Proof of concept for measuring growth of shelf marine calcifiers: ‘a Bryozoan odyssey’
Katerina Achilleos
A
Marine and Freshwater Research 74(14) 1262-1273 https://doi.org/10.1071/MF23114
Submitted: 6 April 2023 Accepted: 22 August 2023 Published: 8 September 2023
Abstract
The variation observed in growth rate estimates of bryozoans raise questions regarding the validity of the methods used to measure growth in these animals. Naturally, the best way for measuring growth rate is to understand the growth in situ, but access is not always straightforward.
This study assesses a field experiment for measuring in situ growth of heavily calcified bryozoans in the open ocean at 56 m, the deepest such deployment attempted for bryozoans.
Cellaria immersa colonies were collected by dredge from the continental shelf off Otago, marked using calcein, mounted on a purpose-built frame, called ‘Odyssey’, and returned to the shelf for 3 months in the Austral summer (November–February).
Data from 10 internodes indicated that growth was, on average, 0.97 ± 0.84 mm year−1 and showed some interesting intracolonial growth patterns.
The data obtained from this study are only indicative at this stage, but we have succeeded in developing a reproducible experimental set-up for in situ growth experiments of shelf bryozoans, enabling us to record growth, lifespan, and calcification rates of heavily calcified and ecologically important species. Understanding such key species is critical to identifying their role in the ecosystem and providing valuable information for future conservation initiatives.
Keywords: bryozoans, calcein, Cellaria, continental shelf, experimental set-up, growth rate, in situ, marine calcifiers, mark–recapture.
Introduction
Bryozoans are important components in reef formations globally, and many species can form three-dimensional structures that provide complex habitat for others, functioning as ecosystem engineers (Wood et al. 2012; Bastos et al. 2018). Many species in this phylum are important carbonate producers, especially in southern hemisphere shelf environments (Smith and Key 2004; Smith 2014). Climate change and anthropogenic activities such as bottom trawling have significantly affected these treasured species (Wood et al. 2012; Swezey et al. 2017a, 2017b; Bernal et al. 2020; Gissi et al. 2021; Mello et al. 2023). However, our lack of understanding of fundamental biological processes in these key species, such as growth rate, renders it difficult to predict the extent of these impacts and the speed or possibility of their recovery.
Regardless of the colonial form, growth in bryozoans can be expressed in the following three ways: (1) ontogeny, the development of an individual zooid either of the ancestrula or any other zooid in the colony; (2) astogeny, the addition of new zooids at the growing margin of the colony by asexual reproduction (budding); and (3) overall thickening by secondary calcification. Nevertheless, measuring growth in bryozoans becomes increasingly more complicated when moving from the short-lived uniserial encrusters to long-lived, erect, complex colonies (e.g. Smith and Key 2020, table 1).
Published growth-rate data are available for 72 bryozoan species, including 37 encrusting, 23 erect flexible and 12 erect rigid forms (Lonhart 2012; Hirose et al. 2020; Smith and Key 2020). Overall, uncalcified or lightly calcified species tend to have faster growth rates and shorter lifespan than do more heavily calcified species (Smith and Key 2020, and references therein). Large, heavily calcified species of considerable ecological interest are largely understudied (Smith and Key 2020), in part because of the technical difficulties of in situ work at depth. Heavily calcified bryozoans are notoriously difficult to maintain in aquaria, likely owing to our lack of knowledge of their ecological preferences (Smith et al. 2022).
Cellaria species form erect calcified arborescent colonies. The overall colony size varies among species, but they can grow up to several centimetres tall (McKinney and Jaklin 2000, 2001; Bader and Schäfer 2005). Cellaria sensu stricto is generally considered to comprise mostly dichotomously branching species, but there are a few exceptions (Achilleos et al. 2020). The branches are cylindrical and are formed by internodes separated by organic joints (i.e. nodes), which gives the colony a certain degree of flexibility. The organic joints are made of rhizoids, which also develop at the proximal part of the colony for attaching and stabilising the colony on the substratum in fimbriate projections (Schack et al. 2018) (Fig. 1). The internodes grow distally by budding, followed by deposition of primary calcification mainly around the newly developed zooids. A secondary calcification layer is also added around the older zooids but at a much slower rate (e.g. Achilleos et al. 2020).
Left: diagrammatic illustration of Cellaria species settlement and astogeny (not to scale). Right: C. immersa from the Otago shelf, New Zealand, at 90-m depth. Scale bar: 6 mm. A, non-feeding ciliated larva; B, ancestrula; C, first internode of the colony; D–F, growth of the colony by forming the sister internodes; n, node; r, rhizoid.
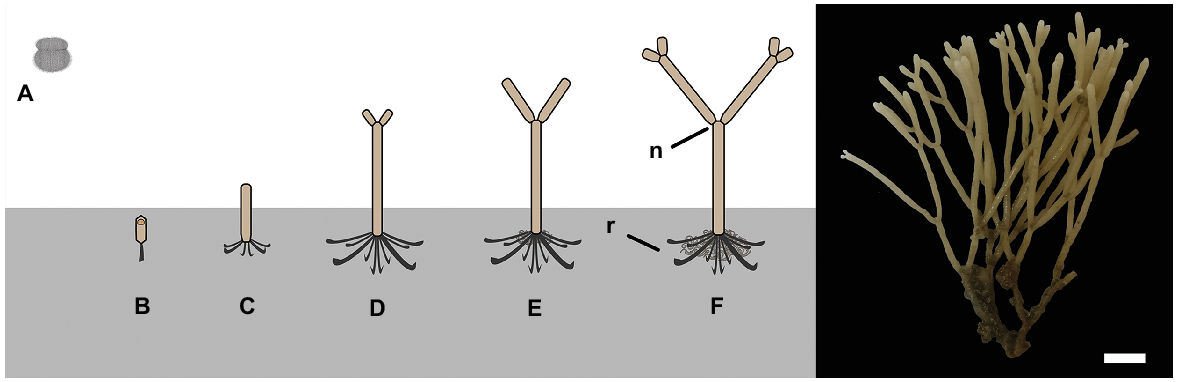
Here, we present a method for mark-and-recapture growth study of shelf sessile calcifiers, using as our example the erect bryozoan species Cellaria immersa, a suitable subject because of its simple shape and growth direction. C. immersa is one of the main habitat-forming species in the shelf environment around New Zealand, and it is found in important multispecies community assemblages, the bryozoan thickets (Wood et al. 2013; Achilleos et al. 2019). Other Cellaria species are also known to form structural biotopes and multispecies assemblages, with the most prominent example being the ‘Cellaria meadows’ found in the Adriatic (McKinney and Jaklin 2000, 2001; Bader 2001). Identifying the growth rate of ecosystem engineer species is critical in providing foundational understanding of their role in the ecosystem and will provide essential information for future conservation initiatives.
Materials and methods
To conduct an in situ growth experiment on the mid-shelf, we used a mark–recapture method where an adult bryozoan colony is marked, replaced in its natural environment for a period of time to grow, and re-captured to measure growth.
Target species and site collection
C. immersa colonies were collected at sea off Otago (170.79°E, 45.84°S) on 10 November 2018, by using a small dredge at 56-m depth, deployed by RV Polaris II. The site location was decided after consideration of both the known distribution of C. immersa and low commercial fishing activity in this region as delineated by the Ministry of Primary Industries (MPI, https://www.mpi.govt.nz/legal/legislation-standards-and-reviews/fisheries-legislation/maps-of-nz-fisheries/), thus reducing the possibility of overlap between the deployment location and known fishing activity.
After collection, 21 large living colonies were chosen for the growth experiment. The health state of the chosen colonies was determined by the eversion of lophophores when immersed underwater, and the completeness (that is, they were not broken) of the colonies.
Experimental design
A robust platform was designed and built to withstand open ocean conditions for the period of deployment. The structure was custom-made for the purpose of the experiment and nicknamed ‘Odyssey’ (Fig. 2). A steel platform (~1 m in diameter, ~90 kg) was used for weighting and supporting of the experimental rack, and stainless-steel pipes in the shape of a metal pyramid (about 1 m in height) were welded to the platform. The pyramid structure allowed for specimens to be attached above the sediment when in situ and provided an attachment point for a marker buoy. A removable steel sheet (50 cm × 50 cm) was used to secure colonies to the experimental set up while underwater, thus reducing the stress of handling on the colonies. Metal crossbars were used to support the upright position of the colonies, resembling their natural orientation in the environment.
Calcein acetoxymethyl (C0875 Sigma-Aldrich) was used to stain the colonies to track the new skeletal growth, because the successful marking of C. immersa colonies using calcein has been demonstrated before by Smith et al. (2019). Twenty large living C. immersa colonies were immersed in seawater with calcein at a concentration of 300 mg L−1 on board for 6 h (Fig. 3a). The water temperature was kept constant at 11°C while staining, the temperature being recorded by CTD (SBE 19) at the time of collection. One colony was left unstained to be used as the negative control in downstream data analysis.
Collection of specimens, and deployment of Odyssey. (a) C. immersa colonies immersed in seawater with calcein; (b) C. immersa colony with epoxy putty and a cable tie for fastening on ‘Odyssey’; (c, d) C. immersa colonies secured on ‘Odyssey’ prior to deployment; (e) a 3D model showing ‘Odyssey’ deployment.
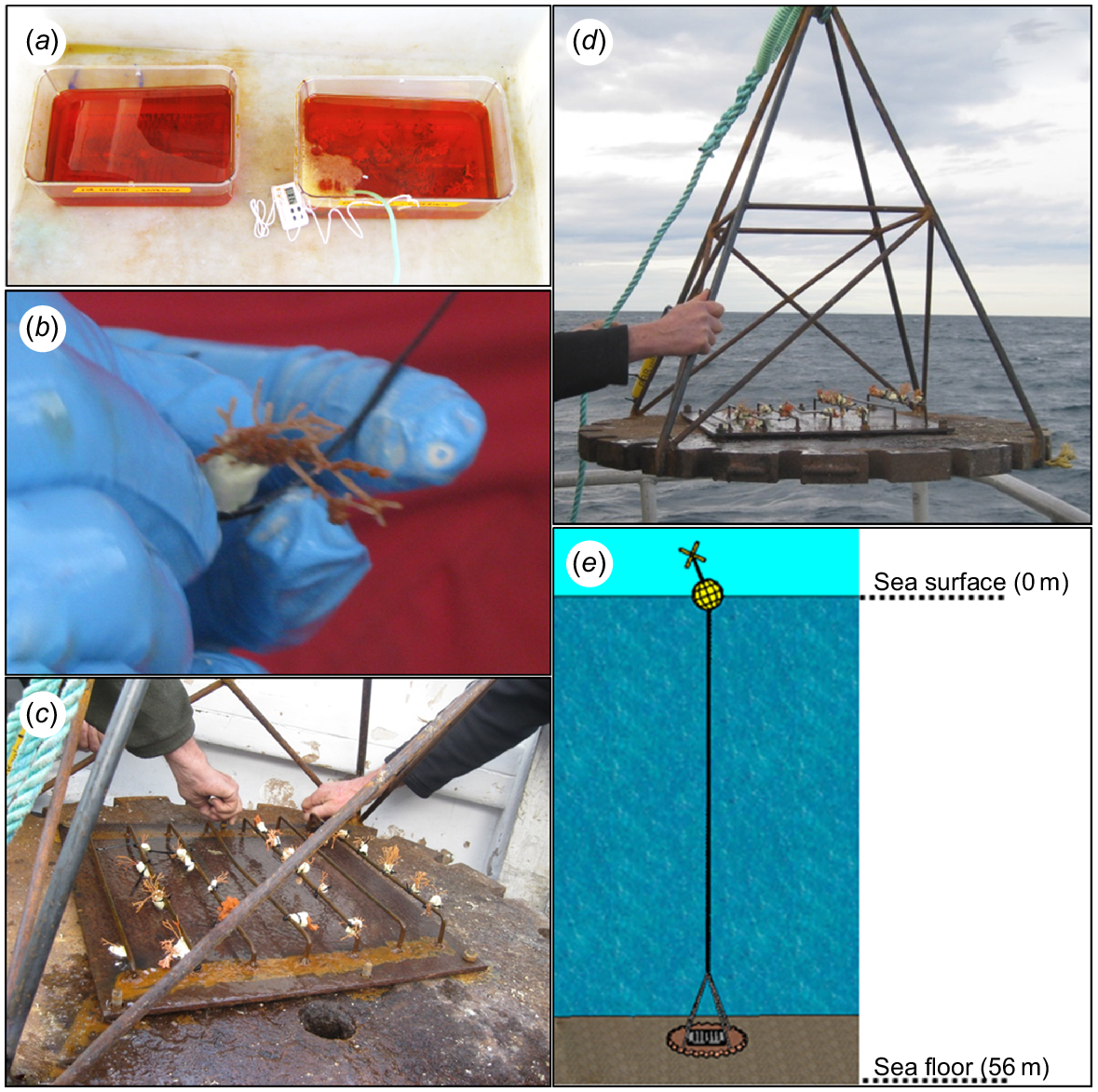
While staining the colonies, a non-toxic epoxy putty (Milliput Standard), widely used for in situ experiments (e.g. Suggett et al. 2013; Camp et al. 2015; Laverick et al. 2019), was moulded around the base of each colony. Cable ties were also moulded in the epoxy to ensure easy and quick attachment and removal of thecolonies from the crossbars (Fig. 3b). After marking was complete, the colonies were secured upright on the metal crossbars while immersed underwater. Prior to deployment the metal sheet with the crossbars was fastened tightly to the metal platform, and a temperature logger (RBRsolo3 T) was secured to it for recording water temperature and salinity (Fig. 3c, d).
Once complete, ‘Odyssey’ was deployed in November 2018 at 56-m depth at the same location from which the colonies were collected (Fig. 3e).
‘Odyssey’ recapture and data analysis
After 3 months (on 15 February 2019), the marker buoy was relocated on the basis of the deployment coordinates. ‘Odyssey’ was retrieved by securing the rope and slowly lifting with a crane. The re-collected C. immersa colonies were transferred underwater to the Portobello Marine Laboratory and were examined under a light microscope to observe whether they were still alive with reacting lophophores. The living colonies were then used for the growth-data analysis.
Calcein acetoxymethyl is a cell-permeant dye that binds to calcium, and it can be incorporated into the growing skeleton, enabling visualisation of any changes regarding skeletal formation by producing a green, fluorescent product (e.g. Tambutté et al. 2012). Therefore, the distal internodes of the living colonies were inspected using a fluorescence microscope (Olympus BX51) under 495-nm excitation frequencies to observe the calcein markings.
The 3-month growth was calculated on the basis of both the length and the volume of the part of the internode that extended distally from the calcein markings. The new growth was then correlated to the overall length and volume of the corresponding internode, to investigate whether growth varies with the age/size of the internode. The volume of the internodes was calculated as shown in Fig. 4, by adding up the volume of the cylinders and the volume of a hemisphere. Measurements were made from the microscopically obtained digital images by using the image software Fiji (ImageJ, ver. 1.52i, https://github.com/fiji; Schindelin et al. 2012).
Seawater environmental data were also retrieved from the temperature logger attached to the ‘Odyssey’ platform. Environmental data (available from Achilleos et al. (2019), including station SD 11 in Supplementary material 2; 170.802°E, 45.841°S), such as satellite-derived sea-surface chlorophyll-a concentration, seafloor temperature, and sediment composition, were also taken into consideration to characterise the location site. This station was chosen because it is adjacent to the deployment site used for the growth experiment.
Results
Five of the colonies were broken off the epoxy; so, 15 colonies were retrieved from Odyssey. Of the 15 colonies, two were alive with reacting lophophores when inspected under the microscope. The rest of the colonies were severely fragmented, with the distal internodes missing, and were therefore excluded from any further analysis. Strong hydrodynamic activity reported a few days prior to collection may have resulted in fragmentation of most of the colonies.
The first colony included 19 internodes in total, nine of which represented the distal growing internodes. The second colony was very young, represented only by its first internode (see C in Fig 1). Both colonies, 10 growing internodes in total, were inspected using a fluorescence microscope to observe the calcein markings (Fig. 5). We compared the fluorescent signal between the 10 internodes and the negative control (Fig. 5i) and confirmed the incorporation of calcein in the skeleton of all 10 internodes prior to the growth analysis.
‘Odyssey’ and specimens that were recaptured. (a) ‘Odyssey’ collection. (b, c) Part of colony one alive and with extended lophophores. Scale bar: 1 mm. (d–f) Arrow showing calcein markings on internodes of Colony 1. Scale bar: 0.5 mm. (g) Colony 2 alive with protruding lophophores. Scale bar: 0.5 mm. (h) Calcein marking on the growing margin of colony two. Scale bar: 0.5 mm. (i) Negative control with no calcein marking. Scale bar: 1 mm.
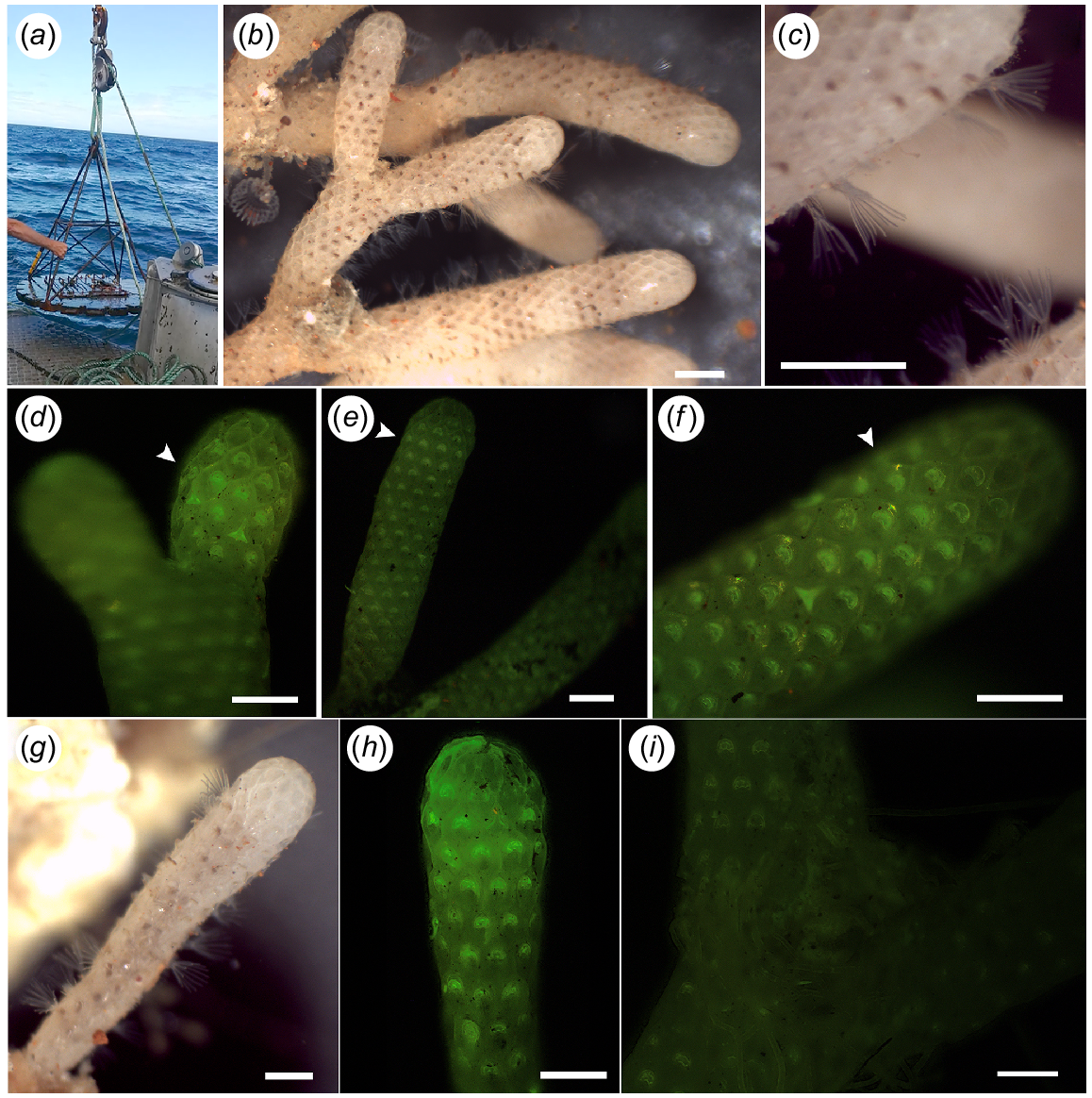
The nine internodes from the first colony varied considerably in their total size, ranging from 1.25 mm to 7.25 mm in length and from 0.6 to 8.81 mm3. Similarly, the total growth during the 3 months ranged from 0.34 mm (0.16 mm3) to 1.12 mm (0.90 mm3) (Table 1). On the basis of these results, the average growth for the first colony is estimated to be 0.97 ± 0.84 mm year−1 (1.83 ± 0.89 mm3 year−1). The second colony did not show any growth during the 3 months, even though calcein marking was observed at the growth margin of the colony, indicating skeletal deposition at the time of collection (Table 1, Fig. 5h). New growth was correlated with the total size of the corresponding internode, and the results suggest that the internodes do not grow continuously at the same rate, but that the growth rate increases with an increasing internode volume (and presumably age) (Table 1, Fig. 6).
Colony | Internode | Total internode (mm) | Total internode (mm3) | Total growth (mm) | Total growth (mm3) | Growth rate (mm month−1) | Growth rate (mm3 month−1) | |
---|---|---|---|---|---|---|---|---|
1 | 1 | 7.251 | 8.813 | 0.900 | 0.560 | 0.300 | 0.187 | |
1 | 2 | 1.618 | 0.861 | 0.738 | 0.365 | 0.246 | 0.122 | |
1 | 3 | 1.250 | 0.679 | 0.822 | 0.415 | 0.274 | 0.138 | |
1 | 4 | 7.471 | 8.634 | 1.124 | 0.900 | 0.375 | 0.300 | |
1 | 5 | 1.957 | 1.053 | 0.495 | 0.273 | 0.165 | 0.091 | |
1 | 6 | 5.606 | 3.403 | 0.900 | 0.850 | 0.300 | 0.283 | |
1 | 7 | 4.498 | 5.320 | 1.068 | 1.00 | 0.356 | 0.197 | |
1 | 8 | 2.815 | 1.971 | 0.340 | 0.165 | 0.113 | 0.055 | |
1 | 9 | 3.611 | 2.921 | 0.684 | 0.36 | 0.228 | 0.120 | |
2 | 1 | 4.036 | 1.534 | 0 | 0 | 0 | 0 |
Cellaria immersa estimated growth rate (mm3 month−1) in logarithmic scale for nine internodes from Colony 1.
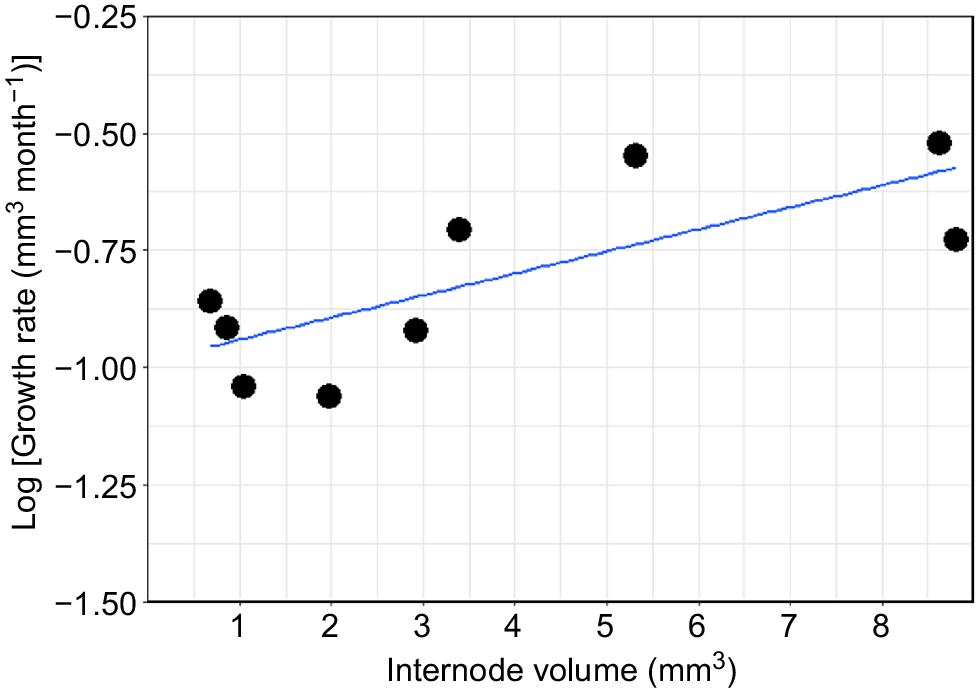
According to the data retrieved from the temperature logger, seafloor temperature ranged from 9.9°C in November 2018 (late spring) to 15.9°C in January 2019 (mid-summer) (Fig. 7). The average temperature during the 3 months was 12.9°C. Most of the variation was observed during November and December (transition from spring into summer), whereas the temperature was mostly settled between 14 and 15°C in January and February. The salinity recorded was at 35 PSU throughout the test period.
Discussion
This is the first study reporting the growth rate of the common New Zealand shelf bryozoan C. immersa, and the deepest in situ transplantation attempted for bryozoans by using calcein staining. Similar studies have been conducted in situ for cold-water corals, indicating that calcein works particularly well for growth studies, especially compared with other staining methods such as alizarin red (Lartaud et al. 2013, 2017). Calcein is also well incorporated by bryozoans, as shown by other studies. Smith et al. (2001) performed the only other in situ experiment by using calcein staining in New Zealand, to measure growth rate for Adeonellopsis colonies at 12–15 m depth. About half of the branches were successfully marked, indicating that either the branches were not depositing calcium at the time or that the calcein concentration used was not high enough to be incorporated in all the branches. Later, calcein marking was also used successfully to measure the growth of heavily calcified erect bryozoans grown under laboratory conditions (Smith et al. 2019).
Experimental design
Despite the rough sea conditions, ‘Odyssey’ performed well and did not drift away from the site location, but some modifications and improvements could be made to the equipment. For example, the addition of an extra protection layer around the ‘metal sheet’ (Fig. 2) could protect colonies from boulders and sediment from being dislodged by high hydrodynamic activity. This extra protective layer was not added initially to avoid disrupting the current flow, especially taking into consideration the suggested positive relationship of Cellaria species with high current activity (see Achilleos et al. 2019). Nevertheless, a wide grid may be able to balance these two parameters for best results.
Calcein concentration used may vary depending on the organism, but it often ranges between 50 and 150 mg L−1 (Smith et al. 2019, 2022). The shorter the immersion time is, the higher is the concentration needed for successful marking (Lartaud et al. 2013, 2017). Therefore, a fairly high concentration of calcein was used here (300 mg L−1) to ensure the marking of the colonies within the timeframe, as there was no fluorescent microscope on board the RV Polaris II to confirm the incorporation of calcein into the skeleton prior to deployment. Nevertheless, confirming the incorporation of calcein into the skeleton prior to deployment is crucial, and the absence of equipment such as a fluorescent microscope on board renders this a challenge. The use of hand-held equipment such as the SE-Mark® fluorescent detector or the Nightsea Bluestar® fluorescent detector combined with the filter glasses may help overcome such challenges during offshore field experiments (see Spires and North 2022).
Overall, calcein does not affect the precipitation of calcite, but high calcein concentrations can affect calcite crystal formation (Magnabosco et al. 2018), thus using lower calcein concentrations for longer when possible is to be preferred. Different concentration of calcein may need to be tested prior to starting an offshore in situ growth experiment if a staining technique has not been used for the targeted species before.
The epoxy used here successfully secured the colonies on ‘Odyssey’, and it was present at the time of collection even when the colonies were completely fragmented. According to the manufacturer’s direction, the epoxy can set in 3–4 h at room temperature. Setting time may take longer if the water is colder, as in this case study. Therefore, it is recommended to apply the epoxy as early as possible to ensure hardening before deployment.
Growth rate and environment
This is the first indication of C. immersa growth rate in situ, at ~1 mm year−1, which is comparable to that of other bryozoans (Fig. 8). If that is so, C. immersa colonies collected from the shelf up to 40 cm in length ( K. Achilleos, pers. obs.) may be as much as 40 years old. Although 3 months represent only a snapshot of the colony, the growth rate of C. immersa during spring and summer is much slower than that of other cellariids. Cellaria incula in the Antarctic can grow 8 mm year−1 for up to 14 years (Brey et al. 1999), whereas temperate Cellaria sinuosa can grow up to 40 mm year−1, but lives only ~2 years (Bader and Schäfer 2005). Melicerita chathamensis, another cellariid from the southern shelf of New Zealand, grows 14 mm year−1 for up to 9 years (Smith and Lawton 2010; Key et al. 2018; Smith and Key 2020). There is a high degree of variation even within the same genus, and it is therefore uncertain whether these differences are species-specific, environment-related, method-related, or due to insufficient data in the case of C. immersa.
Figure adapted from Smith and Key (2020, their appendix table), indicating the summary of growth- and calcification-rate measurements of bryozoan colonies, with newly added C. immersa shown with a red arrow, C. attenuata (Hirose et al. 2020) and W. subtorquata (Lonhart 2012). Different colours represent the different methods used to measure growth. Substrate seeded (in situ, blue); mark–recapture (in situ, green); chemical proxies (grey); mark–recapture (aquarium, light blue); annual growth checks (pink); settling plates (in situ, red); substrate seeded (aquarium, yellow).
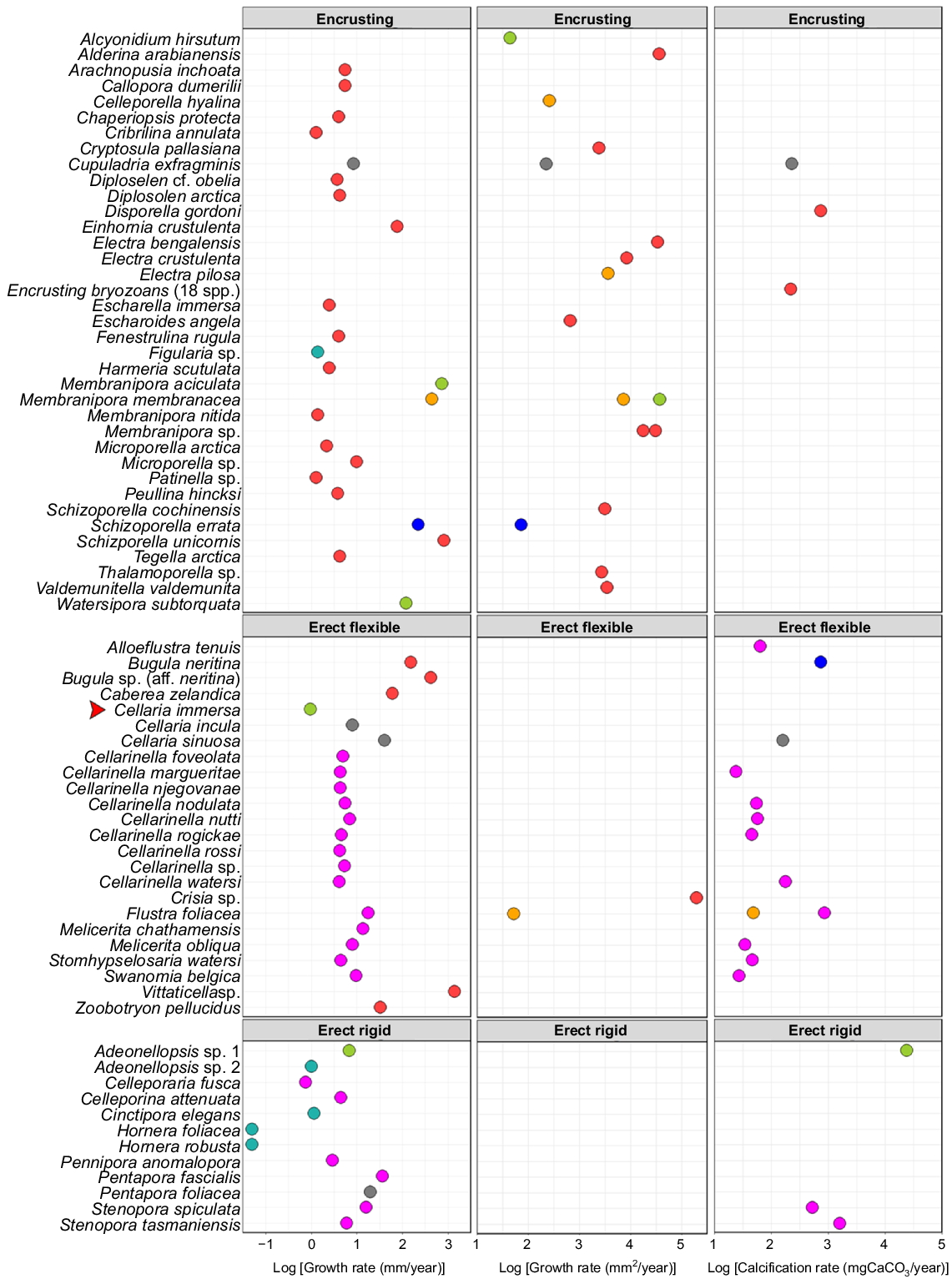
Growth may also vary depending on abiotic parameters and food concentration. Celleporella hyalina, for example, ceases feeding activity when the temperature drops significantly and resumes only when back to the initial temperature (Riisgård and Manríquez 1997). C. sinuosa from the English Channel shows slowed growth during wintertime (9°C−11°C) (Bader and Schäfer 2005). However, the effects of temperature could differ when combined with different food sources (Amui-Vedel et al. 2007). Pentapora fascialis shows longer zooids during wintertime, whereas the opposite pattern was observed for Cryptosula pallasiana (Lombardi et al. 2006; Amui-Vedel et al. 2007). Interestingly, C. pallasiana from the same location showed longer zooids when cultured in lower temperature and fed with Rhodomonas sp. (Amui-Vedel et al. 2007). In certain cases, the effects of the temperature on growth rate are indirect through affecting phytoplankton productivity, which in turn directly affects the growth rate (Barnes and Clarke 1998; Barnes et al. 2006).
According to the data collected herein, the seafloor temperature at the location site between November and February ranges from 9.9 to 15.9°C, with an average temperature of 12.9°C. We did not collect the temperature for the rest of the year; however, on the basis of the literature, the annual mean seafloor temperature is 8.3°C (Achilleos et al. 2019). The most recently reported satellite-derived sea-surface chlorophyll-a value for the location site is 1.17 mg m−3, which is well within the range reported for the known distribution of C. immersa (Achilleos et al. 2019, fig. 3). The mean value for sea-surface temperature reported for the deployment site is 12°C, which falls within the lower end of the surface temperature range documented for this species (Achilleos et al. 2019, fig. 3). The sediment at the site is characterised by higher percentage of sand and bioclastic sediments, which is a common substrate for this species (Achilleos et al. 2019). Further, species in this genus are often associated with intermediate to high-energy environments, up to 2 m s−1 (Wood et al. 2013; Achilleos et al. 2019). However, it is difficult to correlate C. immersa growth rate with environmental data at this point, because we do not have data among seasons for comparisons. It is likely that the growth rate does vary among seasons or even geographic locations, if the environmental parameters differ significantly from the ones reported here.
Our lack of understanding of the exact relationship between the environment and the growth rate in these species makes it difficult to predict the extent of environmental influences, such as climate change. Ocean acidification combined with temperature elevation shows interactive effects on marine calcifiers (e.g. Li et al. 2015). For example, Myriapora truncata showed resilience at a lower pH for a few weeks, but calcification stopped when combined with high temperature (Rodolfo-Metalpa et al. 2010; Lombardi et al. 2011). In contrast, Celleporella cornuta grew faster under high CO2, but produced lighter skeletons with lower skeletal magnesium that were therefore less soluble (Swezey et al. 2017b). It is evident that both acidification and rising temperatures affect the biomineralisation process (i.e. growth) in bryozoans. Therefore, measuring temperature and potentially other parameters while the in situ experiment is on-going is critical in elucidating the potential impact of environmental influences.
Energy distribution within an internode
The annual growth reported here for C. immersa requires the assumption that the colony grows continuously in length at the same rate throughout the year. This notion is unlikely to be true, because at times the colony may have to prioritise other important biological processes (Batson et al. 2020; Smith and Key 2020), such that younger internodes have lower growth rates.
Newly formed internodes are likely to split their energy into primary calcification (i.e. calcium carbonate deposition distally), secondary calcification to strengthen the foundations of the new internode, skeletal resorption for the formation of the node and the formation and growth of the rhizooids (Batson et al. 2020). Schafer et al. (2006) stated that in the early stages of internode formation in C. sinuosa, the cryptocyst and interior walls are fully calcified, whereas the first zooid row on the new branch tends to be almost twice as long as the rest of the autozooids. The same pattern was also observed in C. immersa (K. Achilleos, pers. obs.). While the new internode is growing, rhizooids are formed connecting the two branches that are later separated by skeletal resorption forming the internodes (Schafer et al. 2006; Batson et al. 2020). On the same note, newly formed colonies such as Colony 2 may temporarily pause their distal growth for periods of time, while the organism is putting energy into growing outwards by depositing secondary calcification and growing their rhizoids for securing the foundations of the colony. Horizontal cross-sections of the internodes may show the deposition of secondary calcification through calcein marking (e.g. Mahé et al. 2010), and would be a fruitful area of future study. Further, histological sections or micro-CT imaging of internode growth series would likely indicate the process of skeletal resorption and rhizooid formation.
Another interesting observation was that sister internodes do not necessarily reach the same size. This pattern was also reported by Brey et al. (1999) for C. incula where the sister internodes grew during the same interval but at different rates. Similarly, Internodes 4 and 5 (Table 1) are sister internodes but do not have the same size or growth rate. In fact, Internode 4 was ~7.5 mm long, but Internode 5 was only ~2 mm long. This difference was also reflected in their growth rate, 0.375 and 0.165 mm month−1 respectively. It is unclear why these differences are observed between sister internodes, but the split of energy in one of the internodes and the temporary ‘sacrifice’ of the other could be a sign of insufficient nutrient supply.
It is as yet unknown how nutrient exchange occurs between the internodes, or if any nutrient exchange occurs at all. If not, each internode grows independently. How would a Cellaria colony respond to damage? Another temperate bryozoan, Watersipora subtorquata, grew slower after fragmentation occurred (Hart and Keough 2009). Therefore, if nutrient exchange occurs between the internodes, fragmentation is likely to also affect the growth in C. immersa as the nutrient supply direction would be prioritised towards repairing the injury (i.e. fragmentation). If such exchange does not occur between the internodes, then fragmentation is unlikely to affect the growth of the colony other than the specific internode where fragmentation may have happened.
Reproduction may also affect the growth rate of the sister internodes. Ovicells (i.e. brooding chambers) in Cellaria species are endozooidal and are potentially formed by the immersion of the brood cavity into the colony through skeletal resorption when the internode is mature (Ostrovsky 2013a; Batson et al. 2020). Ovicells are not present in the newly formed internodes or zooids, but they are present on the proximal parts of actively growing internodes (K. Achilleos, pers. obs.). Embryo development is ensured by a cell complex, a placental analogue, transporting nutrients between the embryo and the maternal zooid (Ostrovsky 2013a, 2013b). Therefore, older internodes in the colony may have different energy and nutrient requirements, depending on the reproduction stages.
Methods for measuring growth rate in bryozoans
Bryozoan colony lifespan ranges from a few months to several years (Hirose et al. 2020; Key 2020; Smith and Key 2020, and references therein). The following four popular methods are used for measuring growth rate of bryozoans: (a) direct observation (in situ or aquarium), (b) mark and recapture (in situ or aquarium), (c) growth check lines and (d) chemical proxies (e.g. isotope analysis). Published information on the growth rate of bryozoans is largely based on methods a and c. Direct observation by conducting short-term experiments using settling-plates in situ, although popular, inevitably measures mostly fast-growing opportunistic encrusting species, the first to settle during such experiments. The second most widely used method includes the identification of annual growth checks, which has mostly been used for erect species (Bader and Schäfer 2004; Smith 2007; Hirose et al. 2020; Smith and Key 2020).
Mark and recapture (method b) is the best option for understanding the true growth rate of bryozoans, because growth can be measured accurately in their natural environment. However, field experiments present one major challenge, i.e. relocating the experimental set up, especially when it is left in the open ocean. Therefore, in situ growth experiments have higher possibilities of success when they are performed short term, such as in this study. This is a problem especially when the species of interest is slow-growing, so that a short-term experiment can provide only a snapshot of the growth. Such challenge can be overcome by repeating the same short-term (e.g. 3 months) experiment until a desirable time period is met.
Long-lived species hold environmental information within their skeletons that can be traced by following colony development backwards (Pätzold et al. 1987; Key et al. 2018). The combination of a long colony lifespan with distinctive morphological characteristics and a rich fossil record often kept in museum collections are some of the key features that can be used for paleoenvironmental analysis (Smith 1995, 2007; Lukasik et al. 2000) or even for analysing archaeological artefacts (Key et al. 2014; Achilleos et al. 2022). However, such paleoenvironmental analyses rely heavily on a deep understanding of the living species. The development of a relationship between in situ methods and others such as chemical proxies, growth check analyses and even morphometric analyses will be useful in translating such long-term environmental information (e.g. Smith et al. 2001; Smith and Key 2004). Ideally, such a relationship should be established while the in situ growth experiment is on-going. Specimens can be collected from the same location site to be used for isotopic and growth-check analyses, which can be matched back to the growth observed by calcein marking. At the same time, morphometric analyses can be performed through imaging by scanning electron microscopy.
Conclusions
Field experiments for studying growth in situ are not a novel idea but there is limited information regarding the growth of shelf fauna such as bryozoans, owing to the difficulties associated with such experiments (see Smith and Key 2020, and references therein). Whereas we have discussed it in the context of bryozoan growth rates, this design can also be applied for other sessile invertebrates with difficulty growing in aquariums. Mid- to outer-shelf depths are notoriously hard to study and sample and ‘Odyssey’ provides a window into these challenging marine environments.
Essentially, when breaking down such an experiment, it must consist of the following 10 key components:
Appropriate location for deployment based on the known distribution of the species and fishing activity or other potential disturbances.
Instruments such as a GPS logger and a temperature logger or similar.
A robust and heavy platform, to ensure that the experimental gear will remain at the chosen location during deployment.
Attachment point for enabling deployment and securement of the buoy and any other instruments.
A protective layer such as a mesh, to protect the specimens from exogenous factors (e.g. boulders). Ideally, this layer could be removable to easily reach the specimens post-deployment and collection. The openings of the mesh should be wide enough to not disrupt the current flow but still provide adequate protection.
Removable elements (e.g. metal sheet) for handling the specimens underwater.
A rope and buoy.
Practical materials (e.g. epoxy and cable ties) for fast attachment and removal of the specimens from the set-up.
A cell-permeant dye (e.g. calcein) to label the skeletal structures, especially for complex branching colony forms.
Replication of the entire experimental design.
Declaration of funding
The work was supported by the Doctoral Scholarship, University of Otago, to K. Achilleos, and research funding from the Department of Marine Science, University of Otago. Health Science Career Development postdoc, University of Otago (while preparing this paper for publication).
Acknowledgements
We thank the technical staff at the Portobello Marine Laboratory (University of Otago) and especially Dave Wilson for building the frame ‘Odyssey’. We also thank Bill Dickson and Evan Kenton for their help on board RV Polaris II, for the deployment and collection of ‘Odyssey’. We extend our thanks to Professor Chris Brown (University of Otago, Department of Biochemistry) for his help on board RV Polaris II during ‘Odyssey’ deployment. We are also grateful for the comments of two anonymous reviewers that improved the paper.
References
Achilleos K, Smith AM, Gordon DP (2019) The articulated bryozoan genus Cellaria in the southern Zealandian region: distribution and associated fauna. Marine Biodiversity 49, 2801-2812.
| Crossref | Google Scholar |
Achilleos K, Gordon DP, Smith AM (2020) Cellaria (Bryozoa, Cheilostomata) from the deep: new species from the southern Zealandian region. Zootaxa 4801(2), 201-236.
| Crossref | Google Scholar |
Amui-Vedel A-M, Hayward PJ, Porter JS (2007) Zooid size and growth rate of the bryozoan Cryptosula pallasiana Moll in relation to temperature, in culture and in its natural environment. Journal of Experimental Marine Biology and Ecology 353(1), 1-12.
| Crossref | Google Scholar |
Bader B (2001) Modern bryomol-sediments in a cool-water, high-energy setting: the inner shelf off Northern Brittany. Facies 44(1), 81-103.
| Crossref | Google Scholar |
Bader B, Schäfer P (2004) Skeletal morphogenesis and growth check lines in the Antarctic bryozoan Melicerita obliqua. Journal of Natural History 38(22), 2901-2922.
| Crossref | Google Scholar |
Bader B, Schäfer P (2005) Impact of environmental seasonality on stable isotope composition of skeletons of the temperate bryozoan Cellaria sinuosa. Palaeogeography, Palaeoclimatology, Palaeoecology 226(1–2), 58-71.
| Crossref | Google Scholar |
Barnes DKA, Clarke A (1998) Seasonality of polypide recycling and sexual reproduction in some erect Antarctic bryozoans. Marine Biology 131(4), 647-658.
| Crossref | Google Scholar |
Barnes DKA, Webb K, Linse K (2006) Slow growth of Antarctic bryozoans increases over 20 years and is anomalously high in 2003. Marine Ecology Progress Series 314, 187-195.
| Crossref | Google Scholar |
Bastos AC, Moura RL, Moraes FC, Vieira LS, Braga JC, Ramalho LV, Amado-Filho GM, Magdalena UR, Webster JM (2018) Bryozoans are major modern builders of south Atlantic oddly shaped reefs. Scientific Reports 8(1), 9638.
| Crossref | Google Scholar | PubMed |
Batson PB, Tamberg Y, Taylor PD, Gordon DP, Smith AM (2020) Skeletal resorption in bryozoans: occurrence, function and recognition. Biological Reviews 95(5), 1341-1371.
| Crossref | Google Scholar | PubMed |
Bernal MA, Schunter C, Lehmann R, Lightfoot DJ, Allan BJM, Veilleux HD, Rummer JL, Munday PL, Ravasi T (2020) Species-specific molecular responses of wild coral reef fishes during a marine heatwave. Science Advances 6(12), eaay3423.
| Crossref | Google Scholar |
Brey T, Gerdes D, Gutt J, Mackensen A, Starmans A (1999) Growth and age of the Antarctic bryozoan Cellaria incula on the Weddell Sea shelf. Antarctic Science 11(4), 408-414.
| Crossref | Google Scholar |
Camp EF, Krause S-L, Santos LMF, Naumann MS, Kikuchi RKP, Smith DJ, Wild C, Suggett DJ (2015) The “Flexi-Chamber”: a novel cost-effective in situ respirometry chamber for coral physiological measurements. PLoS ONE 10(10), e0138800.
| Crossref | Google Scholar | PubMed |
Gissi E, Manea E, Mazaris AD, Fraschetti S, Almpanidou V, Bevilacqua S, Coll M, Guarnieri G, Lloret-Lloret E, Pascual M, Petza D, Rilov G, Schonwald M, Stelzenmüller V, Katsanevakis S (2021) A review of the combined effects of climate change and other local human stressors on the marine environment. Science of The Total Environment 755, 142564.
| Crossref | Google Scholar | PubMed |
Hart SP, Keough MJ (2009) Does size predict demographic fate? Modular demography and constraints on growth determine response to decreases in size. Ecology 90(6), 1670-1678.
| Crossref | Google Scholar | PubMed |
Key MM, Jr., Jackson PNW, Falvey LW, Roth BJ (2014) Use of fossil bryozoans in sourcing lithic artifacts. Geoarchaeology 29(5), 397-409.
| Crossref | Google Scholar |
Key MM, Jr, Rossi RK, Smith AM, Hageman SJ, Patterson WP (2018) Stable isotope profiles of skeletal carbonate validate annually-produced growth checks in the bryozoan Melicerita chathamensis from Snares Platform, New Zealand. Bulletin of Marine Science 94(4), 1447-1464.
| Crossref | Google Scholar |
Lartaud F, Pareige S, de Rafelis M, Feuillassier L, Bideau M, Peru E, Romans P, Alcala F, Le Bris N (2013) A new approach for assessing cold-water coral growth in situ using fluorescent calcein staining. Aquatic Living Resources 26(2), 187-196.
| Crossref | Google Scholar |
Lartaud F, Meistertzheim AL, Peru E, Le Bris N (2017) In situ growth experiments of reef-building cold-water corals: the good, the bad and the ugly. Deep Sea Research Part I: Oceanographic Research Papers 121, 70-78.
| Crossref | Google Scholar |
Laverick JH, Green TK, Burdett HL, Newton J, Rogers AD (2019) Depth alone is an inappropriate proxy for physiological change in the mesophotic coral Agaricia lamarcki. Journal of the Marine Biological Association of the United Kingdom 99(7), 1535-1546.
| Crossref | Google Scholar |
Li S, Liu C, Huang J, Liu Y, Zheng G, Xie L, Zhang R (2015) Interactive effects of seawater acidification and elevated temperature on biomineralization and amino acid metabolism in the mussel Mytilus edulis. Journal of Experimental Biology 218(22), 3623-3631.
| Crossref | Google Scholar |
Lombardi C, Cocito S, Occhipinti-Ambrogi A, Hiscock K (2006) The influence of seawater temperature on zooid size and growth rate in Pentapora fascialis (Bryozoa: Cheilostomata). Marine Biology 149(5), 1103-1109.
| Crossref | Google Scholar |
Lombardi C, Rodolfo-Metalpa R, Cocito S, Gambi MC, Taylor PD (2011) Structural and geochemical alterations in the Mg calcite bryozoan Myriapora truncata under elevated seawater pCO2 simulating ocean acidification. Marine Ecology 32(2), 211-221.
| Crossref | Google Scholar |
Lonhart SI (2012) Growth and distribution of the invasive bryozoan Watersipora in Monterey Harbor, California. In ‘Diving For Science 2012. Proceedings of the American Academy of Underwater Sciences 31st Scientific Symposium’. (Eds DL Steller, LK Lobel) pp. 89–98. (American Academy of Underwater Sciences: Dauphin Island, AL, USA)
Lukasik JJ, James NP, McGowran B, Bone Y (2000) An epeiric ramp: low-energy, cool-water carbonate facies in a Tertiary inland sea, Murray Basin, South Australia. Sedimentology 47(4), 851-881.
| Crossref | Google Scholar |
Magnabosco G, Polishchuk I, Erez J, Fermani S, Pokroy B, Falini G (2018) Insights on the interaction of calcein with calcium carbonate and its implications in biomineralization studies. CrystEngComm 20(30), 4221-4224.
| Crossref | Google Scholar |
Mahé K, Bellamy E, Lartaud F, de Rafélis M (2010) Calcein and manganese experiments for marking the shell of the common cockle (Cerastoderma edule): tidal rhythm validation of increments formation. Aquatic Living Resources 23(3), 239-245.
| Crossref | Google Scholar |
McKinney FK, Jaklin A (2000) Spatial niche partitioning in the Cellaria meadow epibiont association, northern Adriatic Sea. Cahiers de Biologie Marine 41(1), 1-17.
| Google Scholar |
McKinney FK, Jaklin A (2001) Sediment accumulation in a shallow-water meadow carpeted by a small erect bryozoan. Sedimentary Geology 145, 397-410.
| Crossref | Google Scholar |
Mello HL, Gordon DP, Ryland JS, Smith AM (2023) Protecting the small: preliminary investigation of bryozoan community change in New Zealand’s oldest marine reserve. New Zealand Journal of Marine and Freshwater Research 57, 425-437.
| Crossref | Google Scholar |
Ostrovsky AN (2013a) From incipient to substantial: evolution of placentotrophy in a phylum of aquatic colonial invertebrates. Evolution 67(5), 1368-1382.
| Crossref | Google Scholar | PubMed |
Pätzold J, Ristedt H, Wefer G (1987) Rate of growth and longevity of a large colony of Pentapora foliacea (Bryozoa) recorded in their oxygen isotope profiles. Marine Biology 96(4), 535-538.
| Crossref | Google Scholar |
Riisgård HU, Manríquez P (1997) Filter-feeding in fifteen marine ectoprocts (Bryozoa): particle capture and water pumping. Marine Ecology Progress Series 154, 223-239.
| Crossref | Google Scholar |
Rodolfo-Metalpa R, Lombardi C, Cocito S, Hall-Spencer JM, Gambi MC (2010) Effects of ocean acidification and high temperatures on the bryozoan Myriapora truncata at natural CO2 vents. Marine Ecology 31(3), 447-456.
| Crossref | Google Scholar |
Schafer P, Bader B, Blaschek H (2006) Morphology and function of the flexible nodes in the cheilostome bryozoan Cellaria sinuosa (Hassall). Courier Forschungsinstitut Senckenberg 257, 119-131.
| Google Scholar |
Schindelin J, Arganda-Carreras I, Frise E, Kaynig V, Longair M, Pietzsch T, Preibisch S, Rueden C, Saalfeld S, Schmid B, Tinevez J-Y, White DJ, Hartenstein V, Eliceiri K, Tomancak P, Cardona A (2012) Fiji: an open-source platform for biological-image analysis. Nature Methods 9(7), 676-682.
| Crossref | Google Scholar | PubMed |
Smith AM (1995) Palaeoenvironmental interpretation using bryozoans: a review. Geological Society, London, Special Publications 83(1), 231-243.
| Crossref | Google Scholar |
Smith AM (2007) Age, growth and carbonate production by erect rigid bryozoans in Antarctica. Palaeogeography, Palaeoclimatology, Palaeoecology 256(1–2), 86-98.
| Crossref | Google Scholar |
Smith AM (2014) Growth and calcification of marine bryozoans in a changing ocean. The Biological Bulletin 226(3), 203-210.
| Crossref | Google Scholar | PubMed |
Smith AM, Key MM, Jr. (2004) Controls, variation, and a record of climate change in detailed stable isotope record in a single bryozoan skeleton. Quaternary Research 61(2), 123-133.
| Crossref | Google Scholar |
Smith AM, Lawton EI (2010) Growing up in the temperate zone: age, growth, calcification and carbonate mineralogy of Melicerita chathamensis (Bryozoa) in southern New Zealand. Palaeogeography, Palaeoclimatology, Palaeoecology 298(3–4), 271-277.
| Crossref | Google Scholar |
Smith AM, Stewart B, Key MM, Jr., Jamet CM (2001) Growth and carbonate production by Adeonellopsis (Bryozoa: Cheilostomata) in Doubtful Sound, New Zealand. Palaeogeography, Palaeoclimatology, Palaeoecology 175(1–4), 201-210.
| Crossref | Google Scholar |
Smith AM, Key MM, Wood ACL (2019) Culturing large erect shelf bryozoans: skeletal growth measured using calcein staining in culture. Australasian Palaeontological Memoirs 52, 131-138.
| Google Scholar |
Smith AM, Batson PB, Achilleos K, Tamberg Y (2022) Collecting and culturing bryozoans for regenerative studies. In ‘Whole-body regeneration: methods and protocols’. (Eds S Blanchoud, B Galliot) pp. 151–177. (Humana: New York, NY, USA) 10.1007/978-1-0716-2172-1_8
Spires JE, North EW (2022) Marking the shells of juvenile and adult eastern oysters, Crassostrea virginica, with the fluorochrome dye calcein and measuring growth and mortality after marking. Journal of Molluscan Studies 88(1), eyac004.
| Crossref | Google Scholar |
Suggett DJ, Dong LF, Lawson T, Lawrenz E, Torres L, Smith DJ (2013) Light availability determines susceptibility of reef building corals to ocean acidification. Coral Reefs 32(2), 327-337.
| Crossref | Google Scholar |
Swezey DS, Bean JR, Ninokawa AT, Hill TM, Gaylord B, Sanford E (2017a) Interactive effects of temperature, food and skeletal mineralogy mediate biological responses to ocean acidification in a widely distributed bryozoan. Proceedings of the Royal Society B: Biological Sciences 284(1853), 20162349.
| Crossref | Google Scholar |
Swezey DS, Bean JR, Hill TM, Gaylord B, Ninokawa AT, Sanford E (2017b) Plastic responses of bryozoans to ocean acidification. Journal of Experimental Biology 220(23), 4399-4409.
| Crossref | Google Scholar |
Tambutté E, Tambutté S, Segonds N, Zoccola D, Venn A, Erez J, Allemand D (2012) Calcein labelling and electrophysiology: insights on coral tissue permeability and calcification. Proceedings of the Royal Society B: Biological Sciences 279(1726), 19-27.
| Crossref | Google Scholar |
Wood ACL, Probert PK, Rowden AA, Smith AM (2012) Complex habitat generated by marine bryozoans: a review of its distribution, structure, diversity, threats and conservation. Aquatic Conservation: Marine and Freshwater Ecosystems 22(4), 547-563.
| Crossref | Google Scholar |
Wood ACL, Rowden AA, Compton TJ, Gordon DP, Probert PK (2013) Habitat forming bryozoans in New Zealand: their known and predicted distribution in relation to broad-scale environmental variables and fishing effort. PLoS ONE 8(9), e75160.
| Crossref | Google Scholar | PubMed |