Functional roles of coral reef primary producers examined with stable isotopes
Sara Godinez-Espinosa




A School of Natural Sciences, Macquarie University, Sydney, NSW 2109, Australia.
B School of Environmental and Life Sciences, University of Newcastle, Ourimbah, NSW 2258, Australia.
C Centre for Tropical Water and Aquatic Ecosystem Research, James Cook University, Cairns, Qld 4879, Australia.
Marine and Freshwater Research 74(7) 601-613 https://doi.org/10.1071/MF22103
Submitted: 8 August 2022 Accepted: 11 April 2023 Published: 3 May 2023
© 2023 The Author(s) (or their employer(s)). Published by CSIRO Publishing. This is an open access article distributed under the Creative Commons Attribution-NonCommercial-NoDerivatives 4.0 International License (CC BY-NC-ND)
Abstract
Context: Primary production on coral reefs varies under changing conditions such as light and nutrient availability. This variation causes changes in basal stable isotopes as photosynthetic and nutrient pathways change.
Aims: This study provides a preliminary baseline of nitrogen (δ15N) and carbon (δ13C) stable isotope profiles in Symbiodinium and macroalgae at a spatial scale and along a depth gradient around an island.
Methods: Coral fragments and macroalgae were collected at depths from the surface to 26 m. δ15N and δ13C stable isotope values were assessed for Symbiodinium relative to cell density per surface area.
Key results: δ15N values showed a uniform nutrient profile across primary producers. However, chlorophyll-a and Symbiodinium density from Montipora stellata had higher concentrations on the southern side of the island. δ15N values of Symbiodinium from Stylophora pistillata and macroalgae did not change with depth. Depth was associated with a significant decrease in Symbiodinium density, and δ13C values in macroalgae.
Conclusions: We attribute these findings to Symbiodinium from S. pistillata as depth increases, decreasing cell density but maintaining chlorophyll-a concentration to satisfy the coral-host nutrient requirements.
Implications: This study sets the scene for future, more comprehensive research on detecting carbon and nitrogen stable isotope values on primary producers in coral reefs.
Keywords: carbon, depth, macroalgae, nitrogen, nutrient, nutrients, photogrammetry, primary productivity, SIA, stable isotope baseline, Symbiodinium, Vanuatu.
Introduction
Primary production in tropical coral reefs occurs from a range of organisms, including corals and macro-, epiphytic and symbiotic algae. The rate of primary production is dictated by environmental factors and an organism’s ability to adapt or acclimate under local conditions (Hoey et al. 2016; Baums et al. 2019). Changes in light and nutrient availability are key drivers of primary production in coral ecosystems that can alter photosynthetic and nutrient pathways of primary producers (Brandl et al. 2019; Johnson et al. 2020). However, the response of primary producers to environmental changes is unlikely to be uniform and may depend on the type of species and magnitude of change (Perry et al. 2011).
Scleractinian corals are sensitive to environmental change, particularly variations in local nitrogen (Lapointe 1997; Hoegh-Guldberg and Williamson 1999) and light (Tremblay et al. 2015) availability. Environmental change can modify coral metabolism (Fabricius 2005) and growth (Carricart-Ganivet et al. 2000), which are then reflected in changes to their soft tissue and preserved skeletal structure (Wheeler and Björnsäter 1992; Chan et al. 2016; Mollica et al. 2018). The relationship between host corals and their endosymbiotic zooxanthellae is complex and also depends on environmental conditions (Baker 2003). Carbon and nitrogen are essential for Symbiodinium photosynthesis, and for the translocation of organic molecules to the coral host (Ezzat et al. 2017). Photosynthetic rates for Symbiodinium are generally higher in shallow water and high light conditions (Reynaud et al. 2009), whereas photosynthetic production conversely decreases under diminishing light conditions and increasing depths (Gattuso et al. 1993; Anthony and Fabricius 2000). However, Symbiodinium in some species of coral can uncouple this relationship and maintain or increase their photosynthetic activity in low light conditions (Lesser et al. 2010; Seemann et al. 2012). Light thus plays a key role in regulating the amount of carbon translocated from Symbiodinium to the coral host, facilitating respiration and growth (Ezzat et al. 2017). This is particularly important in shallow-water corals because photosynthetically-fixed carbon from Symbiodinium may provide ≥70% of their daily carbon budget (Tanaka et al. 2006). This relationship between scleractinian corals and Symbiodinium, and their patterns of interactions with abiotic parameters, offers an opportunity to use corals as bioindicators to assess changes in water quality, trace-nutrient inputs, and nutrient recycling (Reynaud et al. 2009).
Macroalgae grow alongside coral on many reefs and can act as bioindicators of nitrogen by providing accurate records of nitrogen assimilation (Umezawa et al. 2002) and temporal information of biologically available nitrogen in the environment (Costanzo et al. 2001). Although being similar in their use as bioindicators, corals and macroalgae differ in the way they assimilate nitrogen. Macroalgae readily assimilate nutrients directly from the water column (Koop et al. 2001) or from heterotrophs (Williamson and Rees 1994), whereas uptake in hermatypic corals depends on nutrient recycling with their symbiotic algae, in addition to nutrient availability in the water column (Grottoli et al. 2006; Tanaka et al. 2010). It is therefore expected that stable isotope signatures of macrophytes will directly relate to exogenous nutrient availability, whereas the nutrient recycling that occurs in coral tissues will act as a mediator to environmental conditions. Because of this, one can also hypothesise that nutrient signatures of algae, such as macroalgae and their associated algal epiphytes, that are directly exposed to the external environment would differ when compared with those of coral endosymbiotic algae in the same habitat.
Stable isotopes are widely used to examine photosynthetic, nutrient and carbon pathways in marine environments such as coral reefs (Risk et al. 2009; McMahon et al. 2016; Eurich et al. 2019; Koweek et al. 2019). In corals, nitrogen isotopes can discriminate natural and anthropogenic sources of nitrogen, and trophic level by associating the degree of autotrophy or heterotrophy (Owens 1988; Muscatine et al. 2005; Risk et al. 2009). Similarly, carbon stable isotopes can be used as a tool to discriminate carbon fixation pathways (Fry and Sherr 1989; Seemann 2013). The effects of depth on coral and macroalgal stable isotopes have been examined (Sherwood et al. 2008; Alamaru et al. 2009); yet, the effects of depth and mixotrophy on the stable isotope values of Symbiodinium are not well understood.
Little is known of the status of the coral reefs in the Republic of Vanuatu (‘Vanuatu’). Vanuatu is considered a Small Island Developing State (SIDS), which is typified by a vulnerability to impacts associated with climate change, such as an increase in the frequency of extreme weather events, coastal erosion, increases in water temperature, and coral bleaching (Buckwell et al. 2020). For example, bleaching events in Vanuatu caused mass mortality of corals in 2002, and Cyclone Pam caused severe damage to 80% of hard corals in 2015 (Sulu 2007; Burke et al. 2012). In addition, Vanuatu does not harbour a sophisticated sewage treatment system, and much of the terrestrial waste occurs as runoff into adjacent reefs (Mosley and Aalbersberg 2003). These make Vanuatu, and particularly the island of Efate where the majority of Nivans reside, an interesting location for analysing the nutrient profiles of key reef-associated primary producers by using stable isotope analysis.
Here we examined spatial changes in nutrient sources of Symbiodinium from two common scleractinian corals and associated algae on a shallow coral lagoonal reef in Vanuatu by using stable isotope analysis (SIA). The following questions were asked: (1) can SIA detect spatial changes in nutrient signatures (i.e. nitrogen and carbon) for primary producers on a lagoonal reef at a medium scale (i.e. zones around a small island, north, south, east, west); (2) do nutrient profiles of coral endosymbionts differ from those of adjacent macroalgae and epiphytes in a shallow coral lagoonal reef; and (3) does depth influence any nutrient uptake detected in Symbiodinium, macroalgae or epiphytes? For the second question, we hypothesised that in the shallow reef waters, endosymbiotic algae within corals would maintain a uniform nutrient profile because of nutrient recycling between Symbiodinium and the coral host, whereas the nutrient profile of macroalgae (and their epiphytes) would exhibit grater variation linked to exogenous nutrient availability. Finally, we hypothesised that nutrient availability would change as depth increased and light decreased, driving differences in N and C isotopic signatures for macroalgae and epiphytic algae, but not for Symbiodinium because their ability to compensate by increasing chlorophyll-a (Chl-a) concentration and cell density in the coral host.
Materials and methods
Study site and sample collection
Endosymbiotic algae, macroalgae and epiphytes were sampled from the shallow fringing reef surrounding Hideaway Island (17°41′49.2324′S, 168°15′49.0788′E) located in Mele Bay, Efate, Vanuatu, in December 2017 (Fig. 1). Mele Bay is among the more adversely affected sites in Vanuatu (Mosley and Aalbersberg 2003), receiving pollution and high nutrient-load discharges from Tagabe River, including septic runoff (Poustie and Deletic 2014). Additionally, Hideaway Island is located at the mouth of two freshwater rivers that discharge to the north-west and north-east of Hideaway Island. Ten sites were chosen across the reef flat at Hideaway Island, and subsequently categorised into zones according to their cardinal locations in relation to Hideaway Island (western, eastern and southern). North of the island was excluded from sampling because of a lack of fringing reef in that direction.
Hideaway Island (17°41′49.2324′S, 168°15′49.0788′E), Vanuatu. Eastern collection zones are shown in black, southern zones in purple, and western zones in blue. Collection sites are numbered from 1 to 10. Depth sampling occurred on the south-western end of the reef wall, between the southern and western zones. Image courtesy of Google Earth.
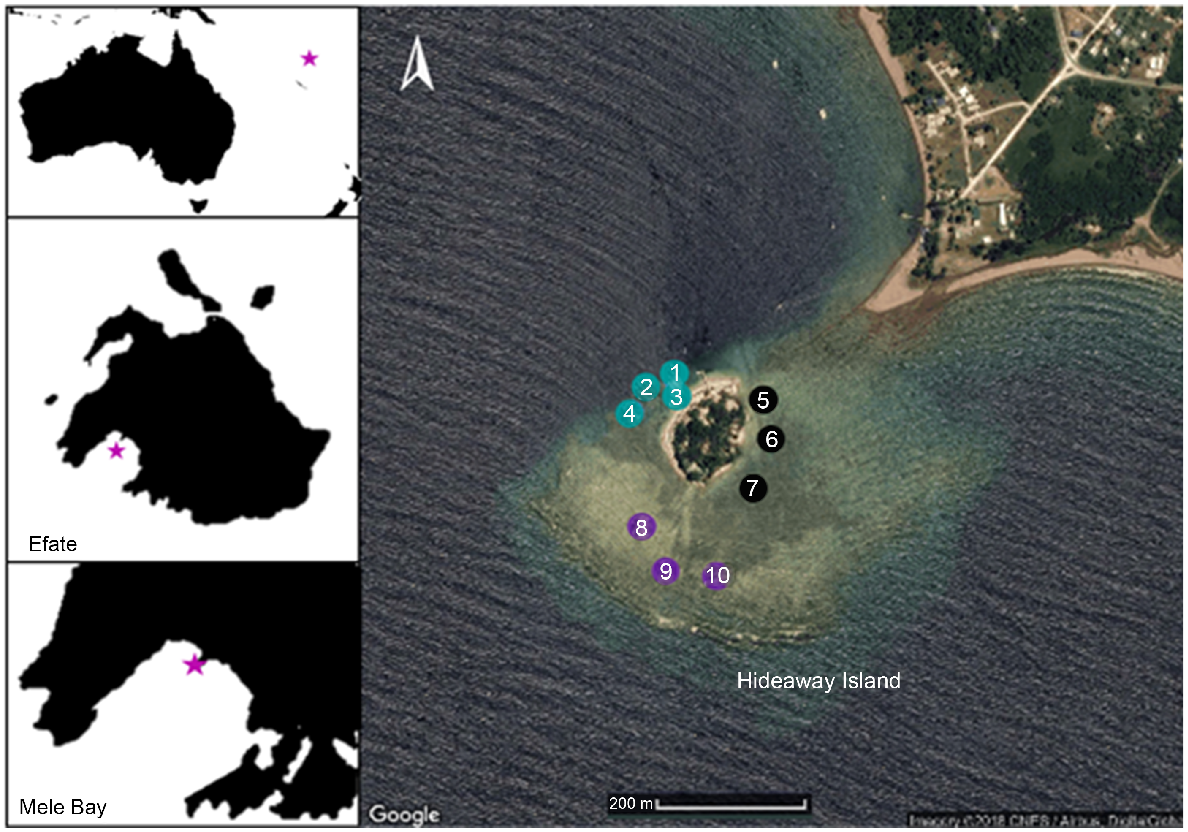
The branching scleractinian coral Montipora stellata and a mix of two species of macroalgae, namely, Actinotrichia fragilis and Galaxaura rugosa, commonly occur on the reef flat surrounding Hideaway Island (T. M. Smith, 2017, pers. obs.). These macroalgae routinely occur together and typically contain small amounts of attached epiphytic algae. This coral (known to harbour endosymbiotic algae), and macroalgae were haphazardly collected at the 10 sites along the reef flat. At each site, three small fragments of live M. stellata (~5-cm tip of a branch) were removed on snorkel at 1-m depth from healthy colonies (Fig. 1) for stable isotope analysis. To ensure that the primary producers came from the same microenvironment, the macroalga closest to the sampled coral was also identified and removed. After collection, all coral and macroalgal samples were placed in individual resealable bags and immediately frozen at −20°C until processing.
To assess the effect of depth on primary producers, one coral fragment (~5 cm tip of a branch) and one adjacent macroalga were collected haphazardly at each depth, while swimming in a zig-zag pattern from depth to the surface over a depth gradient ranging from 26 to 3 m on SCUBA at the south-western area of Hideaway Island. This area was chosen because of its depth profile, but also because it was one of the more distant areas from the river mouths. Because few or no M. stellata colonies could be found at depths greater than 3 m on the reef slope, the scleractinian coral Stylophora pistillata, which occurred across this depth gradient, was sampled instead. Similarly, G. rugosa was not found across the depth gradient; however, Amphiroa rigida and A. fragilis were consistently present; therefore, the closest macroalgal mix of these species to the sampled coral at each depth was collected. Samples were immediately individually bagged and frozen at −20°C until processing.
Symbiodinium preparation
In the laboratory, coral host and endosymbiont tissue were removed from the calcium carbonate skeleton for each coral fragment by using a Waterpik Ultra Water Flosser filled with reverse-osmosis (RO) water. The resulting slurry for each sample was placed in a 50-mL centrifuge tube and homogenised using a knife mill (Retsch GM200) set to Speed 2 for 5 min. Three aliquots of 1 mL were separated from the resulting homogenised slurry of each sample to assess Symbiodinium density. Additionally, three aliquots of 1.5 mL were taken from each slurry to perform measurements of Chl-a concentration. The remaining slurry samples containing host tissue and Symbiodinium were centrifuged (Eppendorf 5810 R) at 1700g and ambient temperature for 5 min to separate the host tissue from Symbiodinium. To ensure all coral host tissue was removed, the pellet containing Symbiodinium was resuspended with 5 mL RO water followed by centrifugation at 600g for 5 min (Wong et al. 2017). Symbiodinium pellets were oven dried at 60°C for 48 h, then ground to a fine powder by using a mortar and pestle, and a quantity of 1–2 mg of each sample was weighed into tin capsules for isotope analysis.
To determine the density of symbiont cells in the coral fragments, eight replicate counts were conducted from each 1-mL aliquot collected above from each sample. Aliquots (10 μL) from the coral host tissue–Symbiodinium slurry were added to a 0.1-mm-deep Improved Neubauer Haemocytometer and cells were counted immediately under a light microscope at 40× magnification (Olympus BX51, Japan). The 1.5-mL aliquots of the homogenised coral host tissue–Symbiodinium slurry of each sample were centrifuged (Eppendorf 5810 R) at 600g for 5 min in a 3-mL tube. The supernatant was discarded and the pellets containing Symbiodinium cells were used to extract Chl-a. A volume of 1 mL of cold 100% acetone was added to each Symbiodinium sample. Pigments were then extracted for 24 h at 4°C (Grottoli et al. 2004). The absorbance of Symbiodinium extract was measured at 630, 660 and 750 nm by using a spectrophotometer (SPECTROstar Nano, BMG Labtech Plate Reader), and the Jeffrey and Humphrey (1975) equation for dinoflagellates was used to standardise the Chl-a concentration.
Surface-area determination for coral
Determination of surface area for coral fragments was important to estimate endosymbiotic algal densities and Chl-a concentration. Structure from motion photogrammetry (House et al. 2018) was used to determine the surface area of each coral fragment. Each individual coral fragment was placed on a rotating table next to a 3 × 3-cm scale and overlapping photographs were taken while rotating the sample in a clockwise direction. Image processing was performed using Agisoft Photoscan Professional (ver. 1.2.5, Agisoft LLC, Saint Petersburg, Russian Federation). Images were aligned using the high to medium accuracy setting, with a key point limit of 40 000 and a tie point limit of 1000. Previous research on similar coral morphology showed no difference in accuracy between the high and medium settings (Raoult et al. 2017). The software then determined the camera position and generated points into a three-dimensional space (Fig. 2). This was followed by the generation of a dense point cloud, again by using the medium to high quality and an aggressive depth filtering. Once the point cloud was generated, a mesh was built from the overlapping images with the following settings: arbitrary surface type, dense cloud source data, high face count, and enabled (default) interpolation. On completion of the mesh build, two markers were placed on the limits of the 3 × 3 square scale to create a scale bar. The model of the coral fragment was then manually trimmed from the rest of the mesh under high resolution, and any holes in the mesh were closed by using the mesh tool, as per Raoult et al. (2017).
Agisoft Photoscan workflow for Stylophora pistillata; (a) aligned points from the coral images into a three-dimensional (3-D) space; (b) alignment of pictures (in blue) and the creation of the dense point cloud; (c) creation of the high polygon mesh of the coral fragment and the scale from the dense point cloud; (d) cropped and completed 3-D model of the coral fragment, including the markers on the scale.
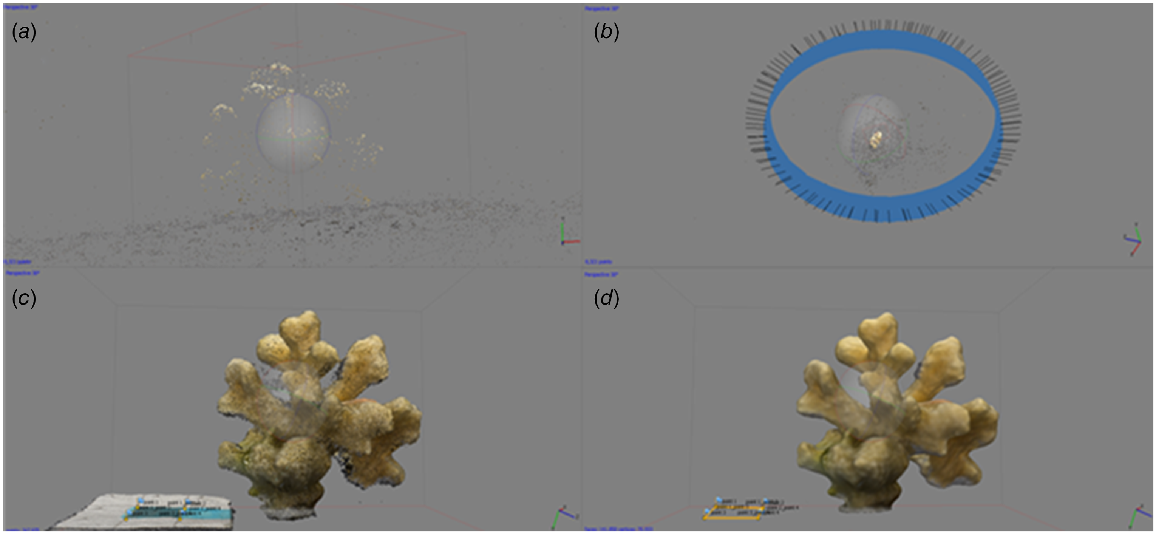
Prior to using ‘structure from motion’ photogrammetry, a preliminary evaluation was performed to compare the results of this method against the aluminium-foil method developed by Marsh (1970). Importantly, although surface-area estimates produced by both methods were statistically indistinguishable, values derived from ‘structure from motion’ photogrammetry exhibited the lowest mean coefficient of variation across coral fragments and as such, this was selected as our method-of-choice when normalising cell densities and Chl-a concentration to surface area in this study.
Macroalgal and epiphyte preparation
Macroalgae and attached epiphytic algae were cleaned and separated following an adaptation of the technique described by Zimba and Hopson (1997). Each macroalga was placed in a 200-mL screw-top jar and RO water was added until the macroalga was completely covered. The jar was closed and manually shaken for 40 s to remove the epiphytes from the macroalga. The macroalga was then removed from the jar and the epiphyte slurry, and the surface of the macroalga was visually assessed and any remaining epiphytes were carefully removed using scalpel and forceps. After removal of epiphytes, the macroalga was rinsed with RO water and oven dried for 48 h at 60°C. Each epiphyte slurry had non-algal epiphytes removed, and was then mixed and filtered through a 100-μm mesh before baking oven-dried for 48 h at 60°C. All samples were ground to a fine powder by using a mortar and pestle, and 6–8 mg of powdered tissue was placed into a separate tin capsule for subsequent stable isotope analysis.
Stable isotope analysis
Samples were analysed for nitrogen (15N:14N) and carbon (13C:12C) stable isotopes by using a Europa EA GSL elemental analyser coupled to a Hydra 2022 mass spectrometer (Sercon Ltd, UK) at Griffith University (Nathan Campus, Brisbane, Qld, Australia). Precision of this spectrometer is within 0.1% for δ15N and δ13C values (Raoult et al. 2015). Ratios of 15N:14N (δ15N) and 13C:12C (δ13C) were expressed as the relative difference between the sample and a standard of atmospheric nitrogen (15N) and Pee Dee Belemnite (13C), in parts per thousand (‰). Elemental precision relative to standards was 0.2 for δ13C and 0.1 for δ15N.
Statistical analysis
All statistical analyses were performed using RStudio (ver. 1.1.453, Posit Software, PBC, Boston, MA, USA, see https://posit.co/) and R (ver. 3.4.4, R Foundation for Statistical Computing, Vienna, Austria, see https://www.r-project.org/). First, it was necessary to determine the optimal coral surface-area measurement technique. Linear mixed models with the package lme4 (ver. 1.8.5, see https://CRAN.R-project.org/package=lme4; Bates et al. 2015) were used to compare the surface-area measurements obtained with structure from motion photogrammetry and the aluminium-foil method. For this analysis, surface area was the response variable and the method used was set as a fixed effect, and sites (1–10) and coral replicates (A, B, C) were treated as random effects.
ANOVA, linear regression models and paired Student’s t-tests were used to test for differences in cell densities, Symbiodinium, Chl-a concentration and δ15N and δ13C values around Hideaway Island and along a depth gradient. Cell densities, Chl-a concentration and δ15N and δ13C values around the island were analysed separately by using a nested ANOVA, where zone (eastern, western, southern) around the island was treated as a fixed factor and site within each zone was treated as a random factor. To assess whether there were more specific spatial differences around the island, a one-way ANOVA where site was treated as a factor was performed for each variable. Post hoc Tukey HSD tests were performed if there was a significant difference across zones and sites in each analysis, by using the package emmeans (ver. 1.1-33, see https://cran.r-project.org/package=emmeans). Differences in cell densities, Symbiodinium Chl-a concentration and δ15N and δ13C values across a depth gradient were compared in separate linear regression analysis using the lme4 package. Additionally, an analysis was performed comparing Chl-a concentration that had been standardised for surface area at different depths by using a linear regression. A paired Student’s t-test was used to test for differences in the δ15N and δ13C values between macroalgae and epiphytes at each site and depth.
Results
Surface-area determination for coral
No significant difference between the measurements of coral surface area calculated from photogrammetry and the aluminium-foil method was observed (F8,47 = 9.44, P = 0.07). However, coral surface-area data derived from photogrammetry exhibited a lower coefficient of variation (55.5 %) than did those from the aluminium-foil method (59.6%). Therefore, the calculated area of each coral fragment determined by photogrammetry was used to normalise the Chl-a concentration and Symbiodinium density to surface area in square centimetres for all coral fragments.
Coral endosymbiont density and Chl-a concentration
For M. stellata, Symbiodinium cell densities (F2,7 = 5.53, P = 0.03; Fig. 3a) and Chl-a concentration (F2,7 = 4.72, P = 0.05; Fig. 3b) were significantly different among the three zones. Samples collected at the southern zone had the highest Chl-a concentration in Symbiodinium, as well as the highest average cell density compared with the western and eastern zones (Table 1).
Mean (±1 s.e.) (a) cell density (105 cells cm−2) and (b) Chl-a concentration (μg cm−2) of Symbiodinium from Montipora stellata at 10 sites around Hideaway Island. Zones in relation to the island are denoted by: w, western; e, eastern; s, southern.
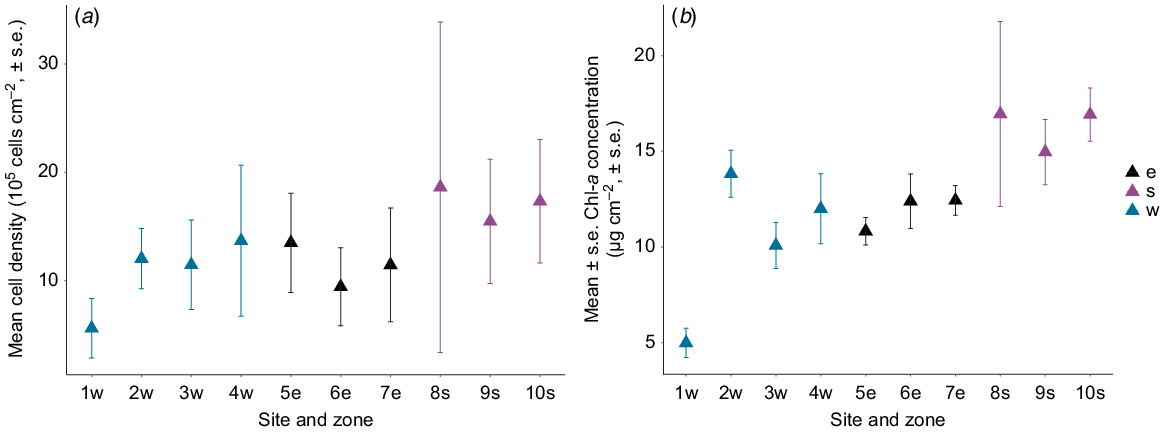
Zone | Cell density (105 cells cm−2) | Chl-a concentration (μg cm−2) |
Eastern | 11.46 ± 0.94 | 11.88 ± 0.58 |
Western | 10.70 ± 0.91 | 10.22 ± 0.92 |
Southern | 17.15 ± 1.19 | 16.27 ± 1.67 |
Cell densities and Chl-a concentration of Symbiodinium from S. pistillata differed in their responses to depth (F1,37 = 14.64, P < 0.001, R2 = 0.26). Symbiodinium cell density decreased significantly as depth increased (F1,37 = 10.38, P < 0.001, R2 = 0.12; Fig. 4a); however, surface area-normalised Chl-a concentration remained unchanged with depth (F1,37 = 2.06, P = 0.15, R2 = 0.03; Fig. 4b).
Spatial changes in the nutrient signatures of primary producers
The δ15N mean values for Symbiodinium from M. stellata did not differ significantly among the three zones however, significant differences were observed at the site level (Table 1; F9,16 = 21.49, P < 0.01). Specifically, Symbiodinium δ15N values from Site 1 in the western zone were higher than those from the remaining of the nine sites (P < 0.01). The majority of macroalgae collected from each site had epiphytic algae attached. No significant differences in δ15N values of macroalgae and epiphytes were observed among the three zones (Table 2); however, nutrient signatures were much more variable for both macroalgae and epiphytic algae than for the endosymbiotic algae. However, significant differences in macroalgal δ15N values were detected when the 10 sites around the island were analysed independently regardless of zone (F9,20 = 3.36, P = 0.01). Specifically, the δ15N values of macroalgae from Site 5 in the eastern zone were significantly higher than those from Sites 2 (P = 0.04) and 4 (P < 0.01) within the western zone (Fig. 5). Overall, δ15N values of epiphytes were lower than those of the macroalgae (Table 2).
Zone | δ15N‰ Symbiodinium | δ15N‰ macroalgae | δ15N‰ epiphytes | δ13C‰ Symbiodinium | δ13C‰ macroalgae | δ13C‰ epiphytes |
Eastern | 4.1 ± 0.1 | 6.4 ± 0.6 | 2.3 ± 0.2 | −14.0 ± 0.2 | −9.9 ± 0.3 | −13.4 ± 0.7 |
Western | 4.4 ± 0.1 | 5.1 ± 0.6 | 2.7 ± 0.3 | −13.8 ± 0.1 | −9.1 ± 0.3 | −14.4 ± 0.7 |
Southern | 4.2 ± 0.1 | 6.0 ± 0.4 | 3.4 ± 0.3 | −14.4 ± 0.1 | −10.1 ± 0.2 | −13.7 ± 1.0 |
Values (mean ± s.e.) of δ15N of Symbiodinium from Montipora stellata, macroalgae, and epiphytes around Hideaway Island. Zones in relation to the island are denoted by: w, western; e, eastern; s southern.
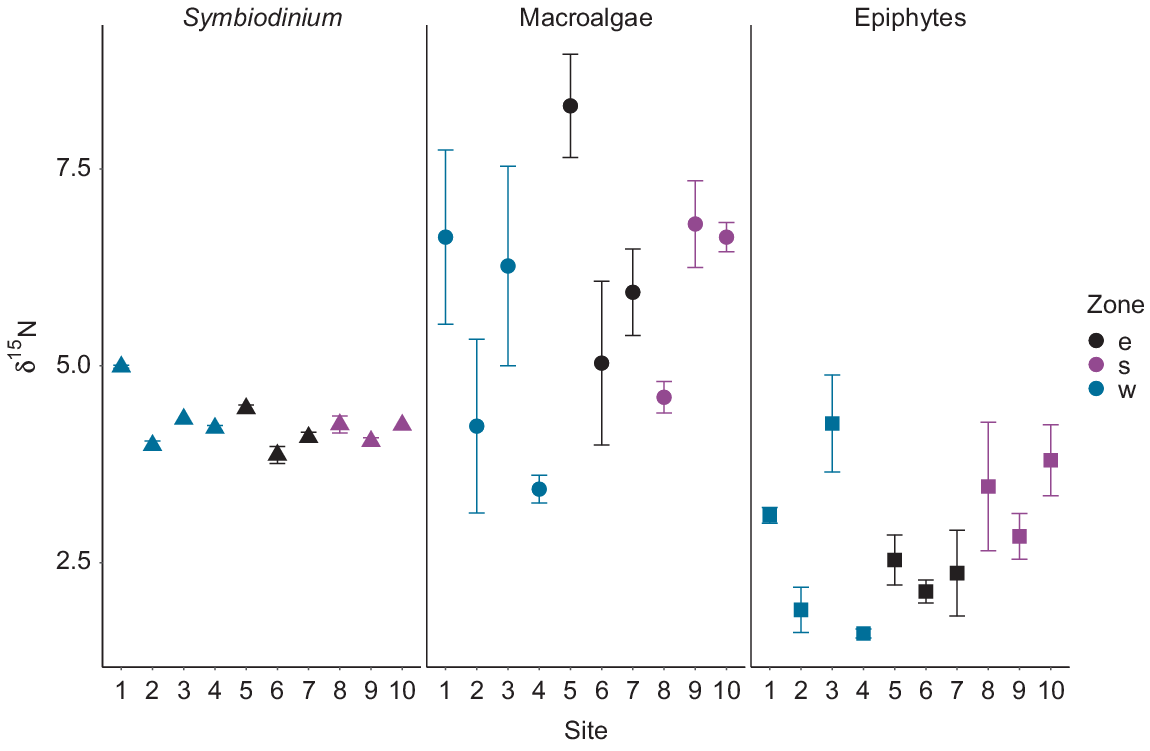
Significant differences were detected between δ13C values of Symbiodinium (F2,23 = 3.77, P = 0.03) among the three zones around Hideaway Island (Fig. 6). Symbiodinium individuals from the western zone were significantly more enriched than those from the southern zone (P = 0.01). However, the δ13C values of Symbiodinium from the southern and eastern zones were similar (post hoc Tukey P > 0.1), together with the eastern and western zones (P > 0.5). The mean δ13C values of macroalgae and epiphytes collected at the three different zones around Hideaway Island at the same depth exhibited no differences among the zones, but were highly variable within a site (Fig. 6). Overall, the macroalgae collected around the island displayed the highest δ13C values compared with those of Symbiodinium and epiphytes (Table 2).
Depth changes in the nutrient signatures of primary producers
Values of δ15N for Symbiodinium from S. pistillata ranged from 3.9‰ at 23.5-m depth to 5.2‰ at 26-m depth (Fig. 7). No significant influence of depth was observed for δ15N from Symbiodinium (F1,9 = 1.59, P = 0.23). δ15N values for macroalgae ranged from 2.8‰ at 15.8-m depth to 4.8‰ at 6.6-m depth. δ15N values for epiphytes varied along the depth gradient, with the lowest δ15N value of 2.6‰ at 13.6- and 17-m depth, and the highest value of 4.6‰ at 23-m depth (Fig. 7). No influence of depth on the δ15N values of macroalgae (F1,15 = 0.67, P = 0.43) or epiphytes (F1,18 = 1.23, P = 0.28) was observed. No significant differences were observed between the mean δ15N values of the macroalgae and the epiphytic algae (P = 0.61). Overall, δ15N values of Symbiodinium were higher than those of the macroalgae and epiphytes.
Variability of δ15N values of Symbiodinium from Stylophora pistillata, macroalgae and epiphytes along a depth gradient from 3 to 26 m.
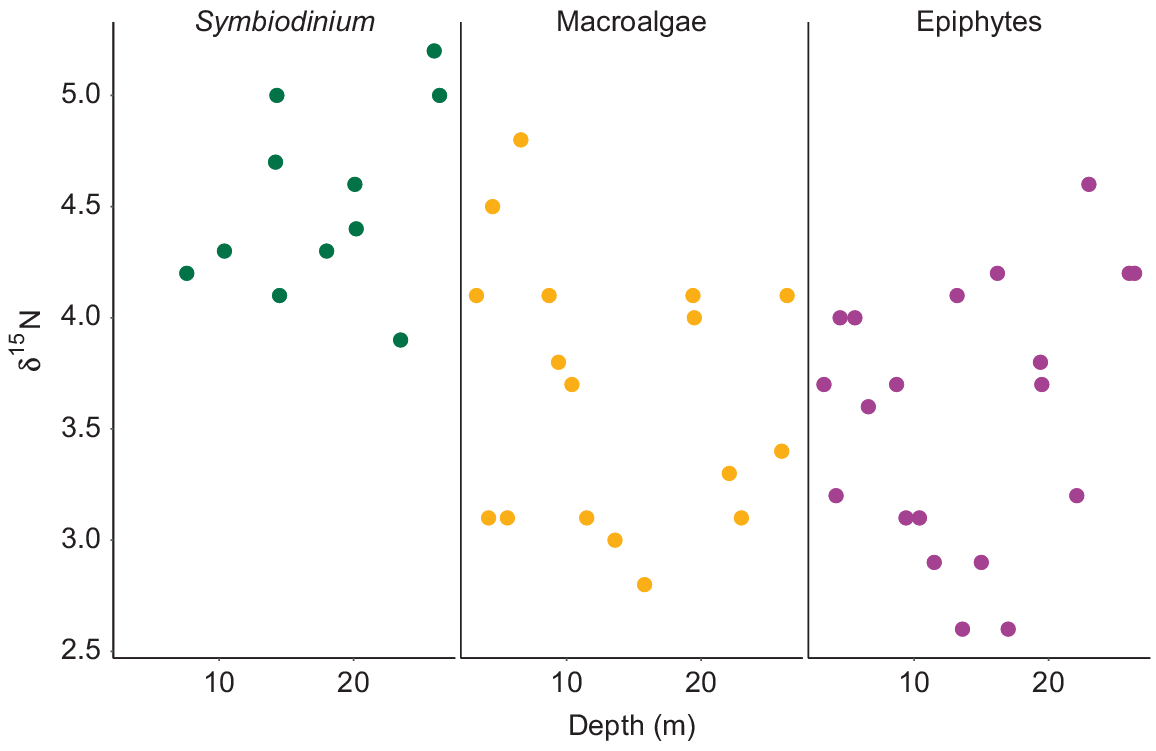
Depth had a significant influence on the δ13C values for macroalgae, but not for the epiphytes or Symbiodinium. δ13C values of Symbiodinium from S. pistillata ranged from −18‰ at 14.3-m depth to −15.2‰ at 10.4-m depth. δ13C values of Symbiodinium exhibited no differences across depth (F1,11 = 1.81, P = 0.21 R2 = 0.06). δ13C values of macroalgae ranged from −20.3‰ at 19.4-m depth to −4.2‰ at 17-m depth, whereas δ13C values of the epiphytes ranged from −24.8‰ at 26.4-m depth to −6.1‰ at 22.1-m depth. δ13C values differed significantly (P < 0.001) between the macroalgae and epiphytes along the depth gradient. Macroalgal δ13C values were higher than the epiphyte δ13C values, and both displayed high variability in values along the depth gradient (Fig. 8). δ13C values of macroalgae varied significantly with depth (F1,22 = 15.47, P < 0.001, R2 = 0.39), whereas no influence of depth was observed in δ13C values of the epiphytes (F1,18 = 2.3, P = 0.15 R2 = 0.06). Macroalgal samples collected at depths of less than 20 m displayed δ13C values up to −10‰, and those collected at depths deeper than 20 m displayed values lower than −10‰. Overall, the δ13C values of Symbiodinium from S. pistillata were more depleted than the δ13C values of macroalgae and epiphytes and displayed lower variability along the depth gradient (Fig. 8).
Discussion
This study examined the nutrient profiles of primary producers on a coral reef flat at a spatial scale and examined the influence of depth on the basis of the function of the producers. The results presented here provide a preliminary baseline for future studies that analyse N and C stable isotopes in benthic primary producers, both at this location, and on similar reef flats. Such baselines provide a useful benchmark tool for assessing anthropogenic pollution, tracking nutrient sources and waste management, and understanding how the relationship among photosynthetic, nutrient and carbon pathways in coral reefs may be affected by future climate change.
Spatial changes in the nutrient signatures of primary producers
Although clear nutrient profiles could be obtained for Symbiodinium, macroalga and epiphytic algae, none of these functional groups of primary producers showed clear spatial patterns of nutrient signatures for nitrogen or carbon at the zonal or site resolution in our study. As such, we were unable to demonstrate that stable isotope analyses can be used to detect spatial changes in primary producers in a lagoonal reef at the scale of this study. However, several factors that could account for this result. Notably, sampling was conducted at a medium scale (i.e. <1 km) around a small island and at only a single point in time, noting that nutrient availability can differ seasonally in coral reef environments (Hatcher 1990). Clearly, a more comprehensive and extensive study that measures nutrients in the surrounding water, in addition to the nutrient profiles of the primary producers, is warranted, ideally encompassing additional locations across multiple time scales so as to properly tease apart the various factors at play.
Variability of nutrient signatures differed among samples collected within sites for macroalgae and epiphytic algae, as observed in the large standard errors at each site. Moreover, generally both carbon and nitrogen values were higher for macroalgae than for epiphytes. Such variances may have been a result of species-specific nutrient profiles; however, the different nutrient values among sites suggest otherwise. Biotic interactions between benthic marine macroalgae and other organisms are quite broad (Hurd et al. 2014). Most of the macroalgae collected around Hideaway Island had epiphytic algae attached, yet macroalgae displayed more enriched δ15N values than did their attached epiphytes. This appears to contradict previous work that suggests that macroalgae usually exhibit a decreased nutrient profile, owing to the fact that epiphytes can access and utilise nutrients before macroalgae, and further exacerbate the issue by shading macroalgal tissue and thus restricting light availability, as observed in Wright et al. (2000) in temperate regions. Further research assessing macroalgae and epiphytic algae at a species level rather than a functional level would be beneficial.
δ15N values of Symbiodinium from M. stellata were substantially less variable at the site scale than were those of macroalgae and epiphytes. This observation supports the hypothesis that macroalgae and epiphytes may be obtaining nitrogen directly from the water column and, thus, may be susceptible to the vagaries of variable exogenous nutrient supply (Umezawa et al. 2002), whereas the endosymbiotic algae can source nitrogen through nutrient-recycling within the coral host, in addition to obtaining it directly from the environment (Grottoli et al. 2006). Interestingly, δ15N values for Symbiodinium (3.9–5‰) in our study were similar to those reported for endosymbiotic algae in coral reef environments lacking anthropogenic nitrogen sources, such as in Dongsha Atoll, Taiwan 4.7‰ (Wong et al. 2017). The δ15N range of macroalga in this study (2.8–8.8‰) was also lower than that previously reported for macroalgae that utilise sewage as a nutrient source, namely +8.5‰ (Lapointe et al. 2005), or +9‰ (Costanzo et al. 2001). These results suggest that no anthropogenic nutrient source was present that could be detected in the δ15N values of benthic primary producers around Hideaway Island at the time of sampling. However, nutrient content in the waters from the river sources and around Hideaway Island need to be directly measured for nitrates, phosphates and faecal coliforms to fully determine the nature and extent of anthropogenic nutrient loading in this ecosystem.
Carbon SIA can be used as a tool to distinguish autotrophic and heterotrophic sources (Seemann 2013). δ13C values of Symbiodinium from M. stellata in this study (−13.1 to −14.7‰) were in a range similar to those previously reported for several symbiotic corals of −10 to −13‰ (Muscatine et al. 1989), −10 to −16‰ (Risk et al. 1994), and −12 to −14‰ (Swart et al. 2005). These previous studies attributed this range of δ13C values to autotrophy in endosymbiotic algae obtaining recycled carbon sources from their hosts. The narrow range of δ13C values of Symbiodinium observed here similarly indicates that the carbon sources are being recycled from the coral host.
Carbon stable isotope values in macroalgae are primarily associated with photosynthetic pathways (Cloern et al. 2002), which often exhibit great diversity across macroalgal lineages (e.g. Zweng et al. 2018). As a result, differences in macroalgal δ13C values vary with species. Green algae are usually more 13C enriched (−12%) than are red algae (−18.3%) (Yamamuro et al. 1995; Wang and Yeh 2003). As with nitrogen, therefore, differences in macroalgal and epiphytic algal δ13C observed in this study may be attributed to species-specific differences in carbon sources. δ13C values of macroalgae had a narrower range and were more enriched (−7.6 to −11.1%) than those of epiphytic algae (−8.3 to −17.8%) in our study, displaying trends similar to those previously reported (Jaschinski et al. 2008; Zheng et al. 2015). The more positive δ13C values observed in the macroalgal samples suggest that macroalgae may rely more on HCO3− as a nutrient source, whereas the more negative δ13C values observed in epiphytic algae suggest that their main carbon source may be CO2. This affinity of carbon sources may be the reason for the observed differences in δ13C values in the macroalgae and epiphytic algae, but it needs to be empirically tested.
Depth changes in the nutrient signatures of primary producers
Depth had a significant effect on the nitrogen and carbon profiles for macroalgae, but not for either epiphytic algae or endosymbiotic algae. Concentrations of both nitrogen and carbon decreased with an increasing depth in macroalgae. High variability of nitrogen and carbon was observed for macroalgae and epiphytes and this, coupled with a lack of replication of depth profiles, makes it difficult to infer any causal relationships here. Similar to our results, previous macroalgal studies have found no clear pattern in these values that could correlate to depth or taxonomy of the species (Marconi et al. 2011).
Depletions in nitrogen and carbon isotopes with increasing depths at Hideaway Island could be attributed to a reduced rate of photosynthesis as a result of reduced light availability. It has been previously reported that macroalgal species with δ13C values more enriched than −10‰ use HCO3− as a carbon source (Raven 1997). Therefore, we suggest that our macroalgae collected at waters 3–20 m deep with δ13C values of −4.2 to −10‰ may have also been using HCO3− as a carbon source, whereas macroalgae in deeper waters (below 20 m depth) may have been using dissolved CO2, which has more depleted δ13C values (Raven 1997). δ13C values in macroalgae in this study suggest that at shallow depths, macroalgae have higher photosynthetic rates than, and utilise a different carbon source from macroalgae from deeper waters. This suggests that macroalgae in our study were relying on photosynthesis for nutrient production.
The variability in cell density and Chl-a concentration decreased for samples collected in depths of 15 m or greater for Symbiodinium from S. pistillata in our study. These results align with previous research that has shown that S. pistillata increases its photosynthetic activity at depth to compensate for the decrease in light, to satisfy the nutritional budgets of the coral host (e.g. Mass et al. 2007). However, contrary to our results, other studies have reported a corresponding increase in Chl-a concentration for Symbiodinium from S. pistillata as depth increases to 30 m (Gattuso et al. 1993). While more research is required to understand why Chl-a concentration did not appear to increase with depth in our study, and the ubiquity of our results in terms of other depth profiles and reefs, our results, nevertheless, remain a useful baseline for future research in this region.
δ15N values of Symbiodinium from S. pistillata remained consistent over the depth gradient. This indicates that any changes in the isotopic composition of coral tissues over depth may be driven by increased heterotrophy as corals reduce their reliance on photosynthesis. Grottoli et al. (2006) found that different species of coral differ in their trophic plasticity depending on environmental factors such as depth, light, and nutrient availability. δ15N values obtained for Symbiodinium here were higher than those previously reported for S. pistillata endosymbionts, but followed the same trend of no enrichment with an increasing depth (Alamaru et al. 2009). This could be explained by different δ15N values of inorganic nitrogen sources present, although this was not explicitly tested in our study. Further studies should increase the depth range at which corals are collected and assess the nitrogen and carbon isotopic composition of sympatric symbiotic corals to determine whether the patterns observed here apply to all endosymbionts.
Symbiodinium δ13C values in this study did not show a decrease with an increasing depth. Our results support previous studies where no significant depletion in the δ13C of Symbiodinium was observed above 30-m depth (Einbinder et al. 2009). Although previous studies have documented depletions in the δ13C values of Symbiodinium and coral tissue with an increasing depth (Muscatine et al. 1989; Alamaru et al. 2009), the observed depletion trends were significant only from 15- to 60-m depth. The constant δ13C values of Symbiodinium from S. pistillata along the depth gradient align with the concept of recycling carbon sources between the coral host and its symbionts (Reynaud et al. 2009). Alternatively, a high rate of carbon and nitrogen recycling between the coral host and Symbiodinium may occur as depth increases, which could result in lower isotopic fractionation (Einbinder et al. 2009). Symbiodinium may have been using CO2 from the coral host for photosynthesis rather than externally available carbon sources in our study. We conclude that depleted and constant δ13C values of Symbiodinium suggest that the carbon source might be sourced from both photosynthesis and the coral host.
Implications
Our research is one of the first studies to successfully show that SIA can be used to detect changes in nitrogen and carbon signatures for primary producers to discern between functional roles on shallow tropical reefs. Although this study was small in scope, its uniqueness sets the scene for future, more comprehensive research on detection of carbon and nitrogen nutritional signatures on primary producers in coral reefs more broadly. Understanding the origins of such nutrients on coral reefs and how uptake may vary in relation to nutrient availability and environmental factors may be key in understanding drivers behind bright and dark spots on coral reefs (Cinner et al. 2016).
Acknowledgements
We thank Sarah Collison for assistance with sample collection, Lydiane Mattio with macroalgal species identification, Aashi Parikh for assistance in the laboratory, and the Marine Ecology Group at Macquarie University for collegial support.
References
Alamaru, A, Loya, Y, Brokovich, E, Yam, R, and Shemesh, A (2009). Carbon and nitrogen utilization in two species of Red Sea corals along a depth gradient: insights from stable isotope analysis of total organic material and lipids. Geochimica et Cosmochimica Acta 73, 5333–5342.| Carbon and nitrogen utilization in two species of Red Sea corals along a depth gradient: insights from stable isotope analysis of total organic material and lipids.Crossref | GoogleScholarGoogle Scholar |
Anthony, KRN, and Fabricius, KE (2000). Shifting roles of heterotrophy and autotrophy in coral energetics under varying turbidity. Journal of Experimental Marine Biology and Ecology 252, 221–253.
| Shifting roles of heterotrophy and autotrophy in coral energetics under varying turbidity.Crossref | GoogleScholarGoogle Scholar |
Baker, AC (2003). Flexibility and specificity in coral-algal symbiosis: diversity, ecology, and biogeography of Symbiodinium. Annual Review of Ecology, Evolution, and Systematics 34, 661–689.
| Flexibility and specificity in coral-algal symbiosis: diversity, ecology, and biogeography of Symbiodinium.Crossref | GoogleScholarGoogle Scholar |
Bates, D, Mächler, M, Bolker, B, and Walker, S (2015). Fitting linear mixed-effects models using lme4. Journal of Statistical Software 67, 1–48.
| Fitting linear mixed-effects models using lme4.Crossref | GoogleScholarGoogle Scholar |
Baums, IB, Baker, AC, Davies, SW, Grottoli, AG, Kenkel, CD, Kitchen, SA, Kuffner, IB, LaJeunesse, TC, Matz, MV, Miller, MW, Parkinson, JE, and Shantz, AA (2019). Considerations for maximizing the adaptive potential of restored coral populations in the western Atlantic. Ecological Applications 29, e01978.
| Considerations for maximizing the adaptive potential of restored coral populations in the western Atlantic.Crossref | GoogleScholarGoogle Scholar |
Brandl, SJ, Rasher, DB, Côté, IM, Casey, JM, Darling, ES, Lefcheck, JS, and Duffy, JE (2019). Coral reef ecosystem functioning: eight core processes and the role of biodiversity. Frontiers in Ecology and the Environment 17, 445–454.
| Coral reef ecosystem functioning: eight core processes and the role of biodiversity.Crossref | GoogleScholarGoogle Scholar |
Buckwell, A, Ware, D, Fleming, C, Smart, JCR, Mackey, B, Nalau, J, and Dan, A (2020). Social benefit cost analysis of ecosystem-based climate change adaptations: a community-level case study in Tanna Island, Vanuatu. Climate and Development 12, 495–510.
| Social benefit cost analysis of ecosystem-based climate change adaptations: a community-level case study in Tanna Island, Vanuatu.Crossref | GoogleScholarGoogle Scholar |
Burke LM, Reytar K, Spalding M, Perry A (2012) ‘Reefs at risk revisited in the coral triangle.’ (World Resources Institute)
Carricart-Ganivet, JP, Beltrán-Torres, AU, Merino, M, and Ruiz-Zárate, MA (2000). Skeletal extension, density and calcification rate of the reef building coral Montastraea annularis (Ellis and Solander) in the Mexican Caribbean. Bulletin of Marine Science 66, 215–224.
Chan, NCS, Wangpraseurt, D, Kühl, M, and Connolly, SR (2016). Flow and coral morphology control coral surface pH: implications for the effects of ocean acidification. Frontiers in Marine Science 3, 10.
| Flow and coral morphology control coral surface pH: implications for the effects of ocean acidification.Crossref | GoogleScholarGoogle Scholar |
Cinner, JE, Huchery, C, MacNeil, MA, Graham, NAJ, McClanahan, TR, Maina, J, Maire, E, Kittinger, JN, Hicks, CC, Mora, C, Allison, EH, D’Agata, S, Hoey, A, Feary, DA, Crowder, L, Williams, ID, Kulbicki, M, Vigliola, L, Wantiez, L, Edgar, G, Stuart-Smith, RD, Sandin, SA, Green, AL, Hardt, MJ, Beger, M, Friedlander, A, Campbell, SJ, Holmes, KE, Wilson, SK, Brokovich, E, Brooks, AJ, Cruz-Motta, JJ, Booth, DJ, Chabanet, P, Gough, C, Tupper, M, Ferse, SCA, Sumaila, UR, and Mouillot, D (2016). Bright spots among the world’s coral reefs. Nature 535, 416–419.
| Bright spots among the world’s coral reefs.Crossref | GoogleScholarGoogle Scholar |
Cloern, JE, Canuel, EA, and Harris, D (2002). Stable carbon and nitrogen isotope composition of aquatic and terrestrial plants of the San Francisco Bay estuarine system. Limnology and Oceanography 47, 713–729.
| Stable carbon and nitrogen isotope composition of aquatic and terrestrial plants of the San Francisco Bay estuarine system.Crossref | GoogleScholarGoogle Scholar |
Costanzo, SD, O’donohue, MJ, Dennison, WC, Loneragan, NR, and Thomas, M (2001). A new approach for detecting and mapping sewage impacts. Marine Pollution Bulletin 42, 149–156.
| A new approach for detecting and mapping sewage impacts.Crossref | GoogleScholarGoogle Scholar |
Einbinder, S, Mass, T, Brokovich, E, Dubinsky, Z, Erez, J, and Tchernov, D (2009). Changes in morphology and diet of the coral Stylophora pistillata along a depth gradient. Marine Ecology Progress Series 381, 167–174.
| Changes in morphology and diet of the coral Stylophora pistillata along a depth gradient.Crossref | GoogleScholarGoogle Scholar |
Eurich, JG, Matley, JK, Baker, R, McCormick, MI, and Jones, GP (2019). Stable isotope analysis reveals trophic diversity and partitioning in territorial damselfishes on a low-latitude coral reef. Marine Biology 166, 17.
| Stable isotope analysis reveals trophic diversity and partitioning in territorial damselfishes on a low-latitude coral reef.Crossref | GoogleScholarGoogle Scholar |
Ezzat, L, Fine, M, Maguer, J-F, Grover, R, and Ferrier-Pagès, C (2017). Carbon and nitrogen acquisition in shallow and deep holobionts of the scleractinian coral S. pistillata. Frontiers in Marine Science 4, 102.
| Carbon and nitrogen acquisition in shallow and deep holobionts of the scleractinian coral S. pistillata.Crossref | GoogleScholarGoogle Scholar |
Fabricius, KE (2005). Effects of terrestrial runoff on the ecology of corals and coral reefs: review and synthesis. Marine Pollution Bulletin 50, 125–146.
| Effects of terrestrial runoff on the ecology of corals and coral reefs: review and synthesis.Crossref | GoogleScholarGoogle Scholar |
Fry B, Sherr EB (1989) δ13C measurements as indicators of carbon flow in marine and freshwater ecosystems. In ‘Stable isotopes in ecological research’. (Eds PW Rundel, JR Ehleringer, KA Nagy) pp. 196–229. (Springer)
Gattuso, J-P, Yellowlees, D, and Lesser, M (1993). Depth- and light-dependent variation of carbon partitioning and utilization in the zooxanthellate scleractinian coral Stylophora pistillata. Marine Ecology Progress Series 92, 267–276.
| Depth- and light-dependent variation of carbon partitioning and utilization in the zooxanthellate scleractinian coral Stylophora pistillata.Crossref | GoogleScholarGoogle Scholar |
Grottoli, AG, Rodrigues, LJ, and Juarez, C (2004). Lipids and stable carbon isotopes in two species of Hawaiian corals, Porites compressa and Montipora verrucosa, following a bleaching event. Marine Biology 145, 621–631.
| Lipids and stable carbon isotopes in two species of Hawaiian corals, Porites compressa and Montipora verrucosa, following a bleaching event.Crossref | GoogleScholarGoogle Scholar |
Grottoli, AG, Rodrigues, LJ, and Palardy, JE (2006). Heterotrophic plasticity and resilience in bleached corals. Nature 440, 1186–1189.
| Heterotrophic plasticity and resilience in bleached corals.Crossref | GoogleScholarGoogle Scholar |
Hatcher, BG (1990). Coral reef primary productivity. A hierarchy of pattern and process. Trends in Ecology & Evolution 5, 149–155.
| Coral reef primary productivity. A hierarchy of pattern and process.Crossref | GoogleScholarGoogle Scholar |
Hoegh-Guldberg, O, and Williamson, J (1999). Availability of two forms of dissolved nitrogen to the coral Pocillopora damicornis and its symbiotic zooxanthellae. Marine Biology 133, 561–570.
| Availability of two forms of dissolved nitrogen to the coral Pocillopora damicornis and its symbiotic zooxanthellae.Crossref | GoogleScholarGoogle Scholar |
Hoey, AS, Howells, E, Johansen, JL, Hobbs, J-PA, Messmer, V, McCowan, DM, Wilson, SK, and Pratchett, MS (2016). Recent advances in understanding the effects of climate change on coral reefs. Diversity 8, 12.
| Recent advances in understanding the effects of climate change on coral reefs.Crossref | GoogleScholarGoogle Scholar |
House, JE, Brambilla, V, Bidaut, LM, Christie, AP, Pizarro, O, Madin, JS, and Dornelas, M (2018). Moving to 3D: relationships between coral planar area, surface area and volume. PeerJ 6, e4280.
| Moving to 3D: relationships between coral planar area, surface area and volume.Crossref | GoogleScholarGoogle Scholar |
Hurd CL, Harrison PJ, Bischof K, Lobban CS (2014) ‘Seaweed ecology and physiology.’ (Cambridge University Press)
Jaschinski, S, Brepohl, DC, and Sommer, U (2008). Carbon sources and trophic structure in an eelgrass Zostera marina bed, based on stable isotope and fatty acid analyses. Marine Ecology Progress Series 358, 103–114.
| Carbon sources and trophic structure in an eelgrass Zostera marina bed, based on stable isotope and fatty acid analyses.Crossref | GoogleScholarGoogle Scholar |
Jeffrey, SW, and Humphrey, GF (1975). New spectrophotometric equations for determining chlorophylls a, b, c1 and c2 in higher plants, algae and natural phytoplankton. Biochemie und Physiologie der Pflanzen 167, 191–194.
| New spectrophotometric equations for determining chlorophylls a, b, c1 and c2 in higher plants, algae and natural phytoplankton.Crossref | GoogleScholarGoogle Scholar |
Johnson, MD, Fox, MD, Kelly, ELA, Zgliczynski, BJ, Sandin, SA, and Smith, JE (2020). Ecophysiology of coral reef primary producers across an upwelling gradient in the tropical central Pacific. PLoS ONE 15, e0228448.
| Ecophysiology of coral reef primary producers across an upwelling gradient in the tropical central Pacific.Crossref | GoogleScholarGoogle Scholar |
Koop, K, Booth, D, Broadbent, A, Brodie, J, Bucher, D, Capone, D, Coll, J, Dennison, W, Erdmann, M, Harrison, P, Hoegh-Guldberg, O, Hutchings, P, Jones, GB, Larkum, AWD, O’Neil, J, Steven, A, Tentori, E, Ward, S, Williamson, J, and Yellowlees, D (2001). ENCORE: the effect of nutrient enrichment on coral reefs. Synthesis of results and conclusions. Marine Pollution Bulletin 42, 91–120.
| ENCORE: the effect of nutrient enrichment on coral reefs. Synthesis of results and conclusions.Crossref | GoogleScholarGoogle Scholar |
Koweek, DA, Forden, A, Albright, R, Takeshita, Y, Mucciarone, DA, Ninokawa, A, and Caldeira, K (2019). Carbon isotopic fractionation in organic matter production consistent with benthic community composition across a coral reef flat. Frontiers in Marine Science 5, 520.
| Carbon isotopic fractionation in organic matter production consistent with benthic community composition across a coral reef flat.Crossref | GoogleScholarGoogle Scholar |
Lapointe, BE (1997). Nutrient thresholds for bottom-up control of macroalgal blooms on coral reefs in Jamaica and southeast Florida. Limnology and Oceanography 42, 1119–1131.
| Nutrient thresholds for bottom-up control of macroalgal blooms on coral reefs in Jamaica and southeast Florida.Crossref | GoogleScholarGoogle Scholar |
Lapointe, BE, Barile, PJ, Littler, MM, and Littler, DS (2005). Macroalgal blooms on southeast Florida coral reefs: II. Cross-shelf discrimination of nitrogen sources indicates widespread assimilation of sewage nitrogen. Harmful Algae 4, 1106–1122.
| Macroalgal blooms on southeast Florida coral reefs: II. Cross-shelf discrimination of nitrogen sources indicates widespread assimilation of sewage nitrogen.Crossref | GoogleScholarGoogle Scholar |
Lesser, MP, Slattery, M, Stat, M, Ojimi, M, Gates, RD, and Grottoli, A (2010). Photoacclimatization by the coral Montastraea cavernosa in the mesophotic zone: light, food, and genetics. Ecology 91, 990–1003.
| Photoacclimatization by the coral Montastraea cavernosa in the mesophotic zone: light, food, and genetics.Crossref | GoogleScholarGoogle Scholar |
Marconi, M, Giordano, M, and Raven, JA (2011). Impact of taxonomy, geography, and depth on δ13C and δ15N variation in a large collection of macroalgae. Journal of Phycology 47, 1023–1035.
| Impact of taxonomy, geography, and depth on δ13C and δ15N variation in a large collection of macroalgae.Crossref | GoogleScholarGoogle Scholar |
Marsh, JA (1970). Primary productivity of reef-building calcareous red algae. Ecology 51, 255–263.
| Primary productivity of reef-building calcareous red algae.Crossref | GoogleScholarGoogle Scholar |
Mass, T, Einbinder, S, Brokovich, E, Shashar, N, Vago, R, Erez, J, and Dubinsky, Z (2007). Photoacclimation of Stylophora pistillata to light extremes: metabolism and calcification. Marine Ecology Progress Series 334, 93–102.
| Photoacclimation of Stylophora pistillata to light extremes: metabolism and calcification.Crossref | GoogleScholarGoogle Scholar |
McMahon, KW, Thorrold, SR, Houghton, LA, and Berumen, ML (2016). Tracing carbon flow through coral reef food webs using a compound-specific stable isotope approach. Oecologia 180, 809–821.
| Tracing carbon flow through coral reef food webs using a compound-specific stable isotope approach.Crossref | GoogleScholarGoogle Scholar |
Mollica, NR, Guo, W, Cohen, AL, Huang, K-F, Foster, GL, Donald, HK, and Solow, AR (2018). Ocean acidification affects coral growth by reducing skeletal density. Proceedings of the National Academy of Sciences 115, 1754–1759.
| Ocean acidification affects coral growth by reducing skeletal density.Crossref | GoogleScholarGoogle Scholar |
Mosley, LM, and Aalbersberg, WGL (2003). Nutrient levels in sea and river water along the ‘coral coast’ of Viti Levu, Fiji. The South Pacific Journal of Natural and Applied Sciences 21, 35–40.
| Nutrient levels in sea and river water along the ‘coral coast’ of Viti Levu, Fiji.Crossref | GoogleScholarGoogle Scholar |
Muscatine, L, Porter, JW, and Kaplan, IR (1989). Resource partitioning by reef corals as determined from stable isotope composition. I. δ13C of zooxanthellae and animal tissue vs depth. Marine Biology 100, 185–193.
| Resource partitioning by reef corals as determined from stable isotope composition. I. δ13C of zooxanthellae and animal tissue vs depth.Crossref | GoogleScholarGoogle Scholar |
Muscatine, L, Goiran, C, Land, L, Jaubert, J, Cuif, J-P, and Allemand, D (2005). Stable isotopes (δ13C and δ15N) of organic matrix from coral skeleton. Proceedings of the National Academy of Sciences of the United States of America 102, 1525–1530.
| Stable isotopes (δ13C and δ15N) of organic matrix from coral skeleton.Crossref | GoogleScholarGoogle Scholar |
Owens, NJP (1988). Natural variations in 15N in the marine environment. Advances in Marine Biology 24, 389–451.
| Natural variations in 15N in the marine environment.Crossref | GoogleScholarGoogle Scholar |
Perry, CT, Kench, PS, Smithers, SG, Riegl, B, Yamano, H, and O’Leary, MJ (2011). Implications of reef ecosystem change for the stability and maintenance of coral reef islands. Global Change Biology 17, 3679–3696.
| Implications of reef ecosystem change for the stability and maintenance of coral reef islands.Crossref | GoogleScholarGoogle Scholar |
Poustie, MS, and Deletic, A (2014). Modeling integrated urban water systems in developing countries: case study of Port Vila, Vanuatu. AMBIO 43, 1093–1111.
| Modeling integrated urban water systems in developing countries: case study of Port Vila, Vanuatu.Crossref | GoogleScholarGoogle Scholar |
Raoult, V, Gaston, TF, and Williamson, JE (2015). Not all sawsharks are equal: species of co-existing sawsharks show plasticity in trophic consumption both within and between species. Canadian Journal of Fisheries and Aquatic Sciences 72, 1769–1775.
| Not all sawsharks are equal: species of co-existing sawsharks show plasticity in trophic consumption both within and between species.Crossref | GoogleScholarGoogle Scholar |
Raoult, V, Reid-Anderson, S, Ferri, A, and Williamson, JE (2017). How reliable is structure from motion (SfM) over time and between observers? A case study using coral reef bommies. Remote Sensing 9, 740.
| How reliable is structure from motion (SfM) over time and between observers? A case study using coral reef bommies.Crossref | GoogleScholarGoogle Scholar |
Raven, JA (1997). Inorganic carbon acquisition by marine autotrophs. Advances in Botanical Research 27, 85–209.
| Inorganic carbon acquisition by marine autotrophs.Crossref | GoogleScholarGoogle Scholar |
Reynaud, S, Martinez, P, Houlbrèque, F, Billy, I, Allemand, D, and Ferrier-Pagès, C (2009). Effect of light and feeding on the nitrogen isotopic composition of a zooxanthellate coral: role of nitrogen recycling. Marine Ecology Progress Series 392, 103–110.
| Effect of light and feeding on the nitrogen isotopic composition of a zooxanthellate coral: role of nitrogen recycling.Crossref | GoogleScholarGoogle Scholar |
Risk, MJ, Sammarco, PW, and Schwarcz, HP (1994). Cross-continental shelf trends in δ13C in coral on the Great Barrier Reef. Marine Ecology Progress Series 106, 121–130.
| Cross-continental shelf trends in δ13C in coral on the Great Barrier Reef.Crossref | GoogleScholarGoogle Scholar |
Risk, MJ, Lapointe, BE, Sherwood, OA, and Bedford, BJ (2009). The use of δ15N in assessing sewage stress on coral reefs. Marine Pollution Bulletin 58, 793–802.
| The use of δ15N in assessing sewage stress on coral reefs.Crossref | GoogleScholarGoogle Scholar |
Seemann, J (2013). The use of 13C and 15N isotope labeling techniques to assess heterotrophy of corals. Journal of Experimental Marine Biology and Ecology 442, 88–95.
| The use of 13C and 15N isotope labeling techniques to assess heterotrophy of corals.Crossref | GoogleScholarGoogle Scholar |
Seemann J, Carballo-Bolanos R, Berry KL, González CT, Richter C, Leinfelder RR (2012) Importance of heterotrophic adaptations of corals to maintain energy reserves. In ‘Proceedings of the 12th international coral reef symposium, 9–13 July 2012, Cairns, Qld, Australia’. (Eds D Yellowlees, TP Hughes) ICRS2012_19A_4, pp. 9–13. (International Society for Reef Studies) Available at https://www.icrs2012.com/proceedings/manuscripts/ICRS2012_19A_4.pdf
Sherwood, OA, Jamieson, RE, Edinger, EN, and Wareham, VE (2008). Stable C and N isotopic composition of cold-water corals from the Newfoundland and Labrador continental slope: examination of trophic, depth and spatial effects. Deep Sea Research Part I: Oceanographic Research Papers 55, 1392–1402.
| Stable C and N isotopic composition of cold-water corals from the Newfoundland and Labrador continental slope: examination of trophic, depth and spatial effects.Crossref | GoogleScholarGoogle Scholar |
Sulu R (2007) ‘Status of coral reefs in the Southwest Pacific.’ (IPS Publications, University of the South Pacific)
Swart, PK, Saied, A, and Lamb, K (2005). Temporal and spatial variation in the δ15N and δ13C of coral tissue and zooxanthellae in Montastraea faveolata collected from the Florida reef tract. Limnology and Oceanography 50, 1049–1058.
| Temporal and spatial variation in the δ15N and δ13C of coral tissue and zooxanthellae in Montastraea faveolata collected from the Florida reef tract.Crossref | GoogleScholarGoogle Scholar |
Tanaka, Y, Miyajima, T, Koike, I, Hayashibara, T, and Ogawa, H (2006). Translocation and conservation of organic nitrogen within the coral-zooxanthella symbiotic system of Acropora pulchra, as demonstrated by dual isotope-labeling techniques. Journal of Experimental Marine Biology and Ecology 336, 110–119.
| Translocation and conservation of organic nitrogen within the coral-zooxanthella symbiotic system of Acropora pulchra, as demonstrated by dual isotope-labeling techniques.Crossref | GoogleScholarGoogle Scholar |
Tanaka, Y, Ogawa, H, and Miyajima, T (2010). Effects of nutrient enrichment on the release of dissolved organic carbon and nitrogen by the scleractinian coral Montipora digitata. Coral Reefs 29, 675–682.
| Effects of nutrient enrichment on the release of dissolved organic carbon and nitrogen by the scleractinian coral Montipora digitata.Crossref | GoogleScholarGoogle Scholar |
Tremblay, P, Maguer, JF, Grover, R, and Ferrier-Pages, C (2015). Trophic dynamics of scleractinian corals: stable isotope evidence. Journal of Experimental Biology 218, 1223–1234.
| Trophic dynamics of scleractinian corals: stable isotope evidence.Crossref | GoogleScholarGoogle Scholar |
Umezawa, Y, Miyajima, T, Yamamuro, M, Kayanne, H, and Koike, I (2002). Fine-scale mapping of land-derived nitrogen in coral reefs by δ15N in macroalgae. Limnology and Oceanography 47, 1405–1416.
| Fine-scale mapping of land-derived nitrogen in coral reefs by δ15N in macroalgae.Crossref | GoogleScholarGoogle Scholar |
Wang, W-L, and Yeh, H-W (2003). δ13C values of marine macroalgae from Taiwan. Botanical Bulletin of Academia Sinica 44, 107–112.
Wheeler, PA, and Björnsäter, BR (1992). Seasonal fluctuations in tissue nitrogen, phosphorus, and N:P for five macroalgal species common to the pacific northwest coast. Journal of Phycology 28, 1–6.
| Seasonal fluctuations in tissue nitrogen, phosphorus, and N:P for five macroalgal species common to the pacific northwest coast.Crossref | GoogleScholarGoogle Scholar |
Williamson, JE, and Rees, TAV (1994). Nutritional interaction in an alga–barnacle association. Oecologia 99, 16–20.
| Nutritional interaction in an alga–barnacle association.Crossref | GoogleScholarGoogle Scholar |
Wong, CWM, Duprey, NN, and Baker, DM (2017). New insights on the nitrogen footprint of a coastal megalopolis from coral-hosted Symbiodinium δ15N. Environmental Science & Technology 51, 1981–1987.
| New insights on the nitrogen footprint of a coastal megalopolis from coral-hosted Symbiodinium δ15N.Crossref | GoogleScholarGoogle Scholar |
Wright, JT, de Nys, R, and Steinberg, PD (2000). Geographic variation in halogenated furanones from the red alga Delisea pulchra and associated herbivores and epiphytes. Marine Ecology Progress Series 207, 227–241.
| Geographic variation in halogenated furanones from the red alga Delisea pulchra and associated herbivores and epiphytes.Crossref | GoogleScholarGoogle Scholar |
Yamamuro, M, Kayanne, H, and Minagawao, M (1995). Carbon and nitrogen stable isotopes of primary producers in coral reef ecosystems. Limnology and Oceanography 40, 617–621.
| Carbon and nitrogen stable isotopes of primary producers in coral reef ecosystems.Crossref | GoogleScholarGoogle Scholar |
Zheng, X, Huang, L, Lin, R, and Du, J (2015). Roles of epiphytes associated with macroalgae in benthic food web of a eutrophic coastal lagoon. Continental Shelf Research 110, 201–209.
| Roles of epiphytes associated with macroalgae in benthic food web of a eutrophic coastal lagoon.Crossref | GoogleScholarGoogle Scholar |
Zimba, PV, and Hopson, MS (1997). Quantification of epiphyte removal efficiency from submersed aquatic plants. Aquatic Botany 58, 173–179.
| Quantification of epiphyte removal efficiency from submersed aquatic plants.Crossref | GoogleScholarGoogle Scholar |
Zweng, RC, Koch, MS, and Bowes, G (2018). The role of irradiance and C-use strategies in tropical macroalgae photosynthetic response to ocean acidification. Scientific Reports 8, 1–11.
| The role of irradiance and C-use strategies in tropical macroalgae photosynthetic response to ocean acidification.Crossref | GoogleScholarGoogle Scholar |