assumed hourly rates (A$40 h−1). To estimate these costs, we generated theoretical protocols for cryopreserving G. vitellina and G. alba founder spermatozoa for long-term storage and thawing for use in IVF to provide variable estimates of full-time equivalent effort (person hours) for biobanking and genetic back-crossing. We generated theoretical protocols in line with existing amphibian reproductive technologies by adapting protocols for amphibian species of similar life history for cryopreservation and similar body mass for IVF protocols (Silla 2011, 2013; Silla and Roberts 2012). Our theoretical cryopreservation protocol is assumed to involve hormone induction of males and preparation and freezing of founder spermatozoa (an estimated 8 h to freeze and store founder spermatozoa from 15 founder males concurrently). Back-crossing (reintroduction of founder spermatozoa to captive females via IVF) sessions were assumed to involve hormone induction of females, thawing of spermatozoa and IVF (an estimated 8 h to perform IVF on 16 females, assuming staggered and concurrent IVF for four females every 2 h following a fixed 36-h time frame of preparatory tasks before the IVF procedures, such as hormone induction). Time frames and the number of animals biobanked and back-crossed in each session are based on author experience as well as relevant time frames reported in protocols used for modelling (e.g. peak spermiation and time between hormone induction and oocyte collection; Silla 2011, 2013; Silla and Roberts 2012).
Genetic modelling
We provide a summary of the genetic modelling methodology here. For detailed genetic modelling procedures, see Howell et al. (2020). We have adapted the methodologies in Howell et al. (2020) to account for different life history traits, back-cross frequency (due to generation length) and effective population size for G. vitellina or G. alba (Table 1).
The predicted rate of loss of heterozygosity was derived from the relationship between the inbreeding coefficient (Ft) and heterozygosity (H) in Eqn 4 (Crow and Kimura 1970):

This allowed determination of residual heterozygosity (Ht/Ho) at each generation for which Ft was estimated. F0 for founders was assumed to be 0.
The increase in inbreeding between generations in the captive colony without back-crossing was determined from the relationship in Eqn 5 (Frankham et al. 2010), which determines Ft (inbreeding coefficient, generation t) from Ne (effective population size) and Ft–1 (inbreeding coefficient, generation t–1):

This allows sequential determination of Ft for any generation (t) up to the number required to reach 100-year heterozygosity benchmarks (25 generations for G. vitellina or G. alba assuming a generation interval of 4 years).
We used an iterative process, as in Howell et al. (2020), using Eqn 6 to determine the effect of recurrent back-crossing (each generation) using frozen founder spermatozoa on the rate of inbreeding for the hypothetical populations modelled in each of the three back-cross scenarios:

where Ne represents the effective number of founder males and t is the number of back-cross generations. For detailed derivation of Eqn 6, see Howell et al. (2020).
This genetic modelling involves a range of general assumptions presented in Howell et al. (2020) and species-specific assumptions for captive G. vitellina and G. alba populations, presented in Table 2.
Results
Holding captive colonies of G. vitellina and G. alba designed to maintain long-term genetic viability under increasingly rigorous genetic retention benchmarks (90%, 95% and 99% of source population Ht/Ho) would require increasing live animal numbers and therefore higher set-up and total program costs (Table 3). Populations with biobanked founder spermatozoa can meet these targets with greatly reduced colony sizes, and therefore reduced program costs (Table 3), compared with non-biobanked populations in captive colonies of G. vitellina and G. alba (Figs 1–3). Costs of current captive populations held into the future are also plotted in Figs 2 and 3 and are provided for comparative purposes only, but do not relate to the genetic or economic captive breeding models. There is no equivalence between the modelled scenarios and the current holdings that are not maintained as breeding colonies.
![]() |
Captive breeding populations of G. vitellina (Ne = 120; n = 400 live individuals in the captive colony) and G. alba (Ne = 120; n = 400) designed to retain 90% of source population heterozygosity (<10% loss in Ht/Ho after 100 years) in a conventional captive colony without the use of biobanking technology would require significant Year 1 start-up costs of more than A$1.1 million for G. vitellina and more than A$718 000 for G. alba, followed by total 100-year program costs of more than A$466 million and more than A$284 million respectively (mean annual expenditure of more than A$4.6 million and more than A$2.8 million; Table 3). These hypothetical populations would meet 90% retention targets with residual Ht/Ho of 0.9009 after the captive period (Table 3; Fig. 1). The more ambitious target of 95% retention in hypothetical G. vitellina (Ne = 244; n = 813) and G. alba (Ne = 244; n = 813) breeding populations would require increased investment of more than A$945 million and more than A$576 million respectively across 100 years to maintain residual Ht/Ho of 0.9500 (Table 3; Fig. 1). The most ambitious genetic retention target presented here of 99% retention in G. vitellina (Ne = 1245; n = 4150) and G. alba (Ne = 1245; n = 4150) populations would require significantly increased investment of more than A$4.8 billion and more than A$2.9 billion across 100 years respectively to maintain residual Ht/Ho of 0.9900 (Table 3; Fig. 1).
Captive breeding populations of G. vitellina and G. alba, designed to meet the above heterozygosity retention targets under the conditions of our modelled populations, could reduce the required number of live individuals in each generation, and therefore program costs, by using biobanked G. vitellina and G. alba frozen founder spermatozoa to back-cross females in every generation to founder males.
Back-crossing every generation at 4-year intervals into G. vitellina and G. alba colonies designed to retain 90% source population heterozygosity (n = 17 founder males; same number of live females per generation) would each require A$948 000 in biobanking costs but would allow reductions of >380 individuals to live G. vitellina and G. alba census colonies of only 17 female individuals per generation. This would reduce G. vitellina and G. alba Year 1 program costs to A$68 000 and A$48 000 respectively and reduce total forecast 100-year program costs to more than A$21 million and more than A$13.3 million respectively to retain residual Ht/Ho of 0.9000 (Table 3; Figs 1–3).
Incorporating biobanking technology into hypothetical G. vitellina and G. alba populations designed to meet 95% and 99% genetic retention targets would result in comparatively higher program costs and colony sizes (n = 33 and 167 founder males; same number of live females respectively), but still considerably less than colony sizes required for non-back-crossed populations (Table 3). Populations of G. vitellina and G. alba designed to maintain 95% of initial source population heterozygosity (n = 33 founder males; same number of live females per generation) using biobanking technology to back-cross every generation would each require more than A$1 million in biobanking-specific investment costs across 100 years. This would allow a reduction in the live colony size of 780 live individuals to a constant live colony size of only 33 G. vitellina and G. alba individuals (live females) in each program. This reduces total forecast 100-year program costs of A$945 million and A$576 million to A$39.7 million and A$24.7 million respectively in order to retain residual Ht/Ho of 0.9500 after the captive period (Table 3; Figs 1–3). Back-crossing every generation into G. vitellina and G. alba populations designed to maintain 99% source heterozygosity (n = 167 live females) would require A$1.7 million in 100-year biobanking-specific investment but would allow a reduction in required census captive colony numbers of 3983 individuals to 167 live G. vitellina and G. alba females in each program. This results in total forecast 100-year program costs of A$195.9 million and more than A$120 million respectively in order to retain residual Ht/Ho of 0.9900 after the captive period (Table 3; Figs 1–3).
We present estimates of costing scenarios (comprised of additional infrastructure and variable labour costs) for incorporating biobanking technology into the modelled amphibian captive breeding program if it were to be conducted at husbandry costs currently encountered in Perth Zoo’s Native Species Breeding Program (Table 3).
In G. vitellina and G. alba populations designed to retain 90%, 95% and 99% heterozygosity, the rate of inbreeding (Ft) is decreased considerably when back-crossing every generation (Table 3; Fig. 1). Biobanked G. vitellina and G. alba populations provide substantial cost reductions against the non-biobanked colony sizes required to achieve equivalent genetic targets (Table 3) and are even less expensive than estimates of the cost of the current head-starting program (Figs 2, 3) for 90% and 95% targets. For biobanked populations designed to meet the more ambitious genetic retention target of 99% Ht/Ho, the costs are considerably higher in G. vitellina and G. alba (Table 3; Figs 2, 3), but not excessively larger than maintaining the current head-start wild population supplementation program. Reductions in live captive colony numbers significantly increase rates of inbreeding in non-back-crossed populations (Fig. 1), leading to very large increases in costs required to achieve genetic targets (Table 3).
Discussion
Based on genetic and cost modelling scenarios generated in this study, we propose biobanking technology could be incorporated as a lower-cost approach to the operation of long-term captive breeding colonies of G. vitellina and G. alba, where the aim is to maintain a high level of wild-type, source population genetic diversity in a colony established from a single group of founders. The implementation of this approach would be subject to the development and optimisation of biobanking protocols for these species. Our modelling suggests significant cost savings, greatly reduced inbreeding and reduced requirement for large live colony sizes from combining conventional colony maintenance approaches with biobanking and ARTs. Extending this approach to other species, biobanking could allow heterozygosity retention targets of 90% of source populations (Soulé et al. 1986) to be achieved across a range of Australian amphibian captive breeding programs, and could be extended to more ambitious targets of 95–99% heterozygosity (Howell et al. 2020) within realistic cost frameworks.
We postulate a broad transitional pathway of actions to incorporate biobanking technology into Australia’s ex situ management of amphibians (Table 4). This would require species-specific protocol development delivered by well-funded applied research programs designed to close knowledge gaps in the reproductive sciences for candidate amphibian species (Table 5). Recognising the potential merits of biobanking technology could provide a new direction for Australian amphibian ex situ management that has the potential to deliver substantial genetic and economic outcomes, and ultimately free economic resources to support captive programs for a greater quantum of at-risk Australian amphibian species.
![]() |
In the genetic modelling of Howell et al. (2020), the three drivers of census population size in captive colonies are the Ne/N ratio, the generation interval and the proportion of initial heterozygosity retained at the end of 100 years. Of these, Ne/N and generation interval vary as a function of life history traits and will vary from species to species. Hence, in the present study, both G. vitellina and alba achieve 90% heterozygosity retention in non-back-crossed colonies over 100 years with N > 400, whereas the equivalent for R. pretiosa is N > 1800 live animals (Howell et al. 2020); these differences are driven by the generation interval (3 years for R. pretiosa; 4 years for G. vitellina and G. alba) and the Ne/N (0.086 for R pretiosa; 0.3 for G. vitellina and G. alba; Mace 1986; Phillipsen et al. 2010). Inspection of model outputs from Howell et al. (2020) and the present study shows that the N required to meet 90% heterozygosity retention with back-crossing for R. pretiosa is 58 (97% decline from 1826), compared with 17 (96% decline from 400) for G. vitellina and G. alba. There is a much greater reduction in census N in absolute terms for species with a lower Ne/N and longer generation interval. This means that species with shorter generation intervals and lower Ne/N ratios will benefit most in terms of cost benefits and a reduced number of live animals required in the colony if biobanking is used.
Economic costs from modelling captive breeding will also vary with life history traits, for example aquatic breeding species such as R. pretiosa (Pearl et al. 2009) versus terrestrial breeding species without an aquatic tadpole stage such as G. vitellina and G. alba (Clulow and Swan 2018), with differing costs of biobanking and ART in the back-crossed colonies. Taking genetic and costing models together, the outcomes of using biobanking may therefore differ between species, in addition to the economic benefits. Nevertheless, absolute numbers that must be kept alive in colonies for equivalent genetic heterozygosity targets are reduced in all cases when back-crossing with biobanked genomes is used. In addition, for a given census population size, there is always a genetic benefit in using biobanking. In practice, benefits to captive breeding institutions will likely vary across species with clutch size (Morrison and Hero 2003). Species with larger clutch sizes are expected to generate a higher output of animals optimal for reintroductions, but also raise overall holding costs if animals are not removed from the colony; here the key benefit is the quantum of genetically fit individuals for release to the wild. Although the overall output benefits of incorporating the biobanking approach into the management of species with small clutch sizes is expected to be lower, the clear potential benefit in this case is potentially maximising the contribution of all females in the colony through assisted reproduction, as well as the genetic fitness of output animals. This approach may be particularly powerful for species with small clutch sizes with captive husbandry issues, which could otherwise be a detrimental combination in breed-for-release programs.
We focused on modelling the minimum numbers of animals needed to be maintained alive in colonies and the minimalist biobanking scenario required to achieve the heterozygosity targets. In practice, larger numbers and variations in biobanking protocols may be pursued to achieve additional outcomes, at relatively little additional expense. For high-value banked genetic resources, some risk management strategies would be recommended, such as storage of replicated samples in multiple locations (Mintzer et al. 2013; Morrin and Robinson 2013). This would increase the costs of biobanking, but not substantially (Della Togna et al. 2020). In addition, some captive-assurance populations may hold larger numbers of live animals to deal with catastrophic demographic events in wild populations, and perhaps an N of around 100 may be closer to a realistic minimum. For example, Species Survival Plan Programs had an average N of 137 (Hodskins 1997). Adjusting live numbers to accommodate these scenarios falls within the range of modelling scenarios in this paper (Table 3; Figs 2, 3), and would not add substantially to costs compared with non-banked scenarios.
The approach suggested here for the Geocrinia species may also deliver major genetic and cost efficiencies to captive breeding programs for other Australian amphibians. For example, consider the maximum numbers of other amphibians kept in captive programs for various Australian amphibians provided in Harley et al. (2018): 410 southern corroboree frogs Pseudophryne corroboree, 465 northern corroboree frogs Pseudophryne pengilleyi, 1000 Baw Baw frogs Philoria frosti, >1000 spotted tree frogs Litoria spenceri and 1340 Alpine tree frogs Litoria verreauxii alpina. It is uncertain what the intrinsic rate of inbreeding in these captive breeding programs is because these captive breeding programs do not yet have any DNA profiling or genetic studbooks. It is also uncertain what the actual Ne/N ratios are (as for the Geocrinia complex in captivity), but these may vary considerably. In modelling for the present paper, we opted for a conservative approach by using the mean captive Ne/N ratio of 0.3 for vertebrate species (Mace 1986) in the absence of more definitive values. Although species-specific values do exist for G. vitellina and G. alba (Table 1; Driscoll 1999), these are much higher than other estimates for amphibians that account for the same variables (Table 1). Nevertheless, we have also run the models with other Ne/N values, resulting in low (Table 1; Supplementary Table S4) and high (Table 1; Supplementary Table S5) extremes of possible colony size under alternative Ne/N ratios (the species-specific wild-derived values as well as the median for various temporal estimates for amphibians) and the relative outcomes are the same. These scenarios still result in similar proportionate benefits genetically (Supplementary Tables S4, S5; Supplementary Figs S1–S4) and from a cost perspective when back-crossing with biobanked founder spermatozoa is undertaken. Because we do not know Ne/N for these other Australian species or the generation intervals in practice in those captive breeding programs, we cannot be certain of the potential reduction in live colony size that could be achieved while achieving 90% (or other) heterozygosity retention targets. Nevertheless, for each of these species, it is likely to be substantial, as for Geocrinia and R. pretiosa mentioned above, with a well over 90% reduction in the required number of live animals in captive colonies.
There is some uncertainty as to the actual costs associated with incorporating biobanking technology into the captive management of amphibian species, regardless of species, because there are no captive programs where such procedures are routinely incorporated into the breeding programs. Our modelling suggests that when these procedures are developed and optimised, these would be minimal compared with total program costs (Table 3). In this respect, our modelling for Geocrinia is consistent with modelling for R. pretiosa (Howell et al. 2020), and likely to hold true for most amphibian species in captive programs. Costs associated with cryostoring G. vitellina and G. alba spermatozoa (A$14 000 in set-up costs and more than A$700 in Year 1 labour costs) and using frozen samples through IVF to generate live offspring (more than A$460 000 in potential accumulated 100-year labour costs) represent 4.5% and 7% of total captive program costs for G. vitellina and G. alba respectively in populations designed to retain 90% heterozygosity (Table 3). Costs associated with performing recurring back-crosses within captive colonies (using ARTs such as IVF) will vary across species and taxa due to differences in life history traits (particularly generation length), as well as in reproductive strategies, which will determine the applicability of available ARTs (e.g. aquatic breeding species vs terrestrial breeders, as mentioned above). R. pretiosa populations designed to retain 90% heterozygosity required accumulated labour costs of C$67 000 (A$69 000) across 100-years for recurrent backcrosses at 3-year intervals (Howell et al. 2020), compared with A$460 000 proposed here for G. vitellina and G. alba for recurrent back-crosses occurring every 4 years (Table 3).
The parameters and assumptions of the genetic modelling (Table 2) ensure that biobanked populations retain the benefits of traditional captive breeding programs and would allow the continued contribution of captive breeding institutions to in situ and ex situ conservation outcomes. A biobanking program for Geocrinia and other species would still provide an output of individuals for research, translocation and disease mitigation, as well as individuals for public displays (providing public engagement and education), transfer of individuals (also applies to frozen material) and the subdivision of populations between institutions to bolster genetics and establish new programs. Howell et al. (2020) noted that reintroducing founder genomes reflecting wild-type, source population genotypes each generation to the captive population would have a range of fitness benefits, including reduced selection for domestication alleles that would benefit translocated animals (Frankham 2008; Allentoft and O’Brien 2010). Output animals from biobanked populations would likely be better suited for successful translocation (Griffiths and Pavajeau 2008; Robert 2009; Skerratt et al. 2016), due to genetic fitness, with the additional benefit of lower costs of colony maintenance before release (Table 3; Figs 2, 3).
There is no doubt that there are various successful captive breeding programs for Australian amphibians mentioned above, and presented in Table 4 and Harley et al. (2018). The Geocrinia species program represents a good example, with high survivorship and regular reintroductions to the wild to bolster in situ populations. Despite documented success, it is unclear whether the financial sustainability exists for this success in Australia’s captive breeding efforts to be maintained long enough to continue bolstering wild populations under persistent threats or to meet the time frames demanded by current accepted global targets for genetic diversity (90% genetic retention for 100 years; Soulé et al. 1986; Harley et al. 2018; Howell et al. 2020). The Geocrinia program would also have the added genetic benefit of regular sourcing of new animals from the wild (therefore likely being subject to minimal domestication and inbreeding, although this cannot be quantified in the present study) and it would be expected that this program would have higher genetic diversity than closed captive breeding programs from the same group of founders. However, programs that rely heavily on collection from in situ environments (particularly for highly threatened species) are highly vulnerable to stochastic events (e.g. bushfire and drought; Hoffmann et al. 2021) as well as the persistent threat of disease (Bower et al. 2017; Scheele et al. 2017). Mass mortality and emerging threats can wipe out significant genetic diversity from in situ populations permanently without added frozen insurance (e.g. the mass amphibian mortality seen in the 2019–20 Black Summer Bushfires; WWF 2020) and the persistence of disease in the landscape usually means this driver of amphibian decline cannot yet be properly mitigated before the release of captive-bred animals back into the wild (Skerratt et al. 2016; Scheele et al. 2017), although see Clulow et al. (2018a) for some emerging hope. Species in other conventional captive breeding programs would be equally vulnerable to this phenomenon without the biobanking approach, and species under no captive management are especially vulnerable. The closed system we advocate in the present study would maintain high levels of source population heterozygosity from single founder groups at lower long-term costs (Table 3; Figs 1–3) and would therefore be largely immune from these challenges and could allow long-term insurance while in situ threats persist.
Della Togna et al. (2020) and Howell et al. (2020) argued that the most promising model for incorporating biobanking and ARTs into the captive management of amphibians to deliver outcomes and make practical use of frozen samples is additional infrastructure within established captive breeding institutions and frameworks. We argue here that many of the strategic partnerships, infrastructure and skills already exist across Australia’s ex situ network that could produce practical biobanking examples with a range of potential candidate species in well-resourced captive breeding and research programs across the network (Harley et al. 2018). An important transitional step towards practical biobanking in captive breeding will be the closing of species-specific knowledge gaps in the underlying reproductive sciences through applied research programs to develop biobanking protocols for candidate species (Table 4). Recent advances in the reproductive sciences for amphibians (Clulow et al. 2014; Clulow and Clulow 2016, 2018), could allow knowledge gaps to be closed in 2–5 years for individual species candidates with targeted applied research programs on the key knowledge gaps precluding the biobanking approach (Table 4). This includes the technological tools of hormone induction and the freezing, cryostorage and thawing of spermatozoa for use in optimised IVF protocols. This will require funding support, leveraged by the argument for the potential genetic and economic benefits of the technology. Funding support will require a focus on ARTs for amphibian conservation, both internally by captive breeding institutions and externally by policy makers, research funding bodies and the non-profit sector. We have provided general per-species budget estimates as an indicator of potential funding requirements and time frames (Table 5).
The actions proposed here could provide an efficient pathway to transition biobanking technology into the ex situ management of Australian amphibians by recognising and quantifying the potential cost and genetic benefits in order to leverage research funding and in-kind support, and facilitate a focused and rapid approach to the required applied research (Table 4). Once a practical reality, biobanking technology in captive breeding programs could generate considerable institutional cost savings, freeing economic resources to allow captive programs with high genetic retention rates for a greater number of at-risk Australian amphibians.
Conflicts of interest
John Clulow, Simon Clulow and Natalie Calatayud are guest Associate Editors. Despite this relationship, they did not at any stage have editor-level access to this manuscript while in peer review, as is the standard practice when handling manuscripts submitted by an editor of this Journal. The authors have no further conflicts of interest to declare.
Acknowledgements
Lachlan G. Howell acknowledges the team at Perth Zoo within the Native Species Breeding Program, including Peter Mawson, Caroline Lawrence, Cathy Lambert and Greg Priest, for hosting him at the zoo for data collection and providing access to costs and other data relevant to the modelling presented in this study. This research did not receive any specific funding, but Lachlan G. Howell acknowledges financial support through the Australian Government’s Research Training Program Scholarship. All authors acknowledge the Nyoongar People of South-West Australia, the traditional owners of the land on which white-bellied and orange-bellied frogs live, and pay respect to the Nyoongar Elders both past and present.
References
Allentoft, M., and O’Brien, J. (2010). Global amphibian declines, loss of genetic diversity and fitness: a review. Diversity (Basel) 2, 47–71.| Global amphibian declines, loss of genetic diversity and fitness: a review.Crossref | GoogleScholarGoogle Scholar |
Ananjeva, N. B., Uteshev, V. K., Orlov, N. L., Ryabov, S. A., Gakhova, E. N., Kaurova, S. A., Kramarova, L. I., Shishova, N. V., and Browne, R. K. (2017). Comparison of the modern reproductive technologies for amphibians and reptiles. Russ. J. Herpetol. 24, 275–290.
| Comparison of the modern reproductive technologies for amphibians and reptiles.Crossref | GoogleScholarGoogle Scholar |
Bishop, P. J., Angulo, A., Lewis, J. P., Moore, R. D., Rabb, G. B., and Moreno, J. G. (2012). The amphibian extinction crisis – what will it take to put the action into the Amphibian Conservation Action Plan? Sapiens 5, 1406.
Bloxam, Q. M. C., and Tonge, S. J. (1995). Amphibians: suitable candidates for breeding-release programmes. Biodivers. Conserv. 4, 636–644.
| Amphibians: suitable candidates for breeding-release programmes.Crossref | GoogleScholarGoogle Scholar |
Bower, D. S., Lips, K. R., Schwarzkopf, L., Georges, A., and Clulow, S. (2017). Amphibians on the brink. Science 357, 454–455.
| Amphibians on the brink.Crossref | GoogleScholarGoogle Scholar | 28774916PubMed |
Browne, R. K., Silla, A. J., Upton, R., Della Togna, G., Marcec-Greaves, R., Shishova, N. V., Uteshev, V. K., Proaño, B., Pérez, O. D., and Mansour, N. (2019). Sperm collection and storage for the sustainable management of amphibian biodiversity. Theriogenology 133, 187–200.
| Sperm collection and storage for the sustainable management of amphibian biodiversity.Crossref | GoogleScholarGoogle Scholar | 31155034PubMed |
Clulow, J., and Clulow, S. (2016). Cryopreservation and other assisted reproductive technologies for the conservation of threatened amphibians and reptiles: bringing the ARTs up to speed. Reprod. Fertil. Dev. 28, 1116–1132.
| Cryopreservation and other assisted reproductive technologies for the conservation of threatened amphibians and reptiles: bringing the ARTs up to speed.Crossref | GoogleScholarGoogle Scholar |
Clulow, S., and Clulow, J. (2018). The state of cryopreservation and assisted reproductive technologies for the conservation of amphibians and reptiles. Cryobiology 85, 142.
| The state of cryopreservation and assisted reproductive technologies for the conservation of amphibians and reptiles.Crossref | GoogleScholarGoogle Scholar |
Clulow, S., and Swan, M. (2018). ‘A Complete Guide to Frogs of Australia.’ (Australian Geographic: Sydney.)
Clulow, J., Trudeau, V. L., and Kouba, A. J. (2014). Amphibian declines in the twenty-first century: why we need assisted reproductive technologies. In ‘Reproductive Sciences in Animal Conservation’. (Eds W. V. Holt, J. L. Brown and P. Comizzoli.) pp. 275–316. (Springer: New York.)
Clulow, S., Gould, J., James, H., Stockwell, M., Clulow, J., and Mahony, M. (2018a). Elevated salinity blocks pathogen transmission and improves host survival from the global amphibian chytrid pandemic: Implications for translocations. J. Appl. Ecol. 55, 830–840.
| Elevated salinity blocks pathogen transmission and improves host survival from the global amphibian chytrid pandemic: Implications for translocations.Crossref | GoogleScholarGoogle Scholar |
Clulow, J., Pomering, M., Herbert, D., Upton, R., Calatayud, N., Clulow, S., Mahony, M. J., and Trudeau, V. L. (2018b). Differential success in obtaining gametes between male and female Australian temperate frogs by hormonal induction: a review. Gen. Comp. Endocrinol. 265, 141–148.
| Differential success in obtaining gametes between male and female Australian temperate frogs by hormonal induction: a review.Crossref | GoogleScholarGoogle Scholar | 29859744PubMed |
Clulow, J., Upton, R., Trudeau, V. L., and Clulow, S. (2019). Amphibian assisted reproductive technologies: moving from technology to application. In ‘Reproductive Sciences in Animal Conservation’. (Eds P. Comizzoli, J. L. Brown and W. V. Holt.) pp. 413–463. (Springer: Cham, Switzerland.)
Conde, D. A., Colchero, F., Güneralp, B., Gusset, M., Skolnik, B., Parr, M., Byers, O., Johnson, K., Young, G., and Flesness, N. (2015). Opportunities and costs for preventing vertebrate extinctions. Curr. Biol. 25, R219–R221.
| Opportunities and costs for preventing vertebrate extinctions.Crossref | GoogleScholarGoogle Scholar | 25784036PubMed |
Conroy, S. D. S. (2001). Population biology and reproductive ecology of Geocrinia alba and G. vitellina, two threatened frogs from southwestern Australia. Ph.D. Thesis, University of Western Australia, Perth.
Crow, J. F., and Kimura, M. (1970). ‘An Introduction to Population Genetics Theory.’ (Harper and Rowe: New York.)
Della Togna, G., Howell, L. G., Clulow, J., Langhorne, C. J., Marcec-Greaves, R., and Calatayud, N. E. (2020). Evaluating amphibian biobanking and reproduction for captive breeding programs according to the Amphibian Conservation Action Plan objectives. Theriogenology 150, 412–431.
| Evaluating amphibian biobanking and reproduction for captive breeding programs according to the Amphibian Conservation Action Plan objectives.Crossref | GoogleScholarGoogle Scholar | 32127175PubMed |
Driscoll, D. A. (1997). Mobility and metapopulation structure of Geocrinia alba and Geocrinia vitellina, two endangered frog species from southwestern Australia. Aust. J. Ecol. 22, 185–195.
| Mobility and metapopulation structure of Geocrinia alba and Geocrinia vitellina, two endangered frog species from southwestern Australia.Crossref | GoogleScholarGoogle Scholar |
Driscoll, D. A. (1998). Genetic structure, metapopulation processes and evolution influence the conservation strategies for two endangered frog species. Biol. Conserv. 83, 43–54.
| Genetic structure, metapopulation processes and evolution influence the conservation strategies for two endangered frog species.Crossref | GoogleScholarGoogle Scholar |
Driscoll, D. A. (1999). Genetic neighbourhood and effective population size for two endangered frogs. Biol. Conserv. 88, 221–229.
| Genetic neighbourhood and effective population size for two endangered frogs.Crossref | GoogleScholarGoogle Scholar |
Farquharson, K. A., Hogg, C. J., and Grueber, C. E. (2017). Pedigree analysis reveals a generational decline in reproductive success of captive Tasmanian devil (Sarcophilus harrisii): implications for captive management of threatened species. J. Hered. 108, 488–495.
| Pedigree analysis reveals a generational decline in reproductive success of captive Tasmanian devil (Sarcophilus harrisii): implications for captive management of threatened species.Crossref | GoogleScholarGoogle Scholar | 28379457PubMed |
Farquharson, K. A., Hogg, C. J., and Grueber, C. E. (2018). A meta-analysis of birth-origin effects on reproduction in diverse captive environments. Nat. Commun. 9, 1055.
| A meta-analysis of birth-origin effects on reproduction in diverse captive environments.Crossref | GoogleScholarGoogle Scholar | 29535319PubMed |
Frankham, R. (1995). Effective population size/adult population size ratios in wildlife: a review. Genet. Res. 66, 95–107.
| Effective population size/adult population size ratios in wildlife: a review.Crossref | GoogleScholarGoogle Scholar |
Frankham, R. (2008). Genetic adaptation to captivity in species conservation programs. Mol. Ecol. 17, 325–333.
| Genetic adaptation to captivity in species conservation programs.Crossref | GoogleScholarGoogle Scholar | 18173504PubMed |
Frankham, R., Ballou, J. D., and Briscoe, D. A. (2010). ‘Introduction to Conservation Genetics.’ 2nd edn. (Cambridge University Press: Cambridge, UK; New York)
Frankham, R., Ballou, J. D., Ralls, K., Eldridge, M., Dudash, M. R., Fenster, C. B., Lacy, R. C., and Sunnucks, P. (2019). ‘A Practical Guide for Genetic Management of Fragmented Animal and Plant Populations.’ (Oxford University Press: Oxford.)
Gagliardo, R., Crump, P., Griffith, E., Mendelson, J., Ross, H., and Zippel, K. (2008). The principles of rapid response for amphibian conservation, using the programmes in Panama as an example. Int. Zoo Yearb. 42, 125–135.
| The principles of rapid response for amphibian conservation, using the programmes in Panama as an example.Crossref | GoogleScholarGoogle Scholar |
Gillespie, G. R., Roberts, J. D., Hunter, D., Hoskin, C. J., Alford, R. A., Heard, G. W., Hines, H., Lemckert, F., Newell, D., and Scheele, B. C. (2020). Status and priority conservation actions for Australian frog species. Biol. Conserv. 247, 108543.
| Status and priority conservation actions for Australian frog species.Crossref | GoogleScholarGoogle Scholar |
González-del-Pliego, P., Freckleton, R. P., Edwards, D. P., Koo, M. S., Scheffers, B. R., Pyron, R. A., and Jetz, W. (2019). Phylogenetic and trait-based prediction of extinction risk for data-deficient amphibians. Curr. Biol. 29, 1557–1563.e3.
| Phylogenetic and trait-based prediction of extinction risk for data-deficient amphibians.Crossref | GoogleScholarGoogle Scholar | 31063716PubMed |
Griffiths, R. A., and Pavajeau, L. (2008). Captive breeding, reintroduction, and the conservation of amphibians. Conserv. Biol. 22, 852–861.
| Captive breeding, reintroduction, and the conservation of amphibians.Crossref | GoogleScholarGoogle Scholar | 18616746PubMed |
Grueber, C. E., Reid-Wainscoat, E. E., Fox, S., Belov, K., Shier, D. M., Hogg, C. J., and Pemberton, D. (2017). Increasing generations in captivity is associated with increased vulnerability of Tasmanian devils to vehicle strike following release to the wild. Sci. Rep. 7, 2161.
| Increasing generations in captivity is associated with increased vulnerability of Tasmanian devils to vehicle strike following release to the wild.Crossref | GoogleScholarGoogle Scholar | 28526824PubMed |
Harley, D., Mawson, P. R., Olds, L., McFadden, M., and Hogg, C. J. (2018). The contribution of captive breeding in zoos to the conservation of Australia’s threatened fauna. In ‘Recovering Australian Threatened Species: A Book of Hope’. (Eds S. Garnett, P. Latch, D. Lindenmayer and J. Woinarski.) pp. 281–294. (CSIRO Publishing: Melbourne.)
Hero J.-M., and Roberts D. (2004). Geocrinia alba. The IUCN Red List of Threatened Species 2004: e.T9031A12952112
Hodskins L. G. (1997). Annual report on conservation and science Volume 1: conservation program reports. Bethesda, MD: American Zoo Aquarium Association.
Hoffmann, E. P., Williams, K., Hipsey, M. R., and Mitchell, N. J. (2021). Drying microclimates threaten persistence of natural and translocated populations of threatened frogs. Biodivers. Conserv. 30, 15–34.
| Drying microclimates threaten persistence of natural and translocated populations of threatened frogs.Crossref | GoogleScholarGoogle Scholar |
Hogg, C. J. (2013). Preserving Australian native fauna: zoo-based breeding programs as part of a more unified strategic approach1. Aust. J. Zool. 61, 101–108.
| Preserving Australian native fauna: zoo-based breeding programs as part of a more unified strategic approach1.Crossref | GoogleScholarGoogle Scholar |
Holt, W. V., Bennett, P. M., Volobouev, V., and Watwon, P. F. (1996). Genetic resource banks in wildlife conservation. J. Zool. (Lond.) 238, 531–544.
| Genetic resource banks in wildlife conservation.Crossref | GoogleScholarGoogle Scholar |
Howell, L. G., and Rodger, J. C. (2018). An examination of funding for terrestrial vertebrate fauna research from Australian federal government sources. Pacific Conserv. Biol. 24, 142–147.
| An examination of funding for terrestrial vertebrate fauna research from Australian federal government sources.Crossref | GoogleScholarGoogle Scholar |
Howell, L. G., Frankham, R., Rodger, J. C., Witt, R. R., Clulow, S., Upton, R. M. O., and Clulow, J. (2020). Integrating biobanking minimises inbreeding and produces significant cost benefits for a threatened frog captive breeding programme. Conserv. Lett. , e12776.
| Integrating biobanking minimises inbreeding and produces significant cost benefits for a threatened frog captive breeding programme.Crossref | GoogleScholarGoogle Scholar |
Kouba, A. J., and Vance, C. K. (2009). Applied reproductive technologies and genetic resource banking for amphibian conservation. Reprod. Fertil. Dev. 21, 719–737.
| Applied reproductive technologies and genetic resource banking for amphibian conservation.Crossref | GoogleScholarGoogle Scholar | 19567216PubMed |
Kouba, A. J., Vance, C., Calatayud, N., Rowlison, T., Langhorne, C., and Willard, S. (2012). Assisted reproductive technologies (ART) for amphibians. In ‘Amphibian Husbandry Resource Guide’. 2nd edn. (Eds V. A. Poole.) pp. 60–118. (Amphibian Taxon Advisory Group, American Association of Zoos and Aquariums: Silver Spring, MD.)
Kouba, A. J., Lloyd, R. E., Houck, M. L., Silla, A. J., Calatayud, N., Trudeau, V. L., Clulow, J., Molinia, F., Langhorne, C., and Vance, C. (2013). Emerging trends for biobanking amphibian genetic resources: the hope, reality and challenges for the next decade. Biol. Conserv. 164, 10–21.
| Emerging trends for biobanking amphibian genetic resources: the hope, reality and challenges for the next decade.Crossref | GoogleScholarGoogle Scholar |
Lees, C., Mcfadden, M., and Hunter, D. (2013). Genetic management of southern corroboree frogs: workshop report and plan. IUCN SSC Conservation Breeding Specialist Group, Apple Valley, MN, USA.
Mace, G. (1986). Genetic management of small populations. Int. Zoo Yearb. 24, 167–174.
| Genetic management of small populations.Crossref | GoogleScholarGoogle Scholar |
Mawson, P. R., and Lambert, C. (2017). Challenges of operating a multi-species breeding-for-release facility at Perth Zoo, Australia. Int. Zoo Yearb. 51, 165–174.
| Challenges of operating a multi-species breeding-for-release facility at Perth Zoo, Australia.Crossref | GoogleScholarGoogle Scholar |
Mintzer, J. L., Kronenthal, C. J., Kelly, V., Seneca, M., Butler, G., Fecenko-Tacka, K., Altamuro, D., and Madore, S. J. (2013). Preparedness for a natural disaster: How Coriell planned for Hurricane Sandy. Biopreserv. Biobank. 11, 216–220.
| Preparedness for a natural disaster: How Coriell planned for Hurricane Sandy.Crossref | GoogleScholarGoogle Scholar | 24845588PubMed |
Monfort, S. L. (2014). ‘Mayday Mayday Mayday’, the millennium ark is sinking! In ‘Reproductive Sciences in Animal Conservation’. (Eds W. V. Holt, J. L. Brown and P. Comizzoli.) pp. 15–31. (Springer: New York)
Morrin, H. R., and Robinson, B. A. (2013). Sustaining a biobank through a series of earthquake swarms: lessons learned from our New Zealand experience. Biopreserv. Biobank. 11, 211–215.
| Sustaining a biobank through a series of earthquake swarms: lessons learned from our New Zealand experience.Crossref | GoogleScholarGoogle Scholar | 24845587PubMed |
Morrison, C., and Hero, J. (2003). Geographic variation in life-history characteristics of amphibians: a review. J. Anim. Ecol. 72, 270–279.
| Geographic variation in life-history characteristics of amphibians: a review.Crossref | GoogleScholarGoogle Scholar |
Morrison, C. E., Johnson, R. N., Grueber, C. E., and Hogg, C. J. (2020). Genetic impacts of conservation management actions in a critically endangered parrot species. Conserv. Genet. 21, 869–877.
| Genetic impacts of conservation management actions in a critically endangered parrot species.Crossref | GoogleScholarGoogle Scholar |
Murphy, J. B., and Gratwicke, B. (2017). History of captive management and conservation amphibian programs mostly in zoos and aquariums. Part I – anurans. Herpetol. Rev. 48, 241–260.
Pearl, C. A., Adams, M. J., and Leuthold, N. (2009). Breeding habitat and local population size of the Oregon spotted frog (Rana pretiosa) in Oregon, USA. Northwest. Nat. (Olymp. Wash.) 90, 136–147.
| Breeding habitat and local population size of the Oregon spotted frog (Rana pretiosa) in Oregon, USA.Crossref | GoogleScholarGoogle Scholar |
Phillipsen, I. C., Bowerman, J., and Blouin, M. (2010). Effective number of breeding adults in Oregon spotted frogs (Rana pretiosa): genetic estimates at two life stages. Conserv. Genet. 11, 737–745.
| Effective number of breeding adults in Oregon spotted frogs (Rana pretiosa): genetic estimates at two life stages.Crossref | GoogleScholarGoogle Scholar |
Ralls, K., Ballou, J. D., and Templeton, A. (1988). Estimates of lethal equivalents and the cost of inbreeding in mammals. Conserv. Biol. 2, 185–193.
| Estimates of lethal equivalents and the cost of inbreeding in mammals.Crossref | GoogleScholarGoogle Scholar |
Robert, A. (2009). Captive breeding genetics and reintroduction success. Biol. Conserv. 142, 2915–2922.
| Captive breeding genetics and reintroduction success.Crossref | GoogleScholarGoogle Scholar |
Roberts D., and Hero J.-M. (2004). Geocrinia vitellina. The IUCN Red List of Threatened Species 2004: e.T9032A12952365
Roberts, D., Conroy, S., and Williams, K. (1999). Conservation status of frogs in Western Australia. In ‘Declines and Disappearances of Australian Frogs’. (Eds A. Campbell.) pp. 177–184. (Environment Australia: Canberra.)
Scheele, B. C., Skerratt, L. F., Grogan, L. F., Hunter, D. A., Clemann, N., McFadden, M., Newell, D., Hoskin, C. J., Gillespie, G. R., and Heard, G. W. (2017). After the epidemic: ongoing declines, stabilizations and recoveries in amphibians afflicted by chytridiomycosis. Biol. Conserv. 206, 37–46.
| After the epidemic: ongoing declines, stabilizations and recoveries in amphibians afflicted by chytridiomycosis.Crossref | GoogleScholarGoogle Scholar |
Scheele, B. C., Pasmans, F., Skerratt, L. F., Berger, L., Martel, A., Beukema, W., Acevedo, A. A., Burrowes, P. A., Carvalho, T., and Catenazzi, A. (2019). Amphibian fungal panzootic causes catastrophic and ongoing loss of biodiversity. Science 363, 1459–1463.
| Amphibian fungal panzootic causes catastrophic and ongoing loss of biodiversity.Crossref | GoogleScholarGoogle Scholar | 30923224PubMed |
Silla, A. J. (2011). Effect of priming injections of luteinizing hormone-releasing hormone on spermiation and ovulation in Gϋnther’s toadlet, Pseudophryne guentheri. Reprod. Biol. Endocrinol. 9, 68.
| Effect of priming injections of luteinizing hormone-releasing hormone on spermiation and ovulation in Gϋnther’s toadlet, Pseudophryne guentheri.Crossref | GoogleScholarGoogle Scholar | 21599916PubMed |
Silla, A. J. (2013). Artificial fertilisation in a terrestrial toadlet (Pseudophryne guentheri): effect of medium osmolality, sperm concentration and gamete storage. Reprod. Fertil. Dev. 25, 1134–1141.
| Artificial fertilisation in a terrestrial toadlet (Pseudophryne guentheri): effect of medium osmolality, sperm concentration and gamete storage.Crossref | GoogleScholarGoogle Scholar | 23174151PubMed |
Silla, A. J., and Byrne, P. G. (2019). The role of reproductive technologies in amphibian conservation breeding programs. Annu. Rev. Anim. Biosci. 7, 499–519.
| The role of reproductive technologies in amphibian conservation breeding programs.Crossref | GoogleScholarGoogle Scholar | 30359086PubMed |
Silla, A. J., and Roberts, J. D. (2012). Investigating patterns in the spermiation response of eight Australian frogs administered human chorionic gonadotropin (hCG) and luteinizing hormone-releasing hormone (LHRHa). Gen. Comp. Endocrinol. 179, 128–136.
| Investigating patterns in the spermiation response of eight Australian frogs administered human chorionic gonadotropin (hCG) and luteinizing hormone-releasing hormone (LHRHa).Crossref | GoogleScholarGoogle Scholar | 22909973PubMed |
Skerratt, L. F., Berger, L., Clemann, N., Hunter, D. A., Marantelli, G., Newell, D. A., Philips, A., McFadden, M., Hines, H. B., and Scheele, B. C. (2016). Priorities for management of chytridiomycosis in Australia: saving frogs from extinction. Wildl. Res. 43, 105–120.
| Priorities for management of chytridiomycosis in Australia: saving frogs from extinction.Crossref | GoogleScholarGoogle Scholar |
Snyder, N. F. R., Derrickson, S. R., Beissinger, S. R., Wiley, J. W., Smith, T. B., Toone, W. D., and Miller, B. (1996). Limitations of captive breeding in endangered species recovery. Conserv. Biol. 10, 338–348.
| Limitations of captive breeding in endangered species recovery.Crossref | GoogleScholarGoogle Scholar |
Soulé, M., Gilpin, M., Conway, W., and Foose, T. (1986). The millenium ark: how long a voyage, how many staterooms, how many passengers? Zoo Biol. 5, 101–113.
| The millenium ark: how long a voyage, how many staterooms, how many passengers?Crossref | GoogleScholarGoogle Scholar |
Wardell-Johnson, G., Roberts, J. D., Driscoll, D., and Williams, K. (1995). Orange-bellied and white-bellied frogs recovery plan, 2nd edition. Department of Conservation and Land Management, Perth, WA, Australia.
Wintle, B. A., Cadenhead, N. C. R., Morgain, R. A., Legge, S. M., Bekessy, S. A., Cantele, M., Possingham, H. P., Watson, J. E. M., Maron, M., and Keith, D. A. (2019). Spending to save: what will it cost to halt Australia’s extinction crisis? Conserv. Lett. 12, e12682.
| Spending to save: what will it cost to halt Australia’s extinction crisis?Crossref | GoogleScholarGoogle Scholar |
Woinarski, J. C. Z., Garnett, S. T., Legge, S. M., and Lindenmayer, D. B. (2017). The contribution of policy, law, management, research, and advocacy failings to the recent extinctions of three Australian vertebrate species. Conserv. Biol. 31, 13–23.
| The contribution of policy, law, management, research, and advocacy failings to the recent extinctions of three Australian vertebrate species.Crossref | GoogleScholarGoogle Scholar |
WWF (2020). Australia’s 2019–2020 bushfires: the wildlife toll. Interim report. Available at: https://www.wwf.org.au/what-we-do/bushfire-recovery/in-depth/resources/australia-s-2019-2020-bushfires-the-wildlife-toll#gs.wfuly6
Zippel, K., Johnson, K., Gagliardo, R., Gibson, R., McFadden, M., Browne, R., Martinez, C., and Townsend, E. (2011). The Amphibian Ark: a global community for ex situ conservation of amphibians. Herpetol. Conserv. Biol. 6, 340–352.