Will the experimental population control of an invasive crayfish influence the diet and trophic position of a native crayfish? An assessment using stable isotopes
Sarah B. O’Hea Miller



A
B
Abstract
Trophic overlap between invasive and native species can cause a competitive displacement of the native. In Australian freshwaters, the invasive crayfish Cherax destructor has proliferated into the ranges of native Euastacus species.
To examine trophic overlap between C. destructor and the critically endangered Euastacus dharawalus and determine whether C. destructor population control causes shifts in the diet and trophic position of E. dharawalus.
We compared δ13C and δ15N values of tissue from both species collected from two sites. Population control of C. destructor was then conducted at one site, whereas the C. destructor population remained uncontrolled at the second site. The δ13C and δ15N values of E. dharawalus were then once again assessed.
Both species occupied similar trophic positions and consumed similar proportions of each food resource prior to control efforts. However, we found increases in the contribution of detritus to the diet of E. dharawalus following the removal of C. destructor, whereas no dietary shifts occurred at the uncontrolled site.
The increase in detrital consumption suggests that control of C. destructor increased the availability of a preferred resource. Further, dietary competition between C. destructor and E. dharawalus is likely and reinforces C. destructor representing a substantial threat to Euastacus crayfish.
Keywords: Cherax, conservation, critically endangered, crustacean, dietary overlap, Euastacus, freshwater, isotopic niche.
Introduction
Competitive interactions between invasive and native species are recognised as a key underlying mechanism behind the displacement of natives (Holway 1999; Thomson 2004; Yang et al. 2012). As an invasive species integrates into a novel ecosystem, several scenarios are possible. Invasives may use previously unexploited resources, thereby minimising competitive interactions with native species (Juncos et al. 2015). Where all resources are being exploited, competition may lead to native–invader trophic niche partitioning (Tran et al. 2015) or each species may increase their niche width to meet their energetic requirements as resources are depleted (Svanbäck and Bolnick 2006; Dominguez Almela et al. 2021). These circumstances may facilitate the co-existence of invasives with natives. However, in instances where resources are limited, interspecific competition with a competitively dominant invasive species can lead to the exclusion of a native (Chase et al. 2002).
In freshwater ecosystems, the spread of invasive species is a threatening process of particular concern (Sala et al. 2000; Carpenter et al. 2011) and trophic overlap between invasives and natives in these ecosystems is considered a driving force in native population decline (Sampson et al. 2009; Feiner et al. 2013; Modesto et al. 2021). However, instances of trophic partitioning enabling co-existence of natives and invaders in freshwaters are also apparent (e.g. Coccia et al. 2016; Bašić et al. 2019). For example, in a controlled experiment, Bašić et al. (2019) reported that the presence of an invasive fish species resulted in the niche constriction and partitioning of a native fish, with no detrimental effects to their condition or growth rate. Further, on the removal of an invasive species, the trophic recovery of natives from partitioning is possible. For example, Rogosch and Olden (2020) reported the displacement of native fish species from higher to lower trophic levels by invading species; then, following the removal of the invasives, the trophic level of natives returned to the higher position.
Freshwater crayfish are particularly problematic invaders in freshwater ecosystems. Their ability to occupy multiple trophic levels due to their omnivorous diets (Nyström et al. 1996) means that invasive crayfish can substantially disrupt the ecological functioning of a freshwater system (Stenroth and Nyström 2003; Geiger et al. 2005). However, in some instances invasive crayfish may have positive engineering effects on ecosystems where they do not naturally occur (Emery-Butcher et al. 2020). Native freshwater crayfish are considered keystone species in ecosystems where they naturally occur because of their broad diet that can include algae, biofilm, macrophytes, macroinvertebrates and vertebrates (Momot et al. 1978; Matthews et al. 1993; Parkyn et al. 1997; Giling et al. 2009; Reynolds et al. 2013; Vollmer and Gall 2014). There have been several studies of the trophic interactions of native and invasive crayfish. Many of these have reported that trophic niche overlap between invasive and native crayfish is evident in invaded ecosystems (Beatty 2006; Olsson et al. 2009; Ercoli et al. 2014: Magoulick and Piercey 2016). However, in some instances, trophic partitioning between invasive and native species has also been reported (Jackson et al. 2014; Pacioglu et al. 2019). Notably, there has yet to be an experimental investigation of the impact of the presence v. absence of an invasive crayfish on the trophic position of a native crayfish.
In freshwater ecosystems where invasive crayfish have become established, stable δ13C and δ15N isotope analysis has been able to provide a representation of food assimilation overlap and trophic interactions between invasive and native crayfish (e.g. Beatty 2006; Olsson et al. 2009; Ercoli et al. 2014; Magoulick and Piercey 2016). In freshwater crayfish, stable isotope analysis provides an advantageous means of assessing diet, as the gastric mill of crayfish renders the accurate identification of gut contents difficult (Linton et al. 2009). When examining trophic interactions between species using δ13C and δ15N isotopes, the isotopic or trophic niche is often used to infer dietary breadth (Layman et al. 2007) and the position of each niche in isotopic space can be used to determine the degree of niche overlap between species (Jackson et al. 2011). Further, using δ15N values of the consumer and base of the food web, the trophic position that each species occupies within a food web can be calculated (Post 2002) and the proximity of these positions between species can then be determined. Additionally, the relative importance of assimilated food items of consumers can be determined using the isotopic values of the consumer and likely dietary sources (Phillips 2012; Parnell et al. 2013). Dietary sources can then be compared between species to determine the likelihood of assimilation overlap. Hence, stable isotope analysis can provide valuable insight into the trophic relationships between invasive and native crayfish and assess the likelihood of competition between the species.
The freshwater crayfish, Cherax destructor, has spread beyond its natural range of the Murray–Darling River system (https://australian.museum/learn/animals/crustaceans/black-yabbie/) and has become an increasingly concerning invader in Australian freshwater systems (Coughran et al. 2009; Coughran and Daly 2012). Cherax destructor exhibits generalist feeding habits (Faragher 1983; Goddard 1988; Beatty 2006), which is likely to be contributing to its success as an invasive species. In Western Australia, C. destructor was reported to occupy a trophic position similar to that of the previously established Cherax cainii in summer; however, in winter there was a large trophic separation between the two species (Beatty 2006). Cherax destructor has not only spread into the range of its congeners, but also those of many native Australian Euastacus species (Furse and Coughran 2011; Coughran and Daly 2012; McCormack 2012). The ability of C. destructor to outcompete certain Euastacus species (Hazlett et al. 2007; Cerato et al. 2019; O’Hea Miller et al. 2022) means that its proliferation into these ranges is troubling. Even so, there has yet to be any research into the trophic interactions between C. destructor and a Euastacus species.
One Euastacus species currently under threat from C. destructor is the critically endangered Euastacus dharawalus or the Fitzroy Falls spiny crayfish (McCormack 2013; McCormack 2016). This species is found only in a 9-km section of Wildes Meadow Creek (WMC) located in the Southern Highlands region of New South Wales (NSW), Australia. The introduction of C. destructor to farm dams in the area is likely to have led to the establishment of the invasive species in WMC. Recent surveys have reported higher abundances of C. destructor than E. dharawalus at some sites (NSW Department of Primary Industries, unpubl. data, 2021) and the two species have been observed competing over food in situ (O’Hea Miller et al. 2023). Thus, the potential for food resource competition between the two species is high, although it is currently unknown to what extent this competition occurs. Understanding the extent and the mechanisms through which C. destructor competitively affects E. dharawalus will assist in the development of management plans for this critically endangered species. Therefore, we used stable isotope analyses to determine (1) the degree of dietary and trophic overlap between E. dharawalus and C. destructor and (2) whether the diet and trophic position of E. dharawalus shifts following population control of the invasive C. destructor.
Materials and methods
Study sites
Wildes Meadow Creek (WMC) is a small stream located above Fitzroy Falls in NSW, Australia, with an approximate total length of 12 km. However, the construction of Fitzroy Falls reservoir in 1974 inundated ~3 km of creek and cut the WMC into two sections of ~7.5 km above and 750 m below the reservoir. A manipulation and control site were selected along the section of creek situated above the reservoir on agricultural land (Fig. 1). The manipulation site was a ~300-m section of creek with the upper 150 m dominated by invasive willows (Salix spp.) and the lower 150 m shaded by native eucalyptus trees. The control site was ~300 m upstream of the manipulation site, with a length of ~300 m. This section of creek consisted of cleared riparian margins with scattered native ferns and willow trees. Macrophytes found in the creek include Persicaria spp., Juncus spp. and Carex spp. Other vertebrate and invertebrate species found in high abundances in WMC include native Galaxias olidus (mountain galaxias), non-native Gambusia holbrooki (eastern mosquitofish) and the native freshwater shrimp Paratya australiensis.
Sample collection and invasive crayfish removal
In March 2020, over a period of 2 days, crayfish were captured at both study sites by using opera house (fitted with platypus escape holes) and small net traps baited with pilchards and set for a period of ~2 h. It was determined that the use of bait to capture crayfish would not interfere with stable isotope analysis (SIA) because this method provides as estimate of assimilated food sources over the long term (Bearhop et al. 2004). Further, diet-switching experiments on crayfish found that weeks to months were needed for carapace and muscle tissue to equilibrate with diets (Mazumder et al. 2018). Therefore, because isotopic turnover for crayfish tissue takes time, if ingested, the bait was not assimilated by crayfish and thus had no effect on the isotopic values of crayfish.
The last segment of the fourth pair of walking legs was clipped from seven E. dharawalus individuals from the manipulation site (four females, three males) and control site (four females, three males) (size range 37–58-mm occipital carapace length, OCL). The removal of this appendage was deemed to have the least effect on a member of the genus Euastacus (Robert McCormack, pers. comm.) and approval was gained prior to appendage removal (DPI Scientific Collecting Permit Number P20/0003 – 1.0). A sample size of seven was selected because E. dharawalus is a critically endangered species and analyses by Mazumder et al. (2008) found that a sample size of seven is appropriate for stable isotope analysis, although a minimum of five is required. Leg samples were also collected from five C. destructor individuals (one female, four males) from the manipulation site and six from the control site (four females, two males) (size range 30–35 mm OCL). Samples were placed in airtight zip-lock bags and stored frozen at −20°C. Additionally, in March 2020 across the length of each site, a diverse range of potential dietary sources was also collected. Macrophytes (Juncus usitatus, Persicaria strigosa and Carex sp.), detritus and algae were collected by hand or by using a pond net. Paratya australiensis, Gambusia holbrooki, Galaxias maculatus were collected either by small net trap or by dip net. Three replicates from each species were collected because this samples size is routinely used for dietary sources in stable isotope mixing model studies (e.g. Jackson et al. 2017; Linzmaier et al. 2020).
To determine the impact that the population control of C. destructor may have on the diet and trophic position of E. dharawalus, regular removals of C. destructor from the manipulation site between March 2020 and February 2021 were undertaken to reduce the abundance of the invader as much as possible. The control site was left undisturbed. Because there is a delay between a dietary shift and isotopic change of an organism’s tissue (Glon et al. 2016), removals of C. destructor were conducted monthly from April 2020 to February 2021 to ensure their consistent reduced abundance over the 12-month period. However, we note that removals were ceased in winter months (June, July and August) because C. destructor was found to be inactive during these months. Baited opera house and small net traps were used to capture C. destructor from the study site. Traps were deployed for a period of 2 h; this was repeated over the course of a day until the number of C. destructor individuals found in the traps was less than three. All C. destructor individuals captured were anesthetised using an ice bath and euthanised by a brain spike. Any E. dharawalus individuals captured were returned to their exact point of capture. Following this, tissue samples were again collected from seven E. dharawalus individuals from both the manipulation site (five females, two males) and the control site (four females, three males) (size range 38–59 mm OCL) in March of 2021. Active tracking of both C. destructor and E. dharawalus showed that both species exhibit very small home ranges (O’Hea Miller et al., unpubl. data) and therefore are unlikely to move between sites.
Sample preparation and analysis
For sample preparation, all walking-leg tissue samples were defrosted, rinsed with reverse osmosis water, oven-dried at 60°C for at least 24 h and then ground into a fine powder with a mortar and pestle. The mortar and pestle were rinsed with acetone between samples to prevent cross-contamination. All dietary samples were rinsed with reverse osmosis water, dried and ground into a fine power. Plant and detritus materials were ground using a laboratory mill grinder (IKA model A11). After processing, subsamples weighing 0.5 mg (±0.1) from each ground sample were loaded into tin capsules and analysed on a continuous-flow stable isotope ratio mass spectrometer (Model Thermo Fisher Flash 2000 HT EA) at the Australian Nuclear Science and Technology Organisation (ANSTO) in Sydney, Australia. The data are reported relative to International Atomic Energy Agency (IAEA) secondary standards, and all results were verified relative to Vienna Pee Dee Belemnite (VPDB) for carbon and air for nitrogen. The results were accurate to ±0.3 (‰) for δ13C and δ15N. The final values are reported in delta (δ) notation in parts per thousand (‰) format relative to IAEA certified standards and were calculated by the following formula:
where X is either δ13C or δ15N (‰) and Rsample is either 13C/12C or 15N/14N of a sample and Rstandard is either 13C/12C or 15N/14N of the secondary standard.
The C:N mass ratio was calculated for each stable isotope sample. Variation of lipid content in tissues can influence δ13C values (DeNiro and Epstein 1978; Post et al. 2007). Therefore, a lipid-normalisation equation (δ13Cnormalised = δ13Cuntreated – 3.32 + 0.99 × C:N; Post et al. 2007) was used for the δ13C values for crayfish tissue samples with an overall mean elemental C:N ratio of >3.5. Lipid correction was not applied to dietary sources because lipids can be assimilated by the consumer (Mazumder et al. 2018).
Data analysis
To assess whether the catch rate of Cherax destructor decreased over the 12-month period, C. destructor catches from each trap were pooled for each month when population control efforts were conducted. These were then divided by the total trap hours to provide a standardised catch per unit effort (CPUE) (i.e. individuals captured per trap hour).
All statistical analyses were conducted using RStudio (ver. 3.5.1, Posit Software, PBC, Boston, MA, USA, see https://posit.co/products/open-source/rstudio/). Normality of the δ13C and δ15N data was first assessed by visual inspection of Q–Q plots and histograms. To test for homogeneity of variances, Levene’s tests were performed on untransformed data. If this returned a significant result, data were log-transformed.
To test for significant isotopic differences between the native and invasive species, a three-way ANOVA was used to compare isotopic values (δ13C and δ15N) between species (E. dharawalus and C. destructor), sites (manipulation and control) and sexes (male and female). A second three-way ANOVA was performed to compare δ13C and δ15N values of E. dharawalus between years (2020 and 2021), sites (manipulation and control) and sexes to determine whether the predominant removal of C. destructor from the manipulation site caused isotopic shifts in E. dharawalus. Tukey’s tests were performed after ANOVA tests to make post hoc comparisons among levels of significant terms.
Trophic positions (TPO) of C. destructor at the manipulation and control sites in 2020 and trophic positions of E. dharawalus at both sites in 2020 and 2021 were calculated using the following formula reported by Post (2002):
where λ is the trophic position of the food source used to estimate δ15Nbase of food web. In the present study, detritus was identified as the likely base of the food web (i.e. λ = 1). Δn is the trophic fractionation constant. Typically, a fractionation constant of 3.4‰ is used (Post 2002); however, as argued by Beatty (2006), crustaceans have been reported to show a lower mean enrichment of ~2‰ (Vanderklift and Ponsard 2003). Therefore, in calculating the trophic positions of C. destructor and E. dharawalus in the present study, a 2‰ trophic fractionation constant was used. To test for significant differences between the trophic positions of the native and invasive species, a two-way ANOVA was then used to compare the calculated trophic positions between species (E. dharawalus and C. destructor) and sites (manipulation and control). To test for significant shifts in the trophic positions of E. dharawalus between years (2020 and 2021) and sites, a second two-way ANOVA was performed.
To establish the isotopic niche width of E. dharawalus and C. destructor at both sites and across both years, the SIBER (Stable Isotope Bayesian Ellipses in R) package (ver. 2.1.8, see https://cran.r-project.org/web/packages/SIBER/index.html; Jackson et al. 2011) was used to compute isotopic niche-width metrics, including carbon range (CR), nitrogen range (NR), total area (TA) and standard ellipse areas corrected for samples sizes (SEAc) (Layman et al. 2007). The CR and NR were measured by the lowest and highest values of either δ13C or δ15N; NR reflects the degree of dietary resource use across trophic levels and CR indicates the variation in primary producer consumption (Layman et al. 2007). The TA metric uses a convex hull to create a niche area that encompasses all data points, including extreme δ13C or δ15N values, and represents the total trophic diversity and dietary breadth of a population (Layman et al. 2007). The SEA differs from the TA representation of trophic niche developed by Layman et al. (2007) in that it is an ellipse that incorporates only the core 40% of the stable isotope data and is therefore less sensitive to outliers and small sample sizes (Jackson et al. 2011). Further, this metric can be altered to reduce the bias in niche area caused by differing sample sizes by slightly enlarging the ellipses of small sample sizes while retaining their geometric shape (SEAc) (Jackson et al. 2011).
These metrics were also calculated on the basis of pooled data from both sites for C. destructor and E. dharawalus in both years to determine species-wide isotopic niche widths. The isotopic niche overlap between E. dharawalus and C. destructor from the control and manipulation site in 2020 and between E. dharawalus from the control and manipulation site in 2020 and 2021 was estimated by calculating the overlap between SEAc of each consumer group by using SIBER (Jackson et al. 2011). This was then expressed in parts per thousand squared (‰2) to represent isotopic niche space for species (Riccialdelli et al. 2017; Suchomel and Belk 2022). Percentage overlap was then calculated using the below formula:
where O is equal to the overlap value (‰), A1 is the SEAc (‰) of the first consumer group and A2 is the SEAc (‰) of the second consumer group.
We quantified the possible relative dietary contributions (%) of likely diet sources to the isotopic make-up of E. dharawalus and C. destructor by using the Bayesian mixing model SIMMR (ver. 0.5.1.216, see https://cran.r-project.org/package=simmr; Parnell et al. 2013) in R (ver. 4.2.2, R Foundation for Statistical Computing, Vienna, Austria, see https://www.r-project.org/). SIMMR default settings for the number of iterations (10 000), burn-in (1000) and Markov-chain Monte Carlo (MCMC) chains (4) were retained in the models. To assess which putative dietary sources should be included in the mixing model, an isotopic bi-plot of the δ13C and δ15N values of crayfish and all sampled putative dietary sources was created. Owing to their proximity to the crayfish in isotopic space, Juncus usitatus, Persicaria strigosa, Carex sp., detritus and algae were selected as source inputs for the model. However, the positioning of Paratya australiensis, Galaxias maculatus and Gambusia holbrooki on the bi-plot indicated that these species were not likely to be assimilated by native or invasive crayfish and were thus not included in SIMMR diet-mixing analyses. The average (±s.e.) fractionation factors used in the mixing models were 1.5‰ (±0.63) for δ13C and 3.4‰ (±0.74) for δ15N, on the basis of fractionation values determined from the literature (Mazumder et al. 2018).
Results
Cherax destructor population control
Catch rates of Cherax destructor underwent a substantial decline from April 2020 to May 2020 (Fig. 2). We note that this is likely to be associated with the reduction in temperature and subsequent reduction in C. destructor activity that occurs at this time of year. Catch rates then increased gradually from September 2020 to November 2020 (Fig. 2), followed by a decline to the lowest recorded catch rate in February 2021 (Fig. 2).
The mean (±s.e.) catch per unit effort (CPUE) of Cherax destructor in each month when population control efforts took place at the manipulation site. Population control efforts were not conducted from June to August 2020 because of low water temperatures and, therefore, limited activity in C. destructor. These months are therefore excluded from the plot.
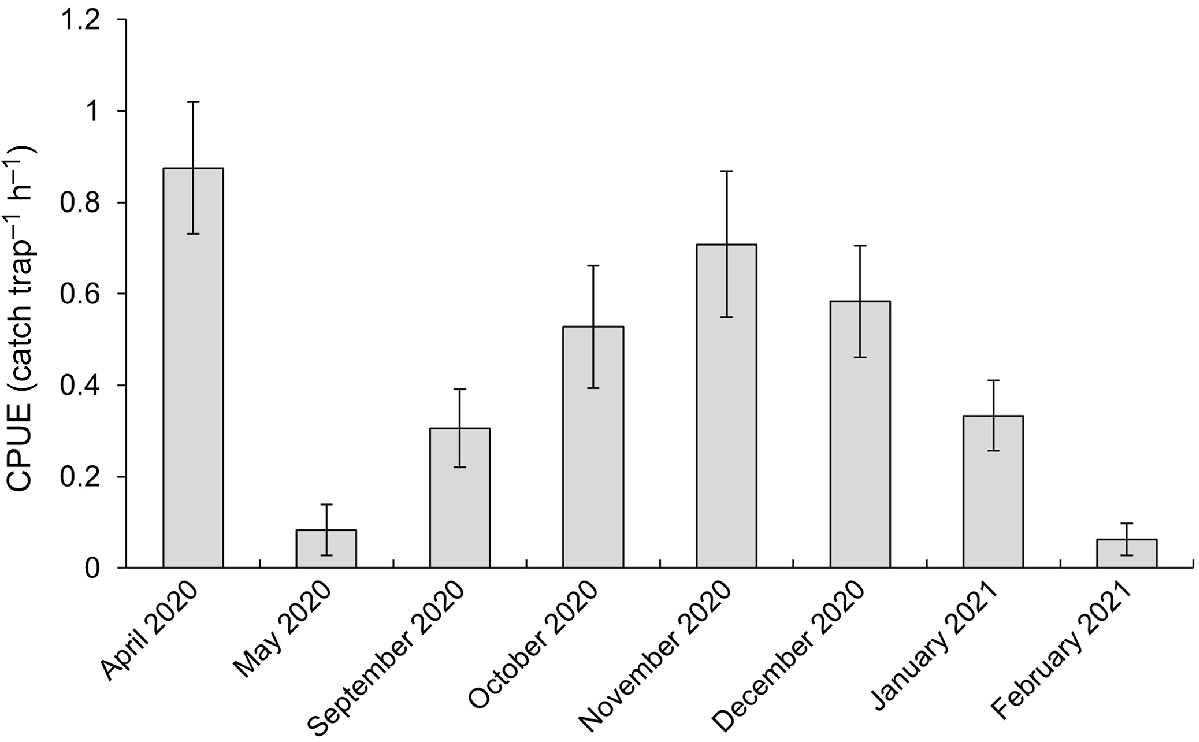
δ13C and δ15N signatures
The δ15N values of E. dharawalus showed a significant year by sex interaction (Table 1). δ15N was significantly depleted in 2021 compared with 2020 at both the manipulation and control sites, although only in female E. dharawalus (Fig. 3). For δ13C values, there was a significant three-way interaction among year, site and sex (Table 1), with δ13C being significantly depleted in 2021 compared with 2020, although only in female E. dharawalus from the control site (Fig. 3).
Source of variation | δ13C | δ15N | |||||||||||
---|---|---|---|---|---|---|---|---|---|---|---|---|---|
SS | d.f.1 | d.f.2 | MS | F | P | SS | d.f.1 | d.f.2 | MS | F | P | ||
Year | 4.32 | 1 | 26 | 4.32 | 10.68 | 0.004 | 18.08 | 1 | 26 | 18.08 | 10.71 | 0.004 | |
Site | 0.24 | 1 | 26 | 0.24 | 0.59 | 0.451 | 0.30 | 1 | 26 | 0.30 | 0.18 | 0.678 | |
Sex | 0.01 | 1 | 26 | 0.01 | 0.02 | 0.882 | 1.43 | 1 | 26 | 1.43 | 0.85 | 0.368 | |
Year × site | 0.30 | 1 | 24 | 0.30 | 0.73 | 0.402 | 0.68 | 1 | 24 | 0.68 | 0.41 | 0.532 | |
Year × sex | 1.57 | 1 | 24 | 1.57 | 3.87 | 0.063 | 7.68 | 1 | 24 | 7.68 | 4.55 | 0.046 | |
Year × site × sex | 2.24 | 1 | 22 | 2.24 | 5.53 | 0.029 | 0.01 | 1 | 22 | 0.01 | <0.01 | 0.981 | |
Residuals | 8.09 | 0.40 | 33.78 | 1.69 |
P-values in bold indicate statistical significance.
Bi-plot of mean (±s.e.) δ13C and δ15N signatures of male and female Euastacus dharawalus and from manipulation (M) and control (C) sites in 2020 and 2021.
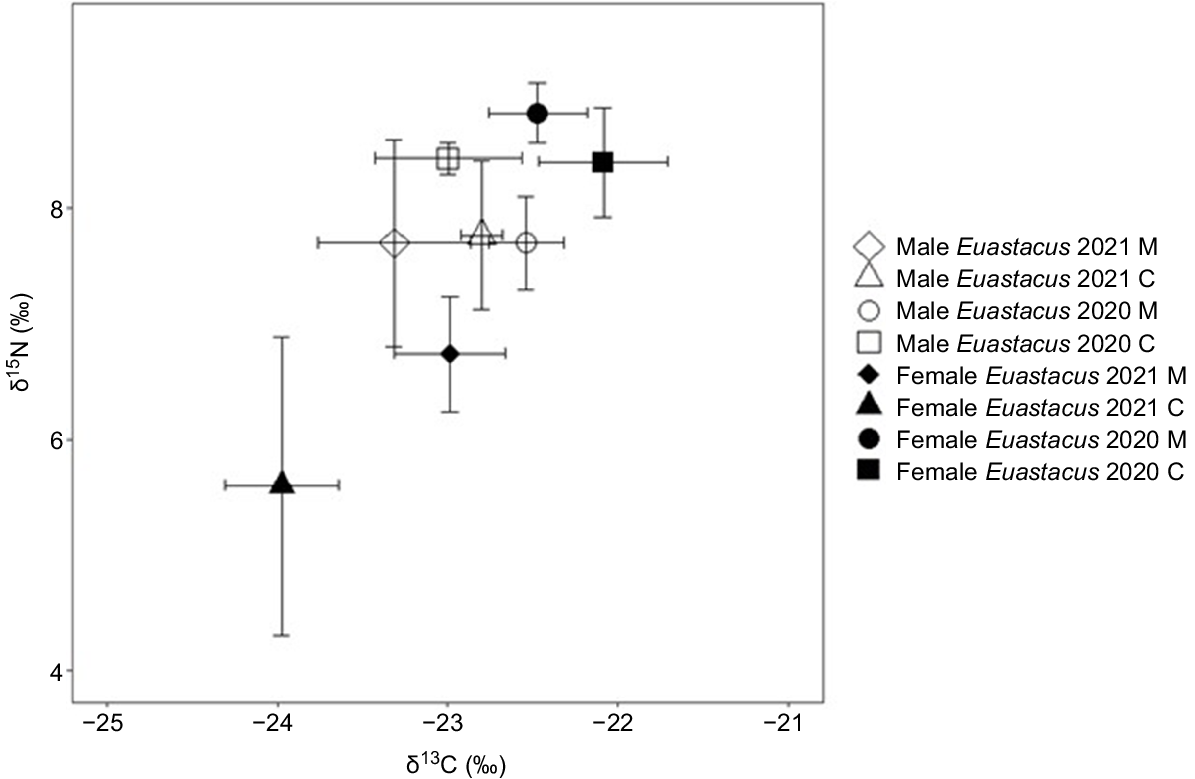
When comparing δ15N enrichment between E. dharawalus and C. destructor in 2020, we found a significant interaction between species and site (Table 2). The δ15N values of C. destructor from the manipulation site were significantly more enriched than were the δ15N values of C. destructor from the control site (Fig. 4). However, there was no significant difference between the δ15N values of E. dharawalus and C. destructor at either site, and there was no significant variation in the δ15N values of E. dharawalus from the control and manipulation site (Fig. 4).
Source of variation | δ13C | δ15N | |||||||||||
---|---|---|---|---|---|---|---|---|---|---|---|---|---|
SS | d.f.1 | d.f.2 | MS | F | P | SS | d.f.1 | d.f.2 | MS | F | P | ||
Species | 36.18 | 1 | 22 | 36.18 | 83.82 | <0.001 | 0.01 | 1 | 22 | 0.01 | 0.01 | 0.905 | |
Site | 3.16 | 1 | 22 | 3.16 | 7.32 | 0.016 | 1.22 | 1 | 22 | 1.21 | 3.07 | 0.099 | |
Sex | 0.07 | 1 | 22 | 0.07 | 0.16 | 0.691 | 0.40 | 1 | 22 | 0.40 | 1.02 | 0.328 | |
Species × site | 4.82 | 1 | 20 | 4.82 | 11.16 | 0.004 | 3.22 | 1 | 20 | 3.22 | 8.12 | 0.012 | |
Species × sex | 0.98 | 1 | 20 | 0.98 | 2.26 | 0.152 | 0.01 | 1 | 20 | 0.01 | 0.03 | 0.876 | |
Species × site × sex | 1.03 | 1 | 18 | 1.03 | 2.38 | 0.142 | 0.09 | 1 | 18 | 0.09 | 0.21 | 0.649 | |
Residuals | 6.91 | 0.43 | 6.33 | 0.40 |
P-values in bold indicate statistical significance.
Bi-plot of mean δ13C and δ15N signatures (±s.e.) of Euastacus dharawalus and Cherax destructor from manipulation (M) and control (C) sites in 2020 and of E. dharawalus from manipulation and control sites in 2021.
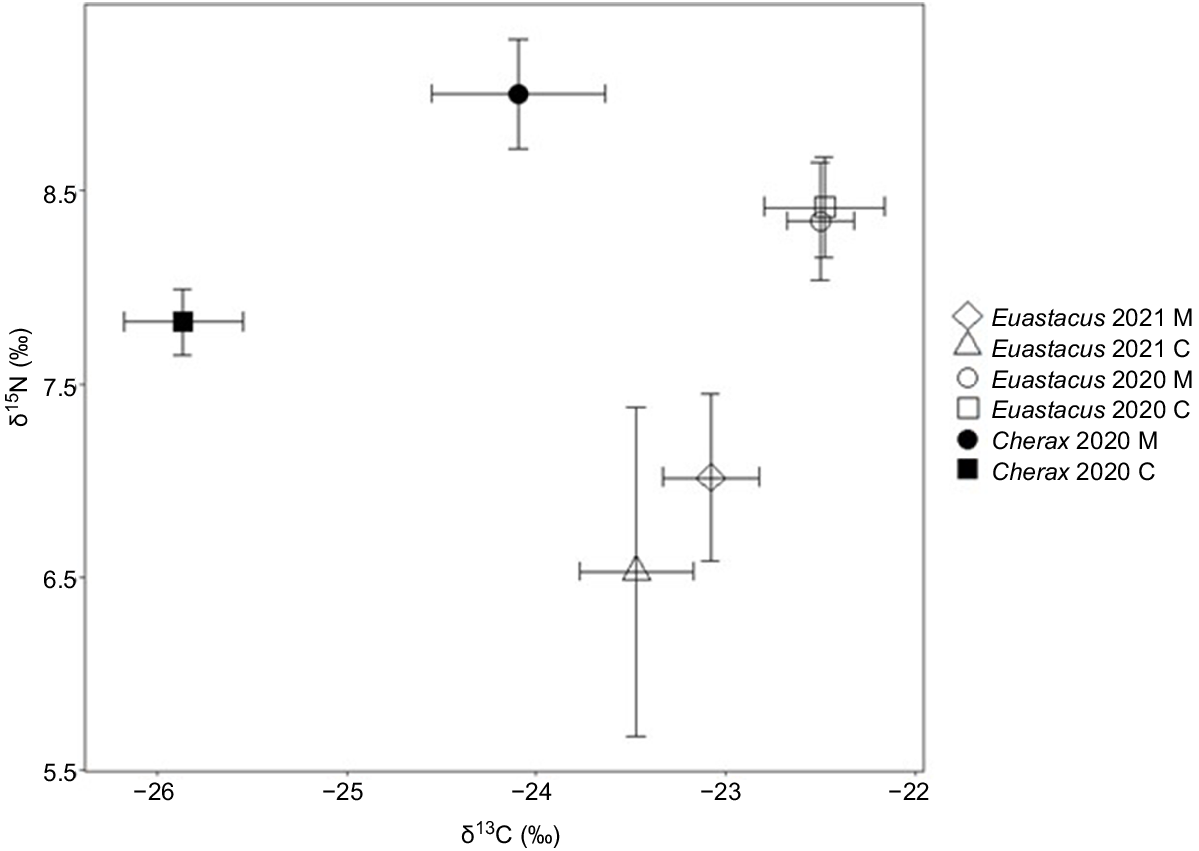
There was also a significant interaction between species and site for the δ13C values of crayfish (Table 2). The δ13C values of C. destructor were significantly more depleted than those of E. dharawalus at both the control and the manipulation site (Fig. 4). However, there was no significant difference in the δ13C values of C. destructor from the manipulation site and C. destructor from the control site (Fig. 4). Finally, no significant difference was detected in the δ13C values of E. dharawalus from the control and manipulation sites in 2020 (Fig. 4).
Trophic positions
Trophic positions of E. dharawalus were significantly related to year (ANOVA: F1,26 = 10.34, P = 0.004), with the trophic positions of E. dharawalus significantly decreasing from 2020 to 2021 at both the manipulation and the control site (Table 3). Trophic positions of E. dharawalus did not differ significantly between the sites (F1,26 = 0.01, P = 0.907) (Table 3), nor was there a significant interaction between year and site (F1,24 = 0.05, P = 0.827).
Consumer | Year | Site | Mean δ15N (±s.e.) of consumer | Mean δ15N (±s.e.) of food-web base | Trophic position of consumer | |
---|---|---|---|---|---|---|
E. dharawalus | 2020 | Control | 8.41 (±0.23) | 3.93 (±0.79) | 3.24 | |
Manipulation | 8.34 (±0.31) | 3.93 (±0.79) | 3.21 | |||
2021 | Control | 6.53 (±1.01) | 3.93 (±0.79) | 2.30 | ||
Manipulation | 7.01 (±0.43) | 3.93 (±0.79) | 2.54 | |||
C. destructor | 2020 | Control | 7.82 (±0.17) | 3.93 (±0.79) | 2.95 | |
Manipulation | 9.00 (±0.28) | 3.93 (±0.79) | 3.54 |
The trophic positions of E. dharawalus and C. destructor in 2020 did not differ significantly (ANOVA: F1,22 = 0.91, P = 0.911) (Table 3). However, there was a significant interaction between species and site (F1,20 = 5.09, P = 0.035), with the trophic position of C. destructor from the control site being significantly lower than that of C. destructor from the manipulation site (Table 3).
Dietary niche metrics
Shifts in the dietary niche metrics from 2020 to 2021 occurred at both the manipulation and the control site. The nitrogen range (NR) of E. dharawalus increased from 2020 to 2021 at both sites, but to a greater extent at the control site (Table 4), indicating an increase in the exploitation of trophically diverse food sources at both sites from 2020 to 2021. The carbon range (CR) of E. dharawalus increased from 2020 to 2021 at the manipulation site, indicating an increase in the consumption of primary producers. However, at the control site, the CR decreased from 2020 to 2021 (Table 4). The isotopic niche space of E. dharawalus underwent expansion from 2020 to 2021, with the TA and SEAc of E. dharawalus increasing from 2020 to 2021 at the species level (Table 4, Fig. 5) as well as at both sites (Table 4, Fig. 6a), thereby indicating an increase in dietary breath of E. dharawalus between the years at both sites. However, the TA and SEAc of E. dharawalus from the control site in 2021 were slightly larger than those of E. dharawalus from the manipulation site in 2021 (Table 4, Fig. 6a).
Species | Year | Site | Isotopic niche metrics | ||||
---|---|---|---|---|---|---|---|
CR (‰) | NR (‰) | TA (‰2) | SEAc (‰2) | ||||
E. dharawalus | 2020 | Control | 2.30 | 2.10 | 2.17 | 1.94 | |
Manipulation | 1.25 | 2.10 | 1.47 | 1.24 | |||
Combined | 2.30 | 2.30 | 3.08 | 1.45 | |||
2021 | Control | 1.94 | 5.90 | 3.83 | 3.21 | ||
Manipulation | 1.90 | 3.10 | 3.43 | 2.98 | |||
Combined | 2.70 | 5.90 | 7.85 | 3.40 | |||
C. destructor | 2020 | Control | 1.63 | 0.90 | 0.95 | 1.11 | |
Manipulation | 2.75 | 1.50 | 2.37 | 2.60 | |||
Combined | 3.79 | 2.30 | 5.12 | 3.00 |
Metrics calculated on the basis of combined data from both sites are also presented to demonstrate species-wide values. Carbon range (CR), nitrogen range (NR), total area (TA) and standard ellipse areas (SEAc) are presented for each group.
Bi-plot displaying the species-wide standard ellipse areas (SEAc) and 95% confidence ellipses calculated from combined δ13C and δ15N data from the manipulation and control sites for Euastacus dharawalus and Cherax destructor in 2020 and E. dharawalus in 2021.
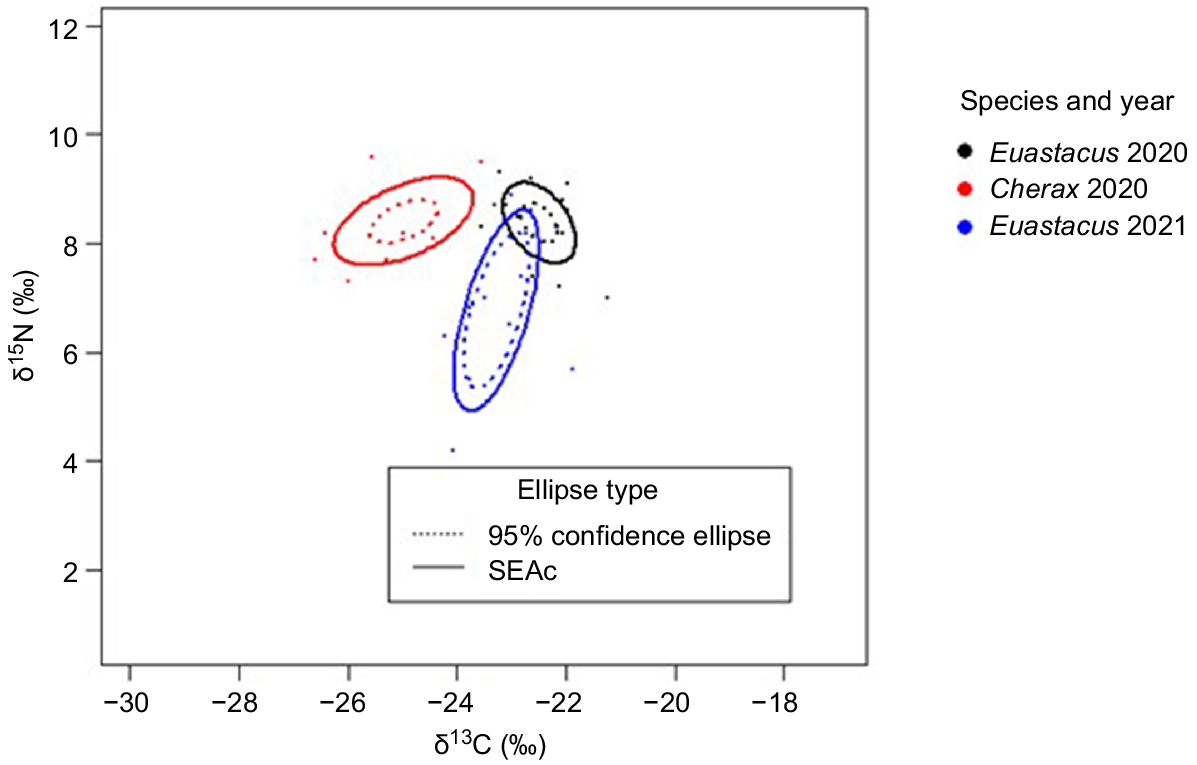
Bi-plots displaying the standard ellipse areas (SEAc) and 95% confidence ellipses calculated from δ13C and δ15N signatures of freshwater crayfish (a) E. dharawalus in 2020 and 2021 at control (C) and manipulation (M) sites and (b) Euastacus dharawalus (native) and Cherax destructor (invasive) at control (C) and manipulation (M) sites in 2020.
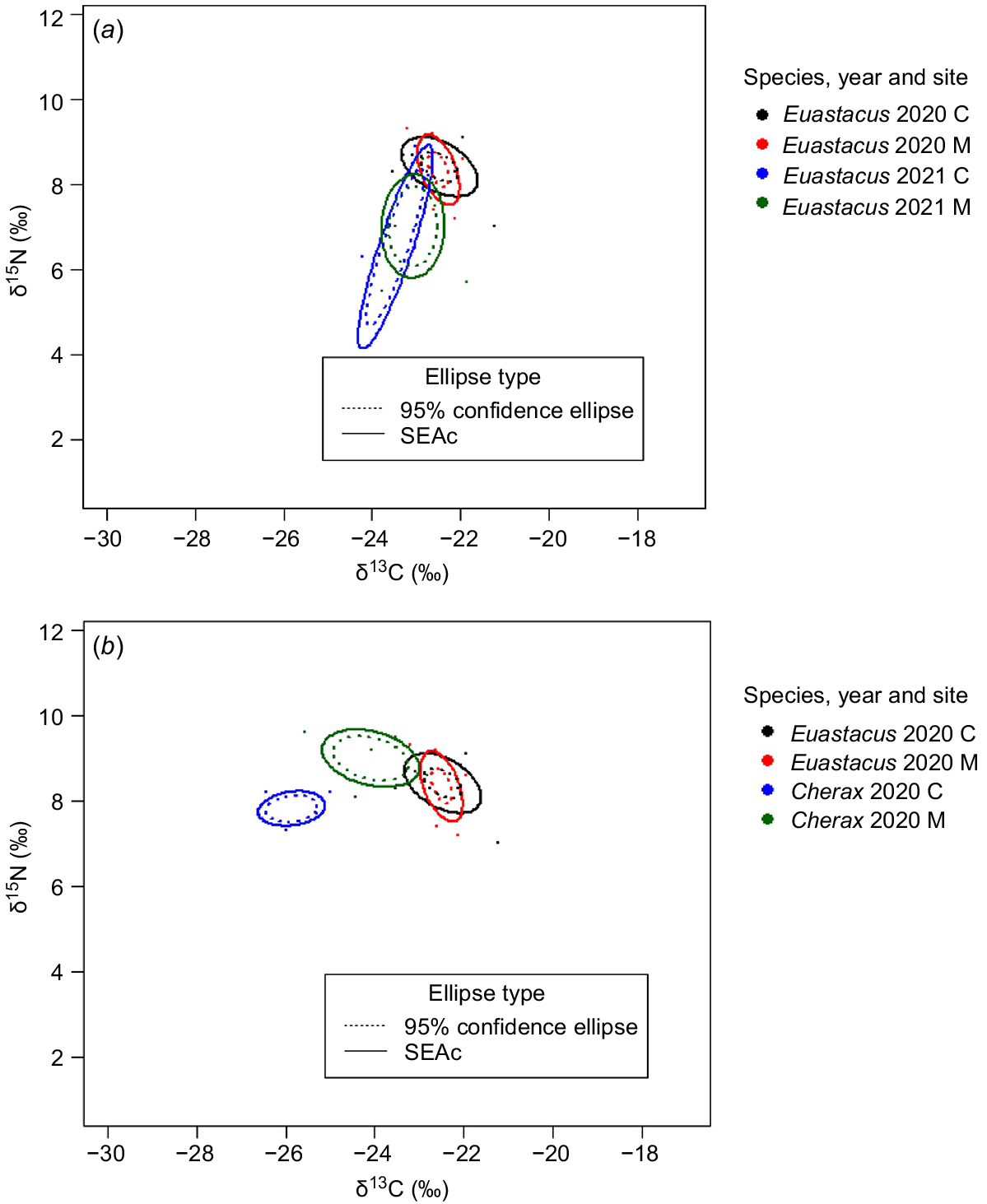
The NR of C. destructor was more constricted than that of E. dharawalus at both sites in 2020 (Table 4). However, at the manipulation site, the CR of C. destructor was wider than the CR of E. dharawalus and, at the control site, the CR of C. destructor was smaller than that of E. dharawalus (Table 4). At the species-level, C. destructor exhibited a wider isotopic niche space (SEAc 3.00‰2) than that of E. dharawalus (SEAc 1.45‰2) (Table 4, Fig. 5). However, at the site level, there was no substantial variation in the SEAc width of C. destructor and E. dharawalus (Table 4, Fig. 6b).
The area of overlap in the isotopic niche spaces of E. dharawalus between the manipulation and control sites decreased from 53% in 2020 to 35% in 2021 (Fig. 6a). Further, the SEAc values of E. dharawalus from the manipulation site in 2020 and 2021 overlapped by 3% (Fig. 6a). At the control site, the SEAc values in 2020 and 2021 overlapped by 9% (Fig. 6a), indicating that a shift occurred in the isotopic niche of E. dharawalus at both sites between the 2 years. At the species level, there was no overlap between the SEAc of E. dharawalus and SEAc of C. destructor (Fig. 5). At the manipulation site, the SEAc of E. dharawalus and that of C. destructor exhibited very little overlap (0.08%), further, at the control site the SEAc of E. dharawalus and the SEAc of C. destructor did not overlap (Fig. 6b), indicating minimal isotopic niche overlap between the two species (see Supplementary Table S1 for exact overlap and similarity values).
Contribution of dietary sources
The most important dietary source for E. dharawalus from both the control and the manipulation site in 2020 was Juncus usitatus (Table 5, Fig. 7). Detritus contributed the next most to the diet of E. dharawalus at both sites relative to other resources in 2020 (Table 5, Fig. 7). Contributions from Persicaria strigosa and Carex sp. at both sites were small relative to that of Juncus and detritus. Algae contributed the least to E. dharawalus diets at both sites in 2020 (Table 5, Fig. 7).
Species | Year | Site | Dietary source (percentage contribution) | |||||
---|---|---|---|---|---|---|---|---|
Juncus | Persicaria | Carex | Detritus | Algae | ||||
E. dharawalus | 2020 | Control | 40 (4–82) | 14 (1–46) | 12 (1–37) | 27 (2–64) | 7 (1–18) | |
Manipulation | 42 (3–85) | 12 (1–41) | 12 (1–37) | 27 (2–67) | 7 (1–18) | |||
2021 | Control | 41 (4–82) | 12 (1–46) | 13 (1–45) | 28 (1–81) | 6 (1–20) | ||
Manipulation | 34 (2–88) | 10 (1–39) | 10 (1–36) | 41 (2–88) | 5 (1–16) | |||
C. destructor | 2020 | Control | 46 (4–84) | 10 (1–32) | 9 (1–30) | 30 (2–73) | 5 (1–14) | |
Manipulation | 32 (3–76) | 19 (2–57) | 20 (2–55) | 19 (2–50) | 10 (1–27) |
Mean percentage contribution is shown, as well as the 2.5th and 97.5th percentiles of the distribution of feasible solutions of each source to consumer diet in parentheses.
Bi-plot of mean δ13C and δ15N (±s.e.) signatures of potential dietary sources and Euastacus dharawalus and Cherax destructor from manipulation (M) and control (C) sites in 2020 and Euastacus dharawalus from manipulation and control sites in 2021.
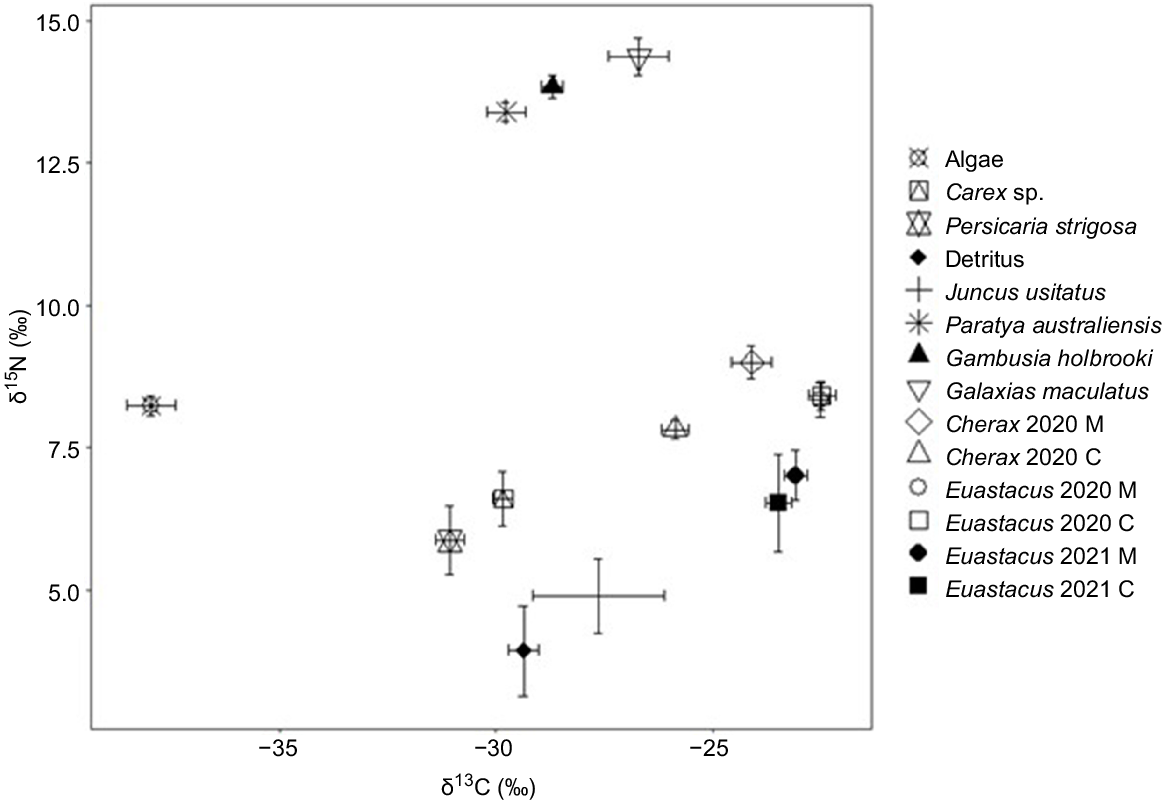
Juncus usitatus also appeared to be the most important component of the diet of C. destructor from the control and manipulation sites in 2020 (Table 5, Fig. 7). For C. destructor from the control site, detritus contributed the next most to their diet, followed by P. strigosa, Carex sp. and algae (Table 5, Fig. 7). However, for C. destructor from the manipulation site, the contribution of the remaining sources was spread more evenly with the next most important source being Carex sp., followed by detritus, P. strigosa and algae (Table 5).
Between 2020 and 2021, there was little variation in the contribution of each resource to the diet of E. dharawalus from the control site (Table 5). However, for E. dharawalus from the manipulation site, there was a reduction in the average contribution of Juncus usitatus from 42 to 34% from 2020 to 2021 (Table 5). Further, there was a substantial increase in the relative contribution of detritus from 27 to 41% from 2020 to 2021 (Table 5). The contributions of the other sources to the diet of E. dharawalus from the manipulation site varied marginally from 2020 to 2021 (Table 5).
Discussion
Diet and niche of Euastacus dharawalus in the presence versus absence of Cherax destructor
This study is the first to experimentally assess the effect of the presence versus absence of an invasive crayfish on the diet and trophic niche of a native crayfish. We found substantial shifts in the relative contributions of two of the five putative resources to the assimilated diet of E. dharawalus at the manipulation site following the removal of C. destructor. Whereas at the control site, the contributions of all analysed resources remained similar over the same time period, indicating the diet shift in E. dharawalus from the manipulation is attributable to the C. destructor removal. However, we also report shifts in the trophic positions and isotopic niches of E. dharawalus from 2020 to 2021 at both the manipulation and the control site. Further, there were significant depletions in the δ15N signatures of female E. dharawalus from 2020 to 2021 at the manipulation site and the control site and the δ13C signatures of female E. dharawalus were significantly depleted only at the control site. Therefore, because of the occurrence of these shifts at both sites, it is unlikely that they are associated with the removal of C. destructor.
We consider the shifts detected in the relative contributions of each resource to the assimilated diet of E. dharawalus at the manipulation site, compared with the very similar contributions across the years at the control site, as a likely an indication of competition between the E. dharawalus and C. destructor. The most notable change at the manipulation site was an 8% decrease in the consumption of Juncus usitatus and a 14% increase in the consumption of detritus by E. dharawalus. This increase in detritus is surprising, considering that it is reported to be less nutritionally beneficial than are macrophytes in crayfish diets (Giling et al. 2009). However, there is evidence to suggest that detritus can be a limited resource in aquatic systems (Dobson and Hildrew 1992; Mcintosh et al. 2005). Further, the biofilm that develops in the process of decay in organic matter may add nutritional value to detritus (Reid et al. 2008) and in situ observations of crayfish fights over detritus patches have been reported as more intense than fights over macrophyte resources (Bergman and Moore 2003). We suggest that detritus is a preferred resource for E. dharawalus, and in the absence of competition from C. destructor, detrital availability is increased, thus increasing consumption by E. dharawalus.
In contrast to the above finding, the shifts in the δ15N and δ13C signatures, NR and CR, trophic positions, and isotopic niche sizes from 2020 to 2021 occurred at both sites. These shifts are likely to be a consequence of environmental variation between the 2 years rather than competition. Seasonal shifts in the isotopic signatures of crayfish are well documented (Beatty 2006; Magoulick and Piercey 2016; Vesely et al. 2020; Wu et al. 2022), yet inter-annual shifts are not evident in the literature (Stenroth et al. 2006). However, in other species inter-annual variation in isotope values has been attributed to environmental change across years (e.g. Kowalczyk et al. 2015; Cerveira et al. 2020). In the present study, the most notable environmental shift from 2020 to 2021 was the transition from a period of drought to regular flow and precipitation levels in the area. In terrestrial ecosystems, isotopic niche sizes reportedly decline during periods of drought (Hayes et al. 2016; Smith et al. 2022), which is consistent with the smaller isotopic niches we found during the drier year of 2020, likely in response to the lower availability of resources. Isotopic shifts during dry periods have also been documented in freshwater. However, these studies have reported lower trophic positions of fish and crayfish during dry periods (Mazumder et al. 2012, 2017), which contrasts with the higher trophic positions of E. dharawalus we found during the drier period. Despite this, our findings indicated that environmental variation can cause isotopic shifts in E. dharawalus.
The cause of the isotopic shifts in only female E. dharawalus individuals from 2020 to 2021, and not males, is unclear. Inter-sexual isotopic variation in freshwater crayfish has been reported in the past (Larson et al. 2017; Lang et al. 2021) and sex has been observed to affect crayfish trophic function under mesocosm conditions (Usio and Townsend 2002). It has also been suggested that trophic segregation of male and female crayfish may reduce competition between the sexes to meet energetic requirements (Gherardi et al. 2001; Scalici and Gibertini 2007). Therefore, in the present study, the increase in available resources from 2020 to 2021, resulting from the end of the drought, may have enabled the sexual segregation of resources in E. dharawalus. However, additional research and a greater sample size is required to further elucidate any inter-sexual trophic segregation in E. dharawalus.
Trophic and dietary overlap between Cherax destructor and Euastacus dharawalus
The present study is the first to investigate trophic and dietary overlap between the invasive Cherax destructor and a sympatric member of the genus Euastacus. Here, we report minimal overlap in the isotopic niche of C. destructor and E. dharawalus, as well as significantly depleted δ13C of C. destructor, compared with that of E. dharawalus. Conversely, we also found no substantial variation in the δ15N enrichment, assimilated diets nor the trophic positions of the two species. Additionally, at a species level C. destructor showed a wider isotopic niche than that of E. dharawalus in 2020, although, within each site, the species’ exhibited a similar niche size. Further, there was significant between-site variation in the isotopic values, isotopic niche, trophic positions and assimilated diet of C. destructor, although this was not the case for E. dharawalus.
The wider isotopic niche of C. destructor than that of E. dharawalus at the species level is not surprising, considering the generalist feeding patterns documented for C. destructor (Faragher 1983; Goddard 1988; Beatty 2006). Further, a broader invasive isotopic niche than that of natives is a pattern previously described in other invasive–native crayfish systems. Both Olsson et al. (2009) and Ercoli et al. (2014) reported that the invasive Pacifastacus leniusculus occupies a broader isotopic niche than does native Astacus astacus at the species level. However, both studies also reported that, at the site-level, P. leniusculus exhibited a niche size similar to that of A. astacus. This is consistent with the similar niche sizes of C. destructor and E. dharawalus at the site level in the present study and is consistent with ecological plasticity in C. destructor.
It is posited that an invaders’ ecological plasticity and capacity to exploit a diverse range of resources plays an important role in their ability to establish in an ecosystem (Schmid 1984; Weis 2010). The broad species-level niche of C. destructor compared with its reduced niche size at the site level, coupled with the between-site variation of their isotopic values, assimilated diet and trophic positions, suggest that C. destructor can alter its diet and trophic position on the basis of the resources available at a site. This is in stark contrast to the minimal between-site variation we found for E. dharawalus in 2020, indicating a more fixed diet across sites in the native species. Diet and trophic plasticity in invasive crayfish relative to their native counterparts has been reported previously (Renai and Gherardi 2004). Further, C. destructor has been found to alter its diet on the basis of the density of resources (Beatty 2006) and has demonstrated dietary flexibility in contrast to a fixed diet exhibited by the native Euastacus bispinosus across sites (Johnston et al. 2011).
The minimal overlap in the isotopic niches of C. destructor and E. dharawalus diverges from what has previously been reported in certain invasive–native crayfish systems. Both Olsson et al. (2009) and Ercoli et al. (2014) reported that the isotopic niches of P. leniusculus and A. astacus strongly overlapped. Conversely, consistent with our findings, Pacioglu et al. (2019) found some degree of isotopic niche partitioning between invasive and native crayfish. Similarly, Jackson et al. (2014) documented no isotopic niche overlap between sympatric invasive crayfish. It is necessary to note that we used SEAc to estimate the isotopic niche width of crayfish, which is considered a more robust measure than the convex hull area (TA) when using lower sample sizes (Jackson et al. 2011). However, SEAc is still known to be influenced by sample size and it is recommended to exercise caution in its application when using sample sizes smaller than 30 (Syväranta et al. 2013). We acknowledge then that, owing to our low sample sizes from each site, our estimates of the isotopic niche sizes and overlap between species must be interpreted with caution.
Despite the apparent isotopic niche partitioning of E. dharawalus and C. destructor, the trophic positions of the two species were similar in 2020. Further, the assimilated diets of E. dharawalus and C. destructor, coupled with the isotopic signatures of the species relative to those of the top consumers and primary producers, indicated a more herbivorous–detrital diet for both crayfish species. However, because our data do not include isotopic signatures of certain primary consumers (i.e. aquatic macroinvertebrate taxa) from the system, we cannot know the contribution of this group to the diets of the crayfish. Even so, considerable dietary overlap between the species is evident, with substantial contributions of Juncus usitatus and detritus to the assimilated diets of both species. Previous research into the diet of Euastacus species is non-existent; however, investigations into the diet of C. destructor have produced variable results. Beatty (2006) reported a predominantly carnivorous diet of C. destructor in Western Australia in the summer, consisting mostly of Gambusia holbrooki. However, this was followed by a shift to a more herbivorous–detrital diet in the winter. Other research has reported that the assimilated diet of C. destructor is primarily carnivorous, and its stomach contents vary between mostly animal to mostly plant matter (Giling et al. 2009; Johnston et al. 2011). These variable findings are consistent with a highly flexible diet in C. destructor.
Conclusions
Overall, this study has indicated that food resource competition between C. destructor and E. dharawalus is highly likely. The increase in the assimilation of detritus in the diet of E. dharawalus at the manipulation site after the removal of C. destructor may be an indication of competition over this resource in the presence of C. destructor. Additionally, on the basis of our isotopic enrichment, assimilated diet and trophic position comparisons between the species, it was evident that there was some degree of trophic and dietary overlap between C. destructor and E. dharawalus, although niche partitioning was also apparent. We also illustrated the concerning capacity of C. destructor to invade an ecosystem because of its ability to alter its trophic position on the basis of resources available. This contrasts with the fixed trophic position exhibited by E. dharawalus across sites, indicating that the trophic position of the native species is less flexible than that of the invasive. However, our findings surrounding the isotopic shift of E. dharawalus after a period of drought indicated that this species may be susceptible to changes in its trophic position and niche size in the face of climatic variation.
We conclude on the basis of these findings that dietary and trophic overlap between C. destructor and Euastacus species is a threat that should be taken into consideration when managing systems where Euastacus species are sympatric with the invasive C. destructor. Additionally, it is recommended that measures preventing the further proliferation of C. destructor throughout Australia’s freshwater systems should be taken. We also suggest that extensive control efforts of C. destructor within the range of E. dharawalus are prioritised as a management strategy in conserving this critically endangered crayfish.
Declaration of funding
This research was funded by Australia’s Nuclear Science and Technology Organisation (ANSTO) FutureNow Scholarship (received by Sarah O’Hea Miller) and supported by Local Land Services (South East) through funding from the Australian Government’s National Landcare Program.
Acknowledgements
We acknowledge and pay respect to the Dharawal people, the traditional custodians of the land on which this research was conducted. The authors thank the NSW Department of Primary Industries and Keegan Bowen for their assistance in the field. We also thank Narelle Hegarty for her assistance in the laboratory. Comments by the editor and two anonymous reviewers improved the clarity of this contribution.
References
Bašić T, Copp GH, Edmonds-Brown VR, Keskin E, Davison PI, Britton JR (2019) Trophic consequences of an invasive, small-bodied non-native fish, sunbleak Leucaspius delineatus, for native pond fishes. Biological Invasions 21, 261-275.
| Crossref | Google Scholar |
Bearhop S, Adams CE, Waldron S, Fuller RA, Macleod H (2004) Determining trophic niche width: a novel approach using stable isotope analysis. Journal of Animal Ecology 73, 1007-1012.
| Crossref | Google Scholar |
Beatty SJ (2006) The diet and trophic positions of translocated, sympatric populations of Cherax destructor and Cherax cainii in the Hutt River, Western Australia: evidence of resource overlap. Marine and Freshwater Research 57, 825-835.
| Crossref | Google Scholar |
Bergman DA, Moore PA (2003) Field observations of intraspecific agonistic behavior of two crayfish species, Orconectes rusticus and Orconectes virilis, in different habitats. The Biological Bulletin 205, 26-35.
| Crossref | Google Scholar | PubMed |
Carpenter SR, Stanley EH, Vander Zanden MJ (2011) State of the world’s freshwater ecosystems: physical, chemical, and biological changes. Annual Review of Environment and Resources 36, 75-99.
| Crossref | Google Scholar |
Cerato S, Davis AR, Coleman D, Wong MYL (2019) Reversal of competitive dominance between invasive and native freshwater crayfish species under near-future elevated water temperature. Aquatic Invasions 14, 518-530.
| Crossref | Google Scholar |
Cerveira LR, Ramos JA, Rodrigues I, Almeida N, Araújo PM, Santos I, Vieira C, Pereira JM, Ceia FR, Geraldes P, Melo T, Paiva VH (2020) Inter-annual changes in oceanic conditions drives spatial and trophic consistency of a tropical marine predator. Marine Environmental Research 162, 105165.
| Crossref | Google Scholar | PubMed |
Chase JM, Abrams PA, Grover JP, Diehl S, Chesson P, Holt RD, Richards SA, Nisbet RM, Case TJ (2002) The interaction between predation and competition: a review and synthesis. Ecology Letters 5, 302-315.
| Crossref | Google Scholar |
Coccia C, Fry B, Ramírez F, Boyero L, Bunn SE, Diz-Salgado C, Walton M, Le Vay L, Green AJ (2016) Niche partitioning between invasive and native corixids (Hemiptera, Corixidae) in south-west Spain. Aquatic Sciences 78, 779-791.
| Crossref | Google Scholar |
Coughran J, Daly G (2012) Potential threats posed by a translocated crayfish: the case of Cherax destructor in coastal drainages of New South Wales, Australia. Crustacean Research Special2012, 5-13.
| Crossref | Google Scholar |
Coughran J, McCormack R, Daly G (2009) Translocation of the Yabby Cherax destructor into eastern drainages of New South Wales, Australia. Australian Zoologist 35, 100-103.
| Crossref | Google Scholar |
DeNiro MJ, Epstein S (1978) Influence of diet on the distribution of carbon isotopes in animals. Geochimica et Cosmochimica Acta 42, 495-506.
| Crossref | Google Scholar |
Dobson M, Hildrew AG (1992) A test of resource limitation among shredding detritivores in low order streams in southern England. Journal of Animal Ecology 61, 69-77.
| Crossref | Google Scholar |
Dominguez Almela V, South J, Britton JR (2021) Predicting the competitive interactions and trophic niche consequences of a globally invasive fish with threatened native species. Journal of Animal Ecology 90, 2651-2662.
| Crossref | Google Scholar | PubMed |
Emery-Butcher HE, Beatty SJ, Robson BJ (2020) The impacts of invasive ecosystem engineers in freshwaters: a review. Freshwater Biology 65, 999-1015.
| Crossref | Google Scholar |
Ercoli F, Ruokonen TJ, Hämäläinen H, Jones RI (2014) Does the introduced signal crayfish occupy an equivalent trophic niche to the lost native noble crayfish in boreal lakes? Biological Invasions 16, 2025-2036.
| Crossref | Google Scholar |
Faragher RA (1983) Role of the crayfish Cherax destructor clark as food for trout in Lake Eucumbene, New South Wales. Marine and Freshwater Research 34, 407-417.
| Crossref | Google Scholar |
Feiner ZS, Rice JA, Bunch AJ, Aday DD (2013) Trophic niche and diet overlap between invasive white perch and resident white bass in a Southeastern Reservoir. Transactions of the American Fisheries Society 142, 912-919.
| Crossref | Google Scholar |
Furse JM, Coughran J (2011) An assessment of the distribution, biology, threatening processes and conservation status of the freshwater crayfish, genus Euastacus (Decapoda: Parastacidae), in continental Australia. III. Case studies and recommendations. New Frontiers in Crustacean Biology 15, 265-274 10.1163/ej.9789004174252.i-354.179.
| Google Scholar |
Geiger W, Alcorlo P, Baltanás A, Montes C (2005) Impact of an introduced Crustacean on the trophic webs of Mediterranean wetlands. Biological Invasions 7, 49-73.
| Crossref | Google Scholar |
Gherardi F, Acquistapace P, Santini G (2001) Foraging by a threatened species – the whiteclawed crayfish, Austropotamobius pallipes. Archiv für Hydrobiologie 152, 339-351.
| Crossref | Google Scholar |
Giling D, Reich P, Thompson RM (2009) Loss of riparian vegetation alters the ecosystem role of a freshwater crayfish (Cherax destructor) in an Australian intermittent lowland stream. Journal of the North American Benthological Society 28, 626-637.
| Crossref | Google Scholar |
Glon MG, Larson ER, Pangle KL (2016) Comparison of 13C and 15N discrimination factors and turnover rates between congeneric crayfish Orconectes rusticus and O. virilis (Decapoda, Cambaridae). Hydrobiologia 768, 51-61.
| Crossref | Google Scholar |
Hayes CL, Talbot WA, Wolf BO (2016) Abiotic limitation and the C3 hypothesis: isotopic evidence from Gunnison’s prairie dog during persistent drought. Ecosphere 7, e01626.
| Crossref | Google Scholar |
Hazlett BA, Lawler S, Geoffery E (2007) Agonistic behavior of the crayfish Euastacus armatus and Cherax destructor. Marine and Freshwater Behaviour and Physiology 40, 257-266.
| Crossref | Google Scholar |
Holway DA (1999) Competitive mechanisms underlying the displacement of native ants by the invasive Argentine ant. Ecology 80, 238-251.
| Crossref | Google Scholar |
Jackson AL, Inger R, Parnell AC, Bearhop S (2011) Comparing isotopic niche widths among and within communities: SIBER – stable isotope Bayesian ellipses in R. Journal of Animal Ecology 80, 595-602.
| Crossref | Google Scholar | PubMed |
Jackson MC, Jones T, Milligan M, Sheath D, Taylor J, Ellis A, England J, Grey J (2014) Niche differentiation among invasive crayfish and their impacts on ecosystem structure and functioning. Freshwater Biology 59, 1123-1135.
| Crossref | Google Scholar |
Jackson MC, Evangelista C, Zhao T, Lecerf A, Britton JR, Cucherousset J (2017) Between-lake variation in the trophic ecology of an invasive crayfish. Freshwater Biology 62, 1501-1510.
| Crossref | Google Scholar |
Johnston K, Robson BJ, Fairweather PG (2011) Trophic positions of omnivores are not always flexible: evidence from four species of freshwater crayfish. Austral Ecology 36, 269-279.
| Crossref | Google Scholar |
Juncos R, Milano D, Macchi PJ, Vigliano PH (2015) Niche segregation facilitates coexistence between native and introduced fishes in a deep Patagonian lake. Hydrobiologia 747, 53-67.
| Crossref | Google Scholar |
Kowalczyk ND, Reina RD, Preston TJ, Chiaradia A (2015) Environmental variability drives shifts in the foraging behaviour and reproductive success of an inshore seabird. Oecologia 178, 967-979.
| Crossref | Google Scholar |
Lang I, Paz-Vinas I, Cucherousset J, Loot G (2021) Patterns and determinants of phenotypic variability within two invasive crayfish species. Freshwater Biology 66, 1782-1798.
| Crossref | Google Scholar |
Larson ER, Twardochleb LA, Olden JD (2017) Comparison of trophic function between the globally invasive crayfishes Pacifastacus leniusculus and Procambarus clarkii. Limnology 18, 275-286.
| Crossref | Google Scholar |
Layman CA, Arrington DA, Montaña CG, Post DM (2007) Can stable isotope ratios provide for community-wide measures of trophic structure? Ecology 88, 42-48.
| Crossref | Google Scholar | PubMed |
Linton SM, Allardyce BJ, Hagen W, Wencke P, Saborowski R (2009) Food utilisation and digestive ability of aquatic and semi-terrestrial crayfishes, Cherax destructor and Engaeus sericatus (Astacidae, Parastacidae). Journal of Comparative Physiology – B. Biochemical Systems and Environmental Physiology 179, 493-507.
| Crossref | Google Scholar |
Linzmaier SM, Musseau C, Matern S, Jeschke JM (2020) Trophic ecology of invasive marbled and spiny-cheek crayfish populations. Biological Invasions 22, 3339-3356.
| Crossref | Google Scholar |
Magoulick DD, Piercey GL (2016) Trophic overlap between native and invasive stream crayfish. Hydrobiologia 766, 237-246.
| Crossref | Google Scholar |
Matthews MA, Reynolds JD, Keatinge MJ (1993) Macrophyte reduction and benthic community alteration by the crayfish Austropotamobius pallipes (Lereboullet). Freshwater Crayfish 9, 289-299.
| Google Scholar |
Mazumder D, Williams RJ, Reid D, Saintilan N, Szymczak R (2008) Variability of stable isotope ratios of glassfish (Ambassis jacksoniensis) from mangrove/saltmarsh environments in southeast Australia and implications for choosing sample size. Environmental Bioindicators 3, 114-123.
| Crossref | Google Scholar |
Mazumder D, Johansen M, Saintilan N, Iles J, Kobayashi T, Knowles L, Wen L (2012) Trophic shifts involving native and exotic fish during hydrologic recession in floodplain wetlands. Wetlands 32, 267-275.
| Crossref | Google Scholar |
Mazumder D, Saintilan N, Wen L, Kobayashi T, Rogers K (2017) Productivity influences trophic structure in a temporally forced aquatic ecosystem. Freshwater Biology 62, 1528-1538.
| Crossref | Google Scholar |
Mazumder D, Johansen MP, Fry B, Davis E (2018) Muscle and carapace tissue-diet isotope discrimination factors for the freshwater crayfish Cherax destructor. Marine and Freshwater Research 69, 56-65.
| Crossref | Google Scholar |
McCormack RB (2013) Conservation of imperiled crayfish, Euastacus dharawalus (Decapoda: Astacidea: Parastacidae), from the Southern Highlands of New South Wales, Australia. Journal of Crustacean Biology 33, 432-439.
| Crossref | Google Scholar |
McCormack RB (2016) Fitzroy Falls crayfish Euastacus dharawalus. In ‘The IUCN Red List of Threatened Species 2016’. e.T153704A188805771. (International Union for Conservation of Nature and Natural Resources) Available at https://www.iucnredlist.org/species/153704/188805771 [erratum published in 2020]
McIntosh AR, Greig HS, McMurtrie SA, Nystöm P, Winterbourn MJ (2005) Top-down and bottom-up influences on populations of a stream detritivore. Freshwater Biology 50, 1206-1218.
| Crossref | Google Scholar |
Modesto V, Dias E, Ilarri M, Lopes-Lima M, Teixeira A, Varandas S, Castro P, Antunes C, Sousa R (2021) Trophic niche overlap between native freshwater mussels (Order: Unionida) and the invasive Corbicula fluminea. Aquatic Conservation: Marine and Freshwater Ecosystems 31, 2058-2071.
| Crossref | Google Scholar |
Momot WT, Gowing H, Jones PD (1978) The dynamics of crayfish and their role in ecosystems. The American Midland Naturalist 99, 10-35.
| Crossref | Google Scholar |
Nyström P, Brönmark C, Granéli W (1996) Patterns in benthic food webs: a role for omnivorous crayfish? Freshwater Biology 36, 631-646.
| Crossref | Google Scholar |
Olsson K, Stenroth P, Nyström P, Granéli W (2009) Invasions and niche width: does niche width of an introduced crayfish differ from a native crayfish? Freshwater Biology 54, 1731-1740.
| Crossref | Google Scholar |
O’Hea Miller SB, Davis AR, Wong MYL (2022) Does habitat complexity and prior residency influence aggression between invasive and native freshwater crayfish? Ethology 128, 443-452.
| Crossref | Google Scholar |
O’Hea Miller SB, Davis AR, Wong MYL (2023) Further insights into invasion: field observations of behavioural interactions between an invasive and critically endangered freshwater crayfish using baited remote underwater video (BRUV). Biology 12, 18.
| Crossref | Google Scholar |
Pacioglu O, Zubrod JP, Schulz R, Jones JI, Pârvulescu L (2019) Two is better than one: combining gut content and stable isotope analyses to infer trophic interactions between native and invasive species. Hydrobiologia 839, 25-35.
| Crossref | Google Scholar |
Parkyn SM, Rabeni CF, Collier KJ (1997) Effects of crayfish (Paranephrops planifrons : Parastacidae) on in-stream processes and benthic faunas: a density manipulation experiment. New Zealand Journal of Marine and Freshwater Research 31, 685-692.
| Crossref | Google Scholar |
Parnell AC, Phillips DL, Bearhop S, Semmens BX, Ward EJ, Moore JW, Jackson AL, Grey J, Kelly DJ, Inger R (2013) Bayesian stable isotope mixing models. Environmetrics 24, 387-399.
| Crossref | Google Scholar |
Phillips DL (2012) Converting isotope values to diet composition: the use of mixing models. Journal of Mammalogy 93, 342-352.
| Crossref | Google Scholar |
Post DM (2002) Using stable isotopes to estimate trophic position: models, methods, and assumptions. Ecology 83, 703-718.
| Crossref | Google Scholar |
Post DM, Layman CA, Arrington DA, Takimoto G, Quattrochi J, Montaña CG (2007) Getting to the fat of the matter: models, methods and assumptions for dealing with lipids in stable isotope analyses. Oecologia 152, 179-189.
| Crossref | Google Scholar |
Reid DJ, Quinn GP, Lake PS, Reich P (2008) Terrestrial detritus supports the food webs in lowland intermittent streams of south-eastern Australia: a stable isotope study. Freshwater Biology 53, 2036-2050.
| Crossref | Google Scholar |
Renai B, Gherardi F (2004) Predatory efficiency of crayfish: comparison between indigenous and non-indigenous species. Biological Invasions 6, 89-99.
| Crossref | Google Scholar |
Reynolds J, Souty-Grosset C, Richardson A (2013) Ecological roles of crayfish in freshwater and terrestrial habitats. Freshwater Crayfish 19, 197-218.
| Google Scholar |
Riccialdelli L, Paso Viola MN, Panarello HO, Goodall RNP (2017) Evaluating the isotopic niche of beaked whales from the southwestern South Atlantic and Southern Oceans. Marine Ecology Progress Series 581, 183-198.
| Crossref | Google Scholar |
Rogosch JS, Olden JD (2020) Invaders induce coordinated isotopic niche shifts in native fish species. Canadian Journal of Fisheries and Aquatic Sciences 77, 1348-1358.
| Crossref | Google Scholar |
Sala OE, Stuart Chapin F, Armesto JJ, Berlow E, Bloomfield J, Dirzo R, Huber-Sanwald E, Huenneke LF, Jackson RB, Kinzig A, Leemans R, Lodge DM, Mooney HA, Oesterheld M, Poff NLR, Sykes MT, Walker BH, Walker M, Wall DH (2000) Global biodiversity scenarios for the year 2100. Science 287, 1770-1774.
| Crossref | Google Scholar | PubMed |
Sampson SJ, Chick JH, Pegg MA (2009) Diet overlap among two Asian carp and three native fishes in backwater lakes on the Illinois and Mississippi rivers. Biological Invasions 11, 483-496.
| Crossref | Google Scholar |
Scalici M, Gibertini G (2007) Feeding habits of the crayfish Austropotamobius pallipes (Decapoda, Astacidae) in a brook in Latium (central Italy). Italian Journal of Zoology 74, 157-168.
| Crossref | Google Scholar |
Schmid B (1984) Niche width and variation within and between populations in colonizing species (Carex flava group). Oecologia 63, 1-5.
| Crossref | Google Scholar | PubMed |
Smith GB, Tucker JM, Pauli JN (2022) Habitat and drought influence the diet of an unexpected mycophagist: fishers in the Sierra Nevada, California. Journal of Mammalogy 103, 328-338.
| Crossref | Google Scholar |
Stenroth P, Nyström P (2003) Exotic crayfish in a brown water stream: effects on juvenile trout, invertebrates and algae. Freshwater Biology 48, 466-475.
| Crossref | Google Scholar |
Stenroth P, Holmqvist N, Nyström P, Berglund O, Larsson P, Granéli W (2006) Stable isotopes as an indicator of diet in omnivorous crayfish (Pacifcastacus leniusculus): the influence of tissue, sample treatment, and season. Canadian Journal of Fisheries and Aquatic Sciences 63, 821-831.
| Crossref | Google Scholar |
Suchomel AD, Belk MC (2022) Comparison of trophic niche position, size, and overlap in an assemblage of Pacific Rockfish (Genus Sebastes) for testing community composition models. Diversity 14, 689.
| Crossref | Google Scholar |
Svanbäck R, Bolnick DI (2006) Intraspecific competition drives increased resource use diversity within a natural population. Proceedings of the Royal Society of London – B. Biological Sciences 274, 839-844.
| Crossref | Google Scholar |
Syväranta J, Lensu A, Marjomäki TJ, Oksanen S, Jones RI (2013) An empirical evaluation of the utility of convex hull and standard ellipse areas for assessing population niche widths from stable isotope data. PLoS ONE 8, e56094.
| Crossref | Google Scholar |
Thomson D (2004) Competitive interactions between the invasive European honey bee and native bumble bees. Ecology 85, 458-470.
| Crossref | Google Scholar |
Tran TNQ, Jackson MC, Sheath D, Verreycken H, Britton JR (2015) Patterns of trophic niche divergence between invasive and native fishes in wild communities are predictable from mesocosm studies. Journal of Animal Ecology 84, 1071-1080.
| Crossref | Google Scholar | PubMed |
Usio N, Townsend CR (2002) Functional significance of crayfish in stream food webs: roles of omnivory, substrate heterogeneity and sex. Oikos 98, 512-522.
| Crossref | Google Scholar |
Vanderklift MA, Ponsard S (2003) Sources of variation in consumer-diet δ15N enrichment: a meta-analysis. Oecologia 136, 169-182.
| Crossref | Google Scholar |
Vesely L, Ercoli F, Ruokonen TJ, Bláha M, Kubec J, Buřič M, Hämäläinen H, Kouba A (2020) The crayfish distribution, feeding plasticity, seasonal isotopic variation and trophic role across ontogeny and habitat in a canyon-shaped reservoir. Aquatic Ecology 54, 1169-1183.
| Crossref | Google Scholar |
Vollmer KL, Gall BG (2014) Complex predator–prey interactions between the rusty crayfish (Orconectes rusticus) and invertebrate and vertebrate prey within their native range. Journal of Freshwater Ecology 29, 267-277.
| Crossref | Google Scholar |
Weis JS (2010) The role of behavior in the success of invasive crustaceans. Marine and Freshwater Behaviour and Physiology 43, 83-98.
| Crossref | Google Scholar |
Wu J, Chen H, Jin B, Winemiller KO, Wu S, Xu W, Zhang H, Wu X (2022) Seasonal variation in resource overlap between red swamp crayfish (Procambarus clarkii) and native species in Poyang Lake Wetland, China. Frontiers in Environmental Science 10, 923962.
| Crossref | Google Scholar |
Yang D, Gonzalez-Bernal E, Greenlees M, Shine R (2012) Interactions between native and invasive gecko lizards in tropical Australia. Austral Ecology 37, 592-599.
| Crossref | Google Scholar |