Cyclic electron flow and Photosystem II-less photosynthesis
Maria Ermakova
A
B
C
Abstract
Oxygenic photosynthesis is characterised by the cooperation of two photo-driven complexes, Photosystem II (PSII) and Photosystem I (PSI), sequentially linked through a series of redox-coupled intermediates. Divergent evolution has resulted in photosystems exhibiting complementary redox potentials, spanning the range necessary to oxidise water and reduce CO2 within a single system. Catalysing nature’s most oxidising reaction to extract electrons from water is a highly specialised task that limits PSII’s metabolic function. In contrast, potential electron donors in PSI span a range of redox potentials, enabling it to accept electrons from various metabolic processes. This metabolic flexibility of PSI underpins the capacity of photosynthetic organisms to balance energy supply with metabolic demands, which is key for adaptation to environmental changes. Here, we review the phenomenon of ‘PSII-less photosynthesis’ where PSI functions independently of PSII by operating cyclic electron flow using electrons derived from non-photochemical reactions. PSII-less photosynthesis enables supercharged ATP production and is employed, for example, by cyanobacteria’s heterocysts to host nitrogen fixation and by bundle sheath cells of C4 plants to boost CO2 assimilation. We discuss the energetic benefits of this arrangement and the prospects of utilising it to improve the productivity and stress resilience of photosynthetic organisms.
Keywords: bundle sheath cell, crop improvement, cyclic electron flow, electron transport, heterocyst, nitrogen fixation, photosynthesis, Photosystem I.
A classic view on photosynthesis – the Z scheme
Light reactions of oxygenic photosynthesis involve two photosystems linked by an intersystem electron transport chain including the Cytochrome b6f complex (Cytb6f). This linear electron flow (LEF) is famously presented by a Z scheme (Hill and Bendall 1960), where key intermediates are plotted against their relative redox potential, and the two photosystems are turned on their side to form a ‘Z’ (Fig. 1). Initially, Emerson and Arnold (1932) and Emerson and Lewis (1943) used flashing light to probe the saturation of photosynthetic oxygen production by the unicellular green alga Chlorella. The stoichiometry supported an aggregate of at least 320 chlorophyll (Chl) molecules for each photon absorbed. However, since the red light yielded a higher quantum efficiency when compared to measurements conducted with far-red light, it was suggested that two photochemical centres with somewhat different Chls power two separate photosynthetic units (Emerson 1957). Hill and Bendall (1960) joined the two units, which they called Photosystem II (PSII) and Photosystem I (PSI), through an intersystem electron transport chain involving Cytb6f. They proposed that water splitting, to form oxygen, occurred on the oxidising side and the reduction of NADP+ at the reducing side of the chain, ATP could be produced via ‘downhill’ electron flow through the intermediate pathway between the two photosystems, and then ATP and NADPH could drive CO2 fixation in the Calvin-Benson-Bassham cycle (hereafter Calvin cycle) (Fig. 1).
A classic view on oxygenic photosynthesis. (a) The Z scheme of electron transport. (b) Main photosynthetic electron pathways conserved from cyanobacteria to land plants. PSII, Photosystem II; PQ, plastoquinone; Cytb6f, Cytochrome b6f complex; PC, Plastocyanin; PSI, Photosystem I; FD, Ferredoxin; FNR, Ferredoxin:NADP+ oxidoreductase.
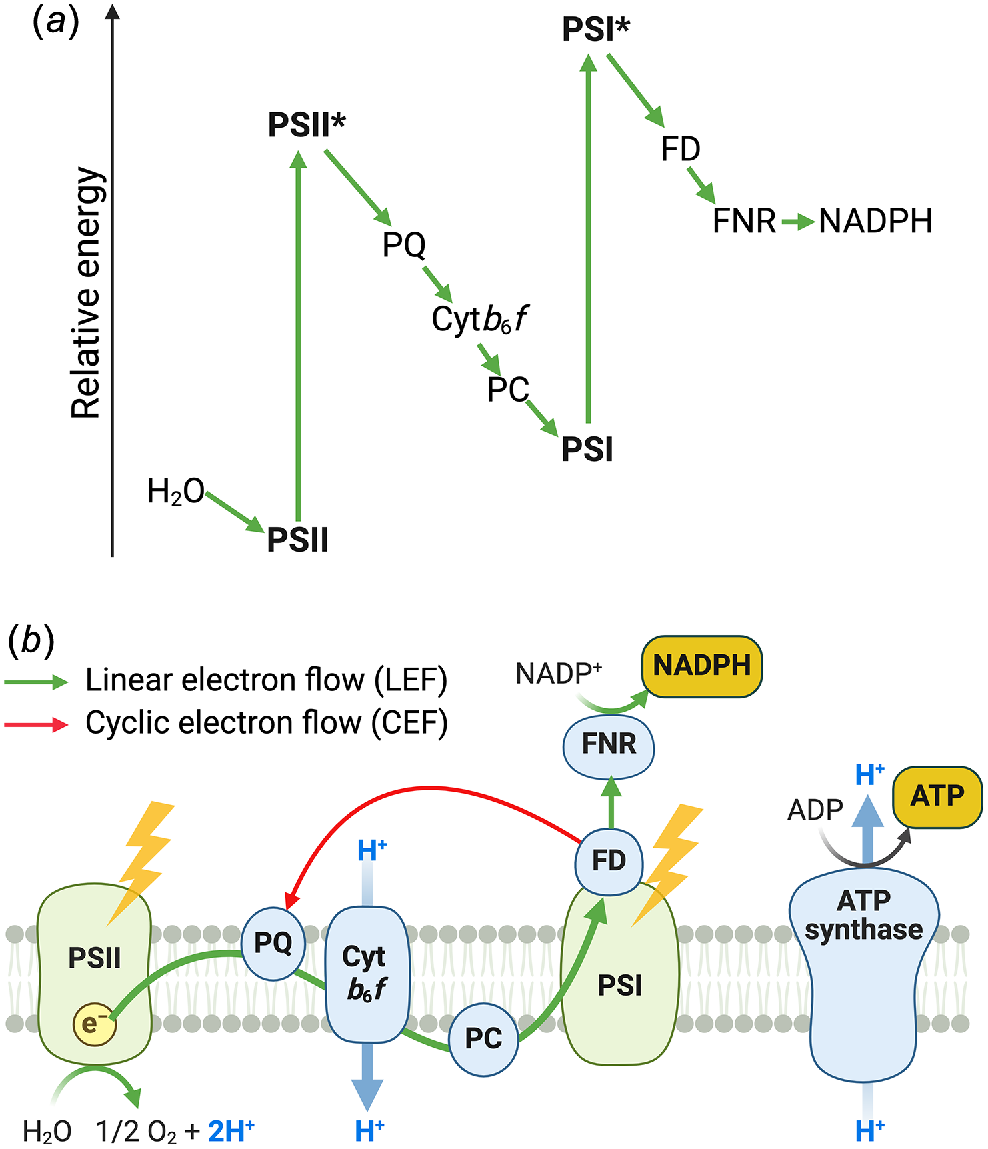
The Z scheme starts with PSII, where a reaction centre P680, formed by a special pair of Chl a molecules with the absorption peak at 680 nm, is oxidised to form P680+ (Fig. 1). The P680+ is nature’s strongest biological oxidising agent capable of splitting water molecules. This occurs on the lumenal side of PSII within the oxygen evolving complex (OEC), which incorporates a Mn4CaO5 cluster. In a four-step reaction, four electrons originating from two water molecules are bound sequentially to OEC, four protons are released into the lumen and one molecule of O2 is evolved as a by-product (Kok et al. 1970). The electron stripped from P680 is transferred by a chain of protein-bound cofactors to a pair of bound plastoquinone (PQ) molecules in the QA/QB pocket, where the electron first reduces QA−/QB before equilibrating to QA/QB−. A second reduction of QA−/QB− leads to the final state where QB accepts a second electron from QA and at the same time accepts two protons from the cytosolic side (or chloroplast stroma in the cases of algae and plants) to form plastoquinol (PQH2). PQH2 is then released from the binding site into the lipid matrix of the thylakoid membrane, being replaced by PQ to form oxidised QA/QB, ready for the next cycle.
In the Z scheme’s second step, PQH2 is oxidised by Cytb6f. Binding of PQH2 to the Qo site of Cytb6f releases two protons into the lumen as one electron is transferred via the Rieske FeS protein/Cytochrome f to reduce a soluble lumenal electron carrier, Plastocyanin (PC) or Cytochrome c6. In the Q-cycle, another electron is returned to PQ in the Qi site of Cytb6f, producing a semiquinone (Sarewicz et al. 2021). Upon accepting a second electron in the next cycle, the semiquinone captures two protons from the cytosolic side of the membrane, gets reduced to PQH2 and migrates to the PQ pool. In this way, the Q-cycle translocates a further 2 H+ per electron from the cytosolic side to the lumen, maximising proton motive force (pmf) across the thylakoid membrane relative to the electron flow. Pmf is then used by ATP synthase for ATP generation (Fig. 1).
In the Z scheme’s third step, the reaction centre of PSI, P700, formed by the second special Chl a pair with the absorption peak at 700 nm, is oxidised and electrons are transferred to soluble electron carriers on the cytosolic side, Ferredoxin (FD) or Flavodoxin. P700+ is reduced by PC or Cytochrome c6 (Fig. 1) while electrons from FD are used for the reduction of NADP+ via FD:NADP+ oxidoreductase (FNR).
The Z scheme presents LEF as an optimal arrangement for sourcing electrons from water, elevating their reducing potential to enable reduction of NADP+ and leveraging electron transport reactions to generate pmf. It also clearly shows how the strong reducing potential of P700* and the highly oxidising potential of P680+ enable combining H2O oxidation and CO2 reduction within a single system. This specialisation of photosystems is what has made the LEF-model of oxygenic photosynthesis so successful that it remained largely unchanged from its evolution in cyanobacteria, through algae and into flowering plants. However, this chemical divergence of the two photosystems also enabled significant metabolic evolution of electron pathways around PSI. Compared to PSII’s unique specialisation in oxidising water, PSI can be reduced from a variety of electron donors (Fig. 1). Due to this metabolic flexibility, PSI can operate cyclic electron flow (CEF) independently of PSII. In CEF, electrons from the reducing side of PSI are shuttled back to the intersystem chain (usually upstream of Cytb6f) to generate additional pmf and increase the ATP/NADPH ratio.
Interestingly, from a research standpoint, discovery of CEF predates the Z scheme: Arnon and co-workers established in the 1950s that isolated chloroplasts could produce ATP using PSI alone (Whatley et al. 1959). This is fitting as CEF could have preceded the evolution of the OEC and the Z scheme and was likely employed by ancient phototrophs to leverage the limited supply of exogenous electrons to generate ATP using solar energy. We next give an overview of the events that led to the appearance of the two photosystems and domination of this arrangement, before exploring how the metabolic flexibility of PSI provides photosynthetic organisms with resilience against environmental stresses.
Evolution of photosystems and oxygenic photosynthesis
As detailed above, oxygenic photosynthesis requires two photoactive Chl-protein macromolecular structures, PSI and PSII (Fig. 1). Sadekar et al. (2006) have demonstrated a convincing structural homology between contemporary photosystems consistent with a common evolutionary origin (see also Orf et al. 2018). Phylogenetic evidence suggests the first organisms on Earth were heterotrophic archaebacteria and bacteria subsisting on organic molecules or hydrogen scavenged from their environment (Martin et al. 2017). The first physical evidence for photosynthetic organisms is found in fossil stromatolites dated to 3.45 bya in which pro-cyanobacteria and cyanobacteria were key organisms (Schopf 2011). However, phylogenetic analysis suggests that photosynthetic organisms preceded these fossils. An early photosynthetic apparatus likely evolved in a precursor to cyanobacteria (Cardona et al. 2019). This photocenter, an ur-photosystem, would have harvested visible light energy through a simple Chl-based set of proteins and was likely geared for ATP formation (Larkum 2006). It is anticipated that anaerobic respiratory electron transport was already operational and therefore cytochromes had already evolved. Indeed, phylogenetic analysis supports the evolution of Cytb6f from the Cytochrome bc1 complex (e.g. see Blankenship 2021). Based on comparative metabolism and genomics of oxygenic and anoxygenic photosynthetic organisms, it is likely that CEF also evolved early (Sánchez-Baracaldo and Cardona 2020).
Both PSI and PSII have evolved from the ur-photosystem either in separate pro-cyanobacteria (Fusion hypothesis) or alongside each other in a single pro-cyanobacterium (Fission hypothesis) (Larkum 2006). A crucial step in the differentiation of functions between the two photosystems was to allow for use of an electron donor such as hydrogen peroxide to PSII, initiating oxygen evolution. This enabled a unidirectional photoactivated flow of electrons from PSII to PSI. All this occurred during the period between 3.8 and 3.5 bya whereupon organisms could be identified as cyanobacteria or pro-cyanobacteria; fully functional cyanobacteria are those that evolved the sophisticated water splitting incorporating a Mn4CaO5 cluster (Cardona et al. 2019; Sánchez-Baracaldo and Cardona 2020). Therefore, cyanobacteria represent a lineage of photosynthetic organisms stretching from possibly 3.8 bya to the present time.
The exact process by which oxygen evolution evolved remains unknown, but there must have been significant selection pressure to use water as the electron donor. Prior to this, energy sources were limited to a relatively short supply of exogenous inorganic and organic compounds. This energetic constraint hindered the diversification and abundance of organisms on the early Earth, effectively preventing the evolution of complex life. The use of water as the primary electron donor massively boosted evolution on the Earth by simultaneously providing an almost unlimited energy resource and supplying molecular oxygen. Although the latter led to the first large extinction of species during the Great Oxygenation Event, it provided a high redox potential for respiration allowing for the extraction of vast amounts of energy from organic substrates.
Between 2.45 and 1.8 bya, endosymbiosis of a cyanobacterium into a eukaryote cell that already had mitochondria first occurred (Fig. 2). At that time, three extant types of simple photosynthetic eukaryotes evolved: (1) Glaucophytes; (2) Rhodophytes; and (3) Chlorophytes (Howe et al. 2008). Primary endosymbiosis gave rise to chloroplasts (plastids) with two membranes: one belonging to the cyanobacterium; and the other coming from an investing membrane of the host cytoplasm. After the primary endosymbiosis, secondary and tertiary endosymbiosis events occurred. This facilitated evolution of complex chloroplasts with more than two envelope membranes, giving rise to many other algal phyla and eventually to land plants (Larkum 2020). The CEF mechanisms evolved in cyanobacteria have made their way into Archaeplastida through the primary endosymbiosis and have further diversified over the course of evolution of algae and embryophytic plants (Fig. 2).
Cyanobacteria have given origin to chloroplasts in an endosymbiosis event. Electron transport pathways of chloroplasts can be tracked back to cyanobacteria where the photosynthetic electron transport chain, including linear and multiple cyclic electron flow pathways, and respiratory electron transport chain are hosted by the same membranes and share electron carriers. Green arrow, linear electron flow; red arrow, cyclic electron flow; blue arrow, (chloro)respiration; PSII, Photosystem II; PQ, plastoquinone; PSI, Photosystem I.
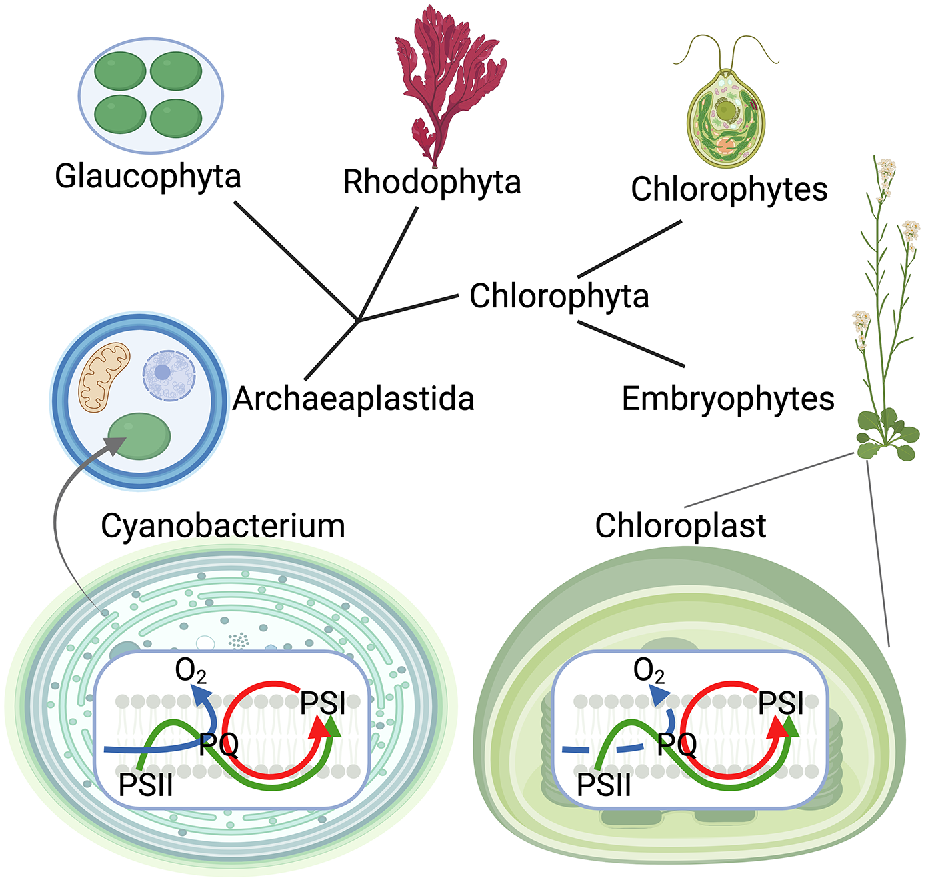
Overview of CEF and CEF-like pathways
LEF produces ATP and NADPH at a fixed rate, which due to a varying number of c subunits of ATP synthase in oxygenic photosynthetic organisms (between 13 and 15), ranges between 2.4 and 2.77 (Turina et al. 2016; Davis and Kramer 2020). Independent of the exact stoichiometry, these numbers fall short of the metabolic needs of photosynthetic organisms. Calvin cycle alone requires 3 ATP per 2 NADPH and the ATP demand is further increased by processes such as photorespiration, starch synthesis, transcription, translation, membrane transport, and repair of photodamaged PSII (Kramer and Evans 2011; Strand and Walker 2023). Operating CO2 concentrating mechanism (CCM) in cyanobacteria, algae and C4 plants also comes with the cost of additional ATP: in C4 plants, at least five molecules of ATP per two molecules of NADPH are required for CO2 fixation (Ermakova et al. 2020). CEF and CEF-like pathways are employed by photosynthetic organisms to increase ATP yield as well as to dynamically adjust the ATP/NADPH ratio generated by the light reactions to match the changing requirements of cellular metabolic processes (Fig. 3).
A diversity of cyclic electron flow (CEF) and pseudo-CEF pathways in oxygenic photosynthetic organisms. (a) NDH-CEF: pathways mediated by type 1 NADH dehydrogenase-like complex (NDH-1) or type 2 NAD(P)H dehydrogenase (NDH-2). (b) PGR-CEF: an antimycin A-sensitive route regulated by the PROTON GRADIENT REGULATION5 (PGR5) and PGR5-LIKE PHOTOSYNTHETIC PHENOTYPE 1 (PGRL1). (c) Pseudo-CEF pathways increase the ATP/NADPH ratio through lowering the net NADPH production (see details in the text): (A) Flavodiiron proteins (Flv), (B) Mehler Ascorbate Peroxidase pathway, (C) the malate valve, (D) chlororespiration. PQ, plastoquinone; Cytb6f, Cytochrome b6f; PC, Plastocyanin; PSI, Photosystem I; FD, Ferredoxin; FNR, Ferredoxin:NADP+ oxidoreductase; MDH, NADPH-dependent malate dehydrogenase; PTOX, plastid terminal oxidase.
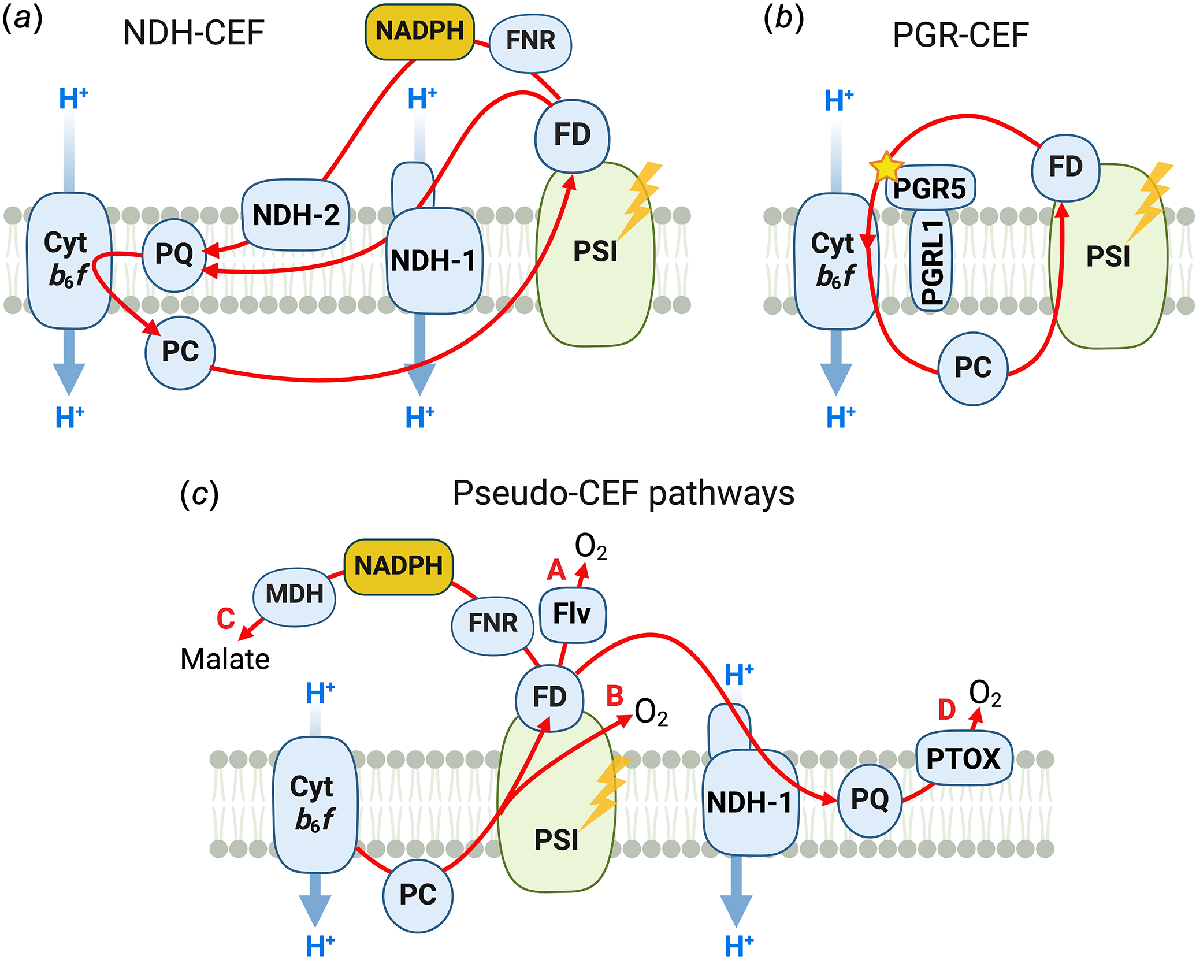
Energetically, CEF around PSI involves downhill electron flow from the photo-reduced low potential end of PSI (P700 → Chl a (A) → Chl a (Ao) → phylloquinone A1 → Fe4S4 complexes Fx, FA and FB → FD), to the photo-oxidised high potential end of PSI. The high-potential components of CEF (PQ, Cytb6f, PC, and P700+) are shared with LEF. Three potential routes have been identified that could fulfil the role of the FD:PQ oxidoreductase: (1) type 1 NADH dehydrogenase-like complex (NDH-1) or type II NAD(P)H dehydrogenase (NDH-2, Peltier et al. 2016); (2) antimycin A-sensitive pathway regulated by PROTON GRADIENT REGULATION 5 (PGR5) and PGR5-LIKE PHOTOSYNTHETIC PHENOTYPE 1 (PGRL1) (Munekage et al. 2002; Hertle et al. 2013); and (3) a direct transfer of electrons from FD to Cytb6f (Malone et al. 2021).
NDH pathways
Evolutionarily, NDH-1 and NDH-2 are present in a wide variety of photosynthetic organisms from cyanobacteria to embryophytic plants. NDH-1 (sometimes called Photosynthetic Complex 1) is a homologue of the H+-pumping Complex 1 of the inner mitochondrial membrane. NDH-1 a transmembrane complex, which oxidises FD and reduces PQ whilst increasing pmf by pumping 2 H+ per electron from the stroma (or cytosol in cyanobacteria) to the thylakoid lumen (Pan et al. 2020; Zhang et al. 2020). This makes the NDH-1-mediated CEF route the most efficient for generating additional ATP, whilst other CEF pathways only result in proton translocation by Cytb6f (Strand et al. 2017). This complex is therefore indispensable in conditions of high ATP demand (Livingston et al. 2010; Nakano et al. 2019; Ermakova et al. 2024).
In other bacteria apart from cyanobacteria, a number of NDH-1 occur, and it is likely that NDH-1 of cyanobacteria evolved from those (Shikanai 2016). NDH-1 is a complex membrane-spanning protein assemblage; even at the most basic level, NDH-1 is made up of 14 subunits (Peltier et al. 2016; Laughlin et al. 2020). For the physiological function, it is necessary to have several other subunits that form a hydrophilic domain and several subunits, which form a hydrophobic domain. Depending on the accessory subunits, NDH-1 can perform multiple functions (Battchikova et al. 2011). For example, NDH-1MS (also known as NDH-13) has a carbonic anhydrase-like module that allows the complex to perform the energised conversion of CO2 to HCO3−, limiting CO2 leakage to the external medium (Schuller et al. 2020). Recent structural models of NDH-1 from Thermosynechococcus elongatus resolved details of the FD-binding site involving NdhS, NdhO and NdhH subunits (Laughlin et al. 2019; Schuller et al. 2020).
The cyanobacterial NDH-1 is ancestral to the chloroplast NADH dehydrogenase-like complex (Burrows et al. 1998; Shikanai 2016), which is referred to as NDH hereafter. In embryophytes, NDH forms a supercomplex with PSI (Peng et al. 2009). NDH-1 is present in cyanobacteria, some algae (Prasinophyceae and the Charophyta), and land plants (Bryophyta, some gymnosperms, and most flowering plants). It is absent from the most algal groups (the Glaucophyta, Rhodophyta, Chlorophyceae, Trebouxiophyceae, Euglenophyta, Bacillariophyceae, Cryptophyta), some gymnosperms and a few flowering plants (Martín and Sabater 2010). As discussed by Zhou et al. (2022), the presence or absence of PGR5-PGRL1, plastid terminal oxidase (PTOX), NDH-2 and other complexes involved in electron transport or translocation of protons can modulate the need for NDH-1.
The thylakoids of most of the oxygenic photosynthetic organisms examined (e.g. cyanobacteria, Chlamydomonas reinhardtii, Symbiodiniaceae, Physcomitrella, Arabidopsis thaliana) also have the non-H+-pumping NDH-2, a homologue of the mitochondrial non-H+-pumping alternative to Complex I (Peltier et al. 2016). NDH-2 is a single polypeptide of around 50 kDa, which resides on the stromal side of the thylakoid membrane; it oxidises NAD(P)H and reduces PQ (Nawrocki et al. 2019a). The flux through NDH-2 is low, and the three NDH-2 homologues in cyanobacteria might rather have a regulatory function in mixotrophic conditions (Howitt et al. 1999; Cooley and Vermaas 2001; Huokko et al. 2017; Huokko et al. 2019). Many green algae lack NDH-1 but have NDH-2 (Jans et al. 2008). At least two NDH-2 are thylakoid-localised in C. reinhardtii, and one of them, NDA2, was shown to reduce PQ by oxidising both NADH and NADPH (Desplats et al. 2009; Terashima et al. 2010). NDA2 is involved in a variety of light-driven processes such as state transitions, CEF and anaerobic hydrogen photoproduction (Jans et al. 2008; Johnson and Alric 2013; Saroussi et al. 2016).
PGR pathway
PGR5-PGRL1 have long been proposed as an alternative system for the low potential components of CEF, forming a so-called antimycin A-sensitive CEF pathway. These proteins are found in all eukaryote oxygenic photosynthetic organisms (Munekage et al. 2002; Yamori and Shikanai 2016) and homologues are present in cyanobacteria (Yeremenko et al. 2005; Dann and Leister 2019). The naming of PGR5 and PGRL1 relates to their role in controlling the transmembrane proton gradient (pH). PGR5 was first identified from an Arabidopsis mutant suffering a major disruption in its ability to generate pH across the thylakoid membrane (Munekage et al. 2002). PGRL1 has also been identified first in Arabidopsis and then in C. reinhardtii (DalCorso et al. 2008; Petroutsos et al. 2009).
The antimycin A-sensitive CEF was originally discovered long ago in plant thylakoids in a study of FD-dependent ATP synthesis (Tagawa et al. 1963). Antimycin A was initially used as an inhibitor of mitochondrial electron and proton transport: in bacteria and mitochondria, it binds to the Qi site of Cytochrome bc1, which inhibits the oxidation of ubiquinol and prevents turnover of the Q cycle (Huang et al. 2005). Whilst the exact mechanism of antimycin A inhibition of photosynthetic electron transport is still a matter of speculation, PGR5 is considered the site of action, among other reasons, because a single point mutation in PGR5 confers resistance to antimycin A (Sugimoto et al. 2013). In line with this, algae C. reinhardtii has a distinct CEF supercomplex consisting of PSI and Cytb6f but not PGR5 that is antimycin A-insensitive (Iwai et al. 2010; Steinbeck et al. 2018).
Since both the action of antimycin A and PGR5-PGRL1 can be traced back to cyanobacteria (Yeremenko et al. 2005; Dann and Leister 2019), both NDH- and PGR-CEF systems have evolved at the earliest stages of oxygenic photosynthesis and were inherited by algae and embryophytes. However, an early function of PGR5 could be in iron uptake (Leister et al. 2022) and the importance of PGR5-PGRL1 in CEF has greatly increased during the evolution of terrestrial plants (Suorsa et al. 2012; Allahverdiyeva et al. 2015; Woodford et al. 2024). Whilst an interaction of PGR5-PGRL1 with FD has been confirmed (Hertle et al. 2013), there is no conclusive evidence that PGR5 or PGRL1 are directly involved in electron transfer (Rühle et al. 2021). In C. reinhardtii, PGRL1 is co-isolated with the CEF supercomplex, yet the deletion of PGRL1 does not hinder the maximum rate of CEF (Nawrocki et al. 2019a, 2019b). This has prompted a suggestion that PGR5-PGRL1 aids in regulating the direct FD:Cytb6f oxidoreduction mentioned earlier as the third possible CEF route (Buchert et al. 2020). For details of a potential mechanism of the direct electron transfer from FD to Cytb6f, see Malone et al. (2021). This is an area of active ongoing research, and the physiological contribution of this mechanism in different photosynthetic organisms is still unresolved.
The PGR-CEF route plays a critical role in photoprotection because it is required to build-up ΔpH and activate the energy dependent non-photochemical quenching (NPQ), which initiates the dissipation of excess light absorbed in the PSII antennae as heat (Müller et al. 2001; Munekage et al. 2002; Cruz et al. 2005). However, the contribution of PGR5-PGRL1 to ATP generation is debated. Engineering Arabidopsis chloroplasts with fluorescent ATP sensors revealed an altered kinetics of ATP production in the absence of PGR5 but was overall inconclusive (Sato et al. 2019). Nevertheless, the fact that PGR5-PGRL1 is associated with avoiding photosynthetic oscillations during light transitions corroborates its function in adjusting the ATP/NADPH ratio (Degen et al. 2024; Shikanai 2024).
Pseudo-CEF pathways
In addition to CEF pathways, which directly increase pmf, and therefore ATP yield by returning electrons from the low to high potential end of PSI, there are multiple pathways, which could be collectively called ‘pseudo-CEF’ (Fig. 3). These pathways provide an electron sink at the PSI acceptor side and increase pmf, and thus the ATP/NADPH ratio, by lowering NADPH production. Through dynamically regulating the ATP/NADPH ratio, these pathways (A–D) play significant roles in photoprotection in different photosynthetic organisms.
Flavodiiron proteins (Flvs) are soluble proteins that were originally recognised in a range of strict or facultative anaerobic bacteria and archaea where they perform detoxification of oxygen or nitric oxide (Wasserfallen et al. 1998). In cyanobacteria, Flvs are primarily involved in photoprotection of PSI and PSII from photoinhibition under high and fluctuating light by directing excess electrons to O2 and reducing it to water (Allahverdiyeva et al. 2011; Santana-Sanchez et al. 2019; Santana-Sánchez et al. 2023). Algae gained Flvs through endosymbiosis and the proteins are present in all algal species analysed so far, including C. reinhardtii (Jokel et al. 2015; Ilík et al. 2017). There is accumulating evidence that Flvs are present in land plants as far as gymnosperms but not in angiosperms (Gerotto et al. 2016; Ilík et al. 2017; Bag et al. 2023). Flv-mediated pseudo-CEF likely contributes to ATP generation in cyanobacteria (Thiel et al. 2019).
Mehler Ascorbate Peroxidase (MAP) pathway, also known as the water–water cycle, involves the transfer of electrons from the reducing side of PSI to molecular oxygen, resulting in the formation of superoxide radicals, which are subsequently reduced to peroxide and then to water (Asada 2000). The pathway typically functions as a safety valve limiting the accumulation of hydrogen peroxide, when Calvin cycle is not fully functional and electrons accumulate on FD. The extent of activity of this pathway has long been under debate but it is likely to play a role during the start of photosynthesis on turning on the light or in the shade at low CO2 (Badger et al. 2000; Sagun et al. 2021). The MAP’s role in regulating the accumulation of H2O2 is proposed to contribute to the chloroplast retrograde signalling by helping chloroplasts to distinguish between brief high light events, such as sun flecks, and persisting high light stress (Fitzpatrick et al. 2022).
The malate valve in higher plants is discussed in detail by Selinski and Scheibe (2019). In this pathway, oxaloacetate within the chloroplast is reduced by NADPH-dependent malate dehydrogenase. The resulting malate is transported out of the chloroplast and may enter mitochondria where it is oxidised with the production of ATP. The net result is therefore an increase of ATP levels at the expense of NADPH in the chloroplast and potentially also ATP generated in mitochondria. Whilst the malate valve can generate more ATP than other pseudo-CEF pathways, the additional ATP is produced in the different compartment and it’s activity is likely limited to low light (Walker et al. 2020).
Chlororespiration is a respiratory electron transport pathway in chloroplasts that involves NDH-1 or NDH-2 and PTOX (Peltier and Cournac 2002). In this pathway, NDH systems accept electrons from FD or NADPH (which might originate either from PSI or from oxidation of stromal reductants) and reduce PQ, which is then oxidised by PTOX reducing O2. PTOX does not contribute to pmf so chlororespiration does not produce ATP unless NDH-1 is involved. Therefore, this pathway functions as an electron sink and carries a small electron load relative to LEF in mature chloroplasts under optimal conditions, but has a more important role under stress, in developing chloroplasts, and in non-photosynthetic plastids (Stepien and Johnson 2018; Zhou et al. 2021; Zolotareva and Polishchuk 2022). It was recently suggested that in plants, PTOX specifically protects PSI from inhibition while negatively impacting PSII activity at high light (Messant et al. 2024). Through adjusting the PQ/PQH2 ratio, chlororespiration can influence the ratio of excitation energy allocated to PSII vs PSI (state transitions) and enable growth of C. reinhardtii under intermittent light (Nawrocki et al. 2019c). The term chlororespiration is sometimes used synonymously with the non-photochemical reduction of the PQ pool. However, these are not interchangeable: although the non-photochemical reduction of PQ represents the initial part of the chlororespiratory pathway, to be called chlororespiration, electron route must end at a terminal oxidase.
Which CEF route is dominant?
While measuring the rate of CEF through PSI presents a challenge by itself (Fan et al. 2016; Furutani et al. 2022), distinguishing between different CEF pathways is even more difficult. In organisms with multiple pathways, this requires genetic modifications or use of inhibitors. However, both strategies can underestimate the significance of a target pathway if there is a compensatory upregulation of alternative pathway(s). Moreover, gene inactivation is not applicable for studying the direct FD:Cytb6f CEF route because Cytb6f is essential for LEF while the use of inhibitors in vivo has the potential problems of failure to reach the active site and lack of specificity. For example, antimycin A that inhibits the PGR5-PGRL1 pathway is also known to inhibit mitochondrial respiration (Ikuma and Bonner 1967). Therefore, care is needed in interpreting in vivo effects of antimycin A in the presence of O2. NDH-1 does not have good inhibitors due to a similar structure to the respiratory Complex I but reverse genetics studies are available for NDH-1 since disabling the pathway is possible without hindering LEF. Despite these potential pitfalls with interpreting experiments utilising inhibitors and genetically engineered plants, some important conclusions have been drawn from them, as discussed below.
The CEF routes operate at different timescales. The direct transfer from FD to Cytb6f is likely the route responsible for the maximum CEF observed in C. reinhardtii (Nawrocki et al. 2019a). This fast CEF is conceivably important for prompt adjustments of the ATP/NADPH ratio and efficient build-up of ΔpH for photoprotection. In contrast, NDH-1 and NDH-2 operate at one to two orders of magnitude slower and are markedly sub-stochiometric with PSI, which has prompted a conclusion that they could not account for the maximum CEF in C3 plants and eukaryote microalgae (Nawrocki et al. 2019a). However, NDH-1 is undoubtfully the major CEF route in cyanobacteria responsible for a large fraction of pmf (Miller et al. 2021). Interestingly, in the cyanobacterium Synechocystis sp. PCC 6803, NDH-1 content is approximately 0.1 that of PSI, which may seem low, but it is about the same as that of Cytb6f per PSI (Table 1; Jackson et al. 2023).
Organism | PSI | PSII | Cytb6f | ATP synthase | NDH-1 | PGR5 | References | |
---|---|---|---|---|---|---|---|---|
Synechocystis sp. PCC 6803 | 1 | 0.3 | Cyt b6 0.06 Cyt f 0.2 | NA | 0.1 | NA | Jackson et al. (2023) | |
Arabidopsis thaliana | 1 | 1.7 | 0.6 | 0.7 | 0.022 | 0.07 | McKenzie et al. (2020) |
PSI, Photosystem I; PSII, Photosystem II; Cyt, Cytochrome; NDH-1, type I NADH dehydrogenase-like complex; NA, not available.
In C3 plants, NDH does not have a strong contribution to pmf in optimal conditions, in line with reports of an NDH/PSI ratio of 0.022 and 0.03 (Table 1; McKenzie et al. 2020; Ibrahim et al. 2022). However, electron flux via NDH and/or abundance of the complex in C3 plants increases under stress conditions (Casano et al. 2001; Wang et al. 2006; Strand et al. 2015; Yamori et al. 2015). Moreover, in leaves of C4 plants, which have to significantly increase their ATP yield compared to C3 plants to fuel the CCM, both NDH abundance and CEF rate are significantly higher than in C3 leaves (Munekage et al. 2010; Nakamura et al. 2013; Munekage and Taniguchi 2016; Sagun et al. 2019). Specifically, in bundle sheath (BS) cells of C4Setaria viridis, the CEF/LEF ratio is approximately 5 and NDH mediates more than 50% of CEF (Ermakova et al. 2024). This suggests that the ‘slow’ CEF system involving NDH-1 is preferable for maximising ATP generation in the long term, at least in cyanobacteria and land plants, while algae present a special case due to their unique CEF arrangements, as discussed earlier (see also Rühle et al. 2021). However, taking the full advantage of NDH-1 for ATP generation requires specific adaptations of cell metabolism as we discuss in detail later.
The co-existence of both fast and slow CEF routes should provide photosynthetic organisms with flexibility of regulation of electron transport and better resilience across diverse environmental conditions. In line with this, cyanobacteria that do not have a fully developed PGR5-PGRL1 employ Flvs as a fast CEF system. Flvs are indispensable during sudden increases of irradiance to remove excess of electrons from the reducing side of PSI (Allahverdiyeva et al. 2013; Nikkanen et al. 2020). However, up to 60% of electrons could be directed by Flvs to O2 that might be wasteful and therefore, over the course of evolution, they have likely been substituted by PGR5-PGRL1 (Allahverdiyeva et al. 2015). The two pathways are indeed somewhat interchangeable because expression of Flvs can partially recover the phenotype of the mutant lacking PGR5 (Yamamoto et al. 2016; Wada et al. 2018).
When CEF is the main electron pathway (PSII-less photosynthesis)
In line with the Calvin cycle requirements, in optimal conditions, the CEF/LEF ratio is approximately 0.13 (Avenson et al. 2005). Often stress conditions increase the CEF/LEF ratio due to the elevated ATP demand for stress mitigation and these situations have been extensively reviewed (Shikanai 2014; Suorsa 2015; Yamori and Shikanai 2016; Nawrocki et al. 2019a). However, in extreme cases, encompassing a strong decrease or a lack of PSII activity, CEF can become a dominant electron pathway. Under those circumstances, the metabolic flexibility of PSI can support CEF via the non-photochemical reduction of the PQ pool. Below, we provide a wide body of evidence across cyanobacteria, algae, and plants for electron supply to CEF from sources other than PSII addressing the energy demand of cells in the absence of functional LEF. These cases range from a temporary adaptation to inactive PSII, when PSII is damaged by environmental stresses such as temperature and salinity, to cells evolved to minimise PSII activity and, finally, to photosynthetic organisms that completely lack PSII. This phenomenon is reported under many different names in the literature including ‘electron transport through PSI from stromal electron-donor pool’ (Havaux 1996), ‘suppression of photosynthetic electron transport by respiration’ (Lajkó et al. 1997), ‘NADPH from the oxidative pentose phosphate pathway drives the operation of CEF’ (Lu et al. 2016). We collectively call these phenomena ‘PSII-less photosynthesis’ and distinguish them by two key criteria:
Electrons for PSI are derived from respiratory substrates or other sources and not from PSII.
Despite potentially using electrons sourced from respiratory processes, these pathways are distinct from (chloro)respiration as they do not end at a terminal oxidase.
Therefore, PSII-less photosynthesis is a combination of the non-photochemical reduction of the PQ pool and CEF (Fig. 4). Below, we review the reported cases of PSII-less photosynthesis to demonstrate the ubiquitous nature of this phenomenon and then discuss potential evolutionary advantages conveyed by such arrangement.
PSII-less photosynthesis combines components of photosynthesis and respiration. (a) In photosynthesis (under light), PSII supplies electrons from water to PSI to produce ATP and NADPH in linear electron flow (LEF, green arrows). PSI can operate cyclic electron flow (CEF, red arrows), generating ATP, independently of PSII but requires a supply of electrons. (b) In (chloro)respiration (independent of light), dehydrogenases (DH) extract electron from various substrates to reduce the Ubiquinone (UQ) or PQ pool and terminal oxidases (OX) transfer electrons to O2. Depending on the OX and involvement of an intersystem chain, ATP can be generated. (c) In PSII-less photosynthesis, PSI operates CEF with electrons provided by DH to produce ATP using light energy. PSII, Photosystem II; PSI, Photosystem I; Cytb6f, Cytochrome b6f; PC, plastocyanin; FD, Ferredoxin; FNR, Ferredoxin:NADP+ oxidoreductase; ATP syn, ATP synthase.
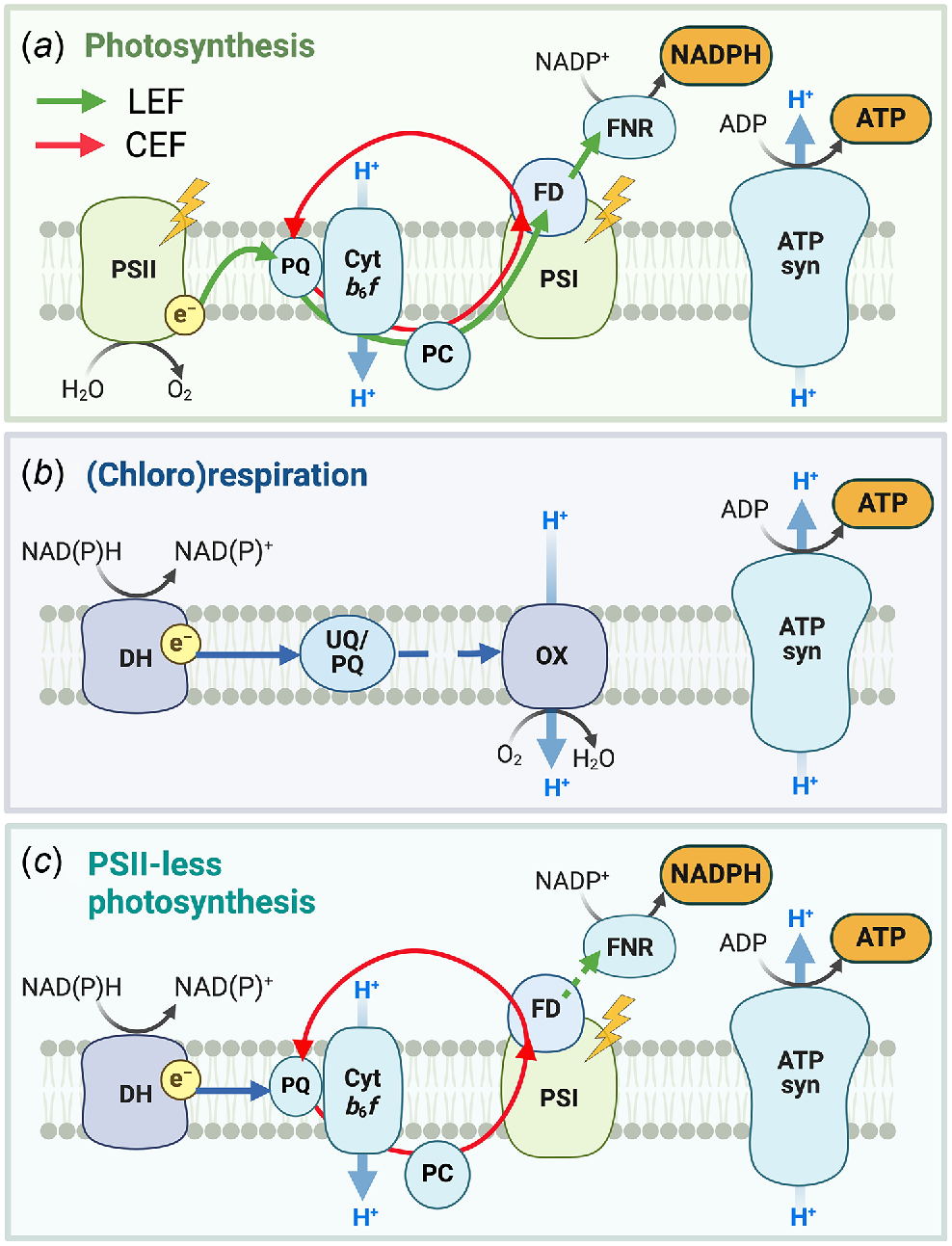
Adaptation to inactive PSII
PSII requires high maintenance and is subject to photodamage and inhibition by factors such as nutrient deficiency, toxicity and salinity (Vass and Cser 2009; Raven 2011; Murata et al. 2012; Lu et al. 2016; Saroussi et al. 2016). As PSII is the most thermally labile and sensitive component of the photosynthetic electron transport chain (Mathur et al. 2014), works examining consequences of the thermal inhibition of PSII have documented electron donation to PSI from alternative electron sources. Other studies employed desiccation or 3-(3,4 dichlorophenyl)−1,1 dimethylurea (DCMU) treatment to abolish PSII activity as well as mutants lacking functional PSII.
Cyanobacteria provide the best studied examples of supplying PSI with non-photochemically derived electrons. The co-existence of respiratory and photosynthetic electron transport chains on the thylakoid membranes of cyanobacteria enables electrons to easily cross from one pathway to another at the level of shared components (Fig. 4): the PQ pool, Cytb6f, or soluble carriers (Vermaas 2001; Ermakova et al. 2016; Lea-Smith et al. 2016). The main respiratory electron input into the PQ pool is thought to be made by succinate dehydrogenase (SDH) that oxidises the tricarboxylic acid cycle (TCA) intermediate succinate to fumarate and reduces PQ. In the absence of SDH, the PQ pool is more oxidised both in darkness and under light, indicating its constitutive activity (Cooley and Vermaas 2001). All sequenced cyanobacterial species also have at least one NDH-2, which often can oxidise both NADH and NADPH (Marreiros et al. 2016). If any NDH-2 activity is present under light, electron transport from NDH-2 to PSI is also likely. After NDH-1 was confirmed to use FD as an electron donor, it became apparent that the complex does not contribute to the respiratory electron influx. However, the gene encoding FNR in cyanobacteria produces short (FNRS) and long (FNRL) forms of the enzyme: FNRL catalyses the reduction of NADP+ whilst FNRS likely mediates the reverse reaction, oxidising NADPH obtained from sugars or organic acids and reducing FD (Thomas et al. 2006). Therefore, together FNRS and NDH-1 could provide electrons to PSI from respiratory NADPH.
With the availability of these alternative routes of electron supply to PSI, there are well-documented instances of cyanobacterial photosynthesis acclimating to insufficient PSII activity. Moderate light exposure causes a major redistribution of respiratory complexes within thylakoid membranes, increasing electrons donation to PSI compared to terminal oxidases (Liu et al. 2012; Liu 2016). Moreover, blocking PSII activity by adding DCMU does not terminate CEF (Liu et al. 2012). A mutant lacking both PSII and respiratory oxidases can source external reductants and organic compounds for growth (Howitt et al. 2001). Based on the redox state of the PQ pool and elevated growth rates under light, the mutant can cycle around PSI electrons derived from the exogenous reductants for energetic gains.
Sulfide can also serve as electron donor to PSI in cyanobacteria. Cohen et al. (1975) showed that Oscillatoria limnetica from the sulfidic Solar Lake near the Gulf of Eilat could carry out CO2 assimilation in the presence of sulfide and DCMU, consistent with the conversion of S2− to So. Later work showed that other cyanobacteria from sulfidic habitats may use sulfide as electron donor to PSI via sulfide-quinone reductase (Hamilton et al. 2018). It is likely that PSII in these cyanobacteria remains functional, but its activity is suppressed through the non-photochemical reduction of the PQ pool.
Chloroplasts of algae and plants have originated from a cyanobacterial endosymbiont, in which thylakoid membranes combined photosynthesis and respiration (Fig. 2). Since the eukaryote hosting the cyanobacterium already had oxidative phosphorylation in mitochondria, the chloroplast thylakoid became more specialised in photosynthetic NADPH and ATP production but retained some components of the respiratory chain. In microalgae, NDA2 is the primary dehydrogenase reducing the PQ pool and its operation could supply electrons to PSI under light (Jans et al. 2008; Johnson and Alric 2013; Saroussi et al. 2016). NDH-1 (with FNR, see discussion in the ‘Plants’ section below) might also reduce the PQ pool with the stromal reductants in some algae where it is retained.
Both terrestrial and some marine green algae can experience periods of desiccation causing severe photodamage of PSII seen by a decrease of the maximum quantum yield of PSII (FV/FM) to zero (Franklin et al. 2003; Gray et al. 2007). A detailed analysis of photosynthetic response to desiccation in both green and red macro algae suggested that stromal reductants were used to poise CEF around PSI when PSII was inhibited (Gao et al. 2011; Gao and Wang 2012; Holzinger and Karsten 2013). In red algae under salinity stress, which results in PSII inhibition, PSI activity was insensitive to DCMU but decreased in the presence of glucosamine (glucose-6-phosphate dehydrogenase inhibitor) and dibromothymoquinone, indicating that NADPH from the oxidative pentose phosphate pathway (OPPP) supported CEF (Lu et al. 2016). This property is especially important in macroalgae, which are intertidal and exposed to strong desiccation at low tide (Franklin et al. 2003; Hanelt and Nultsch 2003). Wang et al. (2023) suggest that PSII-less photosynthesis also has a role in recovery of photosynthesis by the Trebouxia (Chlorophyta: Trebouxiophyeae) photobiont during rehydration of the lichen Cladonia stellaris.
NDH was originally thought to contribute to a chlororespiratory pathway, oxidising NADPH and reducing PQ, in plant chloroplasts (Peltier and Cournac 2002). However, since NDH has been shown to accept electrons from FD, the existence of a chlororespiratory chain became doubtful in land plants and it was thought to be restricted to organisms capable of reverse FNR activity (Peltier et al. 2016). In contrast to cyanobacteria, plant chloroplasts typically have only one form of FNR tailored to catalyse the reduction of NADP+. However, the reverse reaction, although thermodynamically unfavourable, could potentially proceed when the NADPH/NADP+ ratio is high (Carrillo and Ceccarelli 2003). Another possible route for chlororespiratory PQ pool reduction in plants could be NDH-2. Physcomitrella patens has six genes encoding NDH-2, and the products of three of them are targeted to chloroplasts (Xu et al. 2013). The genome of Arabidopsis contains seven open reading frames encoding NDH-2 homologues, and NDC1 has dual targeting to both mitochondria and chloroplasts (Michalecka et al. 2003; Xu et al. 2013). However, NDC1 is more likely involved in prenylquinone and vitamin K1 metabolism in the chloroplast (Piller et al. 2011).
Despite the uncertainty around a biochemical basis for the non-photochemical reduction of the PQ pool in plant chloroplasts, there is some experimental evidence suggesting an alternative electron supply to PSI under stress conditions through the oxidation of stromal reductants (Havaux 1996; Bukhov et al. 2002; Marutani et al. 2012). Among them, an increase of PSI photochemical efficiency in tobacco (Nicotiana tabacum) leaves irradiated with far-red light when mitochondrial respiration is inhibited under anaerobic conditions (Joët et al. 2002). The idea here is that because chloroplasts operate in the cellular context and redox shuttles allow mitochondria and chloroplasts to maintain redox and energetic balance (Raghavendra et al. 1994), conditions provoking reduction of cytosol and mitochondria would result in a reduction of chloroplasts (Havaux 1996; Nellaepalli et al. 2012). Over-reduction of the chloroplast could push the reverse FNR reaction and (via FD and NDH) reduce the PQ pool to leverage this reducing power for generation of ATP through CEF. In another experiment, concomitantly with the loss of PSII activity under heat stress, PSI activity alone was sufficient for maintaining CO2 under the far-red light (Havaux 1996).
Specialised photosynthetic cells with low PSII activity
Heterocysts of filamentous N2-fixing cyanobacteria and bundle sheath (BS) cells of NADP-ME C4 plants present fascinating examples of convergent adaptation in organisms separated by over 1300 million years of evolution (Sage 2004; Sánchez-Baracaldo 2015). Both specialised cell types take advantage of PSI’s metabolic flexibility to adapt to conditions requiring high ATP yield and low [O2]. In both systems, CEF activity is high and PSII activity is very low or absent and, in both cases, the specialised cells must be paired with normal photosynthetic cells that supply reducing power: vegetative cells in case of filamentous cyanobacteria and mesophyll cells in case of C4 plants (Fig. 5).
Schematic representation of two cell-photosynthetic systems employed by (a) filamentous heterocystous N2-fixing cyanobacteria and (b) C4 plants of NADP-ME subtype. In both cases, linear electron flow (green arrows) in vegetative/mesophyll cells supplies reducing power to heterocysts/bundle sheath (BS) cells, which operate PSII-less photosynthesis: non-photochemical reduction of the plastoquinone (PQ) pool (blue arrows) combined with cyclic electron flow (red arrows). A lack of PSII activity in heterocysts/BS cells contributes to maintaining low [O2], beneficial for nitrogenase and Rubisco function. PSII, Photosystem II; Cytb6f, Cytochrome b6f; PC, Plastocyanin; PSI, Photosystem I; FD, Ferredoxin (H = heterocyst-specific, II = BS-specific); FNR, Ferredoxin:NADP+ oxidoreductase (in cyanobacteria, L = long form, S = short form); ATP syn, ATP synthase; OPPP, oxidative pentose phosphate pathway; NDH(-1), type 1 NADH dehydrogenase-like complex; PEPC, phosphoenolpyruvate carboxylase; OAA, oxaloacetate; MDH, NADPH-dependent malate dehydrogenase; ME, NADP+-dependent malic enzyme.
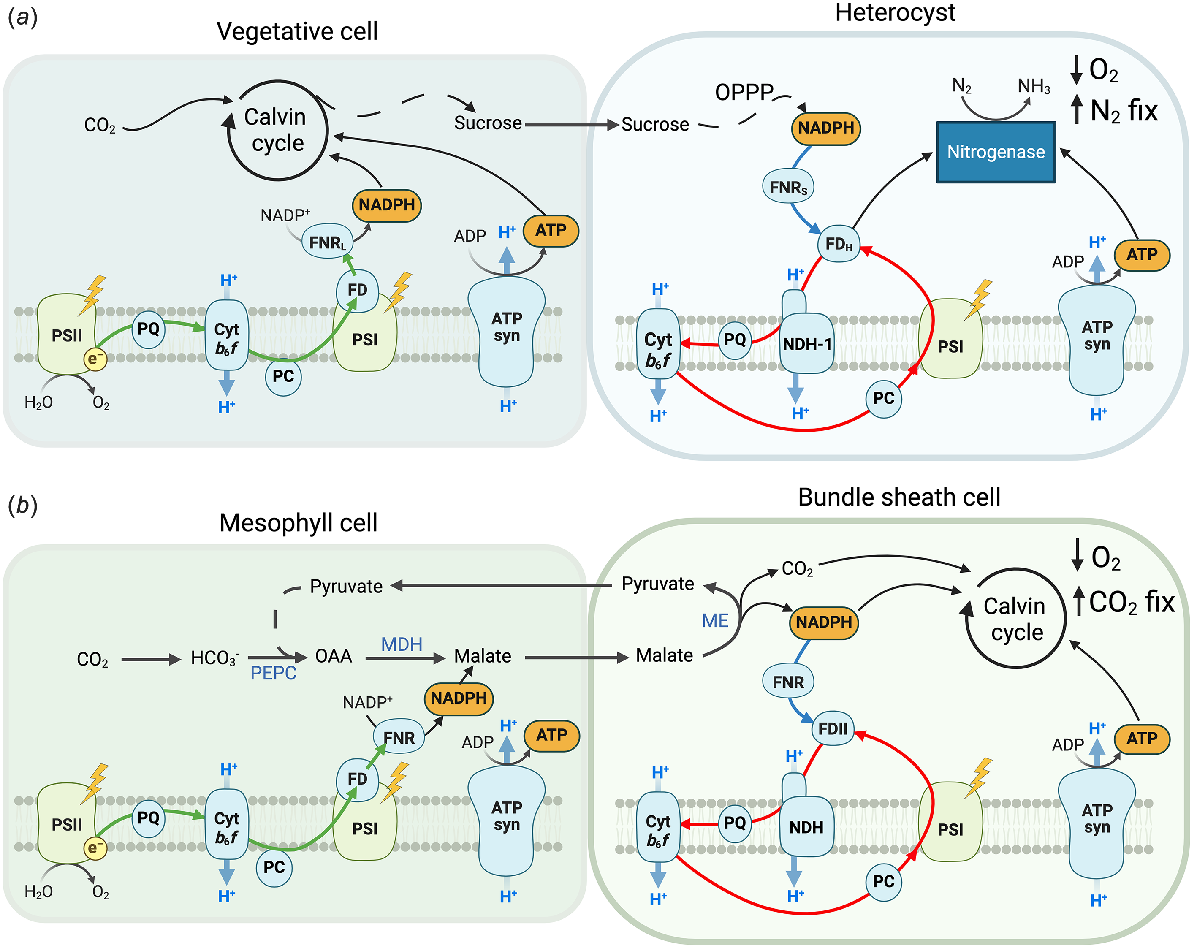
Filamentous heterocyst-forming cyanobacteria comprise a unique group that are remarkably different from unicellular cyanobacteria because they are not only multicellular but have different types of cells in one filament. Heterocysts are specialised N2-fixing cells where a microoxic atmosphere is established in the light, enabling activity of nitrogenase enzyme that is extremely O2 sensitive. Heterocysts develop from vegetative cells upon nitrogen deprivation following a genetic program, and this process involves drastic morphological and physiological changes, including building up additional cell-wall layers (Flores et al. 2019). Walsby (1985) showed that the gas permeability of the heterocyst wall allows sufficient N2 to enter for nitrogenase activity while limiting O2 entry to an extent that allows maintaining microoxic conditions. Active O2-scavenging by respiratory terminal oxidases and Flv3A also contribute to establishing microoxic conditions (Valladares et al. 2007; Ermakova et al. 2013; Ermakova et al. 2014).
Nitrogenase catalyses the reaction of N2 reduction, which requires large quantities of ATP and electrons:
Heterocysts lack enzymes of Calvin cycle and PSII activity since the fast degradation of PSII during heterocyst differentiation is critical for suppressing O2 evolution and establishing microoxic conditions. However, heterocysts retain components of the electron transport chain essential for CEF: PSI, NDH-1, Cytb6f, and ATP synthase (Ow et al. 2008; Cardona et al. 2009; Ow et al. 2009), and active CEF is thought to provide the large amount of ATP required for nitrogen fixation (Tel-Or and Stewart 1976; Almon and Böhme 1980; Ernst et al. 1983). Although PSII might still be present in mature heterocysts (Thiel et al. 1990; Baier et al. 2004; Ferimazova et al. 2013), it can only function in the presence of an exogenously added electron donor indicating inactive OEC (Cardona et al. 2009).
Since heterocysts cannot obtain electrons from water splitting, CEF activity relies on reducing power derived from sucrose imported from vegetative cells through microplasmadesmata connecting cytosols of neighbouring cells (Jüttner 1983; Nürnberg et al. 2015; Santana-Sánchez et al. 2023). Heterocysts contain increased amounts of enzymes of OPPP producing NADPH (Stensjö et al. 2007; Ow et al. 2008; Ow et al. 2009). Because of a high NADPH/NADP+ ratio (Schrautemeier et al. 1984; Schrautemeier et al. 1985), FNRS, catalysing the reduction of FD from NADPH, is the only FNR isoform present in heterocysts (Valladares et al. 1999; Omairi-Nasser et al. 2014; Alcántara-Sánchez et al. 2017). FNRS and NDH-1 can therefore function together to oxidise NADPH and reduce the PQ pool to supply electrons to CEF (Fig. 5).
It is not clear how exactly the large number of electrons required for nitrogen fixation is provided to the nitrogenase. Electron donation to nitrogenase even in darkness implies that electrons from NADPH, via FNRS, could reduce FdxH, a heterocyst-specific FD and the electron donor to nitrogenase (Böhme and Schrautemier 1987). Under light, however, it would be energetically beneficial if these respiratory electrons pass through the photosynthetic electron transport chain before reaching nitrogenase and thereby generate ATP (Magnuson 2019). In fact, a flow of electrons from FdxH to nitrogenase bypassing NDH-1, Cytb6f and PSI, would result in a translocation of 4H+ per e− to the lumen and generate 0.86 ATP per e−, assuming a 14 H+/ATP ratio in the ATP synthase (Pogoryelov et al. 2007). This would suffice for almost a half of the nitrogenase requirement of 2 ATP per e−. The rest of ATP could be generated by cycling the electrons around PSI one-two times. In such an arrangement, nitrogenase and CEF would compete for reduced FdxH but only until a high ATP/ADP ratio would limit ATP synthase activity. Moreover, a lower redox potential of FdxH, compared to the vegetative cell-specific FD, and a better specificity for nitrogenase are important adaptations shifting the balance towards the nitrogenase reaction (Magnuson and Cardona 2016).
C4 photosynthesis includes a metabolic C4 cycle acting as a biochemical CCM that boosts CO2 assimilation (Hatch 1987; von Caemmerer and Furbank 2003). C4 photosynthesis has evolved independently in multiple angiosperm lineages in response to low atmospheric [CO2] mostly during the Neogene period, which began began 24 million years ago and is currently operated by about 5% of the terrestrial plant species (Sage 2004; Sage et al. 2011). Leaves of most C4 plants differentiate two compartments: mesophyll and BS cells (Fig. 5). CO2 entering mesophyll cytosol is initially fixed by phosphoenolpyruvate (PEP) carboxylase (PEPC) into a C4 organic acid, oxaloacetate, giving the name to C4 photosynthesis. Oxaloacetate is then reduced in mesophyll chloroplast to malate, which diffuses to BS cells where it is decarboxylated by the chloroplast NADP+-dependent malic enzyme (NADP-ME). Although other decarboxylation subtypes exist, NADP-ME is the only subtype that is of interest for this review, and it happens to be operated by the most productive C4 crops and also a target for engineering into C3 crops (Furbank 2011; Ermakova et al. 2021a). The decarboxylation of malate produces pyruvate and NADPH and provides CO2 to Rubisco, exclusively located in BS cells. Pyruvate then diffuses back to mesophyll cells and is regenerated into PEP to conclude the C4 cycle. A high activity of PEPC builds up [CO2] around Rubisco allowing maximum velocity of CO2 fixation and suppressing the competing reaction of Rubisco with oxygen. Thereby, C4 plants drastically reduce their energetic expenses on photorespiration and can achieve up to 50% higher biomass yield compared to C3 plants (Zhu et al. 2010).
Since C4 plants must almost double the ATP production compared with ancestral C3 plants, the rate of C4 photosynthesis is often limited by the rate of electron transport (Ermakova et al. 2019, 2023). Similar to C3 photosynthetic cells in optimal condition, C4 mesophyll cells predominantly operate LEF with a minor engagement of CEF (Ermakova et al. 2024; Woodford et al. 2024). However, BS chloroplasts receive at least a half of the required NADPH through malate decarboxylation and can also be indirectly supplied NADPH via the triose phosphate (triose-P) shuttle (Weber and von Caemmerer 2010; von Caemmerer and Furbank 2016). In the latter, a part of 3-phosphoglycerate (PGA) diffuses to mesophyll chloroplasts and is reduced there to triose-P (using NADPH and ATP produced in the mesophyll) before returning to BS cells. Hence, BS cells have little need for LEF and instead operate active CEF to generate the extra ATP required for C4 photosynthesis (Furbank et al. 1990; Asada et al. 1993; Bellasio and Ermakova 2022; Ermakova et al. 2024). As already mentioned, the majority of CEF in BS cells is mediated by NDH, and the indispensable role of NDH in supporting high rates of C4 photosynthesis has been shown in multiple reverse genetic studies (Ishikawa et al. 2016; Peterson et al. 2016; Ogawa et al. 2023; Zhang et al. 2024). The leaf abundance of PGR5-PGRL1 has also increased during the evolutionary transition from C3 to C4 photosynthesis, but PGR5 seems to be distributed more equally between mesophyll and BS cells (Nakamura et al. 2013; Tazoe et al. 2020; Ermakova et al. 2021b). A residual CEF in BS cells of S. viridis lacking NDH could potentially be mediated by PGR5-PGRL1 (Ermakova et al. 2024).
A lack or a strong decrease of O2 evolution in BS cells is proposed to be beneficial for further lowering [O2] around Rubisco and to contribute to the highest quantum yield of CO2 assimilation observed in C4 plants of NADP-ME subtype, compared to other subtypes that retain more PSII activity in the BS (Ehleringer and Pearcy 1983; Bellasio and Lundgren 2024). However, the majority of C4 plants operate a mix of subtypes, which is suggested to aid acclimation to changes in environmental conditions (Furbank 2011; Bellasio and Griffiths 2014). Because of this, PSII activity in BS cells varies depending on growth conditions (Ermakova et al. 2021b; Drozak and Romanowska 2006). Nevertheless, a complete loss of BS PSII activity has been often reported in maize (Zea mays) and sorghum (Sorghum bicolor), which operate almost pure NADP-ME C4 photosynthesis when grown under high light (Woo et al. 1970; Meierhoff and Westhoff 1993). In this case, electrons for CEF could be supplied from NADPH derived from malate decarboxylation (Fig. 5).
The presence of PSII-less photosynthesis in BS cells is supported by PSI activity being able to support CO2 fixation in isolated BS cells supplied with malate alone, when PSII activity was inhibited by DCMU or under far-red light (Osmond 1974). Moreover, this phenomenon was only observed in BS cells of NADP-ME plants and not in other C4 subtypes. It was only later clarified that only NADP-ME plants have increased abundance of NDH in BS cells (Takabayashi et al. 2005) suggesting that NDH is essential for the alternative electron supply to PSI. Similar to heterocysts, BS cells have a specific FD suggested to be tailored for CEF due to decreased interaction with FNR (Kimata and Hase 1989; Kimata-Ariga et al. 2000; Majeran et al. 2005). However, the three FNR isoforms in maize are all expressed, to a different extent, in both cell types, so BS cells do not have a specific FNR (Okutani et al. 2005; Majeran et al. 2008). Future research should clarify whether chloroplast FNR can catalyse the reverse reaction in vivo when the NADPH/NADP+ ratio is high, which is expected in some conditions in BS cells.
Extreme cases with complete PSII absence
UCYN-A is a widely distributed marine diazotrophic cyanobacterium that lacks PSII (Zehr et al. 2008; Tripp et al. 2010). UCYN-A also lacks enzymes of Calvin cycle, TCA and nitrate assimilation, and some enzymes for the synthesis of branched chain amino acids and nucleotides, which suggests a close association of UCYN-A with photolithotrophic plankton (Zehr et al 2008; Tripp et al. 2010). However, the reduced genome of UCYN-A still encodes CEF components: PSI, Cytb6f, FNR, FD, NDH-1 and NDH-2, and ATP synthase. Subtype UCYN-A2 is an endosymbiont of the nanoplanktonic Braarudosphaera bigelowii (Haptophyta: Prymnesiophyceae) (Hagino et al. 2013; Cabello et al. 2020). N2 fixation in UCYN-A2 is limited to the photoperiod, when CEF can supply ATP to nitrogenase, while reductant for N2 fixation is supplied ultimately from oxygenic photosynthesis by the host (Landa et al. 2021; Sarkar et al. 2021).
It is not clear how UCYN-A avoids O2 inhibition of nitrogenase. Among marine cyanobacterial diazotrophs, Richelia has heterocysts, the unicellular Cyanothecea and Crocosphaera only fix N2 at night when respiration maintains intracellular [O2] below the seawater level and the filamentous Trichodesmium fixes N2 in the photoperiod by having oxygenic photosynthesis in some cells and diazotrophy in others (Zehr 2011; Nieves-Morión et al. 2020; Zehr and Capone 2024). UCYN-A fixing N2 under light inside a haptophyte cell carrying out oxygenic photosynthesis has none of these mechanisms limiting O2 inhibition of nitrogenase. Moreover, UCYN-A’s symbiosis with haptophyte is unique among marine diazotrophs in not having the capacity to take up and assimilate nitrate and in maintaining diazotrophy in the presence of combined N. This indicates an extreme adaptation to such a lifestyle with recent findings suggesting that UCYN-A evolved beyond endosymbiosis to become a N2-fixing organelle termed ‘nitroplast’ (Coale et al. 2024). This new perspective implies that PSII-less photosynthesis can be compartmentalised within its own organelle, separate from the chloroplast, within photosynthetic cells to create conditions that favour N2-fixation or other specialised metabolic processes.
An example of a flowering plant with no PSII is the mycoheterotrophic orchid Neottia nidus-avis (Menke and Schmid 1976). Illuminated chloroplasts isolated from the pigmented labella of the flowers of Neottia did not carry out PSII activity but performed phenazine methosulfate-mediated cyclic photophosphorylation. Furthermore, labella of Neottia infiltrated in vivo with 32P-inorganic phosphate showed light-stimulated 32P-ATP production (Menke and Schmid 1976). Cameron et al. (2009) measured a low rate of fixation of supplied 13CO2 by attached Neottia shoots under light but without a dark control so the observed 13CO2 fixation under light could have been due to anaplerotic reactions. The labellum of Neottia has a very low Chl content and a high Chl a/b ratio, relative to photosynthetic structures of co-occurring photoautotophic plants, consistent with the absence of PSII and light-harvesting complex II (Cameron et al. 2009). However, these physiological data contradict the results of sequencing of the plastid genome of Neottia (Logacheva et al. 2011). While the plastid genome contains genes for Chl a synthesis, genes encoding subunits of PSI, PSII, NDH, Cytb6f, and ATP synthase are either absent or non-functional. Unless the functional genes required for operating CEF have been transferred to the nuclear genome, it is impossible to reconcile the physiological data with the plastid genome data and, clearly, further investigation is needed.
Significance of PSII-less photosynthesis and the metabolic flexibility of PSI
Based on the wide range of observations where PSII-less photosynthesis occurs, we suggest a mechanism initiating non-photochemical electron supply to PSI across photosynthetic organisms. Inhibition of the dark O2 reduction by a saturating pulse supplied to C. reinhardtii chloroplasts provides evidence for PSI outcompeting alternative and respiratory oxidases for electrons delivered to the PQ pool by dehydrogenases (Peltier et al. 1987; Cournac et al. 2002). Therefore, when PSII activity is insufficient under light, PSI will quickly oxidise the PQ pool. In cyanobacteria, these conditions directly upregulate SDH activity through the redox state of the PQ pool to maintain electron supply (Cooley et al. 2000; Liu et al. 2012). In algae, the PQ pool is also considered a major regulator of electron transport processes, and a non-photochemical reduction of PQ via NDA2 is activated when the PQ pool is over-oxidised under light (Jans et al. 2008). In plants, specifically in conditions requiring a high ATP/NADPH ratio, NDH together with FNR could initiate NADPH oxidation for poising of CEF when NADPH accumulates in the stroma.
Evolutionary considerations, reviewed at the beginning of this article, suggest that CEF mechanisms evolved at a very early stage, concomitantly with the Z scheme of photosynthesis or even earlier. Considering the widely observed phenomenon of PSII-less photosynthesis enabling PSI to accept electrons from a range of donors in response to limited PSII activity and high ATP demand, one might consider a PSI-centred model of photosynthetic electron transport (Fig. 6). Particularly under stress and in specialised cell types or organelles, PSI alone can maximise ATP generation by cycling non-photochemically sourced electrons. Therefore, practically at any stage of evolution, the Z scheme can reverse to the ‘original’ single-photosystem arrangement when it is energetically beneficial.
Photosystem I (PSI)-centred photosynthesis. PSI can convert electrons into NADPH and ATP (black arrows), or into ATP only via cyclic electron flow (red arrows). When the supply of electrons from water via Photosystem II (PSII) is not sufficient, electrons to PSI can be provided from respiratory reductants (sugars or C4 acids through NAD(P)H, blue arrow) or from reducing substrates like H2S (purple arrow). The flexibility of electron supply to PSI ensures that the capacity for ATP generation using light energy is always available in oxygenic photosynthetic organisms, playing an essential role when PSII activity is insufficient. PQ, plastoquinone; Cytb6f, Cytochrome b6f; PC, Plastocyanin; FD, Ferredoxin; FNR, Ferredoxin:NADP+ oxidoreductase; NDH, type 1 NADH dehydrogenase-like complex.
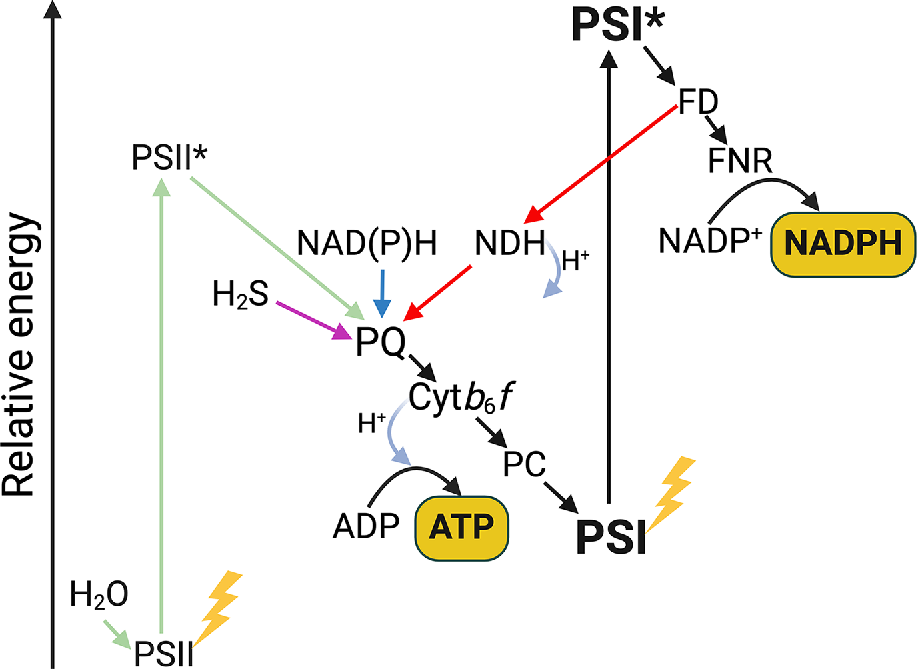
Energetic benefits
We suggest that in conditions when PSII function is limited, reducing PSI with electrons from alternative sources provides oxygenic phototrophs with two energetic advantages. The first one is supporting CEF to generate ATP that enables phototrophs to continue leveraging solar energy even when PSII is damaged. The second advantage is converting NADH, which is produced through respiration, to NADPH that is preferred for many anabolic cellular processes. Whilst cyanobacteria have a transhydrogenase enzyme catalising a direct conversion of NADH to NADPH, this reaction requires pmf (Kämäräinen et al. 2017). In contrast, a diversion of NADH to NADPH through the thylakoid electron transfer chain, driven by PSI, would provide the bonus of generating pmf.
Recycling stored energy through CEF provides numerous physiological advantages compared to respiratory ATP generation. The concept of investing the stored energy into CEF is more attractive because (as discussed in detail in the ‘Heterocysts’ section), from a given NADPH or e− input, the output yields theoretically the same amount of NADPH or e− plus ATP. Bukhov and Carpentier (2004) originally suggested that this approach could generate ATP to support repair processes during recovery from photoinhibition. Repairing damaged PSII is an energy-demanding process that is shown to occur more quickly under light than in darkness (Huang et al. 2018; Yi et al. 2022). The alternative would be to allow PSI to sit in an oxidised state until the damaged PSII is repaired using the finite supplies of energy released through the oxidation of carbohydrate reserves. The concept of leveraging energy parallels investment principles commonly applied in the financial system.
Supporting CEF with electrons from sources other than PSII would additionally help to decrease [O2], and heterocysts and BS cells take advantage of this side effect for hosting enzymes that have improved catalytic properties in the absence of O2, nitrogenase and Rubisco. Multiple parallels that can be drawn between heterocysts and BS cells (Fig. 5) indicate that photosynthetic cells are metabolically adapted to operate either predominantly LEF or CEF and that NDH-1 is the preferred CEF system in cells terminally adapted to operating maximum CEF. As Theeuwen et al. (2022) pointed out, both NDH and PSII compete for PQ as an acceptor. Increasing abundance of NDH in C3 plants would redirect electrons from reducing NADP+ back to PQ pool, downregulating both PSII activity and LEF. The upregulation of CEF at the expense of LEF in heterocysts and BS cells likely explains why, to maximise LEF under optimal conditions, NDH is present in low abundance in C3 plants.
Implications for food security and climate change mitigation
Understanding molecular mechanisms of CEF and phenomena like PSII-less photosynthesis, which could provide plants with better stress resilience, is becoming increasingly important for our efforts aiming at improving food security and mitigating negative effects of climate change. Numerous stresses faced daily by oxygenic phototrophs have more severe impact on PSII than PSI or other electron transport chain components. Many of these stresses, such as heat, drought/desiccation, and excessive irradiance, can impact upon phototrophs simultaneously on a frequent basis. There are considerable advantages available to organisms that can maintain/repair their photosynthetic apparatus using ATP produced through photosynthesis by leveraging their energy reserves instead of ATP produced by respiration, which uses up the energy reserves. Having an option of PSII-less photosynthesis may boost the resilience of phototrophs when they face repeated stress events, especially in response to multiple combined stresses. This pathway therefore could serve as a photoprotective mechanism enabling photosynthetic organisms to acclimate to diverse and fluctuating environmental conditions.
A striking example of the need for phototrophs to better manage their energy budget is the prediction that ‘carbon starvation’ – due to a variety of factors including rising temperatures, vapour pressure deficit, and drought – will be one of the primary drivers of forest mortality across south-west United States by 2050 (McDowell et al. 2016). A single hot, sunny day can destroy the photosynthetic apparatus of a drought-stressed tree (Sevanto et al. 2014). Investigating the natural variation in PSII-less photosynthesis, among other photoprotective mechanisms, in different tree species could therefore inform our deforestation efforts. This knowledge is crucial for maximising the benefits of forest restoration for carbon capture, which is considered one of the most promising approaches to reduce atmospheric CO2 levels (Lewis et al. 2019).
Improving photosynthesis is essential for developing high-yield crops that are better adapted to extreme climates to ensure supply of nutritious food for the rapidly growing population on Earth (Simkin et al. 2019; Walter and Kromdijk 2022; Wu et al. 2023; Croce et al. 2024). A deeper understanding of PSII-less photosynthesis could enable us to develop crops capable of withstanding extended periods without PSII activity. Moreover, uncovering molecular mechanisms enabling increased flux through CEF and generating more ATP, as well as metabolic adaptations of cells with high CEF activity, is essential for engineering C3 plants with CCM. Significant progress has been made in engineering all three major types of CCM into C3 plants: (1) C4 photosynthesis; (2) algal (pyrenoid-based); and (3) cyanobacterial (carboxysome-based) systems (Long et al. 2018; Atkinson et al. 2020; Ermakova et al. 2021a). Installing a CCM into C3 plants would help reduce the oxygenation reaction of Rubisco, increase crop yield by up to 50% and improve nitrogen and water use efficiency (von Caemmerer et al. 2012; McGrath and Long 2014; Hennacy and Jonikas 2020). However, operating a CCM would require significant additional inputs of ATP (Ermakova et al. 2020; Fei et al. 2022). C4 plants achieve higher ATP yield by operating CEF via NDH, whilst cyanobacteria use NDH-1 and Flvs. These electron transport pathways could be considered to engineer C3 chloroplasts for increased ATP generation.
Finally, specialised cells or organelles operating PSII-less photosynthesis and generating ATP while maintaining low [O2] can potentially be used as leaf biofactories, encapsulating enzymes or metabolic pathways sensitive to O2 and/or consuming large quantities of ATP. The most obvious example is nitrogenase, which is a target for engineering into plants due to overuse of nitrogen fertiliser (Allen et al. 2020; Baysal et al. 2022). While nitrogenase is currently targeted to plant mitochondria, inspired by heterocysts and nitroplasts, it could be expressed in specialised cells or organelles with PSII-less photosynthesis, allowing the use of light energy to generate ATP.
Concluding remarks
The energy limitation faced by ancient life provided significant selective pressure to drive divergent evolution of an ur-photosystem towards PSI and PSII. Through the cooperative functions of the two photosystems represented by the Z scheme, life decoupled its evolution from limited energy resources available on the early Earth. The number of organisms incorporating the Z scheme expanded rapidly, including cyanobacteria, which have given origin to plastids in the endosymbiotic event. While there would have been opportunities to evolve organisms relying solely on PSI, once the Z scheme was adopted, there was little selection pressure to eliminate PSII. However, all oxygenic photosynthetic organisms seem to have an insurance policy in case PSII becomes inactive. A ubiquitous mechanism, which we refer to as PSII-less photosynthesis, allows investment of stored carbon resources into CEF around PSI for ATP generation. PSII-less photosynthesis provides environmental flexibility and enhances resilience of photosynthetic organisms to stress, particularly to regular stress events such as tides or hot and dry periods. Moreover, this arrangement has enabled specialisation of heterocysts and BS cells employed to enhance the capabilities of enzymes beyond what is possible in regular photosynthetic cells. Therefore, the metabolic flexibility of PSI has assisted the occupation of diverse global land niches by phototrophs. PSII-less photosynthesis warrants further investigation to understand its role in resilience and to explore potential applications in metabolic engineering of cyanobacteria, algae, and plants with high ATP demands.
Declaration of funding
Work in ME lab is supported by the Australian Research Council’s Discovery Project (DP230100175) and the Thomas Davies Research Grant for Marine, Soil and Plant Biology from the Australian Academy of Sciences.
Author contributions
ME, DF and AWDL have conceived the idea, conducted research and written the article.
Acknowledgements
ME thanks the Australian Society of Plant Scientists for the Peter Goldacre Award and an opportunity to offer this article. We acknowledge valuable comments and seminal contributions to plant science of Prof. John Albert Raven, FRS, FRSE, who contributed to an early draft of this manuscript but sadly passed away before the final version was completed. All figures are created with Biorender.com.
References
Alcántara-Sánchez F, Leyva-Castillo LE, Chagolla-López A, González de la Vara L, Gómez-Lojero C (2017) Distribution of isoforms of ferredoxin-NADP+ reductase (FNR) in cyanobacteria in two growth conditions. The International Journal of Biochemistry & Cell Biology 85, 123-134.
| Crossref | Google Scholar | PubMed |
Allahverdiyeva Y, Ermakova M, Eisenhut M, Zhang P, Richaud P, Hagemann M, Cournac L, Aro E-M (2011) Interplay between flavodiiron proteins and photorespiration in Synechocystis sp. PCC 6803. Journal of Biological Chemistry 286, 24007-24014.
| Crossref | Google Scholar | PubMed |
Allahverdiyeva Y, Mustila H, Ermakova M, Bersanini L, Richaud P, Ajlani G, Battchikova N, Cournac L, Aro E-M (2013) Flavodiiron proteins Flv1 and Flv3 enable cyanobacterial growth and photosynthesis under fluctuating light. Proceedings of the National Academy of Sciences 110, 4111-4116.
| Crossref | Google Scholar |
Allahverdiyeva Y, Suorsa M, Tikkanen M, Aro E-M (2015) Photoprotection of photosystems in fluctuating light intensities. Journal of Experimental Botany 66, 2427-2436.
| Crossref | Google Scholar |
Allen RS, Gregg CM, Okada S, Menon A, Hussain D, Gillespie V, Johnston E, Devilla R, Warden AC, Taylor M, Byrne K, Colgrave M, Wood CC (2020) Plant expression of NifD protein variants resistant to mitochondrial degradation. Proceedings of the National Academy of Sciences 117, 23165-23173.
| Crossref | Google Scholar |
Almon H, Böhme H (1980) Components and activity of the photosynthetic electron transport system of intact heterocysts isolated from the blue-green alga Nostoc muscorum. Biochimica et Biophysica Acta – Bioenergetics 592, 113-120.
| Crossref | Google Scholar |
Asada K (2000) The water–water cycle as alternative photon and electron sinks. Philosophical Transactions of the Royal Society of London. Series B: Biological Sciences 355, 1419-1431.
| Crossref | Google Scholar |
Asada K, Heber U, Schreiber U (1993) Electron flow to the intersystem chain from stromal components and cyclic electron flow in maize chloroplasts, as detected in intact leaves by monitoring redox change of P700 and chlorophyll fluorescence. Plant and Cell Physiology 34, 39-50.
| Crossref | Google Scholar |
Atkinson N, Mao Y, Chan KX, McCormick AJ (2020) Condensation of Rubisco into a proto-pyrenoid in higher plant chloroplasts. Nature Communications 11, 6303.
| Crossref | Google Scholar |
Avenson TJ, Cruz JA, Kanazawa A, Kramer DM (2005) Regulating the proton budget of higher plant photosynthesis. Proceedings of the National Academy of Sciences 102, 9709-9713.
| Crossref | Google Scholar |
Badger MR, von Caemmerer S, Ruuska S, Nakano H (2000) Electron flow to oxygen in higher plants and algae: rates and control of direct photoreduction (Mehler reaction) and rubisco oxygenase. Philosophical Transactions of the Royal Society of London. Series B: Biological Sciences 355, 1433-1446.
| Crossref | Google Scholar |
Bag P, Shutova T, Shevela D, Lihavainen J, Nanda S, Ivanov AG, Messinger J, Jansson S (2023) Flavodiiron-mediated O2 photoreduction at photosystem I acceptor-side provides photoprotection to conifer thylakoids in early spring. Nature Communications 14, 3210.
| Crossref | Google Scholar |
Baier K, Lehmann H, Stephan DP, Lockau W (2004) NblA is essential for phycobilisome degradation in Anabaena sp. strain PCC 7120 but not for development of functional heterocysts. Microbiology 150, 2739-2749.
| Crossref | Google Scholar |
Battchikova N, Eisenhut M, Aro E-M (2011) Cyanobacterial NDH-1 complexes: novel insights and remaining puzzles. Biochimica et Biophysica Acta (BBA) – Bioenergetics 1807, 935-944.
| Crossref | Google Scholar |
Baysal C, Burén S, He W, Jiang X, Capell T, Rubio LM, Christou P (2022) Functional expression of the nitrogenase Fe protein in transgenic rice. Communications Biology 5, 1006.
| Crossref | Google Scholar |
Bellasio C, Ermakova M (2022) Reduction of bundle sheath size boosts cyclic electron flow in C4 Setaria viridis acclimated to low light. The Plant Journal 111, 1223-1237.
| Crossref | Google Scholar |
Bellasio C, Griffiths H (2014) The operation of two decarboxylases, transamination and partitioning of C4 metabolic processes between mesophyll and bundle sheath cells allows light capture to be balanced for the maize C4 pathway. Plant Physiology 164, 466-480.
| Crossref | Google Scholar |
Bellasio C, Lundgren MR (2024) The operation of PEPCK increases light harvesting plasticity in C4 NAD–ME and NADP–ME photosynthetic subtypes: a theoretical study. Plant, Cell & Environment 47, 2288-2309.
| Crossref | Google Scholar |
Böhme H, Schrautemier B (1987) Electron donation to nitrogenase in a cell-free system from heterocysts of Anabaena variabilis. Biochimica et Biophysica Acta (BBA) – Bioenergetics 891, 115-120.
| Crossref | Google Scholar |
Buchert F, Mosebach L, Gäbelein P, Hippler M (2020) PGR5 is required for efficient Q cycle in the cytochrome b6f complex during cyclic electron flow. Biochemical Journal 477, 1631-1650.
| Crossref | Google Scholar |
Bukhov N, Carpentier R (2004) Alternative Photosystem I-driven electron transport routes: mechanisms and functions. Photosynthesis Research 82, 17-33.
| Crossref | Google Scholar |
Bukhov N, Egorova E, Carpentier R (2002) Electron flow to photosystem I from stromal reductants in vivo: the size of the pool of stromal reductants controls the rate of electron donation to both rapidly and slowly reducing photosystem I units. Planta 215, 812-820.
| Crossref | Google Scholar |
Burrows PA, Sazanov LA, Svab Z, Maliga P, Nixon PJ (1998) Identification of a functional respiratory complex in chloroplasts through analysis of tobacco mutants containing disrupted plastid ndh genes. The EMBO Journal 17, 868-876.
| Crossref | Google Scholar |
Cabello AM, Turk-Kubo KA, Hayashi K, Jacobs L, Kudela RM, Zehr JP (2020) Unexpected presence of the nitrogen-fixing symbiotic cyanobacterium UCYN-A in Monterey Bay, California. Journal of Phycology 56, 1521-1533.
| Crossref | Google Scholar |
Cameron DD, Preiss K, Gebauer G, Read DJ (2009) The chlorophyll-containing orchid Corallorhiza trifida derives little carbon through photosynthesis. New Phytologist 183, 358-364.
| Crossref | Google Scholar |
Cardona T, Battchikova N, Zhang P, Stensjö K, Aro E-M, Lindblad P, Magnuson A (2009) Electron transfer protein complexes in the thylakoid membranes of heterocysts from the cyanobacterium Nostoc punctiforme. Biochimica et Biophysica Acta (BBA) – Bioenergetics 1787, 252-263.
| Crossref | Google Scholar |
Cardona T, Sánchez-Baracaldo P, Rutherford AW, Larkum AW (2019) Early Archean origin of Photosystem II. Geobiology 17, 127-150.
| Crossref | Google Scholar |
Carrillo N, Ceccarelli EA (2003) Open questions in ferredoxin-NADP+ reductase catalytic mechanism. European Journal of Biochemistry 270, 1900-1915.
| Crossref | Google Scholar |
Casano LM, Martı́n M, Sabater B (2001) Hydrogen peroxide mediates the induction of chloroplastic Ndh complex under photooxidative stress in barley. Plant Physiology 125, 1450-1458.
| Crossref | Google Scholar |
Coale TH, Loconte V, Turk-Kubo KA, Vanslembrouck B, Mak WKE, Cheung S, Ekman A, Chen J-H, Hagino K, Takano Y, Nishimura T, Adachi M, Le Gros M, Larabell C, Zehr JP (2024) Nitrogen-fixing organelle in a marine alga. Science 384, 217-222.
| Crossref | Google Scholar |
Cohen Y, Jørgensen BB, Padan E, Shilo M (1975) Sulfide-dependent anoxygenic photosynthesis in the cyanobacterium Oscillatoria limnetica. Nature 257, 489-492.
| Crossref | Google Scholar |
Cooley JW, Howitt CA, Vermaas WF (2000) Succinate:quinol oxidoreductases in the cyanobacterium Synechocystis sp. strain PCC 6803: presence and function in metabolism and electron transport. Journal of Bacteriology 182, 714-722.
| Crossref | Google Scholar |
Cooley JW, Vermaas WFJ (2001) Succinate dehydrogenase and other respiratory pathways in thylakoid membranes of Synechocystis sp. Strain PCC 6803: capacity comparisons and physiological function. Journal of Bacteriology 183, 4251-4258.
| Crossref | Google Scholar |
Cournac L, Latouche G, Cerovic Z, Redding K, Ravenel J, Peltier G (2002) In vivo interactions between photosynthesis, mitorespiration, and chlororespiration in Chlamydomonas reinhardtii. Plant Physiology 129, 1921-1928.
| Crossref | Google Scholar |
Croce R, Carmo-Silva E, Cho YB, Ermakova M, Harbinson J, Lawson T, McCormick AJ, Niyogi KK, Ort DR, Patel-Tupper D, Pesaresi P, Raines C, Weber APM, Zhu X-G (2024) Perspectives on improving photosynthesis to increase crop yield. The Plant Cell 36, 3944-3943.
| Crossref | Google Scholar |
Cruz JA, Avenson TJ, Kanazawa A, Takizawa K, Edwards GE, Kramer DM (2005) Plasticity in light reactions of photosynthesis for energy production and photoprotection. Journal of Experimental Botany 56, 395-406.
| Crossref | Google Scholar |
DalCorso G, Pesaresi P, Masiero S, Aseeva E, Schünemann D, Finazzi G, Joliot P, Barbato R, Leister D (2008) A complex containing PGRL1 and PGR5 is involved in the switch between linear and cyclic electron flow in Arabidopsis. Cell 132, 273-285.
| Crossref | Google Scholar |
Dann M, Leister D (2019) Evidence that cyanobacterial Sll1217 functions analogously to PGRL1 in enhancing PGR5-dependent cyclic electron flow. Nature Communications 10, 5299.
| Crossref | Google Scholar |
Davis GA, Kramer DM (2020) Optimization of ATP synthase c–rings for oxygenic photosynthesis. Frontiers in Plant Science 10, 1778.
| Crossref | Google Scholar |
Degen GE, Pastorelli F, Johnson MP (2024) Proton gradient regulation 5 is required to avoid photosynthetic oscillations during light transitions. Journal of Experimental Botany 75, 947-961.
| Crossref | Google Scholar |
Desplats C, Mus F, Cuiné S, Billon E, Cournac L, Peltier G (2009) Characterization of Nda2, a plastoquinone-reducing type II NAD(P)H dehydrogenase in Chlamydomonas chloroplasts. Journal of Biological Chemistry 284, 4148-4157.
| Crossref | Google Scholar |
Drozak A, Romanowska E (2006) Acclimation of mesophyll and bundle sheath chloroplasts of maize to different irradiances during growth. Biochimica et Biophysica Acta (BBA) – Bioenergetics 1757, 1539-1546.
| Crossref | Google Scholar |
Ehleringer J, Pearcy RW (1983) Variation in quantum yield for CO2 uptake among C3 and C4 plants. Plant Physiology 73, 555-559.
| Crossref | Google Scholar |
Emerson R (1957) Dependence of yield of photosynthesis in long-wave red on wavelength and intensity of supplementary light. Science 125, 746.
| Crossref | Google Scholar |
Emerson R, Arnold W (1932) The photochemical reaction in photosynthesis. Journal of General Physiology 16, 191-205.
| Crossref | Google Scholar |
Emerson R, Lewis CM (1943) The dependence of the quantum yield of Chlorella photosynthesis on wave lenghth of light. American Journal of Botany 30, 165-178.
| Crossref | Google Scholar |
Ermakova M, Battchikova N, Allahverdiyeva Y, Aro E-M (2013) Novel heterocyst-specific flavodiiron proteins in Anabaena sp. PCC 7120. FEBS Letters 587, 82-87.
| Crossref | Google Scholar |
Ermakova M, Battchikova N, Richaud P, Leino H, Kosourov S, Isojärvi J, Peltier G, Flores E, Cournac L, Allahverdiyeva Y, Aro E-M (2014) Heterocyst-specific flavodiiron protein Flv3B enables oxic diazotrophic growth of the filamentous cyanobacterium Anabaena sp. PCC 7120. Proceedings of the National Academy of Sciences 111, 11205–11210. 10.1073/pnas.1407327111
Ermakova M, Huokko T, Richaud P, Bersanini L, Howe CJ, Lea-Smith DJ, Peltier G, Allahverdiyeva Y (2016) Distinguishing the roles of thylakoid respiratory terminal oxidases in the cyanobacterium Synechocystis sp. PCC 6803. Plant Physiology 171, 1307-1319.
| Crossref | Google Scholar |
Ermakova M, Lopez-Calcagno PE, Raines CA, Furbank RT, von Caemmerer S (2019) Overexpression of the Rieske FeS protein of the Cytochrome b6f complex increases C4 photosynthesis in Setaria viridis. Communications Biology 2, 314.
| Crossref | Google Scholar |
Ermakova M, Danila FR, Furbank RT, von Caemmerer S (2020) On the road to C4 rice: advances and perspectives. The Plant Journal 101, 940-950.
| Crossref | Google Scholar |
Ermakova M, Arrivault S, Giuliani R, Danila F, Alonso-Cantabrana H, Vlad D, Ishihara H, Feil R, Guenther M, Borghi GL, Covshoff S, Ludwig M, Cousins AB, Langdale JA, Kelly S, Lunn JE, Stitt M, von Caemmerer S, Furbank RT (2021a) Installation of C4 photosynthetic pathway enzymes in rice using a single construct. Plant Biotechnology Journal 19, 575-588.
| Crossref | Google Scholar |
Ermakova M, Bellasio C, Fitzpatrick D, Furbank RT, Mamedov F, von Caemmerer S (2021b) Upregulation of bundle sheath electron transport capacity under limiting light in C4Setaria viridis. The Plant Journal 106, 1443-1454.
| Crossref | Google Scholar |
Ermakova M, Woodford R, Taylor Z, Furbank RT, Belide S, von Caemmerer S (2023) Faster induction of photosynthesis increases biomass and grain yield in glasshouse-grown transgenic Sorghum bicolor overexpressing Rieske FeS. Plant Biotechnology Journal 21, 1206-1216.
| Crossref | Google Scholar |
Ermakova M, Woodford R, Fitzpatrick D, Nix SJ, Zwahlen SM, Farquhar GD, von Caemmerer S, Furbank RT (2024) Chloroplast NADH dehydrogenase-like complex-mediated cyclic electron flow is the main electron transport route in C4 bundle sheath cells. New Phytologist 243, 2187-2200.
| Crossref | Google Scholar |
Ernst A, Böhme H, Böger P (1983) Phosphorylation and nitrogenase activity in isolated heterocysts from Anabaena variabilis (ATCC 29413). Biochimica et Biophysica Acta (BBA) – Bioenergetics 723, 83-90.
| Crossref | Google Scholar |
Fan D-Y, Fitzpatrick D, Oguchi R, Ma W, Kou J, Chow WS (2016) Obstacles in the quantification of the cyclic electron flux around Photosystem I in leaves of C3 plants. Photosynthesis Research 129, 239-251.
| Crossref | Google Scholar |
Fei C, Wilson AT, Mangan NM, Wingreen NS, Jonikas MC (2022) Modelling the pyrenoid-based CO2-concentrating mechanism provides insights into its operating principles and a roadmap for its engineering into crops. Nature Plants 8, 583-595.
| Crossref | Google Scholar |
Ferimazova N, Felcmanová K, Šetlíková E, Küpper H, Maldener I, Hauska G, Šedivá B, Prášil O (2013) Regulation of photosynthesis during heterocyst differentiation in Anabaena sp. strain PCC 7120 investigated in vivo at single-cell level by chlorophyll fluorescence kinetic microscopy. Photosynthesis Research 116, 79-91.
| Crossref | Google Scholar |
Fitzpatrick D, Aro E-M, Tiwari A (2022) True oxygen reduction capacity during photosynthetic electron transfer in thylakoids and intact leaves. Plant Physiology 189, 112-128.
| Crossref | Google Scholar |
Flores E, Picossi S, Valladares A, Herrero A (2019) Transcriptional regulation of development in heterocyst-forming cyanobacteria. Biochimica et Biophysica Acta (BBA) – Gene Regulatory Mechanisms 1862, 673-684.
| Crossref | Google Scholar |
Furbank R, Jenkins C, Hatch MD (1990) C4 photosynthesis: quantum requirement, C4 and overcycling and Q-cycle involvement. Functional Plant Biology 17, 1-7.
| Crossref | Google Scholar |
Furbank RT (2011) Evolution of the C4 photosynthetic mechanism: are there really three C4 acid decarboxylation types? Journal of Experimental Botany 62, 3103-3108.
| Crossref | Google Scholar |
Furutani R, Ohnishi M, Mori Y, Wada S, Miyake C (2022) The difficulty of estimating the electron transport rate at photosystem I. Journal of Plant Research 135, 565-577.
| Crossref | Google Scholar |
Gao S, Wang G (2012) The enhancement of cyclic electron flow around photosystem I improves the recovery of severely desiccated Porphyra yezoensis (Bangiales, Rhodophyta). Journal of Experimental Botany 63, 4349-4358.
| Crossref | Google Scholar |
Gao S, Shen S, Wang G, Niu J, Lin A, Pan G (2011) PSI-driven cyclic electron flow allows intertidal macro-algae Ulva sp. (Chlorophyta) to survive in desiccated conditions. Plant and Cell Physiology 52, 885-893.
| Crossref | Google Scholar |
Gerotto C, Alboresi A, Meneghesso A, Jokel M, Suorsa M, Aro E-M, Morosinotto T (2016) Flavodiiron proteins act as safety valve for electrons in Physcomitrella patens. Proceedings of the National Academy of Sciences 113, 12322-12327.
| Crossref | Google Scholar |
Gray DW, Lewis LA, Cardon ZG (2007) Photosynthetic recovery following desiccation of desert green algae (Chlorophyta) and their aquatic relatives. Plant, Cell & Environment 30, 1240-1255.
| Crossref | Google Scholar |
Hagino K, Onuma R, Kawachi M, Horiguchi T (2013) Discovery of an endosymbiotic nitrogen-fixing cyanobacterium UCYN-A in Braarudosphaera bigelowii (Prymnesiophyceae). PLoS ONE 8, e81749.
| Crossref | Google Scholar |
Hamilton TL, Klatt JM, De Beer D, Macalady JL (2018) Cyanobacterial photosynthesis under sulfidic conditions: insights from the isolate Leptolyngbya sp. strain hensonii. The ISME Journal 12, 568-584.
| Crossref | Google Scholar |
Hatch MD (1987) C4 photosynthesis: a unique blend of modified biochemistry, anatomy and ultrastructure. Biochimica et Biophysica Acta (BBA) – Reviews on Bioenergetics 895, 81-106.
| Crossref | Google Scholar |
Havaux M (1996) Short-term responses of Photosystem I to heat stress. Photosynthesis Research 47, 85-97.
| Crossref | Google Scholar |
Hennacy JH, Jonikas MC (2020) Prospects for engineering biophysical CO2 concentrating mechanisms into land plants to enhance yields. Annual Review of Plant Biology 71, 461-485.
| Crossref | Google Scholar |
Hertle AP, Blunder T, Wunder T, Pesaresi P, Pribil M, Armbruster U, Leister D (2013) PGRL1 is the elusive ferredoxin-plastoquinone reductase in photosynthetic cyclic electron flow. Molecular Cell 49, 511-523.
| Crossref | Google Scholar |
Hill R, Bendall FAY (1960) Function of the two cytochrome components in chloroplasts: a working hypothesis. Nature 186, 136-137.
| Crossref | Google Scholar |
Holzinger A, Karsten U (2013) Desiccation stress and tolerance in green algae: consequences for ultrastructure, physiological and molecular mechanisms. Frontiers in Plant Science 4, 327.
| Crossref | Google Scholar |
Howe CJ, Barbrook AC, Nisbet RER, Lockhart PJ, Larkum AWD (2008) The origin of plastids. Philosophical Transactions of the Royal Society B: Biological Sciences 363, 2675-2685.
| Crossref | Google Scholar |
Howitt CA, Udall PK, Vermaas WF (1999) Type 2 NADH dehydrogenases in the cyanobacterium Synechocystis sp. strain PCC 6803 are involved in regulation rather than respiration. Journal of Bacteriology 181, 3994-4003.
| Crossref | Google Scholar |
Howitt CA, Cooley JW, Wiskich JT, Vermaas WF (2001) A strain of Synechocystis sp. PCC 6803 without photosynthetic oxygen evolution and respiratory oxygen consumption: implications for the study of cyclic photosynthetic electron transport. Planta 214, 46-56.
| Crossref | Google Scholar |
Huang L-S, Cobessi D, Tung EY, Berry EA (2005) Binding of the respiratory chain inhibitor antimycin to the mitochondrial bc1 complex: a new crystal structure reveals an altered intramolecular hydrogen-bonding pattern. Journal of Molecular Biology 351, 573-597.
| Crossref | Google Scholar |
Huang W, Yang Y-J, Zhang S-B, Liu T (2018) Cyclic electron flow around Photosystem I promotes ATP synthesis possibly helping the rapid repair of photodamaged Photosystem II at low light. Frontiers in Plant Science 9, 239.
| Crossref | Google Scholar |
Huokko T, Muth-Pawlak D, Battchikova N, Allahverdiyeva Y, Aro E-M (2017) Role of type 2 NAD(P)H dehydrogenase NdbC in redox regulation of carbon allocation in Synechocystis. Plant Physiology 174, 1863-1880.
| Crossref | Google Scholar |
Huokko T, Muth-Pawlak D, Aro E-M (2019) Thylakoid localized type 2 NAD(P)H dehydrogenase NdbA optimizes light-activated heterotrophic growth of Synechocystis sp. PCC 6803. Plant and Cell Physiology 60, 1386-1399.
| Crossref | Google Scholar |
Ibrahim IM, Mckenzie SD, Chung J, Aryal UK, Leon-Salas WD, Puthiyaveetil S (2022) Photosystem stoichiometry adjustment is a photoreceptor-mediated process in Arabidopsis. Scientific Reports 12, 10982.
| Crossref | Google Scholar |
Ikuma H, Bonner WD, Jr (1967) Properties of higher plant mitochondria. III. Effects of respiratory inhibitors. Plant Physiology 42, 1535-1544.
| Crossref | Google Scholar |
Ilík P, Pavlovič A, Kouřil R, Alboresi A, Morosinotto T, Allahverdiyeva Y, Aro E-M, Yamamoto H, Shikanai T (2017) Alternative electron transport mediated by flavodiiron proteins is operational in organisms from cyanobacteria up to gymnosperms. New Phytologist 214, 967-972.
| Crossref | Google Scholar |
Ishikawa N, Takabayashi A, Noguchi K, Tazoe Y, Yamamoto H, von Caemmerer S, Sato F, Endo T (2016) NDH-mediated cyclic electron flow around Photosystem I is crucial for C4 photosynthesis. Plant and Cell Physiology 57, 2020-2028.
| Crossref | Google Scholar |
Iwai M, Takizawa K, Tokutsu R, Okamuro A, Takahashi Y, Minagawa J (2010) Isolation of the elusive supercomplex that drives cyclic electron flow in photosynthesis. Nature 464, 1210-1213.
| Crossref | Google Scholar |
Jackson PJ, Hitchcock A, Brindley AA, Dickman MJ, Hunter CN (2023) Absolute quantification of cellular levels of photosynthesis-related proteins in Synechocystis sp. PCC 6803. Photosynthesis Research 155, 219-245.
| Crossref | Google Scholar |
Jans F, Mignolet E, Houyoux P-A, Cardol P, Ghysels B, Cuiné S, Cournac L, Peltier G, Remacle C, Franck F (2008) A type II NAD(P)H dehydrogenase mediates light-independent plastoquinone reduction in the chloroplast of Chlamydomonas. Proceedings of the National Academy of Sciences 105, 20546-20551.
| Crossref | Google Scholar |
Joët T, Cournac L, Peltier G, Havaux M (2002) Cyclic electron flow around Photosystem I in C3 plants. In vivo control by the redox state of chloroplasts and involvement of the NADH-dehydrogenase complex. Plant Physiology 128, 760-769.
| Crossref | Google Scholar |
Johnson X, Alric J (2013) Central carbon metabolism and electron transport in Chlamydomonas reinhardtii: metabolic constraints for carbon partitioning between oil and starch. Eukaryote Cell 12, 776-793.
| Crossref | Google Scholar |
Jokel M, Kosourov S, Battchikova N, Tsygankov AA, Aro EM, Allahverdiyeva Y (2015) Chlamydomonas flavodiiron proteins facilitate acclimation to anoxia during sulfur deprivation. Plant and Cell Physiology 56, 1598-1607.
| Crossref | Google Scholar |
Jüttner F (1983) 14C-labeled metabolites in heterocysts and vegetative cells of Anabaena cylindrica filaments and their presumptive function as transport vehicles of organic carbon and nitrogen. Journal of Bacteriology 155, 628-633.
| Crossref | Google Scholar |
Kämäräinen J, Huokko T, Kreula S, Jones PR, Aro E-M, Kallio P (2017) Pyridine nucleotide transhydrogenase PntAB is essential for optimal growth and photosynthetic integrity under low-light mixotrophic conditions in Synechocystis sp. PCC 6803. New Phytologist 214, 194-204.
| Crossref | Google Scholar |
Kimata Y, Hase T (1989) Localization of ferredoxin isoproteins in mesophyll and bundle sheath cells in maize leaf. Plant Physiology 89, 1193-1197.
| Crossref | Google Scholar |
Kimata-Ariga Y, Matsumura T, Kada S, Fujimoto H, Fujita Y, Endo T, Mano JI, Sato F, Hase T (2000) Differential electron flow around photosystem I by two C4-photosynthetic-cell-specific ferredoxins. The EMBO Journal 19, 5041-5050.
| Crossref | Google Scholar |
Kok B, Forbush B, Mcgloin M (1970) Cooperation of charges in photosynthetic O2 evolution–I. A linear four step mechanism. Photochemistry and Photobiology 11, 457-475.
| Crossref | Google Scholar |
Kramer DM, Evans JR (2011) The importance of energy balance in improving photosynthetic productivity. Plant Physiology 155, 70-78.
| Crossref | Google Scholar |
Lajkó F, Kadioglu A, Borbély G, Garab G (1997) Competition between the photosynthetic and the (chloro)respiratory electron transport chains in cyanobacteria, green algae and higher plants. Effect of heat stress. Photosynthetica 33, 217-226.
| Crossref | Google Scholar |
Landa M, Turk-Kubo KA, Cornejo-Castillo FM, Henke BA, Zehr JP (2021) Critical role of light in the growth and activity of the marine N2-fixing UCYN-A symbiosis. Frontiers in Microbiology 12, 666739.
| Crossref | Google Scholar |
Laughlin TG, Bayne AN, Trempe J-F, Savage DF, Davies KM (2019) Structure of the complex I-like molecule NDH of oxygenic photosynthesis. Nature 566, 411-414.
| Crossref | Google Scholar |
Laughlin TG, Savage DF, Davies KM (2020) Recent advances on the structure and function of NDH-1: the complex I of oxygenic photosynthesis. Biochimica et Biophysica Acta (BBA) - Bioenergetics 1861, 148254.
| Crossref | Google Scholar |
Lea-Smith DJ, Bombelli P, Vasudevan R, Howe CJ (2016) Photosynthetic, respiratory and extracellular electron transport pathways in cyanobacteria. Biochimica et Biophysica Acta (BBA) – Bioenergetics 1857, 247-255.
| Crossref | Google Scholar |
Leister D, Marino G, Minagawa J, Dann M (2022) An ancient function of PGR5 in iron delivery? Trends in Plant Science 27, 971-980.
| Crossref | Google Scholar |
Lewis S, Wheeler C, Mitchard E, Koch A (2019) Restoring natural forests is the best way to remove atmospheric carbon. Nature 568, 25-28.
| Crossref | Google Scholar |
Liu L-N (2016) Distribution and dynamics of electron transport complexes in cyanobacterial thylakoid membranes. Biochimica et Biophysica Acta (BBA) – Bioenergetics 1857, 256-265.
| Crossref | Google Scholar |
Liu L-N, Bryan SJ, Huang F, Yu J, Nixon PJ, Rich PR, Mullineaux CW (2012) Control of electron transport routes through redox-regulated redistribution of respiratory complexes. Proceedings of the National Academy of Sciences 109, 11431-11436.
| Crossref | Google Scholar |
Livingston AK, Cruz JA, Kohzuma K, Dhingra A, Kramer DM (2010) An Arabidopsis mutant with high cyclic electron flow around Photosystem I (hcef) Involving the NADPH dehydrogenase complex. The Plant Cell 22, 221-233.
| Crossref | Google Scholar |
Logacheva MD, Schelkunov MI, Penin AA (2011) Sequencing and analysis of plastid genome in mycoheterotrophic orchid Neottia nidus-avis. Genome Biology and Evolution 3, 1296-1303.
| Crossref | Google Scholar |
Long BM, Hee WY, Sharwood RE, Rae BD, Kaines S, Lim Y-L, Nguyen ND, Massey B, Bala S, von Caemmerer S, Badger MR, Price GD (2018) Carboxysome encapsulation of the CO2-fixing enzyme Rubisco in tobacco chloroplasts. Nature Communications 9, 3570.
| Crossref | Google Scholar |
Lu X, Huan L, Gao S, He L, Wang G (2016) NADPH from the oxidative pentose phosphate pathway drives the operation of cyclic electron flow around photosystem I in high-intertidal macroalgae under severe salt stress. Physiologia Plantarum 156, 397-406.
| Crossref | Google Scholar |
Magnuson A (2019) Heterocyst thylakoid bioenergetics. Life 9, 13.
| Crossref | Google Scholar |
Magnuson A, Cardona T (2016) Thylakoid membrane function in heterocysts. Biochimica et Biophysica Acta (BBA) – Bioenergetics 1857, 309-319.
| Crossref | Google Scholar |
Majeran W, Cai Y, Sun Q, Van Wijk KJ (2005) Functional differentiation of bundle sheath and mesophyll maize chloroplasts determined by comparative proteomics. The Plant Cell 17, 3111-3140.
| Crossref | Google Scholar |
Majeran W, Zybailov B, Ytterberg AJ, Dunsmore J, Sun Q, Van Wijk KJ (2008) Consequences of C4 differentiation for chloroplast membrane proteomes in maize mesophyll and bundle sheath cells. Molecular & Cellular Proteomics 7, 1609-1638.
| Crossref | Google Scholar |
Malone LA, Proctor MS, Hitchcock A, Hunter CN, Johnson MP (2021) Cytochrome b6f – orchestrator of photosynthetic electron transfer. Biochimica et Biophysica Acta (BBA) - Bioenergetics 1862, 148380.
| Crossref | Google Scholar |
Marreiros BC, Sena FV, Sousa FM, Batista AP, Pereira MM (2016) Type II NADH:quinone oxidoreductase family: phylogenetic distribution, structural diversity and evolutionary divergences. Environmental Microbiology 18, 4697-4709.
| Crossref | Google Scholar |
Martín M, Sabater B (2010) Plastid ndh genes in plant evolution. Plant Physiology and Biochemistry 48, 636-645.
| Crossref | Google Scholar |
Martin WF, Bryant DA, Beatty JT (2017) A physiological perspective on the origin and evolution of photosynthesis. FEMS Microbiology Reviews 42, 205-231.
| Crossref | Google Scholar |
Marutani Y, Yamauchi Y, Kimura Y, Mizutani M, Sugimoto Y (2012) Damage to photosystem II due to heat stress without light-driven electron flow: involvement of enhanced introduction of reducing power into thylakoid membranes. Planta 236, 753-761.
| Crossref | Google Scholar |
Mathur S, Agrawal D, Jajoo A (2014) Photosynthesis: response to high temperature stress. Journal of Photochemistry and Photobiology B: Biology 137, 116-126.
| Crossref | Google Scholar |
McDowell NG, Williams AP, Xu C, Pockman WT, Dickman LT, Sevanto S, Pangle R, Limousin J, Plaut J, Mackay DS, Ogee J, Domec JC, Allen CD, Fisher RA, Jiang X, Muss JD, Breshears DD, Rauscher SA, Koven C (2016) Multi-scale predictions of massive conifer mortality due to chronic temperature rise. Nature Climate Change 6, 295-300.
| Crossref | Google Scholar |
McGrath JM, Long SP (2014) Can the cyanobacterial carbon-concentrating mechanism increase photosynthesis in crop species? A theoretical analysis. Plant Physiology 164, 2247-2261.
| Crossref | Google Scholar |
McKenzie SD, Ibrahim IM, Aryal UK, Puthiyaveetil S (2020) Stoichiometry of protein complexes in plant photosynthetic membranes. Biochimica et Biophysica Acta (BBA) – Bioenergetics 1861, 148141.
| Crossref | Google Scholar |
Meierhoff K, Westhoff P (1993) Differential biogenesis of photosystem-II in mesophyll and bundle-sheath cells of monocotyledonous NADP-malic enzyme-type C4 plants: the nonstoichiometric abundance of the subunits of photosystem-II in the bundle-sheath chloroplasts and the translational activity of the plastome-encoded genes. Planta 191, 23-33.
| Crossref | Google Scholar |
Menke W, Schmid GH (1976) Cyclic photophosphorylation in the mykotrophic orhid Neottia nidus-avis. Plant Physiology 57, 716-719.
| Crossref | Google Scholar |
Messant M, Hani U, Lai T-L, Wilson A, Shimakawa G, Krieger-Liszkay A (2024) Plastid terminal oxidase (PTOX) protects photosystem I and not photosystem II against photoinhibition in Arabidopsis thaliana and Marchantia polymorpha. The Plant Journal 117, 669-678.
| Crossref | Google Scholar |
Michalecka AM, Staffan Svensson Å, Johansson FI, Agius SC, Johanson U, Brennicke A, Binder S, Rasmusson AG (2003) Arabidopsis genes encoding mitochondrial type II NAD(P)H dehydrogenases have different evolutionary origin and show distinct responses to light. Plant Physiology 133, 642-652.
| Crossref | Google Scholar |
Miller NT, Vaughn MD, Burnap RL (2021) Electron flow through NDH-1 complexes is the major driver of cyclic electron flow-dependent proton pumping in cyanobacteria. Biochimica et Biophysica Acta (BBA) – Bioenergetics 1862, 148354.
| Crossref | Google Scholar |
Müller P, Li X-P, Niyogi KK (2001) Non-photochemical quenching. A response to excess light energy. Plant Physiology 125, 1558-1566.
| Crossref | Google Scholar |
Munekage YN, Taniguchi YY (2016) Promotion of cyclic electron transport around photosystem I with the development of C4 photosynthesis. Plant and Cell Physiology 57, 897-903.
| Crossref | Google Scholar |
Munekage YN, Eymery F, Rumeau D, Cuiné S, Oguri M, Nakamura N, Yokota A, Genty B, Peltier G (2010) Elevated expression of PGR5 and NDH-H in bundle sheath chloroplasts in C4Flaveria species. Plant and Cell Physiology 51, 664-668.
| Crossref | Google Scholar |
Munekage Y, Hojo M, Meurer J, Endo T, Tasaka M, Shikanai T (2002) PGR5 is involved in cyclic electron flow around photosystem I and is essential for photoprotection in Arabidopsis. Cell 110, 361-371.
| Crossref | Google Scholar |
Murata N, Allakhverdiev SI, Nishiyama Y (2012) The mechanism of photoinhibition in vivo: re-evaluation of the roles of catalase, α-tocopherol, non-photochemical quenching, and electron transport. Biochimica et Biophysica Acta (BBA) – Bioenergetics 1817, 1127-1133.
| Crossref | Google Scholar |
Nakamura N, Iwano M, Havaux M, Yokota A, Munekage YN (2013) Promotion of cyclic electron transport around photosystem I during the evolution of NADP–malic enzyme-type C4 photosynthesis in the genus Flaveria. New Phytologist 199, 832-842.
| Crossref | Google Scholar |
Nakano H, Yamamoto H, Shikanai T (2019) Contribution of NDH-dependent cyclic electron transport around photosystem I to the generation of proton motive force in the weak mutant allele of pgr5. Biochimica et Biophysica Acta (BBA) – Bioenergetics 1860, 369-374.
| Crossref | Google Scholar |
Nawrocki WJ, Bailleul B, Picot D, Cardol P, Rappaport F, Wollman FA, Joliot P (2019a) The mechanism of cyclic electron flow. Biochimica et Biophysica Acta (BBA) – Bioenergetics 1860, 433-438.
| Crossref | Google Scholar |
Nawrocki WJ, Bailleul B, Cardol P, Rappaport F, Wollman FA, Joliot P (2019b) Maximal cyclic electron flow rate is independent of PGRL1 in Chlamydomonas. Biochimica et Biophysica Acta (BBA) – Bioenergetics 1860, 425-432.
| Crossref | Google Scholar |
Nawrocki WJ, Buchert F, Joliot P, Rappaport F, Bailleul B, Wollman F-A (2019c) Chlororespiration controls growth under intermittent light. Plant Physiology 179, 630-639.
| Crossref | Google Scholar |
Nellaepalli S, Kodru S, Tirupathi M, Subramanyam R (2012) Anaerobiosis induced state transition: a non photochemical reduction of PQ pool mediated by NDH in Arabidopsis thaliana. PLoS ONE 7, e49839.
| Crossref | Google Scholar |
Nieves-Morión M, Flores E, Foster RA (2020) Predicting substrate exchange in marine diatom-heterocystous cyanobacteria symbioses. Environmental Microbiology 22, 2027-2052.
| Crossref | Google Scholar |
Nikkanen L, Santana Sánchez A, Ermakova M, Rögner M, Cournac L, Allahverdiyeva Y (2020) Functional redundancy between flavodiiron proteins and NDH-1 in Synechocystis sp. PCC 6803. The Plant Journal 103, 1460-1476.
| Crossref | Google Scholar |
Nürnberg DJ, Mariscal V, Bornikoel J, Nieves-Morión M, Krauß N, Herrero A, Maldener I, Flores E, Mullineaux C (2015) Intercellular diffusion of a fluorescent sucrose analog via the septal junctions in a filamentous cyanobacterium. mBio 6, e02109.
| Crossref | Google Scholar |
Ogawa T, Kobayashi K, Taniguchi YY, Shikanai T, Nakamura N, Yokota A, Munekage YN (2023) Two cyclic electron flows around photosystem I differentially participate in C4 photosynthesis. Plant Physiology 191, 2288-2300.
| Crossref | Google Scholar |
Okutani S, Hanke GT, Satomi Y, Takao T, Kurisu G, Suzuki A, Hase T (2005) Three maize leaf ferredoxin:NADPH oxidoreductases vary in subchloroplast location, expression, and interaction with ferredoxin. Plant Physiology 139, 1451-1459.
| Crossref | Google Scholar |
Omairi-Nasser A, Galmozzi CV, Latifi A, Muro-Pastor MI, Ajlani G (2014) NtcA is responsible for accumulation of the small isoform of ferredoxin:NADP oxidoreductase. Microbiology 160, 789-794.
| Crossref | Google Scholar |
Orf GS, Gisriel C, Redding KE (2018) Evolution of photosynthetic reaction centers: insights from the structure of the heliobacterial reaction center. Photosynthesis Research 138, 11-37.
| Crossref | Google Scholar |
Osmond CB (1974) Carbon reduction and photosystem II deficiency in leaves of C4 plants. Australian Journal of Plant Physiology 1, 41-50.
| Google Scholar |
Ow SY, Cardona T, Taton A, Magnuson A, Lindblad P, Stensjö K, Wright PC (2008) Quantitative shotgun proteomics of enriched heterocysts from Nostoc sp. PCC 7120 using 8-plex isobaric peptide tags. Journal of Proteome Research 7, 1615-1628.
| Crossref | Google Scholar |
Ow SY, Noirel J, Cardona T, Taton A, Lindblad P, Stensjö K, Wright PC (2009) Quantitative overview of N2 fixation in Nostoc punctiforme ATCC 29133 through cellular enrichments and iTRAQ shotgun proteomics. Journal of Proteome Research 8, 187-198.
| Crossref | Google Scholar |
Pan X, Cao D, Xie F, Xu F, Su X, Mi H, Zhang X, Li M (2020) Structural basis for electron transport mechanism of complex I-like photosynthetic NAD(P)H dehydrogenase. Nature communications 11, 610.
| Crossref | Google Scholar |
Peltier G, Cournac L (2002) Chlororespiration. Annual Review of Plant Biology 53, 523-550.
| Crossref | Google Scholar |
Peltier G, Aro E-M, Shikanai T (2016) NDH-1 and NDH-2 plastoquinone reductases in oxygenic photosynthesis. Annual Review of Plant Biology 67, 55-80.
| Crossref | Google Scholar |
Peltier G, Ravenel J, Verméglio A (1987) Inhibition of a respiratory activity by short saturating flashes in Chlamydomonas: evidence for a chlororespiration. Biochimica et Biophysica Acta (BBA) – Bioenergetics 893, 83-90.
| Crossref | Google Scholar |
Peng L, Fukao Y, Fujiwara M, Takami T, Shikanai T (2009) Efficient operation of NAD(P)H dehydrogenase requires supercomplex formation with photosystem I via minor LHCI in Arabidopsis. The Plant Cell 21, 3623-3640.
| Crossref | Google Scholar |
Peterson RB, Schultes NP, Mchale NA, Zelitch I (2016) Evidence for a role for NAD(P)H dehydrogenase in concentration of CO2 in the bundle sheath cell of Zea mays. Plant Physiology 171, 125-138.
| Crossref | Google Scholar |
Petroutsos D, Terauchi AM, Busch A, Hirschmann I, Merchant SS, Finazzi G, Hippler M (2009) PGRL1 participates in iron-induced remodeling of the photosynthetic apparatus and in energy metabolism in Chlamydomonas reinhardtii. Journal of Biological Chemistry 284, 32770-32781.
| Crossref | Google Scholar |
Piller LE, Besagni C, Ksas B, Rumeau D, Bréhélin C, Glauser G, Kessler F, Havaux M (2011) Chloroplast lipid droplet type II NAD(P)H quinone oxidoreductase is essential for prenylquinone metabolism and vitamin K1 accumulation. Proceedings of the National Academy of Sciences 108, 14354-14359.
| Crossref | Google Scholar |
Pogoryelov D, Reichen C, Klyszejko AL, Brunisholz R, Muller DJ, Dimroth P, Meier T (2007) The oligomeric state of c rings from cyanobacterial F-ATP synthases varies from 13 to 15. Journal of Bacteriology 189, 5895-5902.
| Crossref | Google Scholar |
Raghavendra AS, Padmasree K, Saradadevi K (1994) Interdependence of photosynthesis and respiration in plant cells: interactions between chloroplasts and mitochondria. Plant Science 97, 1-14.
| Crossref | Google Scholar |
Raven JA (2011) The cost of photoinhibition. Physiologia Plantarum 142, 87-104.
| Crossref | Google Scholar |
Rühle T, Dann M, Reiter B, Schünemann D, Naranjo B, Penzler J-F, Kleine T, Leister D (2021) PGRL2 triggers degradation of PGR5 in the absence of PGRL1. Nature Communications 12, 3941.
| Crossref | Google Scholar |
Sadekar S, Raymond J, Blankenship RE (2006) Conservation of distantly related membrane proteins: photosynthetic reaction centers share a common structural core. Molecular Biology and Evolution 23, 2001-2007.
| Crossref | Google Scholar |
Sage RF (2004) The evolution of C4 photosynthesis. New Phytologist 161, 341-370.
| Crossref | Google Scholar |
Sage RF, Christin P-A, Edwards EJ (2011) The C4 plant lineages of planet Earth. Journal of Experimental Botany 62, 3155-3169.
| Crossref | Google Scholar |
Sagun JV, Badger MR, Chow WS, Ghannoum O (2019) Cyclic electron flow and light partitioning between the two photosystems in leaves of plants with different functional types. Photosynthesis Research 142, 321-334.
| Crossref | Google Scholar |
Sagun JV, Badger MR, Chow WS, Ghannoum O (2021) Mehler reaction plays a role in C3 and C4 photosynthesis under shade and low CO2. Photosynthesis Research 149(1–2), 171-185.
| Crossref | Google Scholar |
Sánchez-Baracaldo P (2015) Origin of marine planktonic cyanobacteria. Scientific Reports 5, 17418.
| Crossref | Google Scholar |
Sánchez-Baracaldo P, Cardona T (2020) On the origin of oxygenic photosynthesis and Cyanobacteria. New Phytologist 225, 1440-1446.
| Crossref | Google Scholar |
Santana-Sanchez A, Solymosi D, Mustila H, Bersanini L, Aro E-M, Allahverdiyeva Y (2019) Flavodiiron proteins 1–to-4 function in versatile combinations in O2 photoreduction in cyanobacteria. eLife 8, e45766.
| Crossref | Google Scholar |
Santana-Sánchez A, Nikkanen L, Werner E, Tóth G, Ermakova M, Kosourov S, Walter J, He M, Aro E-M, Allahverdiyeva Y (2023) Flv3A facilitates O2 photoreduction and affects H2 photoproduction independently of Flv1A in diazotrophic Anabaena filaments. New Phytologist 237, 126-139.
| Crossref | Google Scholar |
Sarewicz M, Pintscher S, Pietras R, Borek A, Bujnowicz Ł, Hanke G, Cramer WA, Finazzi G, Osyczka A (2021) Catalytic reactions and energy conservation in the Cytochrome bc1 and b6f complexes of energy-transducing membranes. Chemical Reviews 121, 2020-2108.
| Crossref | Google Scholar |
Sarkar D, Landa M, Bandyopadhyay A, Pakrasi HB, Zehr JP, Maranas CD (2021) Elucidation of trophic interactions in an unusual single-cell nitrogen-fixing symbiosis using metabolic modeling. PLoS Computational Biology 17, e1008983.
| Crossref | Google Scholar |
Saroussi SI, Wittkopp TM, Grossman AR (2016) The type II NADPH dehydrogenase facilitates cyclic electron flow, energy-dependent quenching, and chlororespiratory metabolism during acclimation of Chlamydomonas reinhardtii to nitrogen deprivation. Plant Physiology 170, 1975-1988.
| Crossref | Google Scholar |
Sato R, Kawashima R, Trinh MDL, Nakano M, Nagai T, Masuda S (2019) Significance of PGR5-dependent cyclic electron flow for optimizing the rate of ATP synthesis and consumption in Arabidopsis chloroplasts. Photosynthesis Research 139, 359-365.
| Crossref | Google Scholar |
Schopf JW (2011) The paleobiological record of photosynthesis. Photosynthesis Research 107, 87-101.
| Crossref | Google Scholar |
Schrautemeier B, Böhme H, Böger P (1984) In vitro studies on pathways and regulation of electron transport to nitrogenase with a cell-free extract from heterocysts of Anabaena variabilis. Archives of Microbiology 137, 14-20.
| Crossref | Google Scholar |
Schrautemeier B, Böhme H, Böger P (1985) Reconstitution of a light-dependent nitrogen-fixing and transhydrogenase system with heterocyst thylakoids. Biochimica et Biophysica Acta (BBA) – Bioenergetics 807, 147-154.
| Crossref | Google Scholar |
Schuller JM, Saura P, Thiemann J, Schuller SK, Gamiz-Hernandez AP, Kurisu G, Nowaczyk MM, Kaila VRI (2020) Redox-coupled proton pumping drives carbon concentration in the photosynthetic complex I. Nature Communications 11, 494.
| Crossref | Google Scholar |
Selinski J, Scheibe R (2019) Malate valves: old shuttles with new perspectives. Plant Biology 21, 21-30.
| Crossref | Google Scholar |
Sevanto S, McDowell NG, Dickman LT, Pangle R, Pockman WT (2014) How do trees die? A test of the hydraulic failure and carbon starvation hypotheses. Plant, Cell & Environment 37, 153-161.
| Crossref | Google Scholar |
Shikanai T (2014) Central role of cyclic electron transport around photosystem I in the regulation of photosynthesis. Current Opinion in Biotechnology 26, 25-30.
| Crossref | Google Scholar |
Shikanai T (2016) Chloroplast NDH: A different enzyme with a structure similar to that of respiratory NADH dehydrogenase. Biochimica et Biophysica Acta (BBA) – Bioenergetics 1857, 1015-1022.
| Crossref | Google Scholar |
Shikanai T (2024) Research on photosynthetic oscillations supports the classical concept of cyclic electron transport producing ATP. Journal of Experimental Botany 75, 667-669.
| Crossref | Google Scholar |
Simkin AJ, López-Calcagno PE, Raines CA (2019) Feeding the world: improving photosynthetic efficiency for sustainable crop production. Journal of Experimental Botany 70, 1119-1140.
| Crossref | Google Scholar |
Steinbeck J, Ross IL, Rothnagel R, Gäbelein P, Schulze S, Giles N, Ali R, Drysdale R, Sierecki E, Gambin Y, Stahlberg H, Takahashi Y, Hippler M, Hankamer B (2018) Structure of a PSI–LHCI–cyt b6f supercomplex in Chlamydomonas reinhardtii promoting cyclic electron flow under anaerobic conditions. Proceedings of the National Academy of Sciences 115, 10517-10522.
| Crossref | Google Scholar |
Stensjö K, Ow SY, Barrios-Llerena ME, Lindblad P, Wright PC (2007) An iTRAQ-based quantitative analysis to elaborate the proteomic response of Nostoc sp. PCC 7120 under N2 fixing conditions. Journal of Proteome Research 6, 621-635.
| Crossref | Google Scholar |
Stepien P, Johnson GN (2018) Plastid terminal oxidase requires translocation to the grana stacks to act as a sink for electron transport. Proceedings of the National Academy of Sciences 115, 9634-9639.
| Crossref | Google Scholar |
Strand DD, Walker BJ (2023) Energetic considerations for engineering novel biochemistries in photosynthetic organisms. Frontiers in Plant Science 14, 1116812.
| Crossref | Google Scholar |
Strand DD, Fisher N, Kramer DM (2017) The higher plant plastid NAD(P)H dehydrogenase-like complex (NDH) is a high efficiency proton pump that increases ATP production by cyclic electron flow. Journal of Biological Chemistry 292, 11850-11860.
| Crossref | Google Scholar |
Strand DD, Livingston AK, Satoh-Cruz M, Froehlich JE, Maurino VG, Kramer DM (2015) Activation of cyclic electron flow by hydrogen peroxide in vivo. Proceedings of the National Academy of Sciences 112, 5539-5544.
| Crossref | Google Scholar |
Sugimoto K, Okegawa Y, Tohri A, Long TA, Covert SF, Hisabori T, Shikanai T (2013) A single amino acid alteration in PGR5 confers resistance to antimycin A in cyclic electron transport around PSI. Plant and Cell Physiology 54, 1525-1534.
| Crossref | Google Scholar |
Suorsa M (2015) Cyclic electron flow provides acclimatory plasticity for the photosynthetic machinery under various environmental conditions and developmental stages. Frontiers in Plant Science 6, 800.
| Crossref | Google Scholar |
Suorsa M, Järvi S, Grieco M, Nurmi M, Pietrzykowska M, Rantala M, Kangasjärvi S, Paakkarinen V, Tikkanen M, Jansson S, Aro E-M (2012) PROTON GRADIENT REGULATION5 is essential for proper acclimation of Arabidopsis photosystem I to naturally and artificially fluctuating light conditions. Plant Cell 24, 2934-2948.
| Crossref | Google Scholar |
Tagawa K, Tsujimoto HY, Arnon DI (1963) Role of chloroplast ferredoxin in the energy conversion process of photosynthesis. Proceedings of the National Academy of Sciences of the United States of America 49, 567-572.
| Crossref | Google Scholar |
Takabayashi A, Kishine M, Asada K, Endo T, Sato F (2005) Differential use of two cyclic electron flows around photosystem I for driving CO2-concentration mechanism in C4 photosynthesis. Proceedings of the National Academy of Sciences of the United States of America 102, 16898-16903.
| Crossref | Google Scholar |
Tazoe Y, Ishikawa N, Shikanai T, Ishiyama K, Takagi D, Makino A, Sato F, Endo T (2020) Overproduction of PGR5 enhances the electron sink downstream of Photosystem I in a C4 plant, Flaveria bidentis. The Plant Journal 103, 814-823.
| Crossref | Google Scholar |
Tel-Or E, Stewart WDP (1976) Photosynthetic electron transport, ATP synthesis and nitrogenase activity in isolated heterocysts of Anabaena cylindrica. Biochimica et Biophysica Acta (BBA) – Bioenergetics 423, 189-195.
| Crossref | Google Scholar |
Terashima M, Specht M, Naumann B, Hippler M (2010) Characterizing the anaerobic response of Chlamydomonas reinhardtii by quantitative proteomics. Molecular & Cellular Proteomics 9, 1514-1532.
| Crossref | Google Scholar |
Theeuwen TPJM, Lawson AW, Tijink D, Fornaguera F, Becker FFM, Caracciolo L, Fisher N, Kramer DM, Wijnker E, Harbinson J, Aarts MGM (2022) The NDH complex reveals a trade-off that constrains maximising photosynthesis in Arabidopsis thaliana. bioRxiv
| Crossref | Google Scholar |
Thiel T, Hartnett Jr T, Pakrasi HB (1990) Examination of photosystem II in heterocysts of the cyanobacterium Nostoc sp. ATCC 29150. In ‘Current Research in Photosynthesis: Proceedings of the VIIIth International Conference on Photosynthesis Stockholm’, 6–11 August 1989, Sweden. (Ed. M Baltscheffsky) pp. 291–294. (Springer)
Thiel K, Patrikainen P, Nagy C, Fitzpatrick D, Pope N, Aro E-M, Kallio P (2019) Redirecting photosynthetic electron flux in the cyanobacterium Synechocystis sp. PCC 6803 by the deletion of flavodiiron protein Flv3. Microbial Cell Factories 18, 189.
| Crossref | Google Scholar |
Thomas J-C, Ughy B, Lagoutte B, Ajlani G (2006) A second isoform of the ferredoxin:NADP oxidoreductase generated by an in-frame initiation of translation. Proceedings of the National Academy of Sciences 103, 18368-18373.
| Crossref | Google Scholar |
Tripp HJ, Bench SR, Turk KA, Foster RA, Desany BA, Niazi F, Affourtit JP, Zehr JP (2010) Metabolic streamlining in an open-ocean nitrogen-fixing cyanobacterium. Nature 464, 90-94.
| Crossref | Google Scholar |
Turina P, Petersen J, Gräber P (2016) Thermodynamics of proton transport coupled ATP synthesis. Biochimica et Biophysica Acta (BBA) – Bioenergetics 1857, 653-664.
| Crossref | Google Scholar |
Valladares A, Muro-Pastor AM, Fillat MF, Herrero A, Flores E (1999) Constitutive and nitrogen-regulated promoters of the petH gene encoding ferredoxin:NADP+ reductase in the heterocyst-forming cyanobacterium Anabaena sp. FEBS Letters 449, 159-164.
| Crossref | Google Scholar |
Valladares A, Maldener I, Muro-Pastor AM, Flores E, Herrero A (2007) Heterocyst development and diazotrophic metabolism in terminal respiratory oxidase mutants of the cyanobacterium Anabaena sp. strain PCC 7120. Journal of Bacteriology 189, 4425-4430.
| Crossref | Google Scholar |
Vass I, Cser K (2009) Janus-faced charge recombinations in photosystem II photoinhibition. Trends in Plant Science 14, 200-205.
| Crossref | Google Scholar |
Vermaas WFJ (2001) Photosynthesis and respiration in cyanobacteria. Encyclopedia of Life Sciences. doi:10.1038/npg.els.0001670
von Caemmerer S, Furbank RT (2003) The C4 pathway: an efficient CO2 pump. Photosynthesis Research 77, 191-207.
| Crossref | Google Scholar |
von Caemmerer S, Furbank RT (2016) Strategies for improving C4 photosynthesis. Current Opinion in Plant Biology 31, 125-134.
| Crossref | Google Scholar |
von Caemmerer S, Quick WP, Furbank RT (2012) The development of C4 rice: current progress and future challenges. Science 336, 1671-1672.
| Crossref | Google Scholar |
Wada S, Yamamoto H, Suzuki Y, Yamori W, Shikanai T, Makino A (2018) Flavodiiron protein substitutes for cyclic electron flow without competing CO2 assimilation in rice. Plant Physiology 176, 1509-1518.
| Crossref | Google Scholar |
Walker BJ, Kramer DM, Fisher N, Fu X (2020) Flexibility in the energy balancing network of photosynthesis enables safe operation under changing environmental conditions. Plants 9, 301.
| Crossref | Google Scholar |
Walsby AE (1985) The permeability of heterocysts to the gases nitrogen and oxygen. Proceedings of the Royal Society of London. Series B. Biological Sciences 226, 345-366.
| Google Scholar |
Walter J, Kromdijk J (2022) Here comes the sun: how optimization of photosynthetic light reactions can boost crop yields. Journal of Integrative Plant Biology 64, 564-591.
| Crossref | Google Scholar |
Wang P, Duan W, Takabayashi A, Endo T, Shikanai T, Ye J-Y, Mi H (2006) Chloroplastic NAD(P)H dehydrogenase in tobacco leaves functions in alleviation of oxidative damage caused by temperature stress. Plant Physiology 141, 465-474.
| Crossref | Google Scholar |
Wang S, Li W, Wufuer R, Duo J, Pei L, Pan X (2023) The key role of cyclic electron flow in the recovery of photosynthesis in the photobiont during rehydration of the lichen Cladonia stellaris. Plants 12, 4011.
| Crossref | Google Scholar |
Wasserfallen A, Ragettli S, Jouanneau Y, Leisinger T (1998) A family of flavoproteins in the domains Archaea and Bacteria. European journal of Biochemistry 254, 325-332.
| Crossref | Google Scholar |
Weber APM, von Caemmerer S (2010) Plastid transport and metabolism of C3 and C4 plants—comparative analysis and possible biotechnological exploitation. Current Opinion in Plant Biology 13, 256-264.
| Crossref | Google Scholar |
Whatley FR, Allen MB, Arnon DI (1959) Photosynthesis by isolated chloroplasts: VII. Vitamin K and riboflavin phosphate as cofactors of cyclic photophosphorylation. Biochimica et Biophysica Acta 32, 32-46.
| Crossref | Google Scholar |
Woo KC, Anderson JM, Boardman NK, Downton WJS, Osmond CB, Thorne SW (1970) Deficient photosystem II in agranal bundle sheath chloroplasts of C4 plants. Proceedings of the National Academy of Sciences 67, 18-25.
| Crossref | Google Scholar |
Woodford R, Watkins J, Moore M, Nix SJ, Yee S, Chan KX, Pogson B, von Caemmerer S, Furbank RT, Ermakova M (2024) PGR5 promotes energy-dependent non-photochemical quenching to enable efficient C4 photosynthesis under fluctuating light. bioRxiv
| Crossref | Google Scholar |
Wu A, Brider J, Busch FA, Chen M, Chenu K, Clarke VC, Collins B, Ermakova M, Evans JR, Farquhar GD, Forster B, Furbank RT, Groszmann M, Hernandez-Prieto MA, Long BM, Mclean G, Potgieter A, Price GD, Sharwood RE, Stower M, van Oosterom E, von Caemmerer S, Whitney SM, Hammer GL (2023) A cross-scale analysis to understand and quantify the effects of photosynthetic enhancement on crop growth and yield across environments. Plant, Cell & Environment 46, 23-44.
| Crossref | Google Scholar |
Xu L, Law SR, Murcha MW, Whelan J, Carrie C (2013) The dual targeting ability of type II NAD(P)H dehydrogenases arose early in land plant evolution. BMC Plant Biology 13, 100.
| Crossref | Google Scholar |
Yamamoto H, Takahashi S, Badger MR, Shikanai T (2016) Artificial remodelling of alternative electron flow by flavodiiron proteins in Arabidopsis. Nature Plants 2, 16012.
| Crossref | Google Scholar |
Yamori W, Shikanai T (2016) Physiological functions of cyclic electron transport around photosystem I in sustaining photosynthesis and plant growth. Annual Review of Plant Biology 67, 81-106.
| Crossref | Google Scholar |
Yamori W, Shikanai T, Makino A (2015) Photosystem I cyclic electron flow via chloroplast NADH dehydrogenase-like complex performs a physiological role for photosynthesis at low light. Scientific Reports 5, 13908.
| Crossref | Google Scholar |
Yeremenko N, Jeanjean R, Prommeenate P, Krasikov V, Nixon PJ, Vermaas WFJ, Havaux M, Matthijs HCP (2005) Open reading frame ssr2016 is required for antimycin A-sensitive Photosystem I-driven cyclic electron flow in the cyanobacterium Synechocystis sp. PCC 6803. Plant and Cell Physiology 46, 1433-1436.
| Crossref | Google Scholar |
Yi X-P, Yao H-S, Fan D-Y, Zhu X-G, Losciale P, Zhang Y-L, Zhang W-F, Chow WS (2022) The energy cost of repairing photoinactivated photosystem II: an experimental determination in cotton leaf discs. New Phytologist 235, 446-456.
| Crossref | Google Scholar |
Zehr JP (2011) Nitrogen fixation by marine cyanobacteria. Trends in Microbiology 19, 162-173.
| Crossref | Google Scholar |
Zehr JP, Capone DG (2024) Unsolved mysteries in marine nitrogen fixation. Trends in Microbiology 32, 532-545.
| Crossref | Google Scholar |
Zehr JP, Bench SR, Carter BJ, Hewson I, Niazi F, Shi T, Tripp HJ, Affourtit JP (2008) Globally distributed uncultivated oceanic N2-fixing cyanobacteria lack oxygenic photosystem II. Science 322, 1110-1112.
| Crossref | Google Scholar |
Zhang C, Shuai J, Ran Z, Zhao J, Wu Z, Liao R, Wu J, Ma W, Lei M (2020) Structural insights into NDH-1 mediated cyclic electron transfer. Nature Communications 11, 888.
| Crossref | Google Scholar |
Zhang Q, Tian S, Chen G, Tang Q, Zhang Y, Fleming AJ, Zhu X-G, Wang P (2024) Regulatory NADH dehydrogenase-like complex optimizes C4 photosynthetic carbon flow and cellular redox in maize. New Phytologist 241, 82-101.
| Crossref | Google Scholar |
Zhou Q, Wang C, Yamamoto H, Shikanai T (2021) PTOX-dependent safety valve does not oxidize P700 during photosynthetic induction in the Arabidopsis pgr5 mutant. Plant Physiology 188, 1264-1276.
| Crossref | Google Scholar |
Zhou Q, Yamamoto H, Shikanai T (2022) Distinct contribution of two cyclic electron transport pathways to P700 oxidation. Plant Physiology 192, 326-341.
| Crossref | Google Scholar |
Zhu X-G, Long SP, Ort DR (2010) Improving photosynthetic efficiency for greater yield. Annual Review of Plant Biology 61, 235-261.
| Crossref | Google Scholar |
Zolotareva E, Polishchuk O (2022) Chlororespiration as a protective stress-inducible electron transport pathway in chloroplasts. The Open Agriculture Journal 16, e187433152208151.
| Crossref | Google Scholar |