The suitability of a dynamic coastal lake to support the diadromous fish Galaxias maculatus
Christopher G. Meijer

A
B
C
Abstract
Globally, intermittently closed and open lakes and lagoons (ICOLLs) can constitute highly productive coastal environments that support a range of fisheries. With growing pressures on fish communities, understanding the role of ICOLL management in population dynamics is increasingly important for conservation.
To determine whether the flood-driven management and environmental degradation of New Zealand’s largest coastal lake, Te Waihora–Lake Ellesmere, has created a conflict with the life history of diadromous īnanga, Galaxias maculatus Jenyns, potentially limiting persistence of this species.
The timing of post-larval migration of īnanga was assessed over 2 years (2021 and 2022) and compared with historic patterns of managed lake openings. Immigrating īnanga individuals were tagged to assess subsequent development, before potential reproductive output was quantified using artificial spawning substrates.
Peak migration periods were mismatched with the timing of most lake openings. After quickly transiting into tributaries, īnanga seemingly died within a few months. No spawning was detected.
The mismatch between lake opening management and the annual life history of īnanga prevents the reliable supply of post-larvae needed to sustain a persistent population, with severe environmental degradation being likely to restrict subsequent survival through summer conditions. This study has highlighted the need for management interventions to be tuned to the life histories of at-risk species.
Keywords: diadromy, Galaxiidae, ICOLLs, life history, managed openings, match–mismatch hypothesis, New Zealand, population persistence.
Introduction
Globally, coastal lakes and lagoons are biodiversity hotspots because of their shallow morphology, elevated productivity, and position as a conduit between freshwater and marine ecosystems (Basset et al. 2007; Kruk et al. 2009; Drake et al. 2011; Pérez-Ruzafa et al. 2011). Their high biodiversity is typically supported by high diversity of habitats created by the natural fluxes of these systems, with cyclical changes owing to the mixing of coastal and land-derived processes leading to dynamic local conditions (Laugier et al. 1999; Chacón Abarca et al. 2021). However, this combination also makes coastal lakes and lagoons susceptible to changes from land-based and marine-derived impacts, which affect the functioning of these dynamic systems and potentially make management problematic (Erostate et al. 2022). Therefore, effective management of these ecologically valuable but vulnerable habitats is important for maintaining their role in supporting local biodiversity.
When the seaward connections of coastal lakes and lagoons are ephemeral, creating intermittently closed and open lakes and lagoons (ICOLLs), managed openings are often used to curtail flooding risks to surrounding land and nearby infrastructure. However, the timing and duration of such openings can have profound impacts on the ICOLL and their biodiversity (e.g. Morris and Turner 2010; Schallenberg et al. 2010; Davies-Vollum et al. 2019). For example, diadromous fishes require unimpeded access between marine and freshwater environments to complete their life cycle, and migration barriers limit their persistence in blocked freshwater environments (Rolls 2011). Therefore, an understanding of the match or mismatch between the timing of openings and peak migration periods is a necessary baseline when determining the ecological value of dynamic coastal lake environments for migratory fishes.
Diadromy is not obligatory for all diadromous fishes, with many exhibiting facultative diadromy (e.g. Waters and Wallis 2001; Arai et al. 2006; Heim-Ballew et al. 2020) or displaying some flexibility in the timing of key migrations (e.g. Tibblin et al. 2016). This flexibility could allow individuals or populations to respond to local environmental conditions, thereby capitalising on those conditions to maximise beneficial outcomes or minimise energetic costs (Delgado and Ruzzante 2020). Thus, when forced to migrate in suboptimal condition because of ill-timed opening of intermittent migration pathways, diadromous fishes may potentially extend transition periods between marine and freshwater habitats to maximise the benefits of resource-rich coastal lake environments (Shrimpton 2012). More specifically, the elevated productivity levels of coastal lakes may provide opportunities for under-developed juvenile fishes to spend extended periods within these environments to fuel further development (Sorensen and Hobson 2005). This would be evident through juveniles spending prolonged periods within the brackish environment before entering a tributary, or repeatedly moving between the brackish environment and tributaries to use resources across both habitats (e.g. Murase and Iguchi 2019). Understanding use of coastal lakes and lagoons by immigrating fishes for further development is, therefore, important in the context of migratory fishes responding to managed intermittent migration pathways.
Īnanga (Galaxias maculatus Jenyns) is an amphidromous fish species, widely distributed across the southern hemisphere (McDowall 2010), that can form non-diadromous populations because of a flexible life history. There are several examples of persistent non-diadromous īnanga populations in Australia, Chile, Argentina and New Zealand (Pollard 1971; Macchi et al. 1999; Ling et al. 2001; Barriga et al. 2007; Rojo et al. 2020). These populations have forgone oceanic migration and instead rely on larval development within lakes. Consequently, owing to its underlying flexibility with migration timing and overall life history, īnanga is a model organism to assess whether contemporary management of an ICOLL conforms to the needs of the resident migratory fishes.
Īnanga has an annual life history, so successful spawning must occur every year for a population to replenish (Stevens et al. 2016), although replenishment may be supported or subsidised by other catchments if post-larvae are able to enter from the sea (i.e. persist as a sink population). Spawning generally occurs in brackish, tidally influenced lower reaches of coastal waterways among vegetation inundated during spring tide events (Hickford and Schiel 2013; Stevens et al. 2016). Tide-dependent spawning can become problematic in ICOLLs because fluctuating water levels may not reflect the tidal cycle, instead being driven by interactions between river flows and the duration since the last opening of the lake to the sea (Haines et al. 2006; Hinwood and McLean 2015). In extensive shallow waterbodies, large fluxes in water displacement and associated localised changes in water depth can occur during and after strong winds, potentially providing fluctuating water levels that are needed for spawning. Īnanga spawning can also be triggered by changes in local water levels as a result of rainfall-induced flooding (Pollard 1972; Chapman et al. 2006; Orchard and Schiel 2022). Therefore, to avoid inadvertently creating sink populations, conservation management of migratory fishes in ICOLLs requires a clear understanding of the capacity for self-sustainment through local, as opposed to externally sourced, recruitment.
New Zealand’s largest coastal lake, Te Waihora–Lake Ellesmere, is near New Zealand’s second-largest city, Christchurch, but is situated in a predominantly agricultural catchment. Although historically dominated by native diadromous fishes, including īnanga (see Glova and Sagar 2000; Jellyman and Smith 2008), this lake has undergone significant change since the late 1960s (Gerbeaux and Ward 1991; Schallenberg et al. 2010), most notably severe eutrophication, the loss of the once-dominant macrophyte beds, and a shift to a consistently turbid condition (Gerbeaux and Ward 1991). The impact of sediment inputs is exacerbated by the shallowness of the lake (no more than 2.2 m), with continual resuspension of lakebed sediments during strong winds (Gerbeaux 1993; Hamilton and Mitchell 1996). Phytoplankton productivity in Te Waihora is high owing to nutrient inputs from surrounding land, but turbidity and reduced light levels limit seasonal blooms (Gerbeaux and Ward 1991; Mackenzie 2016). Water quality of this lake is slow to respond (i.e. improve) when the lake is opened to the sea (Schallenberg et al. 2010). As for many coastal ICOLLs, therefore, the contemporary environmental conditions of Te Waihora and the associated management interventions (e.g. the managed opening regime) may have led to changes in its capacity to support its former biodiversity and abundances of species.
For this study, we used Te Waihora as the focal coastal ecosystem to assess the following three key aspects of īnanga life history: (1) the alignment (match–mismatch) of historic lake openings with peak periods of inward migration of post-larval īnanga, (2) the use of lake habitat by migrating post-larval īnanga before entering tributaries, and (3) the location of īnanga spawning sites within an ICOLL and its tributaries. Specifically, for (1), we adapted the match–mismatch hypothesis proposed by Cushing (1986), which compares the timings of plankton production and hatching of larval fish to infer recruitment success, to determine whether misalignment between timings of lake openings and migrations of post-larval īnanga were responsible for īnanga abundance in Te Waihora and its tributaries. For (2), we hypothesised that īnanga could prolong migration through the lake to capitalise on its high productivity, to support initial growth and development before entering the tributaries. For (3), we hypothesised that īnanga spawning locations would be clustered around the lower reaches of the tributaries, but potentially limited by suitable spawning vegetation.
Materials and methods
Connections between lake openings and īnanga life history
Data for historic lake openings, from c. 1920 to present were provided by Environment Canterbury. Data for openings prior to 1950 were excluded because of incomplete or inconsistent data entries. A theorised distribution was created for the phenology of īnanga migration in Canterbury by using previous galaxiid migration work in New Zealand (McDowall 1968; McDowall 1995), and calibrated using abundance data collected by surveying each opening event between June 2021 and October 2022.
For each opening event, nine double-winged fyke nets (3 m long × 0.5 m deep, with 3-m wings and 1-mm mesh) were positioned along the western shore near the outlet channel to sample migrating post-larval īnanga (Fig. 1a, b). To minimise the effects of any difference in tidal surges, sampling occurred when lake height dropped below 0.9 m above sea level (using the 1937 Lyttelton vertical datum); above this level, high tides were observed to slow the flow of water through the lake opening. Sampling also occurred in daylight hours and for at least 3 h on the incoming tide, when the magnitude of īnanga migrations is typically greatest (see McDowall and Eldon 1980). For each opening event, sampling was completed over 3 calm days to minimise weather-related impacts on post-larvae catch rates (e.g. differences in tidal surges through the opening owing to strong winds). If the catch of īnanga post-larvae was inconsistent across the 3 days (i.e. daily catch was either excessively high or low), sampling was repeated until an apparent baseline for īnanga abundance during each opening could be identified. For each successful sampling day, the individual catches of all nine nets were combined into a cumulative catch to remove the large variability and inherent interdependence of capture rates among the nine nets on the basis of their positions in relation to the lake opening (Fig. 1b).
(a) Map of Te Waihora–Lake Ellesmere, showing the position of the lake outlet during artificial openings, the post-larvae sampling area (blue mark near lake outlet) and the placement sites for straw bales (red markers). (b) Area near lake outlet (striped area), showing the positioning of the nine nets used to survey influxes of post-larval īnanga. (c) Map of nearby Banks Peninsula to highlight the relative proximity of the two spawning comparison sites (Peraki Bay Stream and Barrys Bay Stream). Inset shows the location of Te Waihora within New Zealand.
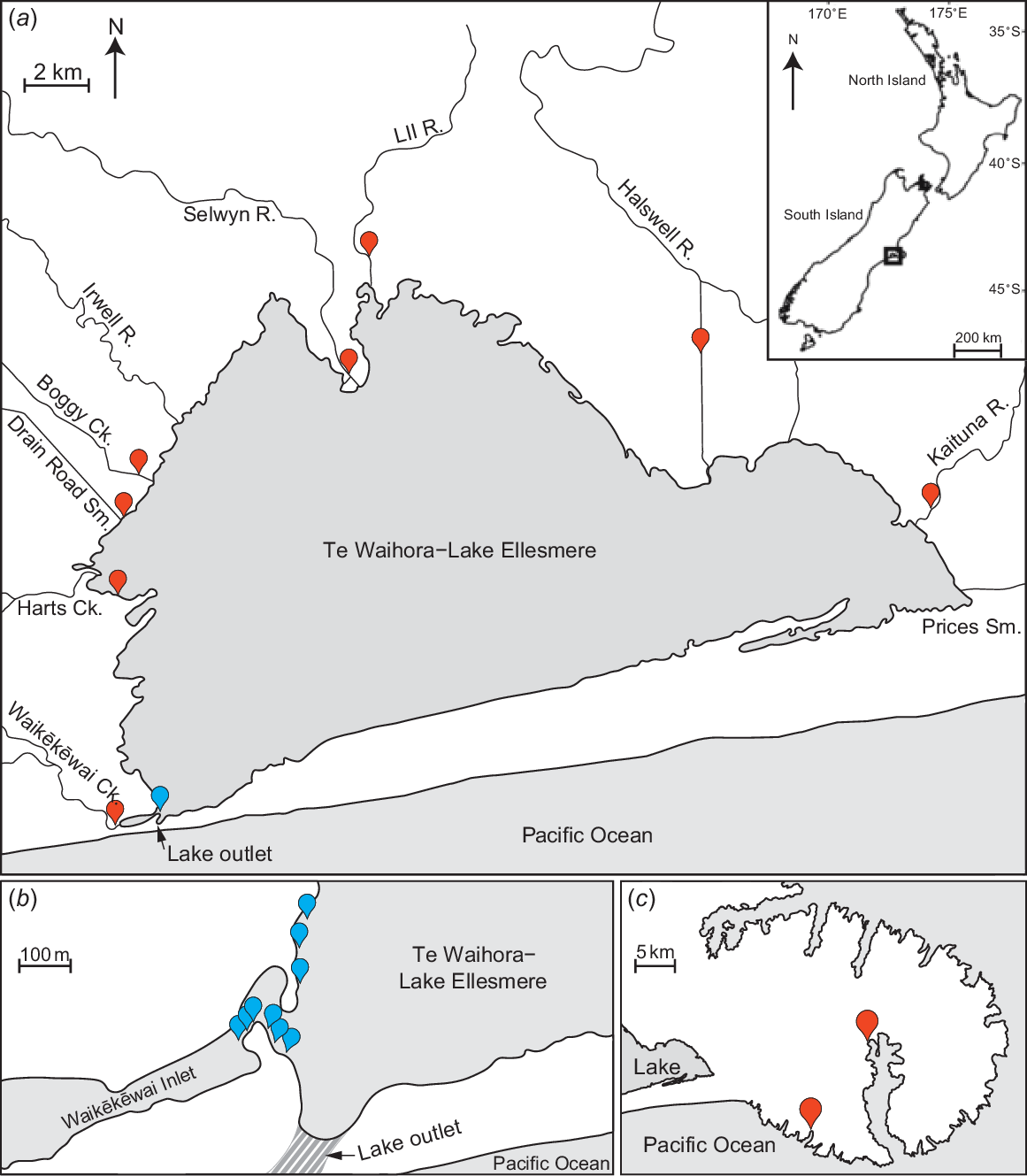
Lake opening data were grouped into weekly bins, where an opening event was deemed to have included a week if it was open for at least 1 day of that week (e.g. a week was still included if the opening closed on Day 2). A histogram was used to visualise any potential bias in the timing of lake-opening events. The lake-opening histogram was compared with a theorised distribution for the phenology of post-larval īnanga migration to identify any potential mismatch between the distributions. Using the ‘lme4’ R package (ver. 1.1-33, see https://CRAN.R-project.org/package=lme4; Bates et al. 2015), a generalised linear mixed-effects model with a Poisson distribution was used to compare differences in relative īnanga abundance among lake openings, and a linear mixed-effects model was used to identify changes in bodyweight, as a proxy for body condition, with īnanga size and lake-opening timing. ‘Year’ was included as the random effect to account for any potential annual variation.
To assess impacts of opening duration and timing on population size, records for historic īnanga abundance in and around Te Waihora were collated from the New Zealand Freshwater Fish Database (National Institute of Water and Atmospheric Research 2022), with the focus on fish caught between November and May, inclusive, using passive sampling methods. These months were selected to cover the entirety of time between expected migration into freshwater (pre-November) and senescence following spawning (which is likely to occur between March and May) for typical īnanga populations. A generalised linear model with a quasi-Poisson distribution (to account for overdispersion) was used to compare the mean number of īnanga caught per site in these historic lake surveys against the proportion of time the lake was opened during the preceding peak migration period (1 September to 31 October) for each year.
Use of Te Waihora by migrating post-larvae
In September 2021, following the detection of large shoals of īnanga post-larvae entering Te Waihora through the lake opening, all tributaries were surveyed for īnanga twice weekly with double-winged (see above) and larger single-winged fyke nets (5 m long × 0.7 m deep, with 5-m wing and 4-mm mesh). To prevent larger fishes, such as eels, from entering the fyke nets and causing intra-net predation, a 20-mm-mesh exclusion grid was fitted across the entrance of the nets. As much as practical, the wing of each fyke net was positioned to intercept all water flow within a tributary, with the cod-end in deeper or shaded sections of the tributary to minimise potential mortality from excessive temperature on warmer days. Fyke nets were left in situ for 24 h, with all captured īnanga being released after measurements were taken. The two tributaries where the largest shoals of īnanga post-larvae were caught (i.e. LII River and Kaituna River; Fig. 1a) were resurveyed longitudinally at least monthly until March, with five sites along the LII River and 10 sites along the Kaituna River. Lengths and weights of 50 īnanga individuals from each tributary were recorded in each sampling round. Captured īnanga individuals were anaesthetised with Aqui-S (at 20 mg of Aqui-S per litre of water), and total length and weight were recorded to the nearest 1 mm and 0.1 g. Fish were then placed into a container of stream water to recover and released once equilibrium was maintained. For sampling rounds with successful īnanga catches, potential differences in fish condition were analysed with a mixed-effects model, with tributary included as a random factor. However, because of the large variation in catch sizes, all abundances were fourth-root-transformed and compared among dates with a one-way ANOVA (‘stream’ was removed because it was not a significant predictor).
To assess how quickly immigrating post-larvae moved from the lake outlet into the tributaries, a tag and recapture program was initiated in August 2022. Over 2 days, all post-larval īnanga caught at the lake outlet were tagged (stained) by placing them into solutions of either neutral red (Day 1) or bismarck brown (Day 2). By using methods adapted from Allibone (2012), the stains were mixed on site by using pre-weighed portions to achieve 0.15 g L−1 of bismarck brown or 0.05 g L−1 of neutral red; both stain solutions were buffered with sodium bicarbonate (1 g L−1). Post-larvae were immersed for 10 min in a stain solution and then released at the place of capture, with a subset of 20 from each day being returned to the laboratory to assess stain longevity and mortality. A control group of 20 unstained individuals was also retained to eliminate potential lethal and sublethal effects that arose from capture and retention in the laboratory (i.e. were not a result of staining). All retained post-larvae were kept in 20-L buckets of aerated lake water. The buckets were housed in a chilled laboratory room (~14°C air temperature) and post-larvae were fed approximately every 12 h with TetraMin Tropical Flakes. Prior to feeding, any deceased individuals were removed, measured, weighed, and tallied. After 96 h, when all traces of the stains were gone (Supplementary Fig. S1), all surviving post-larvae were euthanised in a solution of Aqui-S (at 200 mg of Aqui-S per litre of water). Total length and weight were measured to the nearest 0.01 mm and 0.01 g, and ANCOVA was used to compare the length–weight relationships of post-larvae between the two stains and the control group.
On the basis of the approximate rates at which post-larval īnanga entered major tributaries following the lake opening on 21 September 2021, the transit time of stained post-larval īnanga within Te Waihora was estimated by sampling the four nearest tributaries (i.e. Waikēkēwai Creek, Harts Creek, Drain Road Stream and Boggy Creek; Fig. 1a) for 3 days following staining. Again, double-winged (1-mm mesh) and single-winged (4-mm mesh) fyke nets were used to effectively cover all potential passages of fish travelling upstream, with the nets left in situ over 4 days but checked every 24 h. Captured post-larval īnanga individuals were placed into a white container to identify stained individuals. Stained post-larvae, and up to 30 unstained individuals, were measured and weighed to assess potential sublethal effects of staining under field conditions. ANCOVA was used to compare the length–weight relationships of post-larvae between stained and unstained groups from these four tributaries.
All sampling procedures were approved by the University of Canterbury Animal Ethics Committee (permit number 2021/19R), with the collection of post-larval īnanga for research purposes permitted by the Department of Conservation.
Assessment of īnanga spawning in Te Waihora
Waikēkēwai Creek contained the only confirmed historic īnanga spawning site in the tributaries of Te Waihora, although spawning has not been confirmed there since the removal of critical riparian spawning habitat in May 1990 (see Taylor et al. 1992). We specifically targeted this site on Waikēkēwai Creek alongside equivalent sites (i.e. less than 4 km from the lake) on the other seven tributaries.
To identify any movements or aggregations of īnanga potentially linked to spawning, the lowest section of all tributaries of Te Waihora were surveyed monthly using single-winged fyke nets set along the river margins in 2021 and 2022. This was undertaken from August until March, when survey frequency then increased to fortnightly to maximise the likelihood of detecting a īnanga spawning aggregation. For all surveys, there was an intention to survey more intensively along any tributaries where potential spawning-driven īnanga aggregations were detected.
By March 2021, no spawning aggregations had been detected, with only seven īnanga individuals being caught (between January and March) across three tributaries. Subsequently, the artificial spawning habitats developed by Hickford and Schiel (2013) to attract egg deposition were adopted, with two sets of three straw bales being deployed at each identified site. The bales were positioned side-by-side and secured by two steel fence posts connected by fencing wire, with the front face of the bales positioned ~20 cm into the water to allow for spawning that is not necessarily associated with increases in water level (Supplementary Fig. S2). Twelve sets of artificial spawning habitats were situated along tributaries (two sets at one site on each of six tributaries), where local water levels were affected by any change in overall lake levels (Fig. 1a). Two additional sets of artificial spawning habitats were positioned along the western shores of the lake itself, near Harts Creek, in the area of high īnanga densities identified by Glova and Sagar (2000). All artificial spawning habitats were checked fortnightly alongside ongoing fish surveys to detect potential spawning aggregations, providing an alternative way to detect the presence of īnanga if eggs were deposited between fishing surveys.
In 2022, two potential spawning aggregations of adult inanga were caught in early March in Boggy Creek and Waikēkēwai Creek (12 and 8 fish respectively; Fig. 1a). No other īnanga individuals were caught in the other six tributaries. Eight sets of artificial spawning habitats were deployed along these two tributaries and checked fortnightly during fish surveys. Note that the fortnightly fish surveys were also completed in the other four tributaries where artificial spawning habitats were not deployed. As comparison sites, two streams on Banks Peninsula (Peraki Bay Stream and Barrys Bay Stream; Fig. 1c) were selected on the basis of their similar agriculture-dominated catchments, with eight sets of artificial spawning habitats being placed along each. Again, the presence of eggs would indicate that īnanga was present within the tributary, but potentially missed by fortnightly surveys. All data analyses were run in R (ver. 4.3.1, R Foundation for Statistical Computing, Vienna, Austria, see https://www.r-project.org/), using the R-Studio interface (ver. 2023.6.0.421, Posit Software, PBC, Boston, MA, USA, see https://posit.co/products/open-source/rstudio/).
Results
Connections between lake openings and īnanga life history
The phenology of īnanga post-larval migrations into Te Waihora matched those identified by McDowall (1968), with a peak in inward migration during September and October. Greater catches of post-larval īnanga occurred during September openings (~450 post-larvae per 3 h; Fig. 2a) than in June (40 post-larvae), July (60 post-larvae) and August (240 post-larvae; χ2 = 1706.2, d.f. = 3, P < 0.001). Furthermore, the September 2022 opening also yielded the largest catch across both years, with almost 15 000 īnanga post-larvae caught over 3 h on 29 September 2022. The size of this migration event was ~33 times larger than numbers caught on days either side during this same opening event. However, lake openings have historically been more common in July and August (Fig. 2b) to reduce lake levels following flood events. The lake was open during these months 40–50% of previous years compared with 20–30% for September–October.
Temporal variability in (a) the mean (±s.e.) number (per 3-h sampling) of īnanga post-larvae entering Te Waihora during the incoming tide and (b) how this typically has not aligned with historic lake openings (1950–2022). Sampling was undertaken across 3 days during any opening events in 2021 (closed circles) and 2022 (open circles). In (b), lake openings (bars) are presented as proportions of total years. A line of best fit was created by fitting a normal distribution in migration magnitude that matched the relative abundance of īnanga post-larvae. This line assumes that migration rates peak in late September but are high throughout September and October, as supported by McDowall (1968, 1995). Note that the assumed decline in migration rates (dashed line) was unable to be validated with empirical data but was formed by inversing the observed increase from July to September. This line is presented in both panels, with catch values from (a) converted to likelihood values in (b) by using the upper standard error value for September 2022 as the 100% baseline.
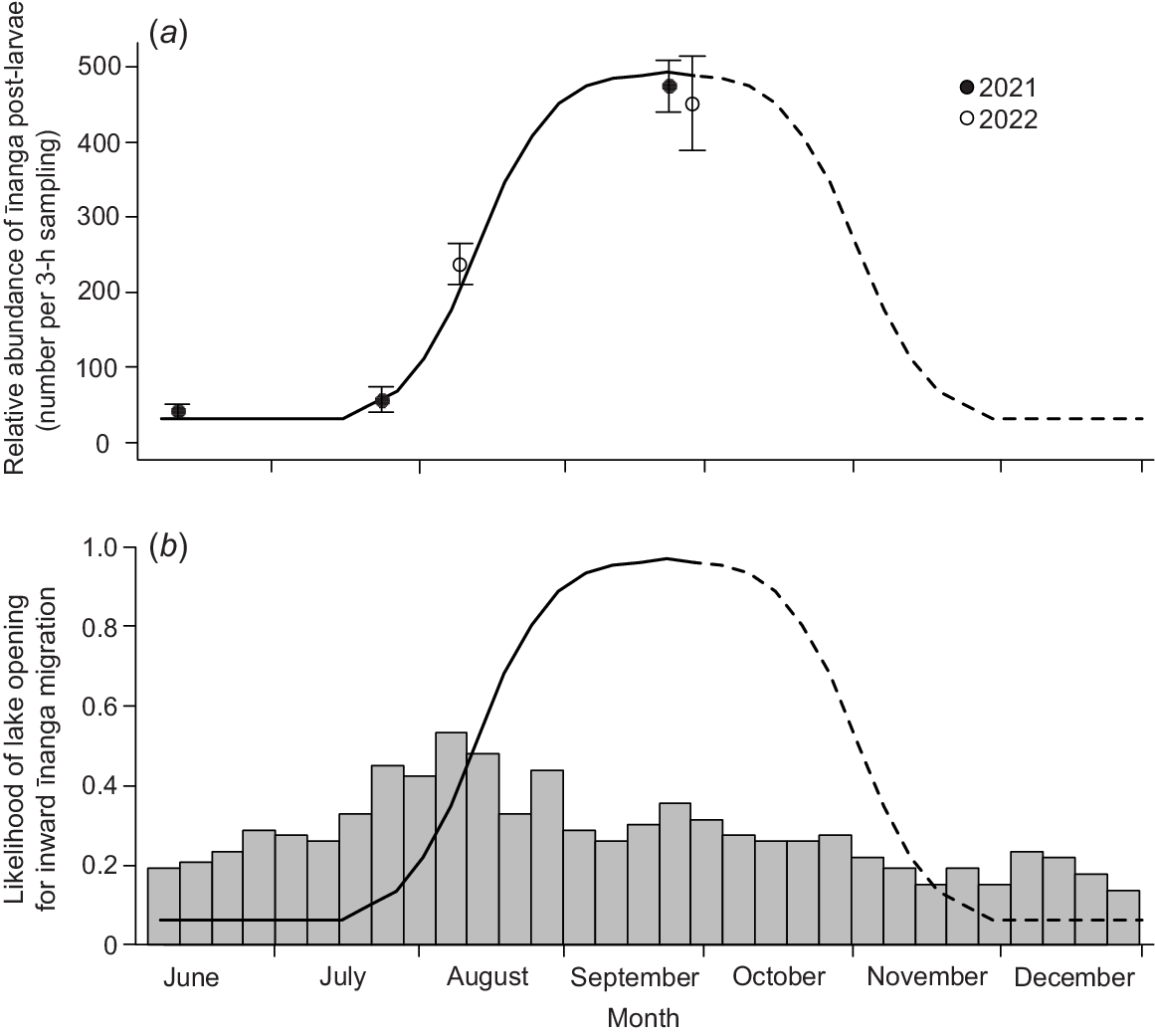
Post-larvae entering the lake during the June opening were significantly shorter than those caught in later months (F3,558.5 = 106.2, P < 0.001). For body condition, there was a significant interaction between body length and the timing of the opening, with the largest post-larvae caught in September being in best condition overall (F3,552.84 = 6.7, P < 0.001; Fig. 3). It is likely that this change in post-larvae condition underpinned the historic connections between population size of adult īnanga and the duration and timing of a lake opening, where longer openings during the peak migration period (September and October) correlated with an increased īnanga abundance in tributaries over the following months (F1,12 = 7.9, P = 0.017; Fig. 4).
Mixed-effects model prediction and 95% confidence interval for change in post-larval īnanga weight owing to total body length and the timing of lake opening. Each point represents an individual post-larval īnanga caught during one of five Te Waihora opening events (June, July and September 2021, and August and September 2022).
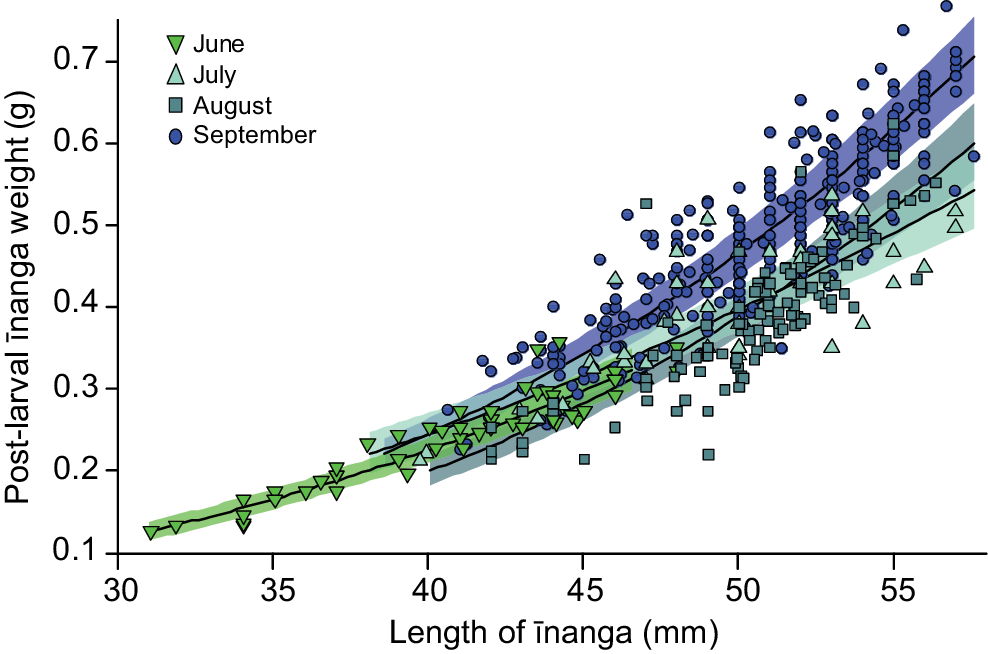
Model prediction and 95% confidence interval for an increase in īnanga abundance with longer lake openings during the peak migration period. Points represent the mean (±s.e.) abundance reported for each survey or year, with aggregate means used for years that had multiple independent surveys (e.g. separate surveys conducted by different organisations).
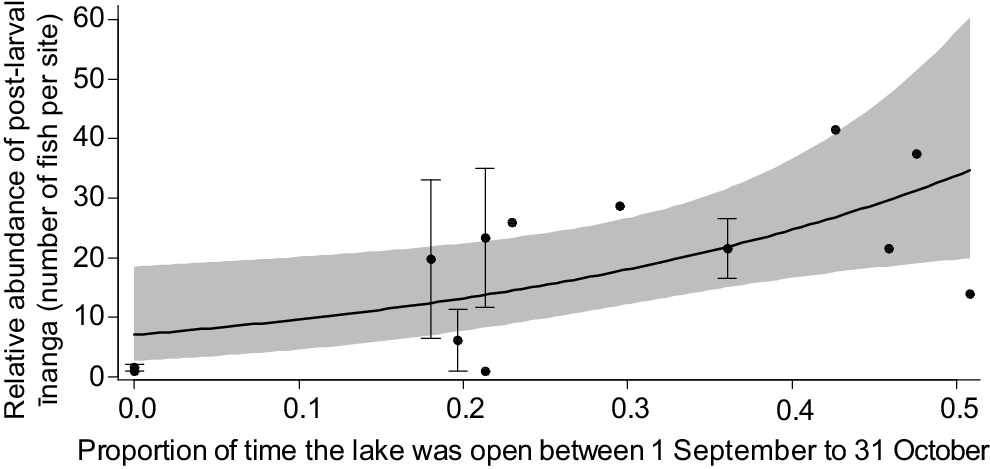
Use of Te Waihora by migrating post-larvae
Within 2 weeks of the lake opening on 21 September 2021, īnanga post-larvae were caught entering all surveyed tributaries of Te Waihora. The time taken for post-larvae to appear in the tributaries ranged from 3 to 14 days after the opening (Supplementary Table S1). Abundance (F3,45 = 5.1, P = 0.003; Fig. 5a) and overall length-dependent condition (F3,247.47 = 27.8, P < 0.001; Fig. 5b) of īnanga in LII River and Kaituna River initially increased and then declined over the following months, with no fish being caught after 8 December.
(a) Mean (±s.e.) īnanga post-larvae abundance, and (b) mixed-effects model fits and 95% confidence interval for length–weight relationship for Kaituna River and LII River, following a lake opening in September 2021. Note that first sampling (4 October) reflects the last day for quantifying shoals of īnanga post-larvae moving through the lower reaches of eight tributaries (see Table S1); so, only two sites were surveyed in each river on that day. Importantly, no īnanga individuals were found in either river after 8 December.
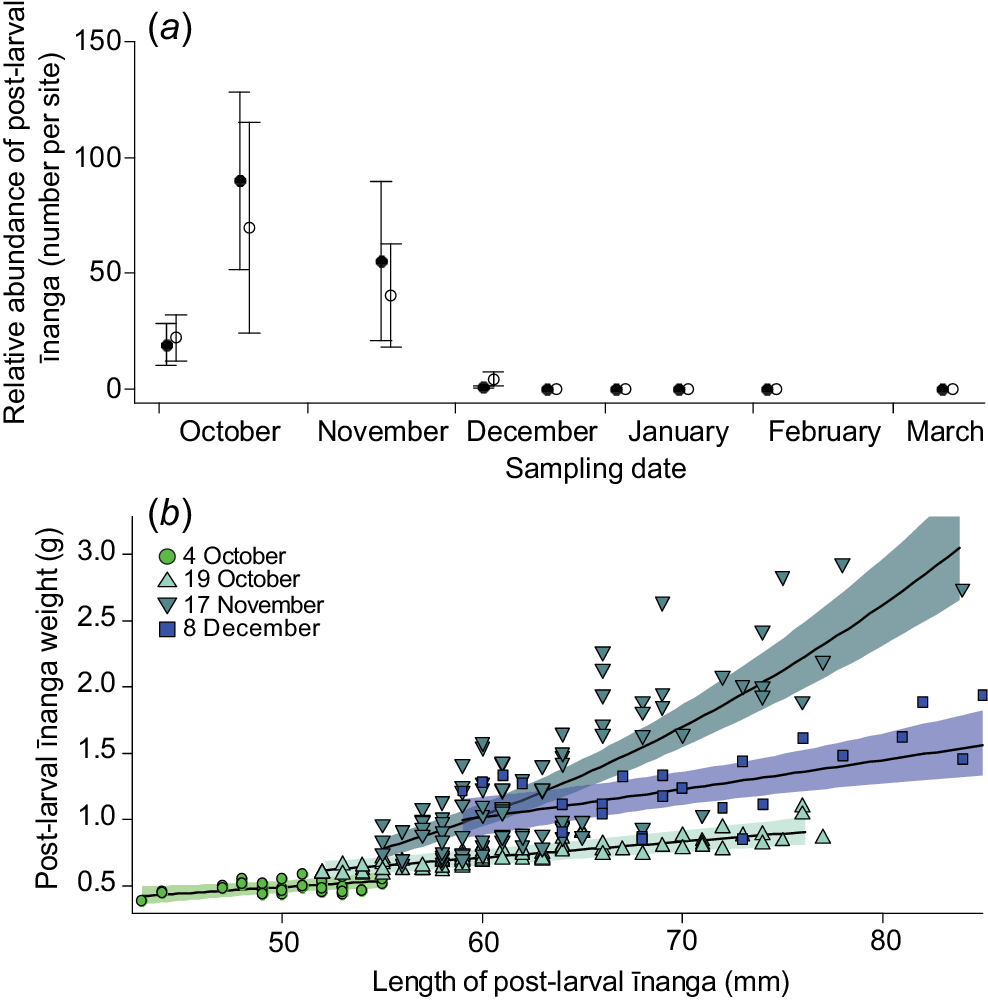
Stained īnanga post-larvae were caught in the closest tributary (Waikēkēwai Creek; Fig. 1a) within 24 h of release at the outlet. Stained post-larvae were found in all four surveyed tributaries within 3 days (Supplementary Table S2). The type of stain had no effect on the length–weight regressions for laboratory-housed post-larvae (F2,49 = 0.9, P = 0.40; Supplementary Fig. S3a) or field-caught post-larvae (F2,134 = 0.7, P = 0.50; Fig. S3b). However, there was a potential difference in mortality after 96 h, with the deceased proportion for neutral red and bismarck brown at 25 and 10% respectively, compared with zero deaths in the control (Table S3).
Assessment of īnanga spawning in Te Waihora
In 2021, following the deployment of straw bales in the eight road-accessible tributaries, no īnanga individuals were caught in the fortnightly surveys. No evidence of spawning was observed in the artificial spawning habitats before the bales were degraded during flooding following a significant rainfall event from 29 to 31 May 2021.
In 2022, following the deployment of artificial spawning habitats in the four streams (two for Te Waihora tributaries and two on Banks Peninsula), no īnanga shoals were detected in the Te Waihora tributaries, although small shoals (50–100 individuals) were seen in both Banks Peninsula streams in early April. Despite the lack of prominent aggregations typically associated with spawning, four gravid female īnanga individuals (95–110 mm long) were caught in Boggy Creek in April 2022. When revisiting the site 2 weeks later, two gravid īnanga individuals were found, of size similar to that of the individuals found in early April. However, neither individual appeared able to swim freely, with significant fungal growths that encased most of their gut cavity. Again, in 2022, the straw bales were degraded beyond a useable state by flooding from significant rainfall event between 17 and 19 July. No īnanga eggs were found in the two tributaries of Te Waihora, but eggs were identified in both Banks Peninsula streams in mid-April.
Discussion
Coastal lakes and lagoons constitute important biodiversity hotspots, but anthropogenic degradation and mismanagement are likely to have diminished their capacity to support historic levels of biodiversity. For Te Waihora, there are many reasons why īnanga does not or cannot form a persistent population throughout the network of tributaries. This includes the mismatch in openings to the sea relative to major influxes of post-larvae, and the severe degradation of available habitat. It was clear from the intensive sampling that when large numbers of īnanga enter the lake in spring, they use it only as a transitory pathway to access the network of tributaries; however, even then, only a few individuals remained after 3–4 months. Consequently, there was no sign of any mature adults successfully spawning the following autumn. Because īnanga is an annual species, there is a need for recruitment from internal (i.e. from spawning within the bounds of the lake) or external sources of post-larval recruits (i.e. timely lake openings to coincide with major influxes of post-larvae). Nonetheless, this replenishment through the annual influx of post-larval recruits also means that each year presents a new and potentially different scenario for this lake-tributary system where improvements to the available adult habitat would be likely to lead to immediate responses by the local īnanga population.
The temporal trends in post-larval īnanga influxes are consistent with those reported in earlier work by McDowall (1968, 1995), with a peak in September–October; however, this is the first time this has been specifically quantified for Te Waihora. When considering diadromous populations, these post-larvae are vital for population persistence, particularly for annual species such as īnanga, where the removal or reduction of the post-larval influx for just 1 year could immediately lead to a smaller resident population (Watson et al. 2021). Nonetheless, in congruence with previous New Zealand-wide assertions by McDowall (1995), īnanga post-larvae were still immigrating into freshwater habitats outside of peak periods in diminished numbers and physical condition, likely reducing the likelihood of successfully reaching maturity (see Sogard 1997). Therefore, the mismatch between openings and peak inward migration is in direct conflict with achieving ongoing persistence of diadromous fishes in ICOLLs, as well as coastal habitats, in general.
However, successful immigration of post-larvae into the coastal lake environment did not immediately lead to a persistent population, with individuals transiting quickly into the tributaries, largely disappearing by austral summer. The high productivity within shallow coastal lake habitats (see Drake et al. 2011) is likely to have supported the initial post-migration growth of īnanga. However, īnanga subsequently declined in both condition and abundance by the onset of summer, with larger īnanga individuals exhibiting the biggest reduction in weights. Summer conditions driving environmental changes in both riverine and shallow-lake habitats, such as reduced water levels and increased algal blooms (Mosley 2015), are likely to have contributed to the eventual decline of larger īnanga individuals. The loss of these larger individuals would directly affect ongoing population viability because of reduced reproductive output. Importantly, this coastal lake habitat is experiencing decades of ongoing environmental degradation, including increased habitat homogeneity (i.e. loss of macrophyte beds), turbidity from suspended sediment and excessive algal growth (see Gerbeaux and Ward 1991; Gerbeaux 1993; Schallenberg et al. 2010). This raises the question of whether any refugia were present for developing īnanga to survive the summer dry. Therefore, the lack of individuals surviving to spawning age highlights a key bottleneck on this population, with this coastal lake habitat being likely to form a sink habitat in its current state.
The lack of survival through the summer may explain the lack of spawning detected over two consecutive summers. Despite its typical spawning strategy linked to the tidal cycle and no spawning being detected in this non-tidal coastal system, īnanga can spawn in non-tidal environments. For example, riverine populations spawn in response to fluctuations in water level (Orchard and Schiel 2022), whereas for landlocked lacustrine populations, spawning is linked to the floodwater inundation of riparian vegetation along the lower reaches of tributaries and lakeside margins (Pollard 1971; Chapman et al. 2006; Laurenson et al. 2012). Although these scenarios occur within the tributaries of Te Waihora, the deployment of artificial spawning habitats, a proven substitute for natural vegetation (Hickford and Schiel 2013), did not produce successful spawning. We conclude that, with declining numbers and body condition by onset of summer, īnanga was already diminished to such an extent that the aggregations of mature individuals needed for spawning were unable to be attained.
Confirmed non-diadromous populations of īnanga persist across much of their distribution, in New Zealand and overseas. In northern New Zealand, a small number of lakes support populations of non-diadromous īnanga, namely the Kai Iwi and Poutu lakes (Ling et al. 2001). In Australia, several lowland lakes support non-diadromous īnanga, such as Moates Lake, Lake Bullen Merri and Lake Corangamite (Chapman et al. 2006; Laurenson et al. 2012). In South America, non-diadromous īnanga is found across many freshwater habitats, from Lake Gutiérrez and the Piedra del Águila Reservoir in Argentina (Barriga et al. 2002, 2007) to Laguna Negra, Colico Lake and Llanquihue Lake in Chile (Rojo et al. 2020; Astorga et al. 2022; Ramírez-Álvarez et al. 2022). Although these lakes cover an expansive range in size and productivity, from the deep lakes in Patagonia to the shallow coastal lakes in northern New Zealand (e.g. the 860-km2 Llanquihue Lake in Chile, compared with the 0.44-km2 euthrophic Lake Kaiiwi in northern New Zealand), none is close to the supertrophic nature of Te Waihora and the harsh summertime conditions that may develop, which may explain the lack of non-diadromous īnanga in this lake. Potential causes for absence of a persistent īnanga population include changes to food availability, poor habitat conditions (e.g. low dissolved oxygen and high temperatures), and interactions with other fishes. However, determining the exact cause is outside the bounds of this study and requires further investigation.
Ongoing population persistence is further complicated by the short-lived, high-turnover annual life history of īnanga, where any disruption or disturbance to its life cycle would have immediate effects on population size and the likelihood of persistence. Within New Zealand, three other migratory galaxiids (banded kōkopu, G. fasciatus Gray; giant kōkopu, G. argenteus Gmelin; and kōaro, G. brevipinnis Günther) form non-diadromous populations supported by lake-based larval development and subsequent recruitment (Augspurger et al. 2017; Hicks et al. 2017; David et al. 2019). These species are all longer-lived, perennial species, where population persistence is not as intrinsically linked to annual recruitment as observed with īnanga. These longer-lived species typically require considerably smaller populations to withstand stochastic disturbances than do their short-lived counterparts (Vélez-Espino and Koops 2012), owing to their ability to buffer against variability in larval survival and the resulting recruitment success, avoiding the boom–bust cycle of short-lived species (Goodwin et al. 2006). Thus, to ensure population persistence when managing fish habitat with anthropogenic motives, such as flooding control, there is a need to understand and incorporate the underlying life histories of local fishes into the decision-making process.
Overall, this study has highlighted two key factors, the mismatched lake openings and environmental degradation, leading to this dynamic ICOLL having diminished capacity to support short-lived diadromous fish. However, arguably the most important factor is the annual life history of īnanga, preventing the accumulation of adults over several years and leaving any potential population vulnerable to stochastic disturbance. However, therein also lies the opportunity for change, with short-lived species able to recover quickly following targeted restoration effort or general environmental improvements (Höckendorff et al. 2017; van Treeck et al. 2020). Therefore, although it is unlikely that a persistent, resident population of īnanga will establish under current conditions, corrective action that links anthropogenic management of this dynamic coastal lake to the annual life history of target species, such as aligning opening regimes and improving survival over summer, should lead to rapid and profound responses within the resident population.
Data availability
The data used in this study will be made available upon request to the corresponding author.
Declaration of funding
C. G. Meijer was supported by scholarship jointly funded by University of Canterbury and Environment Canterbury. D. R. Schiel was financially supported by the New Zealand Ministry of Business, Innovation and Employment (C01X1615; https://app.dimensions.ai/details/grant/grant.7565396). The funders had no role in study design, data collection and analysis, decision to publish or preparation of the paper.
Acknowledgements
We are grateful to Ben Crichton and Nixie Boddy for extensive field assistance; Jesse Burns and Zoe Smeele for field and laboratory assistance; the Marine Ecology Research Group for discussion and feedback; two anonymous referees and the Associate Editor Gerry Closs for comments that improved the paper; and Sefeti Erasito (Taumutu Rūnanga), Miriam Clark (Taumutu Rūnanga) and the Mana Moana Environmental Pou (Wairewa Rūnanga) for advice and support regarding indigenous knowledge. We also thank Environment Canterbury and University of Canterbury for financial and logistical support.
References
Arai T, Yang J, Miyazaki N (2006) Migration flexibility between freshwater and marine habitats of the pond smelt Hypomesus nipponensis. Journal of Fish Biology 68, 1388-1398.
| Crossref | Google Scholar |
Astorga MP, Valenzuela A, Segovia NI, Poulin E, Vargas-Chacoff L, González-Wevar CA (2022) Contrasting patterns of genetic diversity and divergence between landlocked and migratory populations of fish Galaxias maculatus, evaluated through mitochondrial DNA sequencing and nuclear DNA microsatellites. Frontiers in Genetics 13, 854362.
| Crossref | Google Scholar | PubMed |
Augspurger JM, Warburton M, Closs GP (2017) Life-history plasticity in amphidromous and catadromous fishes: a continuum of strategies. Reviews in Fish Biology and Fisheries 27, 177-192.
| Crossref | Google Scholar |
Barriga JP, Battini MA, Macchi PJ, Milano D, Cussac VE (2002) Spatial and temporal distribution of landlocked Galaxias maculatus and Galaxias platei (Pisces: Galaxiidae) in a lake in the South American Andes. New Zealand Journal of Marine and Freshwater Research 36, 345-359.
| Crossref | Google Scholar |
Barriga JP, Battini MA, Cussac VE (2007) Annual dynamics variation of a landlocked Galaxias maculatus (Jenyns 1842) population in a northern Patagonian river: occurrence of juvenile upstream migration. Journal of Applied Ichthyology 23, 128-135.
| Crossref | Google Scholar |
Basset A, Galuppo N, Sabetta L (2007) Environmental heterogeneity and benthic macroinvertebrate guilds in Italian lagoons. Transitional Waters Bulletin 1, 48-63.
| Google Scholar |
Bates D, Mächler M, Bolker B, Walker S (2015) Fitting linear mixed-effects models using lme4. Journal of Statistical Software 67, 1-48.
| Crossref | Google Scholar |
Chacón Abarca S, Chávez V, Silva R, Martínez ML, Anfuso G (2021) Understanding the dynamics of a coastal lagoon: drivers, exchanges, state of the environment, consequences and responses. Geosciences 11, 301.
| Crossref | Google Scholar |
Chapman A, Morgan DL, Beatty SJ, Gill HS (2006) Variation in life history of land-locked lacustrine and riverine populations of Galaxias maculatus (Jenyns 1842) in Western Australia. Environmental Biology of Fishes 77, 21-37.
| Crossref | Google Scholar |
Cushing DH (1986) The migration of larval and juvenile fish from spawning ground to nursery ground. ICES Journal of Marine Science 43, 43-49.
| Crossref | Google Scholar |
David BO, Jarvis M, Ӧzkundakci D, Collier KJ, Hicks AS, Reid M (2019) To sea or not to sea? Multiple lines of evidence reveal the contribution of non-diadromous recruitment for supporting endemic fish populations within New Zealand’s longest river. Aquatic Conservation: Marine and Freshwater Ecosystems 29, 1409-1423.
| Crossref | Google Scholar |
Davies-Vollum KS, Zhang Z, Agyekumhene A (2019) Impacts of lagoon opening and implications for coastal management: case study from Muni-Pomadze lagoon, Ghana. Journal of Coastal Conservation 23, 293-301.
| Crossref | Google Scholar |
Delgado ML, Ruzzante DE (2020) Investigating diadromy in fishes and its loss in an -Omics Era. iScience 23, 101837.
| Crossref | Google Scholar | PubMed |
Drake DC, Kelly D, Schallenberg M (2011) Shallow coastal lakes in New Zealand: current conditions, catchment-scale human disturbance, and determination of ecological integrity. Hydrobiologia 658, 87-101.
| Crossref | Google Scholar |
Erostate M, Ghiotti S, Huneau F, Jouffroy D, Garel E, Garrido M, Pasqualini V (2022) The challenge of assessing the proper functioning conditions of coastal lagoons to improve their future management. Science of The Total Environment 803, 150052.
| Crossref | Google Scholar | PubMed |
Gerbeaux P (1993) Potential for re-establishment of aquatic plants in Lake Ellesmere (New Zealand). Journal of Aquatic Plant Management 31, 122-128.
| Google Scholar |
Gerbeaux P, Ward JC (1991) Factors affecting water clarity in Lake Ellesmere, New Zealand. New Zealand Journal of Marine and Freshwater Research 25, 289-296.
| Crossref | Google Scholar |
Glova GJ, Sagar PM (2000) Summer spatial patterns of the fish community in a large, shallow, turbid coastal lake. New Zealand Journal of Marine and Freshwater Research 34, 507-522.
| Crossref | Google Scholar |
Goodwin NB, Grant A, Perry AL, Dulvy NK, Reynolds JD (2006) Life history correlates of density-dependent recruitment in marine fishes. Canadian Journal of Fisheries and Aquatic Sciences 63, 494-509.
| Crossref | Google Scholar |
Haines PE, Tomlinson RB, Thom BG (2006) Morphometric assessment of intermittently open/closed coastal lagoons in New South Wales, Australia. Estuarine, Coastal and Shelf Science 67, 321-332.
| Crossref | Google Scholar |
Hamilton DP, Mitchell SF (1996) An empirical model for sediment resuspension in shallow lakes. Hydrobiologia 317, 209-220.
| Crossref | Google Scholar |
Heim-Ballew H, Moody KN, Blum MJ, Mcintyre PB, Hogan JD (2020) Migratory flexibility in native Hawai’ian amphidromous fishes. Journal of Fish Biology 96, 456-468.
| Crossref | Google Scholar | PubMed |
Hickford MJH, Schiel DR (2013) Artificial spawning habitats improve egg production of a declining diadromous fish, Galaxias maculatus (Jenyns, 1842). Restoration Ecology 21, 686-694.
| Crossref | Google Scholar |
Hicks AS, Jarvis MG, David BO, Waters JM, Norman MD, Closs GP (2017) Lake and species specific patterns of non-diadromous recruitment in amphidromous fish: the importance of local recruitment and habitat requirements. Marine and Freshwater Research 68, 2315-2323.
| Crossref | Google Scholar |
Hinwood JB, McLean EJ (2015) Predicting the dynamics of intermittently closed/open estuaries using attractors. Coastal Engineering 99, 64-72.
| Crossref | Google Scholar |
Höckendorff S, Tonkin JD, Haase P, Bunzel-Drüke M, Zimball O, Scharf M, Stoll S (2017) Characterizing fish responses to a river restoration over 21 years based on species’ traits. Conservation Biology 31, 1098-1108.
| Crossref | Google Scholar | PubMed |
Kruk C, Rodríguez-Gallego L, Meerhoff M, Quintans F, Lacerot G, Mazzeo N, Scasso F, Paggi JC, Peeters ETHM, Marten S (2009) Determinants of biodiversity in subtropical shallow lakes (Atlantic coast, Uruguay). Freshwater Biology 54, 2628-2641.
| Crossref | Google Scholar |
Laugier T, Rigollet V, de Casabianca M-L (1999) Seasonal dynamics in mixed eelgrass beds, Zostera marina L. and Z. noltii Hornem., in a Mediterranean coastal lagoon (Thau Lagoon, France). Aquatic Botany 63, 51-69.
| Crossref | Google Scholar |
Laurenson LJB, French RP, Jones P, Ierodiaconou D, Gray S, Versace VL, Rattray A, Brown S, Monk J (2012) Aspects of the biology of Galaxias maculatus. Journal of Fish Biology 81, 1085-1100.
| Crossref | Google Scholar | PubMed |
Ling N, Gleeson DM, Willis KJ, Binzegger SU (2001) Creating and destroying species: the ‘new’ biodiversity and evolutionarily significant units among New Zealand’s galaxiid fishes. Journal of Fish Biology 59, 209-222.
| Crossref | Google Scholar |
Macchi PJ, Cussac VE, Alonso MF, Denegri MA (1999) Predation relationships between introduced salmonids and the native fish fauna in lakes and reservoirs in northern Patagonia. Ecology of Freshwater Fish 8, 227-236.
| Crossref | Google Scholar |
McDowall RM (1995) Seasonal pulses in migrations of New Zealand diadromous fish and the potential impacts of river mouth closure. New Zealand Journal of Marine and Freshwater Research 29, 517-526.
| Crossref | Google Scholar |
Morris BD, Turner IL (2010) Morphodynamics of intermittently open–closed coastal lagoon entrances: new insights and a conceptual model. Marine Geology 271, 55-66.
| Crossref | Google Scholar |
Mosley LM (2015) Drought impacts on the water quality of freshwater systems; review and integration. Earth-Science Reviews 140, 203-214.
| Crossref | Google Scholar |
Murase I, Iguchi K (2019) Facultative amphidromy involving estuaries in an annual amphidromous fish from a subtropical marginal range. Journal of Fish Biology 95, 1391-1398.
| Crossref | Google Scholar | PubMed |
National Institute of Water and Atmospheric Research (2022) New Zealand freshwater fish database. Available at https://niwa.co.nz/information-services/nz-freshwater-fish-database [Verified 5 May 2022]
Orchard S, Schiel DR (2022) Whitebait conservation and protected areas at non-tidal rivermouths: integrating biogeography and environmental controls on īnanga (Galaxias maculatus) spawning grounds. Pacific Conservation Biology 28, 140-153.
| Crossref | Google Scholar |
Pollard DA (1971) The biology of a landlocked form of the normally catadromous salmoniform fish Galaxias maculatus (Jenyns). I. Life cycle and origin. Marine and Freshwater Research 22, 91-124.
| Crossref | Google Scholar |
Pollard DA (1972) The biology of a landlocked form of the normally catadromous salmoniform fish Galaxias maculatus (Jenyns). IV. Nutritional cycle. Marine and Freshwater Research 23, 39-48.
| Crossref | Google Scholar |
Pérez-Ruzafa A, Marcos C, Pérez-Ruzafa IM, Pérez-Marcos M (2011) Coastal lagoons: ‘transitional ecosystems’ between transitional and coastal waters. Journal of Coastal Conservation 15, 369-392.
| Crossref | Google Scholar |
Ramírez-Álvarez R, Contreras S, Vivancos A, Reid M, López-Rodríguez R, Górski K (2022) Unpacking the complexity of longitudinal movement and recruitment patterns of facultative amphidromous fish. Scientific Reports 12, 3164.
| Crossref | Google Scholar | PubMed |
Rojo JH, Fernández DA, Figueroa DE, Boy CC (2020) Phenotypic and genetic differentiation between diadromous and landlocked puyen Galaxias maculatus. Journal of Fish Biology 96, 956-967.
| Crossref | Google Scholar | PubMed |
Rolls RJ (2011) The role of life-history and location of barriers to migration in the spatial distribution and conservation of fish assemblages in a coastal river system. Biological Conservation 144, 339-349.
| Crossref | Google Scholar |
Schallenberg M, Larned ST, Hayward S, Arbuckle C (2010) Contrasting effects of managed opening regimes on water quality in two intermittently closed and open coastal lakes. Estuarine, Coastal and Shelf Science 86, 587-597.
| Crossref | Google Scholar |
Sogard SM (1997) Size-selective mortality in the juvenile stage of teleost fishes: a review. Bulletin of Marine Science 60, 1129-1157.
| Google Scholar |
Sorensen PW, Hobson KA (2005) Stable isotope analysis of amphidromous Hawaiian gobies suggests their larvae spend a substantial period of time in freshwater river plumes. Environmental Biology of Fishes 74, 31-42.
| Crossref | Google Scholar |
Stevens JCB, Hickford MJH, Schiel DR (2016) Evidence of iteroparity in the widely distributed diadromous fish inanga Galaxias maculatus and potential implications for reproductive output. Journal of Fish Biology 89, 1931-1946.
| Crossref | Google Scholar | PubMed |
Tibblin P, Forsman A, Borger T, Larsson P (2016) Causes and consequences of repeatability, flexibility and individual fine-tuning of migratory timing in pike. Journal of Animal Ecology 85, 136-145.
| Crossref | Google Scholar | PubMed |
van Treeck R, Van Wichelen J, Wolter C (2020) Fish species sensitivity classification for environmental impact assessment, conservation and restoration planning. Science of The Total Environment 708, 135173.
| Crossref | Google Scholar | PubMed |
Vélez-Espino LA, Koops MA (2012) Capacity for increase, compensatory reserves, and catastrophes as determinants of minimum viable population in freshwater fishes. Ecological Modelling 247, 319-326.
| Crossref | Google Scholar |
Waters JM, Wallis GP (2001) Cladogenesis and loss of the marine life-history phase in freshwater galaxiid fishes (Osmeriformes: Galaxiidae). Evolution 55, 587-597.
| Crossref | Google Scholar | PubMed |
Watson AS, Hickford MJH, Schiel DR (2021) Freshwater reserves for fisheries conservation and enhancement of a widespread migratory fish. Journal of Applied Ecology 58, 2135-2145.
| Crossref | Google Scholar |