A review of inorganic contaminants in Australian marine mammals, birds and turtles
Chad V. Jarolimek A E , Josh J. King A , Simon C. Apte

A CSIRO Environment, New Illawarra Road, Menai, NSW 2234, Australia.
B Australian Registry of Wildlife Health, Taronga Conservation Society, Mosman, NSW 2088, Australia.
C Centre for Planetary Health and Food Security, School of Environment and Science, Griffith University, Nathan, Qld 4111, Australia.
D Science, Economics and Insights Division, Department of Planning and Environment, Lidcombe, NSW 2141, Australia.
E Present address: School of Mathematical and Physical Sciences, University of Technology Sydney, Ultimo, NSW 2007, Australia.
![]() Chad Jarolimek is Technical Services Manager at the School of Mathematical and Physical Sciences, University of Technology, Sydney, Australia. His background is in environmental analytical chemistry with a strong focus on the ultratrace analysis of inorganic contaminants in environmental matrices. |
![]() Josh King is a Research Scientist at CSIRO Environment, Lucas Heights, Australia. He has expertise in development and application of analytical methods for challenging environmental matrices. His research interests have focussed on the transport and fate of inorganic contaminants in environmental systems. |
![]() Dr Simon Apte is a Senior Principal Research Scientist at CSIRO Environment, Lucas Heights, Australia. His team specialises in the ultratrace analysis of trace elements in aquatic systems including the low level determination of mercury speciation in waters, sediments and biota. One of his main research activities is assessing the impacts on mining on aquatic systems particularly in the Asia-Pacific region. |
![]() Jane Hall is a PhD student in the Southern Ocean Persistent Organic Pollutants Program based within the Environmental Futures Research Institute at Griffith University, Australia. She is also a wildlife health specialist at the Australian Registry of Wildlife Health at the Taronga Conservation Society Australia. In 2016, she was awarded a Churchill Fellowship to investigate ways to improve Australia's capacity to manage wildlife disease incidents. Her research interests span both terrestrial and aquatic species under the One Health paradigm, and current studies are focused on the health of Australian fur seal species, specifically Arctocephalus forsteri. |
![]() Dr Anil Gautam holds a PhD in Chemistry from Macquarie University in Sydney. He has been working in NSW public service sectors for more than 12 years providing scientific advice to NSW government agencies to help them address chemical pollution issues. His area of expertise includes measuring and monitoring chemicals in the environment, analytical chemistry including laboratory management systems, providing scientific advice regarding chemical pollution incident and environmental data management and visualisation. |
![]() Dr Megan Louise Gillmore is a senior scientist in the Environmental Forensics team at the New South Wales (NSW) Department of Planning and Environment. Megan has a PhD in ecotoxicology and her research has primarily focussed on developing tools for assessing the impact of sediment-bound contaminants on the biological health of aquatic ecosystems. In her current role Megan plays a vital role responding to pollution incidents such as chemical spills and wildlife deaths by providing scientific evidence and technical expertise for the protection of the NSW community and environment. |
![]() Christopher Doyle is an ecotoxicologist with the New South Wales (NSW) Department of Planning and Environment where he leads a team of specialist scientists in providing pollution monitoring and impact assessment services to environmental regulators and incident management teams. Christopher has more than 20 years of experience in assessing the presence of chemical pollutants in the environment and has a particular research interest in the accumulation and effects of contaminants in marine wildlife. |
Environmental Chemistry 20(4) 147-170 https://doi.org/10.1071/EN23057
Submitted: 29 May 2023 Accepted: 11 August 2023 Published: 12 September 2023
© 2023 The Author(s) (or their employer(s)). Published by CSIRO Publishing. This is an open access article distributed under the Creative Commons Attribution 4.0 International License (CC BY)
Environmental context. Metal concentrations can build up to potentially harmful levels in marine mammals as they are at the top of the food chain. This review summarises the information available on metal concentrations in marine mammals, birds and turtles from around Australia. Despite large data gaps, the available data suggest that metal concentrations are similar to those encountered in other regions of the world.
Abstract. A comprehensive compilation of the published data for trace element concentrations (metals and metalloids) in Australian marine mammals, birds and turtles is presented. The majority of studies have relied on the utilisation of opportunistically collected samples, animal strandings and bycatch. This has resulted in large gaps in geographical, temporal and species coverage data. For instance, little or no data are available for cetaceans in New South Wales or the Northern Territory, and out of 14 endemic species of dolphins, data only exist for seven species. The aforementioned data gaps make it hard to identify statistically significant trends, a problem compounded by data being reported in the form of ranges without raw data. Trace element concentrations measured in various marine species and their tissue types are extremely variable, with ranges typically spanning several orders of magnitude, but are generally comparable with international data. Trends in contaminant concentrations with tissue type follow generally accepted patterns of behaviour for higher organisms, with the highest mercury concentrations in liver and cadmium in kidney tissues. Herbivores have lower contaminant loadings than carnivores, reflecting the importance of diet, and there are identifiable age-related trends for elements such as mercury. The lack of supporting pathology on dead and stranded animals and data on specimens from uncontaminated locations restrict conclusions on organism health impacts. There have been some attempts to use non-invasive sampling of indicator tissues such as fur, bristle and feathers. However, it is currently difficult to extrapolate these data to estimate contaminant concentrations in major organs. Recommendations for future investigations are made.
Keywords: bioaccumulation, birds, cadmium, lead, marine fauna, mercury, trace elements, turtles.
Introduction
While all environmental biomes may experience the effects of chemical contamination, the marine environment is a major sink for potentially toxic trace elements. Contaminants are bioaccumulated by marine organisms through the intake of food and/or exchange of water and often reach high concentrations in their tissues. In particular, mercury and cadmium are known to bioaccumulate in marine food webs (Luoma and Rainbow 2008). Despite regulatory initiatives, trace elements continue to be a threat to the environment (UN Environment 2019).
Marine mammals are particularly susceptible to contaminant bioaccumulation and biomagnification as many species have relatively long life spans and some are apex predators (UNEP 2010; Jepson et al. 2016). Bioaccumulation of potentially toxic trace elements, such as mercury, cadmium and lead, can occur in tissues such as liver, kidney and muscle (Luoma and Rainbow 2008; UN Environment 2019). Owing to ethical issues, research on contaminants in marine mammals is largely opportunistic, as in almost all jurisdictions, tissue samples are predominantly collected from the marine animals that die along their coasts, with material collected at various stages of decomposition. Frequently, not all relevant tissue types, such as blubber, muscle, liver, kidney and brain, are available for analysis, and basic biological data including species, age and sex is also fragmented. This presents challenges in data interpretation and understanding data trends and bioaccumulation processes.
The last review of this important topic in Australia was conducted over 25 years ago (Kemper et al. 1994) and it was noted that the tissue concentrations of toxic contaminants in marine mammals collected from Australian waters were generally low when compared with many other parts of the world, however, cadmium, lead and mercury concentrations were elevated in some species and across some geographical locations. Since then, at least 33 relevant papers on contaminants in Australian marine fauna have been published. In this review, a comprehensive compilation of published data for inorganic contaminants in marine mammals is presented along with available data for birds and turtles. These animals were also included owing to their abundance and important role in Australian marine ecosystems. The review focusses on trace metals and metalloids that are viewed as potentially harmful environmental contaminants when present at elevated concentrations and are subject to regulation through the application of water quality guidelines. Where possible, trends in the data are examined including comparisons with international data. In addition, the important issue of analytical quality control (QC) is examined. Knowledge gaps are identified and recommendations for future investigations are made.
Methods
A comprehensive literature search of published papers and reports was conducted using Google Scholar, Web of Science, Scopus, ScienceDirect, SpringerLink and in-house library catalogues, and relevant data compiled. Examples of the key words used include: inorganic contaminants in Australian marine mammals, marine mammals, dolphins, whales, seals, cetaceans, pinnipeds, dugongs, manatees, birds, albatross, penguins, feathers, turtles, metals, mercury and cadmium. In addition, relevant reports published by Australian State and Federal agencies were also sourced. The papers and reports included in this review were limited to those in which samples were collected from Australian waters between 1974 and 2022. The resulting data were compiled as a series of spreadsheets (Supplementary material). Where available, the key parameters compiled were location, date, species, sex, maturity/age, tissue concentration plus additional comments provided on sample collection or analysis. Where not already implemented by the original authors, data in this review have been rounded to a maximum of three significant figures, reflecting the realistically achievable accuracy of the analytical methods.
A number of publications did not provide analytical detection limits and reported concentrations of some contaminants as ‘0’ (zero) or ‘ND’ (not detected). These data are reported in the Supplementary material in their original format, however, in the body of this paper, where possible we identified the next lowest reported value from the same study and used this as a substitute value for the limit of detection.
Data synthesis
Data for this review were sourced from 43 published articles and reports comprising approximately 2000 individual samples. The combined data sets were assessed for reporting trends over time (Table 1) and sample distribution across states and territories (Fig. 1). Cetaceans were by far the most sampled fauna, with almost 40% of samples coming from a single season Western Australia (WA) whale harvest in the 1970s (Canella and Kitchener 1992). Ignoring this data spike, sample numbers for marine fauna have generally increased over the last five decades, highlighting the increased awareness of using marine organisms as sentinels of environmental health. Dugongs are perhaps the exception to this trend, with sample numbers peaking in the 1990s, with no dugong trace element data reported since 2000. As illustrated in Fig. 1, the national distribution of fauna types sampled followed the general habitats that the organisms occupy (e.g. dugongs and turtles in Queensland (QLD)) or common stranding locations (e.g. cetaceans in Tasmania).
Time periodA | Cetaceans | Dugongs | Pinnipeds | Seabirds | Marine turtles |
---|---|---|---|---|---|
Pre-1970 | 0 | 0 | 0 | 35 | 0 |
1970s | 414 | 45 | 0 | 1 | 0 |
1980s | 14 | 2 | 0 | 45 | 0 |
1990s | 257 | 3 | 0 | 124 | 55 |
2000s | 68 | 0 | 35 | 109 | 20 |
2010–present | 0 | 0 | 0 | 311 | 171 |
Dates not provided | 157 | 0 | 16 | 127 | 0 |
ATime periods refer to time of sample collection, not publication.
Heat map and pie charts showing fauna types and sample numbers (in brackets) analysed across Australia.
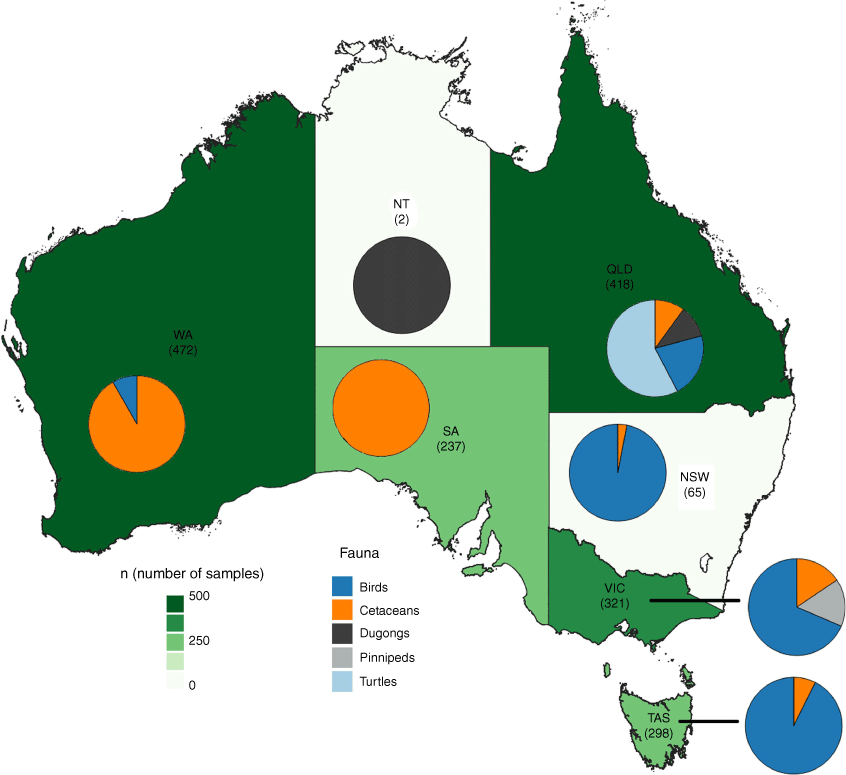
Contaminant concentrations measured in various marine species and their tissue types were extremely variable, with concentration ranges typically spanning several orders of magnitude. Consequently, where possible, data were summarised in this report in the form of ranges. Full data sets as presented in the original publications are collated in the Supplementary material.
Details of methodology and QC information play an important role in supporting the reliability and comparability of analytical data. Desirable QC includes details on methodology, calibration standards (traceability), blanks (to check that the analytical signal is not affected by contamination), precision checks (replicate measurements), accuracy checks using reference materials and spike recoveries.
Using the above QC components, a scoring regime was established for the Australian sourced publications to enable an assessment of QC reported in the studies (Fig. 2). Studies that provided information on 5–6 desirable QC indicators were given an ‘A’ QC score, 3–4 items a ‘B’ QC score, 1–2 items a ‘C’ QC score and zero items a ‘D’ QC score. Most studies (over 80%) received either a ‘C’ or ‘B’ QC score. There were three studies that received a ‘D’ QC score as they did not report any information on QC. These publications were typically the oldest publications, or where data was sourced from unpublished sources such as the contaminants review by Kemper et al. (1994), sperm whale mercury data from the Cheyne Whaling station in WA (Canella and Kitchener 1992) and a cetaceans stranding study where details of the strandings were the focus and not contaminant residue analysis (McManus et al. 1984). The seven studies receiving an ‘A’ QC score were all published after 2012. Over the last 20 years, greater importance has been placed on reporting thorough method descriptions and QC data allowing for much greater confidence in the data and for interstudy comparisons to be conducted, where described methodologies are comparable.
Quality control score of publications on contaminants in Australian marine fauna (1981–2021). See text for explanation of the scoring system.
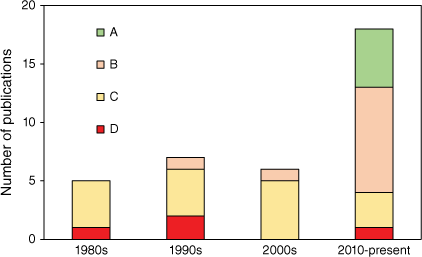
There were significant gaps in QC data, with 22% of all studies stating they had analysed reference materials without providing reference material results. Nineteen percent of studies stated they had analysed spiked samples but did not provide any recovery data, and 95% of studies did not provide information on analytical precision or the frequency of replicate analysis. In addition, a number of studies did not report moisture content making comparisons across studies when either dry or wet weight concentrations are reported difficult. Overall, QC has steadily improved over the last 40 years, however, even in the majority of publications produced since 2010, a complete inventory of QC information is missing with only 28% of the studies conducted providing comprehensive QC data. Analytical methods have also improved with highly sensitive multi-elemental analysis techniques, such as inductively coupled plasma mass spectrometry (ICPMS), replacing atomic absorption spectrometry (AAS). Advances in sample preparation include the widespread use of microwave-assisted sample digestion techniques in place of open beaker digestions. Within the analytical chemistry community it is generally accepted that the improvements in methodology and adoption of more rigorous and extensive QC procedures have improved the accuracy of results. Full adoption of the QC measures listed above is therefore strongly recommended.
Faunal summaries
Data on the concentrations of trace elements in cetaceans sampled in Australian waters were sourced from 13 papers and two reports, covering a period from 1976 to 2020. Twenty species and nine tissue types are represented. Mercury, cadmium and lead were the most reported elements. The ranges of kidney, liver and skin concentrations of key trace elements in cetaceans are presented in Tables 2, 3 and 4, with additional elements and tissues reported in the Supplementary material. For ease of presentation, the data are organised under the common names dolphins and whales in Tables 2 and 3. Note that most data are for odontocetes (dolphins and toothed whales) with only very limited data for a single mysticete (baleen whales) species (Table 3). The species with the highest sample numbers was the sperm whale (Physeter macrocephalus) (n = 416), due to a large number of samples collected during a 1976 whale harvest conducted in WA, which were principally analysed for mercury concentrations in muscle tissue (Canella and Kitchener 1992). Since 1982, most samples were from species such as the common dolphin (Delphinus delphis) (n = 151), Indo-Pacific bottlenose dolphin (Tursiops aduncus) (n = 88) and the false killer whale (Pseudorca crassidens) (n = 38). Most dolphin specimens were obtained from the South Australian Museum, which has been collecting and archiving dolphin specimens that have died from entanglements, intentional killing, disease and natural causes in South Australia (SA) since 1988 (Butterfield and Gaylard 2005).
Common name | Species | Tissue | Element | ||
---|---|---|---|---|---|
Cd | Hg | Pb | |||
Odontocetes | |||||
Bottlenose dolphin | Tursiops truncatus | Liver | <0.01–48.1A,H,D,J (38) | 0.14–1570A,C,H,D,J (41) | 0.01–1A,H,D,J (27) |
Kidney | <0.02–38.7A,B,C,H (41) | 0.27–2H (2) | <0.03–0.04A,H (3) | ||
Muscle | 0.05–0.1A (4) | 0.22–0.77A (4) | 0.05–0.1A (4) | ||
Bone | – | – | <0.6–61.6A,D,J (26) | ||
Indo-Pacific bottlenose dolphin | Tursiops aduncus | Liver | <0.01–100D,J (78) | 0.28–2875D,J (74) | 0.004–15.2D,J (85) |
Muscle | 0.01–0.04I (3) | 0.88–2.22I (3) | – | ||
Bone | – | – | 0.29–16D,J (56) | ||
Burrunan dolphin | Tursiops australis | Liver | – | 100–840F (6) | – |
Blubber | – | 0.32–7.2F (30) | 0.05–12F (30) | ||
Common dolphin | Delphinus delphis | Liver | <0.01–11A,D,H,J (102) | 0.15–165A,D,H,J (79) | <0.04–3A,D,H,J (116) |
Kidney | <0.01–36.2A,B,H (38) | 0.11H (1) | 0.05–0.47A,H (17) | ||
Muscle | 0.06–0.10A (2) | 0.83–1.37A (2) | 0.10–0.22A (2) | ||
Bone | – | – | 0.41–2.4A,D,J (80) | ||
Pantropical spotted dolphin | Stenella attenuata | Liver | 2.7–3.4A(3) | 5.77–30.6A (2) | <0.01A (2) |
Kidney | 9.4A (1) | – | – | ||
Muscle | <0.05A(2) | 0.82–1.01A (2) | 0.05A (2) | ||
Blubber | – | 0.42–0.46A (2) | 0.05–0.06A (2) | ||
Australian humpback dolphin | Sousa sahulensis | Liver | 0.39G (1) | – | 0.04G (1) |
Kidney | <0.004G (1) | – | <0.002G (1) | ||
Muscle | <0.004–0.0076G (4) | – | <0.002–0.061G (4) | ||
Blubber | <0.004G (4) | – | <0.002–0.0025G (4) | ||
Skin | <0.004–0.0066G (5) | 1.67–15.7E (17) | <0.002–0.22G (5) | ||
Snubfin dolphin | Orcaella heinsohni | Skin | – | < 0.1–7.22E (22) | – |
Common name | Species | Tissue | Element | ||
---|---|---|---|---|---|
Cd | Hg | Pb | |||
Odontocetes | |||||
Pilot whale | Globicephala spp. | Liver | <0.4–22A,C (6) | 2.19A (1) | <1A (6) |
Kidney | <0.4–23A,C (5) | 0.51A (1) | <1A (6) | ||
Muscle | – | 0.29–6.2B (6) | – | ||
Melon-headed whale | Peponocephala electra | Liver | 8–21D (3) | 13–141D (3) | <0.04–0.1D (3) |
Kidney | 46–69D (3) | 2.6–5.1D (3) | <0.04D (3) | ||
False killer whale | Pseudorca crassidens | Liver | 0.05–75.8A (38) | 41–479A (38) | 0.05–0.47A (11) |
Kidney | 29.7–106A (37) | 59–143A (26) | – | ||
Muscle | <0.05–0.45A (38) | 5–50A (27) | <0.05A (11) | ||
Blubber | 0.05–2.9A (11) | 0.69–6.21A (11) | <0.05A (11) | ||
Killer whale | Orcinus orca | Liver | <0.4A (1) | 1.52A (1) | – |
Kidney | <0.4A (1) | 0.49A (1) | <0.4A (1) | ||
Sperm whale | Physeter macrocephalus | Liver | 1.16–11.2A (2) | 1.25–34A (2) | <0.05–0.56A (2) |
Kidney | 14.3A (1) | 5.3A (1) | 0.77A (1) | ||
Muscle | <0.05A (1) | 0.23–3.45A,E (416) | <0.05A (1) | ||
Blubber | <0.05A (1) | 0.11A (1) | <0.05A (1) | ||
Skin | – | 1.1–6.9F (19) | 0.30–16.6G (19) | ||
Pygmy sperm whale | Kogia breviceps | Liver | 14.3A (1) | 15.9A (1) | 0.07A (1) |
Blubber | 0.11A (1) | 0.58A (1) | <0.05A (1) | ||
Beaked whale | Mesoplodon spp. | Liver | <0.05–13.5A (4) | 93.4–94.0A (2) | <0.05A (3) |
Kidney | 53.6A (1) | – | – | ||
Muscle | 0.05–0.06A (2) | 0.80–2.69A (3) | <0.05A (2) | ||
Blubber | <0.05A (2) | 0.31–0.52A (2) | 0.05A (2) | ||
Mysticetes | |||||
Pygmy right whale | Caperea marginata | Liver | 1.8–15A (2) | 0.05A (1) | <2A (2) |
Muscle | 0.05A (1) | < 0.01–0.06A,B (11) | <0.05A (1) | ||
Blubber | 0.05A (1) | 0.27A (1) | 0.30A (1) |
Common name | Species | Tissue | Element | ||
---|---|---|---|---|---|
Cu | Se | Zn | |||
Odontocetes | |||||
Bottlenose dolphin | Tursiops truncatus | Liver | 4.9–85A,E (13) | <1–433A,E,H (19) | 26–163A,E,H (19) |
Kidney | 4.7–10E (2) | 1.9–3.4E (2) | 26–40E (2) | ||
Indo-Pacific bottlenose dolphin | Tursiops aduncus | Liver | 0.62–74A (50) | <1–1510A,H (94) | 24–566A,H (94) |
Muscle | 0.96–1.4G (3) | – | 13.1–205G (3) | ||
Burrunan dolphin | Tursiops australis | Blubber | – | 0.52–6.5B (30) | – |
Common dolphin | Delphinus delphis | Liver | 3.0–71A,E (49) | <1–78A,E,H (116) | 26–197A,E,H (116) |
Kidney | 3.9E (1) | 2.6E (1) | 20E (1) | ||
Australian humpback dolphin | Sousa sahulensis | Liver | 4.8C (1) | 68C (1) | 32C (1) |
Kidney | 0.71C (1) | 0.69C (1) | 27C (1) | ||
Muscle | 0.64–0.86C (4) | 0.41–1.5C (4) | 9.9–41C (4) | ||
Blubber | 0.08–0.29C (4) | 0.55–1.6C (4) | 3.1–26C (4) | ||
Skin | 1.1–1.5C (5) | 2.0–9.6C (5) | 27–260C (5) | ||
Pilot whale | Globicephala spp. | Liver | 5.6D (1) | – | 31D (1) |
Kidney | 3.0D (1) | – | 22D (1) | ||
Melon-headed whale | Peponocephala electra | Liver | 2.1–4.9E (3) | 6.2–58E (3) | 22–47E (3) |
Kidney | 2.4–2.9E (3) | 3.4–4.0E (3) | 27–30E (3) | ||
Sperm whale | Physeter macrocephalus | Skin | – | 20.3–125F (19) | – |
Beaked whales | Mesoplodon spp. | Liver | 2.7D (1) | – | 10D (1) |
Discrepancies in sample numbers between species, locations and how studies have categorised age make comparison between studies difficult. Nevertheless, broad intra and inter species trends are apparent, such as generally higher mercury concentrations observed in the livers of odontocetes (range 0.14–2880 mg kg−1) compared to the mysticete (pygmy right whale, 0.05 mg kg−1), most likely reflecting differing dietary sources, and the accumulation of mercury in liver tissue and cadmium in kidney tissue of odontocetes (Tables 2 and 3). No data on mercury concentrations in brain tissue of Australian cetaceans were found, despite the known neurotoxicological effects of mercury on mammalian brains and reported high accumulation of mercury in dolphin brain tissue (López-Berenguer et al. 2020).
Skin sampling of live cetaceans by remote biopsy is a widely used, minimally invasive technique (Noren and Mocklin 2012; Crain et al. 2014; Garrigue and Derville 2022), which has resulted in a recent increase in samples available for more targeted trace element contaminant research (Stavros et al. 2011; Aubail et al. 2013). Some relationships of skin trace element concentration to internal organ concentration in dolphins have been reported in international studies for mercury (Stavros et al. 2011; Aubail et al. 2013) and to a lesser extent arsenic (Sun et al. 2017). Several Australian studies have investigated the use of skin as a biomonitoring tool (Savery et al. 2013, 2014; Weijs et al. 2016; Cagnazzi et al. 2020). Only one of these studies measured skin as well as internal organ concentrations and, due to the opportunistic nature of sampling, liver and kidney concentrations were only available for one animal (Weijs et al. 2016).
Pinnipeds: seals
There are only three publications reporting trace element data for pinnipeds from Australian waters (Bacher 1985, Kemper et al. 1994, Lynch et al. 2012). All data is for Arctocephalus pusillus, which is known by several common names but when used in reference to Australian fur seals is more accurately represented by the subspecies A. p. doriferus. The majority of samples were collected off the Victorian coast. Tissue types include liver, kidney, brain, bone, spleen and hair, for a limited suite of trace elements comprising cadmium, cobalt, mercury, magnesium, nickel, lead and zinc. Data for the most reported elements are presented in Table 5, with additional elements and tissues provided in the Supplementary material.
Common name | Species | Tissue | Non-essential | Essential | ||||
---|---|---|---|---|---|---|---|---|
Cd | Hg | Pb | Cu | Se | Zn | |||
Pinnipeds | ||||||||
Australian fur seal | Arctocephalus pusillus | Liver | 0.76–39.2A (6) | 0.97–170B (16) | – | – | – | – |
Kidney | 5.30–28.7A (4) | 0.13–1.71B (16) | – | – | – | – | ||
Muscle | – | 0.09–1.90B (16) | – | – | – | – | ||
Brain | – | 0.04–2.53B (16) | – | – | – | – | ||
Spleen | – | 0.78–3.80B (16) | – | – | – | – | ||
Bone | – | – | <1–2.3A (4) | – | – | – | ||
HairI | – | 1.07–19.8B (16) | 0.158 ± 0.042C,L (35) | – | – | 155 ± 14C,L (35) | ||
BloodJ | – | 0.28 ± 0.10C,L (12) | – | – | – | – | ||
Sirenian | ||||||||
Dugong | Dugong dugon | LiverK | <0.001–15A,D,E,F,G (125) | <0.001–0.29E,F,H,M (83) | <0.01–0.81A,D,E,F (165) | 1.8–472D,E,F,G (124) | <0.01–1.6E,F (77) | 12–1410D,E,F,G (124) |
Kidney | 0.04–59D,E,G (32) | 0.01–0.05E,H (4) | <0.02–0.07D,E (31) | 0.52–4.4D,E,G (32) | 1.1–7.9E (3) | 14–55D,E,G (32) | ||
Muscle | 0.01–0.05A,D,E,G (30) | 0.002–1.05A,H (5) | 0.03–0.25A,D,E (56) | 0.10–0.71D,E,G (28) | 0.06–0.15E (2) | 0.1–28D,E,G (28) | ||
Blubber | 0.05A (2) | 0.01–0.02A (2) | 0.05A (2) | – | – | – |
AKemper et al. (1994).BBacher (1985); CLynch et al. (2012); DDenton et al. (1980); EGladstone (1996); FHaynes et al. (2005); GMarsh (1989); HDenton and Breck (1981); Img kg−1 dry weight; Jmg L−1 wet weight; KDugong liver results reported on a dry weight basis, were converted into wet weight using a dry:wet factor of 3.8 (Denton et al. 1980); LTwo sets of means and s.d. reported in study pooled; MA suspected outlier value of 65.8 mg kg−1 Hg for dugong liver (Kemper et al. 1994) has been omitted.
Most studies focussed on mercury, cadmium and lead in varying tissue types. Bacher (1985) found the highest mercury concentrations in liver, with mean concentrations 1–2 orders of magnitude higher than those found in kidneys, muscle and spleen. These trends are consistent with international literature (Thompson 1990). The mean hepatic mercury concentration reported by Bacher (1985) of 62 ± 45 mg kg−1 wet weight is at the high end of the range when compared to data for other pinnipeds reported in the international literature (means ranging from 0.2 to 70 mg kg−1 (Thompson 1990; Marcovecchio et al. 1991; Becker et al. 1997; Julshamn and Grahl-Nielsen 2000; Riget et al. 2005; Brunborg et al. 2006; Gerpe et al. 2009)). There was also a clear trend in the Australian data of increasing mercury concentration with seal age in liver (r = 0.975, P < 0.001), spleen (r = 0.799), brain (r = 0.711) and hair (r = 0.760) (Bacher 1985). This is likely influenced by diet, as well as age (Julshamn and Grahl-Nielsen 2000; Gerpe et al. 2009). Age related mercury bioaccumulation is a well-known effect in marine mammals and piscivorous fish owing to detoxification, storage and low excretion rates (Rainbow 2018). The observed increase in liver mercury concentration with age could be a result of formation of insoluble mercuric selenide (HgSe), which can account for over 50% total mercury (Wagemann et al. 2000), and its long body half-life, e.g. up to 500 days in ringed seals (Ewald et al. 2019).
Sirenians: dugongs
Trace element data for dugongs were sourced from four published papers (Denton et al. 1980; Denton and Breck 1981; Marsh 1989; Haynes et al. 2005) and one report from the Great Barrier Reef Marine Park Authority (Gladstone 1996). All of the samples analysed were collected from QLD and the Northern Territory (NT) between 1974 and 2000, with no data for WA. Five tissue types were analysed, with most focus on trace element concentrations in liver samples. Data for the most reported elements are presented in Table 5, with additional elements and tissues in the Supplementary material. Possibly due to their herbivorous diets, a broader range of elements have been targeted for analysis in dugong studies, including both essential (iron, copper and zinc) and non-essential (cadmium, mercury and lead) elements. High concentrations of iron in dugong liver (from 120 to approximately 45 000 mg kg−1 wet weight) were observed, such that haemosiderin deposits were detected in some individuals; a result observed in mammals in cases of prolonged dietary iron ingestion (Denton et al. 1980; Mazzaro et al. 2004; Rosen and Worthy 2018). Dietary related high concentrations of iron storage in the liver of dugongs is well known in the literature, and likely of little pathological significance (Woolford et al. 2015).
Comparatively high concentrations of copper have been measured in dugong livers (up to 472 mg kg−1 wet weight) compared to other marine mammals (e.g. dolphin maximum Cu of 85 mg kg−1 wet weight, Table 4). Similar trends have been found in other sirenians internationally (i.e. Trichechus manatus livers containing up to 240 mg Cu kg−1 wet weight) (O’Shea et al. 1984). Copper concentrations also decreased with age, a trend not unusual in mammals (Denton et al. 1980). Haynes et al. (2005) identified positive correlations between age and liver concentrations of aluminium, cadmium, iron, mercury and zinc in dugongs.
High concentrations of cadmium have also been identified in the kidneys of dugongs (0.04–59 mg kg−1 wet weight) (Table 5). While these concentrations are within the range reported for other marine mammals (Tables 2 and 3), given their herbivorous diet, this trend is unexpected. Given the low cadmium concentration in a seagrass diet (0.14–1.0 mg kg−1 dry weight (Thomas et al. 2020; Waterhouse et al. 2021)), incidental ingestion of sediments has been proposed as a potential source of trace elements (Denton et al. 1980), although other studies have found negligible sediment in the stomachs of dugongs (Heinsohn and Birch 1972; Erftemeijer et al. 1993). Concentrations of cadmium in kidneys of the dugongs were comparable to manatees (ranging from not detectable to 38 mg kg−1) from Florida, USA (O’Shea et al. 1984).
Reptiles: marine turtles
Trace element data were sourced from four papers (Pople et al. 1998; van de Merwe, et al. 2010; Ikonomopoulou et al. 2011; Villa et al. 2017) and two reports (Gladstone 1996; Gaus et al. 2012). Over the period 1990–2014, a total of 264 individual animals were sampled across five species, with the green turtle (Chelonia mydas) accounting for 88% of samples. Blood was the most commonly investigated matrix (n = 207). Studies have typically investigated both essential elements (e.g. cobalt, copper, selenium and zinc) and non-essential elements (cadmium, mercury and lead). Data for the most reported elements are presented in Table 6, with additional elements and tissues in the Supplementary material. Like marine mammals, liver and kidney tissues typically contained the highest concentrations of both essential and non-essential elements, with mercury highest in liver tissues, and cadmium highest in kidney tissues. Green turtle liver mercury concentrations ranged from <0.01 to 1.6 mg kg−1 wet weight (Table 6) and were comparable to those found in dugongs (Table 5), which often share habitat and feeding areas. Kidney cadmium concentrations in the green turtle (Table 6) ranged from 1.7 to 101 mg kg−1 wet weight, which are similar to those found in seabirds (Hindell et al. 1999) and other marine mammals such as dugong, odontocetes and pinnipeds. Van de Merwe et al. (2010) identified strong correlations between green turtle blood and liver for mercury (r = 0.94), and kidney for both cadmium (r = 0.98) and arsenic (r = 0.98).
Common name | Species | Tissue | Non-essential | Essential | ||||
---|---|---|---|---|---|---|---|---|
Cd | Hg | Pb | Cu | Se | Zn | |||
Green turtle | Chelonia mydas | Liver | 2.5–56.9A,B,C,D (64) | <0.01–1.6A,B,C,D (45) | <0.05–1.10A,C,D (17) | 0.84–180A,C,D (26) | 0.07–10.4A,B,C,D (49) | 17–93A,B,C,D (56) |
Kidney | 1.7–101A,B,C,D (64) | <0.01–0.72A,B,C,D (46) | 0.047–0.15A,C,D (12) | 0.81–45A,C,D (26) | 0.09–5.1A,B,C,D (49) | 15.4–48A,B,C,D (56) | ||
Muscle | 0.01–7.8A,C (23) | < 0.01–0.05A,C (11) | 0.02–0.31A,C (23) | 0.14–30A,C (23) | 0.07–3.6A,C (23) | 6.0–39A,C (23) | ||
BloodG | 0.0011–0.122C,D,E (187) | 0.00025–0.038C,D (56) | 0.0002–0.18C,D,E (187) | 0.40–1.9C,D,E (187) | 0.028–9.1C,D,E (187) | 3.5–17C,D,E (187) | ||
Loggerhead turtle | Caretta caretta | Liver | 7.3–35.1B (8) | <0.001–0.032B (6) | – | – | 1.42–2.70B (6) | 13.7–32.6B (5) |
Kidney | 11.4–39.4B (5) | 0.033–0.067B (3) | – | – | 1.28–1.78B (3) | 16.7–21.3B (5) | ||
Hawksbill turtle | Eretmochelys imbricata | Liver | 2.4–6.2B (3) | 0.036–0.048B (2) | – | – | 2.68–3.65B (2) | 17.7–30.3B (3) |
Kidney | 3.6–12.7B (3) | 0.034–0.038B (2) | – | – | 2.22–2.49B (2) | 13.2–20.9B (3) | ||
Olive ridley turtle | Lepidochelys olivacea | Liver | 6.4B (1) | – | – | – | – | 14.8B (1) |
Kidney | 29.8B (1) | – | – | – | – | 18.8B (1) | ||
Flatback turtle | Natator depressus | BloodG | < 0.0001F (20) | < 0.0001F (20) | < 0.0001F (20) | 0.004–0.010F (20) | – | 0.098–0.21F (20) |
AGladstone (1996); BPople et al. (1998); Cvan de Merwe et al. (2010); DGaus et al. (2012); EVilla et al. (2017); FIkonomopoulou et al. (2011); Gmg L−1 wet weight.
Two studies have reported high concentrations of cobalt (maximum 840 μg L−1), arsenic (maximum 20 mg L−1) and selenium (maximum 9.1 mg L−1) in the blood of green turtles (van de Merwe et al. 2010; Gaus et al. 2012; Villa et al. 2017), while in another study on flatback turtles, blood cobalt concentrations were all below 0.1 μg L−1 (Ikonomopoulou et al. 2011). While the selenium concentrations of Australian green turtles may appear high (maximum liver Se concentration of 10 mg kg−1), they were similar to those observed in green turtles from Mexico (Labrada-Martagón et al. 2011). The maximum concentration of arsenic in blood of 20 mg L−1 (van de Merwe et al. 2010; Gaus et al. 2012) is one of the highest measured in green turtles globally (Cortés-Gómez et al. 2017), although the range in this study was very wide (0.09–20 mg L−1) and samples were taken from moribund animals rather than healthy individuals. Live green turtles sampled in San Diego Bay had much lower blood arsenic concentrations (0.16 ± 0.026 mg kg−1 wet weight) (Komoroske et al. 2011). In general, arsenic concentrations were highest in muscle tissue, a common trend in green turtles globally. International studies have identified the relatively non-toxic compound arsenobetaine to be the predominant form of arsenic in turtle muscle tissue, making up around 85% of total arsenic (Agusa et al. 2008). No studies on arsenic speciation in turtle blood were found. Concentrations of arsenic in seagrass samples from relatively pristine areas of Northern Australia have been measured at concentrations from 1.5 to 138 mg kg−1 dry weight (Thomas et al. 2020; Waterhouse et al. 2021), orders of magnitude higher than those reported internationally, providing a potential dietary source of arsenic.
Tissue trace element concentrations were reported for 706 individual bird samples with data for feathers, liver, blood, muscle and kidney. Little penguins (Eudyptula novaehollandiae) were the most sampled bird species, followed by other penguin species and members of the Laridae family (gulls, terns and noddies, fairy prion, albatross and short-tailed shearwater) (Tables 7 and 8). Mercury (n = 752), cadmium (n = 418) and lead (n = 530) were by far the most commonly reported metals, with the essential elements selenium, copper and zinc the next reported category (combined n = 781). Concentrations of the most reported elements are presented in Tables 7 and 8, with a complete data summary available in the Supplementary material.
Common name | Species | Tissue | Non-essential | Essential | |||||
---|---|---|---|---|---|---|---|---|---|
Cd | Hg | Pb | Cu | Se | Zn | ||||
Procellariiformes | |||||||||
Shy albatross | Thalassarche cauta | Liver | – | 13.4 ± 1.6A (29) | – | – | – | – | |
Kidney | 15.1 ± 1.4A (29) | – | – | – | – | – | |||
Royal albatross | Diomedea epomophora | Liver | – | 36.8 ± 12.1A (9) | – | – | – | – | |
Kidney | 48.1 ± 6.7A (9) | – | – | – | – | – | |||
Wandering albatross | Diomedea exulans | Liver | – | 164 ± 41A (22) | – | – | – | – | |
Kidney | 38.3 ± 3.5A (22) | – | – | – | – | – | |||
Fairy prion | Pachyptila turtur | Liver | 0.46–15C (28) | – | <.4–1.0C (28) | 4.5–12.5C (28) | – | 14–95C (28) | |
Muscle | <0.1–3.7C (28) | – | <0.4–1.0C (28) | 3.8–8.7C (28) | – | 11–44C (28) | |||
Short-tailed shearwater | Ardenna tenuirostris | Muscle | <0.01–0.05D,E (34) | <0.01–0.03D (14) | <0.01–0.49D (14) | 1.92–6.7D,E (34) | 0.55–1.0E (20) | 8.46–111D,E (34) | |
Flesh-footed shearwater | Ardenna carneipes | BloodJ | 0.554 ± 0.109I (12) | ||||||
Great-winged petrel | Pterodroma macroptera | BloodJ | 3.36 ± 0.18I (15) | ||||||
Accipitriformes | |||||||||
White-bellied sea eagle | Haliaeetus leucogaster | Liver | 0.05B (1) | 0.95B (1) | 0.22B (1) | 3.8B (1) | 1.5B (1) | 94B (1) | |
Muscle | <0.01–0.01B (2) | 0.35–0.45B (2) | 0.04–0.81B (2) | 0.79–5.5B (2) | 0.36–0.51B (2) | 27–40B (2) | |||
Sphenisciformes | |||||||||
Little penguin | Eudyptula minor | BloodJ | <0.02–0.15F (19) | 0.43–5.55F,G,H (186) | 0.02–0.20F,G,H (180) | 1.23–3.45G,H (163) | 3.45–70.5F,G,H (190) | 22.5–51.7G,H (165) |
Common name | Species | Non-essential | Essential | ||||
---|---|---|---|---|---|---|---|
Cd | Hg | Pb | Cu | Se | Zn | ||
Sphenisciformes | |||||||
Little penguin | Eudyptula minor | 0.01–0.13B (35) | 0.87–5.9B,H (45) | 0.03–0.80B (35) | 7.7–16B (35) | 1.4–5.6B (35) | 64–100B (35) |
Gentoo penguin | Pygoscelis papua | – | 0.09–0.23C (37) | – | – | – | – |
King penguin | Aptenodytes patagonicus | – | 0.03–0.15C (30) | – | – | – | – |
Eastern rockhopper penguin | Eudyptes chrysocome filholi | – | 0.03–0.21C (33) | – | – | – | – |
Royal penguin | Eudyptes schlegeli | – | 0.04–0.46C (42) | – | – | – | – |
Charadriiformes | |||||||
Brown noddy | Anous stolidus | 0.30 ± 0.10D (15) | 0.93 ± 0.25D (15) | 2.64 ± 1.32D (15) | – | – | – |
Black noddy | Anous minutus | 0.11 ± 0.03D (15) | 1.55 ± 0.29D (15) | 2.28 ± 0.36D (15) | – | – | – |
Silver gull | Larus novaehollandiae | 0.11–0.38A (60) | 0.82–0.96A (60) | 3.8–10.1A (60) | – | 0.51–0.62A | – |
Sooty tern | Onychoprion fuscatus | .26 ± 0.04D (15) | 0.45 ± 0.18D (15) | 1.29 ± 0.73D (15) | – | – | – |
Caspian tern | Hydroprogne caspia | <0.001E (39) | 2.27 ± 1.44E (39) | 0.074 ± 0.13E (39) | – | 0.82 ± 0.24E (39 | |
Procellariiformes | |||||||
Flesh-footed shearwaterJ | Ardenna carneipes | 0.34 ± 0.84F,I (57) | 5.51 ± 7.1F,I (88) | 0.40 ± 0.55F,I (88) | 14.3 ± 17.3F,I (88) | – | 71.1 ± 43.1F,I (88) |
Short-tailed shearwaterJ | Ardenna tenuirostris | 0.01–0.09G (10) | 0.05–0.28G (10) | 0.19–0.70G (10) | 11.2–23.9G (10) | – | 66.0–115G (10) |
Mercury concentrations in feathers ranged from 0.03 to 5.9 mg kg−1 dry weight, with highest concentrations reported in little penguins from coastal Victoria (Finger et al. 2015). Finger et al. (2015) reported significant positive correlations between feather and blood trace element concentrations for mercury (r = 0.68), lead (r = 0.70) and iron (r = 0.59). Lavers and Bond (2013) found poor correlations between feather and muscle element concentrations in short-tailed shearwaters.
A comparison of historical feather samples sourced from museums (1930–1976) with more recent feather samples (2002–2003) from four penguin species from Macquarie Island reported generally quite low Hg concentrations (<0.5 mg kg−1) and identified decreases in mercury concentrations over time for king and royal penguins, but no differences for gentoo and rockhopper penguins (Gilmour et al. 2019a) . The potential problems arising from use of museum feather samples is covered later in this review. Differences between species appeared to vary just as much as within species over time and may reflect changes in diet, habitat or anthropogenic sources of contamination (Gilmour et al. 2019a).
The internal organ distributions of trace elements in seabirds is suggested to be similar to that of marine mammals, where mercury has a propensity for accumulating in liver tissue, cadmium in kidney tissue, and low levels of other non-essential elements throughout (Thompson 1990). Arsenic and lead concentrations generally followed this trend, with most results lower than 1 mg kg−1, with the exception of some feather lead concentrations up to 10 mg kg−1 in silver gulls from Sydney, New South Wales (NSW), likely due to foraging in highly urbanised and industrialised environments (Burger and Gochfeld 1999). While no Australian seabird studies examined element concentrations across multiple internal organs, the highest reported mercury and cadmium concentrations were in the liver and kidney tissues, respectively, of the wandering albatross (Diomedea exulans, 610 mg Hg kg−1 wet weight; 81 mg Cd kg−1 wet weight) (Hindell et al. 1999). It is important to note that albatross (Brothers 1991) and shearwaters are migratory species (Lavers and Bond 2013) and their metal concentrations may not be representative solely of the Australian environment.
Behaviour of key contaminants
Mercury was the most reported inorganic contaminant, with data from all taxonomic groups of marine mammals, as well as from seabirds and turtles. Mercury concentrations in Australian marine fauna generally followed the commonly reported global trends of highest concentrations observed in liver tissues, increasing mercury concentrations with age and food chain biomagnification with higher concentrations in predators compared to herbivores (Tables 2, 3, 5 and Fig. 3) (Thompson 1990; Bowles 1999).
Reported ranges of mercury in liver tissue and cadmium in kidney tissue of Australian marine fauna (see Supplementary material for data sources).
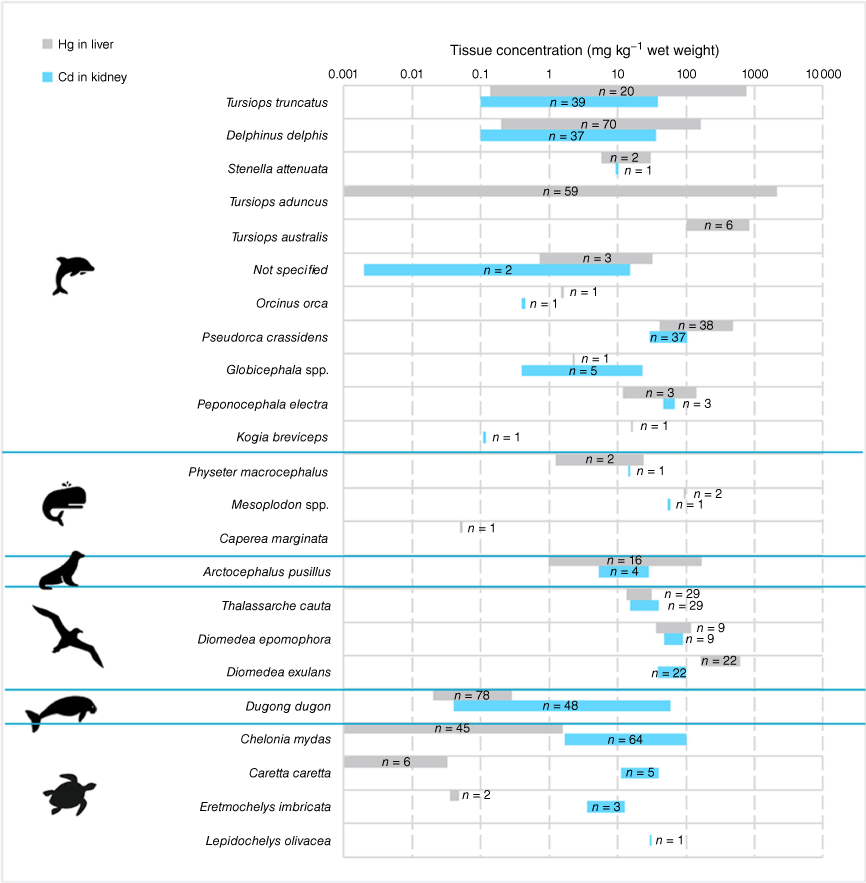
It is generally assumed that contaminant levels in Southern Hemisphere cetaceans are lower than their northern counterparts (Lavery et al. 2008), however, the mercury concentrations reported in the liver of Tursiops aduncus (maximum of 2875 mg kg−1 wet weight) from SA are some of the highest reported globally (Butterfield and Gaylard 2005). Tursiops truncatus and T. australis had similarly, albeit slightly lower mercury liver concentrations, which were approximately an order of magnitude higher than that of Delphinus delphis from the same location (Lavery et al. 2008; Monk et al. 2014), which has been attributed to differences in diets of the Tursiops spp. and Delphinus spp. (Lavery et al. 2008). While T. aduncus had the highest reported liver mercury concentrations, it also had the greatest range of reported concentrations (Fig. 3). The authors speculated that the high concentrations observed may result from a combination of naturally high background mercury concentrations, additional anthropogenic mercury inputs and the feeding strategies of T.aduncus which have a varied diet and generally feed on bottom- or near-bottom-dwelling cephalopods and fish (Butterfield and Gaylard 2005). They also noted that further research is needed to explain why T. aduncus hepatic mercury concentrations are elevated.
Lavery et al. (2008) identified significant differences in concentrations between stranding locations, with higher concentrations observed for samples collected nearshore, within industrially impacted gulfs as compared to those from open ocean. There were also significant differences (P < 0.001) in hepatic mercury content with age (as determined by tooth category), with older animals having higher concentrations than younger animals, which in part explains the large range of concentrations observed (Butterfield and Gaylard 2005). This commonly observed age-related trend in marine mammals (Thompson 1990) was also observed by Bacher (1985) in the Australian fur seal (A. pusillus, r = 0.975 P < 0.001). However, this trend is not well documented in seabirds (Thompson 1990). Nevertheless, hepatic mercury concentrations reported in adult Australian wandering albatross (Diomedea exulans) have been shown to be significantly greater than in juveniles (Hindell et al. 1999).
It is clear that hepatic mercury concentrations in Australian marine fauna are highly diet dependent, with species feeding on higher trophic level organisms exhibiting higher concentrations. The herbivorous dugong (D. dugon), the predominantly herbivorous green turtle (C. mydas) and the mysticete pygmy right whale (C. marginate), all low trophic level feeders, had some of the lowest reported liver mercury concentrations in Australian marine fauna (Fig. 3). The importance of diet on mercury accumulation has also been highlighted in the international literature by the marked increase in liver mercury concentration of South American fur seal (Arctocephalus australis) pups after weaning, where concentrations increased approximately eight-fold compared to suckling pups (Gerpe et al. 2009). Australian fur seals species (Arctocephalus spp.) diet similarly changes with geographical location, and environmental and breeding seasons (Fea et al. 1999; Hume et al. 2004; Littnan et al. 2007). Higher hepatic mercury concentrations in T. aduncus compared to T. truncatus and D. delphis have also been attributed to different feeding grounds, and therefore target prey, with the former preferring the potentially more contaminated nearshore shallow gulfs and bays, and the latter preferring offshore pelagic habitats (Lavery et al. 2008). The importance of diet was also indicated by the analysis of seabird blood, where great-winged petrels (3.36 ± 0.18 mg kg−1 wet weight) from WA, which feed primarily on squid, had significantly higher (P < 0.05) concentrations of mercury in blood than the flesh-footed shearwater (0.554 ± 0.109 mg kg−1 wet weight) feeding on lower trophic level schooling fish (Gilmour et al. 2019b).
Internal organ partitioning of mercury within organisms followed the general trend of liver > kidney > muscle (Tables 2, 3 and 5). The limited number of Australian studies that sampled multiple tissue types from individuals identified liver/kidney concentration factors ranging from 1.5 to 16 for marine mammals (Bacher 1985; Kemper et al. 1994; Gladstone 1996; Law et al. 2003) and 0.3–3 for turtles (Gladstone 1996; Pople et al. 1998; van de Merwe et al. 2010). There is no paired organ data available for birds. Examination of internal organ mercury load alone can be problematic, however, as it relies upon strandings or bycatch of animals, which results in small sample sizes. For context, Fig. 3 displays hepatic mercury concentrations from 207 cetaceans sampled between 1989 and 2004, while Canella and Kitchener (1992) were able to utilise a sperm whale muscle tissue dataset obtained from 414 samples collected over a whale harvest period of only 5 months in 1976. The small size of the subsequent data pool makes assessment of potential toxicological effects of the mercury load difficult. It also makes it problematic to assess the potential role that metal load may have played in the stranding behaviour of the animals, with no studies having investigated potential differences in metal loads of stranded versus by-caught animals.
There was only one Australian study that measured brain mercury concentrations in marine fauna. Bacher (1985) reported brain mercury concentrations in Australian fur seals (A. pusillus) ranging from 0.04 to 2.53 mg kg−1 wet weight (mean 0.70 mg kg−1).
To overcome sampling issues, many recent studies have investigated the use of matrices such as skin, fur, feathers or blood as minimally invasive indicators of metal contamination in live animals, with several studies identifying positive correlations between these indicator tissues and internal organ loads. Bacher (1985) found a correlation between liver and hair mercury concentrations in Australian fur seals (r = 0.69, P < 0.005), while Aubail et al. (2013) identified liver–skin correlations in four dolphin species, including T. truncatus (r = 0.81, P < 0.001) and D. delphis (r = 0.70, P < 0.001) specimens from the North Atlantic Ocean. While feather mercury concentrations in birds are less indicative of internal organ load (Eagles-Smith et al. 2008; Lavers and Bond 2013), they are thought to provide a snapshot of organism metal load at the time of growth (Gochfeld 1980). Mercury is known to accumulate in the feathers of birds in the form of methylmercury (Rainbow 2018), with partitioning of mercury into feathers and subsequent moult forming an effective detoxification mechanism for some species (Braune and Gaskin 1987). This has been particularly useful for assessment of metal loads in penguins, as they moult all feathers annually in a single event, allowing for a good measure of annual mercury accumulation (Carravieri et al. 2013). Partitioning of metals into feathers has also been postulated as an effective method for birds to remove metals from their body, with plumage concentrations of some elements such as mercury accounting for up to 93% of body burden, despite only accounting for 12% of body weight (Braune and Gaskin 1987).
The use of feathers has allowed for much larger sample sizes to be utilised, across larger spatial and temporal ranges (Finger et al. 2016). One such study over large temporal scale by Gilmour et al. (2019a), utilising a museum collection of ‘historic’ penguin feathers as well as ‘modern’ feathers both collected from four species of penguins from Macquarie Island, showed generally quite low concentrations of mercury (means < 0.5 mg kg−1 dry weight). Concentrations for two of the species were lower (P < 0.05) in the ‘modern’ samples compared with the ‘historic’ samples, with no significant differences in the other two species. Due to the opportunistic sampling of the modern feathers from carcases, along with the unknown moult stage of the historic birds, it is difficult to make judgements about the temporal trends observed. It is important to note that total mercury was measured on these feathers, which is not recommended, particularly in museum feathers, due to potential historic sample contamination from inorganic mercury containing preservatives. For these reasons, methylmercury determination is recommended for feather samples (Bond et al. 2015; Rainbow 2018).
More can be drawn from differences between the modern feather samples if all birds are sampled at similar stages of moulting. In such an instance, gentoo and rockhopper penguins had 3.5–7 times higher mercury concentrations than the king and royal penguins (Gilmour et al. 2019a). It has previously been suggested that the rockhopper penguins feed closer to shore than royal penguins (Horne 1985), with the different prey in each location potentially explaining the differences observed, which again highlights the importance of diet in mercury accumulation.
While no Australian studies were identified that examined mercury speciation in marine mammals, based on international literature, it is widely accepted that most marine fauna are exposed to mercury in the form of methylmercury (Lynch et al. 2012; Rainbow 2018), which is much more readily bioaccumulated and biomagnified than inorganic mercury. Within marine mammals, methylmercury is typically the predominant form of mercury in muscle and skin tissue, comprising 89–97% of total mercury in some arctic whales and seals (Wagemann et al. 1998). Methylmercury was also found to be the dominant form of mercury in the blood of bottlenose dolphins from the southeast coast of the USA (57–96% of total mercury) (Stavros et al. 2008; Woshner et al. 2008). However, in liver tissue where total mercury concentrations are typically highest, the contribution of methylmercury to total mercury was far lower ranging from 3 to 12% in arctic mammals (Wagemann et al. 1998). It has been postulated that marine mammals have developed detoxification processes to demethylate organo-mercury and store the mercury as inert mercuric selenide species (Cuvin-Aralar and Furness 1991; Wagemann et al. 1998). The formation of mercuric selenide (HgSe, also known as tiemannite) was observed predominantly in liver tissue of striped dolphin (S. coeruleoalba) from Japan, but also to a lesser extent in kidney, lung, spleen, pancreas, muscle and brain, which highlights the important role selenium plays in the detoxification of mercury throughout the body (Nakazawa et al. 2011). This detoxification product has also recently been discovered in humans (Moser et al. 2020).
Selenium was often measured in studies examining mercury with molar ratios of the two elements close to one, leading to the conclusion that mercury was present as HgSe (Savery et al. 2013; Monk et al. 2014). Potential protective effects offered by selenium in regard to mercury toxicity have received considerable attention in the international literature. It has been suggested that Hg:Se molar ratios <1 (i.e selenium is present in excess over mercury) are protective against adverse effects of mercury (Ganther et al. 1972; Koeman et al. 1973; El Begearmi et al. 1977; Ralston et al. 2008; Peterson et al. 2009). Laboratory studies involving rats fed a high methylmercury diet in conjunction with high selenium, showed no neurotoxic effects, while rats fed low selenium diets did (Friedman et al. 1978). Observational studies of ringed seals with hepatic concentrations of up to 510 mg kg−1 mercury and 270 mg kg−1 selenium showed no obvious signs of toxicity from either element, suggesting a potentially mutually protective effect (Wagemann and Muir 1984; Cuvin-Aralar and Furness 1991).
While the idea of organ threshold concentrations, above which toxicological effects are observed, is appealing, this is likely not realistic as marine mammals appear to have evolved with detoxification mechanisms to deal with many inorganic contaminants. Rather, it has been suggested that the rate of mercury uptake (and subsequent rate of detoxification) is a more critical factor in determining toxicity than a threshold value (Rainbow 2018). It would therefore seem pertinent to assess the dietary uptake rates of marine mammals when assessing the potential acute or chronic toxicological impacts of mercury.
Methylmercury is the predominant form of mercury in seabirds, comprising over 90% of total mercury in liver, kidney, muscle and feathers (Burger and Gochfeld 2004). As noted previously, a detoxification strategy used by many bird species involves the channelling of methylmercury into feathers, which are then offloaded during moulting (Rainbow 2018). Methylmercury binds strongly with the disulfides in keratin, making the mercury bound within the feather stable over long time periods. It is for this reason, along with the fact that some museum feather samples were preserved using HgCl2, that methylmercury measurement is the favoured technique for museum feather samples (Bond et al. 2015), although all Australian studies measured total mercury in feathers. There is evidence that some seabirds may convert methylmercury into inorganic mercury, with the methylmercury content of some seabirds only accounting for ~6% of total mercury (Lock et al. 1992). It is thought that birds with slow moult cycles have less opportunity to offload ingested mercury through moulting, so have therefore developed demethylation processes as an alternative detoxification strategy (Lock et al. 1992).
Data on mercury speciation in marine turtles are much more limited than other marine fauna, likely owing to the generally low concentrations of mercury present. However, it has been found that methylmercury can account for 4–23% of total mercury in green turtles (C. mydas) and 10–65% in loggerhead turtles (C. caretta) (Kampalath et al. 2006). Interestingly, it was also noted that methylmercury concentrations were generally highest in liver tissues, although as a percentage of total mercury, liver tissue was lower than muscle or kidney, suggesting demethylation is occurring within the liver (Kampalath et al. 2006).
The influence of diet on cadmium concentrations in marine fauna has been reported in many studies, with cephalopods such as octopus, cuttlefish and squid and demersal fish being identified as dietary sources high in cadmium for cetaceans (Thompson 1990; Long et al. 1998; Lischka et al. 2021) and seabirds (Burger and Gochfeld 2001). An up to three times higher cadmium kidney concentration in wandering albatross compared to shy albatross has also been attributed to differences in diet (Hindell et al. 1999).
It is known that marine mammals and seabirds accumulate cadmium in the kidney, where it is usually associated with metallothioneins, and tissue concentrations tend to decrease in the order: kidney > liver > muscle (McIntyre and Kinne 1979; Thompson 1990; Kemper et al. 1994; Long et al. 1998; Law et al. 2003) (Tables 2, 3 and 5). Long et al. (1998) focused on cadmium in T. truncatus (n = 18) and D. delphis (n = 20) specimens, with cadmium concentrations in kidney three-fold higher than in the liver, and 26-fold higher than in the muscle. Similar cadmium kidney to liver ratios for T. truncatus and D. delphis were reported by Kemper et al. (1994). Kemper et al. (1994) also determined that cadmium accumulation between the species was not significantly different and cadmium concentrations were weakly, positively related to dolphin body length (r value not given).
A comparison of cadmium kidney tissue data within and between Australian species is hindered by limited sample numbers. For example, there is only one set of cadmium in kidney data for Australian pinnipeds (A. pusillus), with the dataset complicated by age-related accumulation effects. Despite this limitation, renal cadmium concentrations decreased in the following order: cetaceans (<0.002–106 mg kg−1 wet weight) > marine turtles (1.7–101 mg kg−1 wet weight) > dugong (0.04–59 mg kg−1 wet weight) > seals (5.3–28.7 mg kg−1 wet weight) (Fig. 3). The false killer whale (P. crassidens) had the highest renal cadmium concentration of the marine mammals, ranging from 29.7 to 106 mg kg−1 wet weight (n = 37) (Kemper et al. 1994). False killer whales and many other large Delphinidae such as pilot whales (G. melas) feed primarily on fish and cephalopods (Baird 2009; Lischka et al. 2021). High renal cadmium concentrations of 97–614 mg kg−1 dry weight, have been reported in pilot whales stranded in New Zealand (Lischka et al. 2021). Globally, the highest levels of cadmium reported in other marine vertebrates have been found in kidney tissue of Ross seals (Ommatophoca rossii, 422 mg g−1 dry weight), narwhals (Monodon monoceros, 205 mg kg−1 wet weight) and macaroni penguins (Eudyptes chrysolophus, 166 mg g−1 wet weight) (Thompson 1990). Dugong (D. dugon) showed the same pattern of cadmium distribution as cetaceans, with the kidney containing the highest concentrations ranging from 0.04 to 59 mg kg−1 wet weight (n = 32) (Denton et al. 1980; Marsh 1989; Gladstone 1996).
Four Australian studies show interesting age related renal cadmium accumulation effects in different species. Denton et al. (1980) demonstrated a positive correlation between cadmium concentration and age in kidney and liver tissue in dugongs, while Long et al. (1998) found there were no demonstrable differences in renal cadmium concentrations between juveniles and subadult/adult T. truncatus and D. delphis. Hindell et al. (1999) found that renal cadmium for two albatross species, D. exulans and D. epomophora, did not increase with age, suggesting some degree of cadmium regulation takes place in these birds, an observation which has been reported in other studies of seabirds (Thompson 1990).
A study of Australian green turtles (C. mydas, n = 38) in Moreton Bay, Southeast Queensland (Pople et al. 1998) found a significant decrease in renal cadmium concentration with increasing age of turtles. It has been proposed that this observed decrease could be due to the change of diet that green turtles undergo, from carnivorous young to herbivorous as they age (Fraga et al. 2018). The mean renal cadmium concentrations ranged from 1.7 to 75.9 mg kg−1 wet weight and were amongst the highest concentrations recorded globally (Pople et al. 1998). It has been suggested that these somewhat elevated cadmium concentrations in the low trophic level C. mydas are a naturally occurring phenomenon (Gladstone 1996; Pople et al. 1998).
In fur seals, Arctocephalus spp., cadmium in kidney ranged from 5.3 to 28.7 mg kg−1 wet weight (n = 4), while in liver it ranged from 0.76 to 39.2 mg kg−1 wet weight (n = 6). These values are unpublished data taken from the Kemper et al. (1994) review, with no data on cadmium in Australian pinnipeds being published since. These values are consistent with those reported in international studies (Julshamn and Grahl-Nielsen 2000; Riget et al. 2005; Brunborg et al. 2006; Gerpe et al. 2009). While no trends could be observed in the Australian pinniped data (owing to low sample numbers), cadmium in kidney tissue has been shown to increase with age in the South American fur seal (A. australis), with weaned pups (20.9 ± 5.0 mg kg−1 wet weight) having much higher renal cadmium concentrations compared to suckling pups (0.06 ± 0.04 mg kg−1 wet weight) (Gerpe et al. 2009). This clearly highlights the impact diet has on cadmium concentrations.
There is little information in the Australian literature regarding the use of non-, or minimally invasive indicator matrices such as feathers (Lavers and Bond 2013) or blood (van de Merwe et al. 2010) to assess cadmium internal organ load. Lavers and Bond (2013) found no correlation between cadmium in feathers and muscle tissue of short-tailed shearwaters. To date, no Australian studies have investigated the use of indicator matrices for cadmium in cetaceans, pinnipeds or dugong. Numerous international studies on cetacean species have found no correlation between skin and internal organ cadmium concentrations (e.g. Aubail et al. 2013; Sun et al. 2017), suggesting that unlike mercury, indicator matrices are not useful at predicting internal cadmium organ load for these fauna.
While indicator matrices may not provide adequate detail on internal organ cadmium loads, they may be useful in assessing differences between populations of species. Feathers and blood have been used to compare recent metal exposures in seabirds in numerous studies (Burger and Gochfeld 1995; Finger et al. 2015). Cadmium in green turtle blood was analysed in four studies (van de Merwe et al. 2010; Ikonomopoulou et al. 2011; Gaus et al. 2012; Villa et al. 2017), however, significant correlations (R2 = 0.96) with kidney cadmium concentrations were demonstrated in only one of these studies (van de Merwe et al. 2010). This is consistent with an international feeding study of the freshwater red-eared slider turtle (Trachemys scripta elegans) (Guirlet and Das 2012), which demonstrated that cadmium blood levels remained low due to a high binding affinity for metallothionein in the liver, even when exposed to a highly contaminated diet, making blood cadmium concentration a poor biomonitor of elevated cadmium exposure (Villa et al. 2019).
There is limited information in the literature regarding the health consequences of elevated cadmium concentrations in Australian marine fauna. Much is known of the toxic effects of cadmium in humans, with toxicity typically presenting as kidney dysfunction, or weakening of the bones due to cadmium replacing calcium (Friberg et al. 1992). A threshold or critical cadmium concentration of 200 mg kg−1 wet weight is often associated with kidney damage in terrestrial mammals (Das et al. 2000). Renal cadmium concentrations in marine mammals have been observed at levels much greater than those observed in terrestrial mammals, with up to 580 mg kg−1 wet weight in Arctic ringed seals showing no pathological effects (Dietz et al. 1998). Marine mammals may be able to tolerate high cadmium concentrations, primarily due to binding to metallothionein (Das et al. 2000). The presence of higher concentrations of metallothionein in marine mammals compared to terrestrial mammals, and therefore a greater ability to detoxify contaminants such as cadmium, has been postulated to be as a consequence of greater exposure to metallothionein-inducing metals such as cadmium, copper, mercury and zinc (Teigen et al. 1999). Lischka et al. (2021) highlighted this capacity by comparison of the cadmium intake by pilot whales to human exposure limits, noting that if a human consumed the body weight equivalent mass of cadmium, they would exceed daily maximum intake levels by almost 1500%.
Three species reported in this review are routinely harvested by indigenous people for food: the short-tailed shearwater (A. tenuirostris), dugong (D. dugon) and green turtle (C. mydas) (Table 9). While no maximum permissible concentrations of cadmium in marine fauna have been set, The Australian Food Standard for metal contaminants lists maximum concentrations in muscle, liver and kidney tissues from cattle, sheep and pigs at 0.05, 1.25 and 2.5 mg kg−1, respectively (Commonwealth of Australia 2016).
Cadmium source | Liver | Kidney | Muscle |
---|---|---|---|
Dugong | < 0.001–15A,B,C,D,E (125) | 0.04–59B,C,E (32) | 0.01–0.05A,B,C,E (30) |
Green turtle | 2.5–56.9C,F,G,H (64) | 1.7–101C,F,G,H (64) | 0.01–7.8C,G (23) |
Short-tailed shearwater | – | – | < 0.01–0.05I,J (34) |
Australian Food Standard | 1.25 | 2.5 | 0.05 |
All of the green turtles from the studies of Pople et al. (1998) and van de Merwe et al. (2010) exceed the Australian Food Standards for cattle, sheep and pigs. Muscle cadmium concentrations in the short-tailed shearwater reported by Lavers and Bond (2013) were all low (<0.04 mg kg−1 wet weight), although Puskic et al. (2020) reported one out of 20 samples at the Australian Food Standard guideline of 0.05 mg kg−1 (data reported as dry weight and converted into wet weight). It is difficult to make general statements regarding concentrations in dugongs, as many studies (Denton et al. 1980; Kemper et al. 1994; Haynes et al. 2005) only supplied concentrations ranges, although maximum concentrations were above the Australian Food Standard guidelines. However, the liver or kidney concentrations in all three dugongs reported by Gladstone (1996) and two dugongs reported by Marsh (1989) exceeded the Australian Food Standard guidelines. Gladstone (1996) identified that consumption of small quantities of dugong liver or kidney, or green turtle liver, kidney, intestine or muscle by Torres Strait Islanders would put them above the provisional tolerable weekly intake guidelines. Long term consumption rates, as well as cadmium speciation, would need to be taken into consideration before conclusions about public health impacts could be made (Pople et al. 1998).
Only a few studies have examined the concentration of lead in marine fauna (Tables 2, 3, 5, 6 and 7). Lead concentrations are generally very low in soft tissues and the element is effectively detoxified through its association with phosphate and deposition in bones (Rainbow 2018).
The most frequently analysed tissue was liver followed by muscle, blubber, kidney, bone and skin. The concentration of lead in blubber and muscle of marine mammals was generally low (< 0.3 mg kg−1 wet weight), except for one T. truncatus specimen from NSW which had a blubber lead value of 3.41 mg kg−1 wet weight (Kemper et al. 1994) and one specimen of Burrunan dolphin from Victoria with 12 mg kg−1 wet weight (Monk et al. 2014). For lead in muscle, the highest values were found in fairy prions (P. turtur) from Tasmanian waters (< 0.4–1.0 mg kg−1 wet weight) (Brothers and Brown 1987).
The highest concentrations of lead were found in the livers of specimens of T. aduncus, from the Spencer Gulf, SA (0.004–15.2 mg kg−1 wet weight) (Butterfield and Gaylard 2005; Lavery et al. 2008). In comparison, lead concentrations in the liver of the common dolphin (D. delphis) from UK and New Zealand waters ranged from < 0.01 to 1.0 mg kg−1 (Law et al. 1991; Stockin et al. 2007), whereas striped dolphin (S. coeruleoalba) from Japanese waters had hepatic lead concentrations ranging from 0.03 to 0.64 mg kg−1 wet weight (n = 30) (Honda et al. 1983). Within the cohort of SA dolphins analysed by Lavery et al. (2008), T. aduncus had greater concentrations of lead in liver and bone than T. truncatus or D. delphis. It was suggested that the higher concentrations in T. truncatus may be due to their coastal habitat having high contaminant loads, and also differences in their diet compared to the other dolphin species. Four studies presented data on lead in dugong, with all concentrations in the liver and kidney below 1 mg kg−1 wet weight (Denton et al. 1980; Kemper et al. 1994; Gladstone 1996; Haynes et al. 2005).
Lead was analysed in the bone of dolphins from SA, due to concerns around lead emissions from the smelter located at Port Pirie. The highest lead value of 61.6 mg kg−1 was recorded in the bone of a young T. truncatus (Kemper et al. 1994). Kemper et al. (1994) suggested that this high lead concentration might be due to the type of bone that was analysed, which included vertebral epiphyses. Since lead is concentrated in the epiphyseal regions during the early part of bone deposition (i.e. growth), it is possible that the very high level of lead in the animal was a symptom of an earlier phase of exposure to lead contamination. In a more recent study, Lavery et al. (2008) found lead concentrations in bone from Tursiops sp. from the sample location ranged from 0.29 to 16.0 mg kg−1 wet weight and for D. delphis ranged from 0.42 to 2.4 mg kg−1 wet weight.
Less data were available for other trace element contaminants in Australian marine fauna. While all Australian marine mammals had arsenic concentrations <1 mg kg−1 wet weight, comparatively high arsenic concentrations were reported in green turtle (C. mydas) muscle (up to 14 mg kg−1) and blood (up to 20 mg L−1) (van de Merwe et al. 2010; Gaus et al. 2012). International work has identified the relatively non-toxic compound arsenobetaine to be the predominant form of arsenic in green turtle muscle tissue, making up around 85% of total arsenic (Agusa et al. 2008). Arsenobetaine has been identified as the major arsenic species in tissues of marine mammals (Fujihara et al. 2003), including the plasma of pinnipeds (Kuenstl et al. 2009). While green turtle blood concentrations up to 20 mg As L−1 have been reported, samples were taken from either moribund or dead animals (van de Merwe et al. 2010), or those from areas characterised by turtles in poor health and associated elevated incidence of mortality (Gaus et al. 2012). Otherwise, healthy turtles sampled from industrialised coastal environments in Queensland had maximum blood arsenic concentrations approximately an order of magnitude lower (maximum of 2.8 mg L−1) (Villa et al. 2017).
Data on essential trace elements in marine fauna were also limited. Cobalt was measured in very few marine mammal samples, with the highest reported concentration being 19 mg kg−1 wet weight in the liver of dugong from North QLD (Denton et al. 1980). Villa et al. (2017) identified high concentrations of cobalt in green turtle (C. mydas) blood of up to 840 μg L−1 at sites in the Great Barrier Reef regions of Queensland. There were significant differences in blood cobalt concentration between individuals from the baseline site away from anthropogenic point sources, and coastal populations closer to identified point sources of contaminants. A separate study of seagrass from similar locations revealed a similar trend with seagrass cobalt concentrations an order of magnitude greater at the anthropogenically impacted coastal sites compared to the baseline site (Thomas et al. 2020).
Copper and zinc were occasionally measured across studies. In cetaceans there was a relatively small variation between individuals of the same species due to homeostatic control of these essential elements (Weijs et al. 2016; Rainbow 2018); a trend also observed in fairy prions (P. turtur) (Brothers and Brown 1987). A comprehensive review by Rainbow (2018) highlighted that marine mammal livers and kidneys tend to contain 3–30 mg kg−1 wet weight copper in a metabolically available form to meet the essential needs of the animal, with little evidence of detoxified storage. Relatively high hepatic copper concentrations have been found in dugongs ranging from 1.8 to 472 mg kg−1 wet weight (Table 5), however, the measured concentrations were generally lower than those found in manatees from Florida, USA (O’Shea et al. 1984). In pinnipeds, zinc data was limited to a single study of Australian fur seal fur (Lynch et al. 2012).
Conclusions
The Australian data for contaminants in marine fauna are characterised by large data gaps in terms of geographical, temporal and species coverage. This is the result of opportunistic sampling relying upon animal strandings or bycatch. For instance, out of 14 endemic species of dolphins in Australia, contaminants data only exist for seven species sampled. There is surprisingly little data for NSW, particularly for marine fauna occupying Sydney Harbour or the surrounding coastal areas of Sydney, a major urban and industrial centre.
The aforementioned data gaps make it hard to identify statistically significant trends in the data, a problem compounded by the way in which data have been presented, i.e. as a range with no raw data available. This has prevented any long-term, inter-study analysis being undertaken.
Overall, QC has steadily improved over the last 40 years, however, even in the majority of publications produced since 2010, a complete inventory of QC information is missing with only 28% of the studies conducted providing comprehensive QC data.
Contaminant concentrations measured in various marine species and their tissue types are extremely variable with concentration ranges typically spanning several orders of magnitude. However, trends with contaminant concentrations and tissue type follow globally accepted patterns of behaviour with regards to tissue type, diet and age.
Based on the data that are available in the literature, there is currently little evidence to suggest that trace metal bioaccumulation at the levels observed in Australian fauna is impacting on the health of marine animals. This can be attributed to well evolved detoxification mechanisms that excrete or sequester metals in non-toxic forms. Some concerns have been raised about the sub-lethal effects of high metal loadings, such as immunosuppression, in some marine mammals.
There have been some attempts to use minimally invasive sampling of indicator tissues such as fur, bristle and feathers. However, it is currently difficult to extrapolate this data to contaminant concentrations in major organs. Bird feathers provide an opportunity for temporal trend analysis over many decades as they are often stored in archive collections in museums; however, storage conditions may impact analysis and interpretation of results.
Recommendations
Publications should include details of analytical methodology and analytical quality control (QC) information. This should include a description of the sampling and analytical methodologies employed, calibration, blanks (to show the results are not affected by contamination), replicate measurements, spike recoveries and certified reference material analysis data (analysed in the same batch as the samples). While a range of analytical techniques may yield acceptable results if correctly implemented with the recommended QC measures, contemporary analytical methods focus on the use of microwave assisted digestion procedures followed by sensitive multi-elemental analysis by ICPMS and/or cold vapour AAS or atomic fluorescence spectrometry (AFS) for mercury analysis. Detailed recommendations on sampling and storage of marine biota samples can be found in the publication by the Puget Sound Water Quality Authority (1994).
In order to allow meta-analysis of data collected across studies, it is important that researchers provide sufficient detail of their data sets. This should include summary statistics such as mean, median and range, and ideally the raw data should be accessible. It is also critical that conversion factors, such as the percentage dry weight of tissues (i.e. moisture content), are reported to allow re-processing of the data where needed.
Although the sampling of organisms is likely to proceed on a largely opportunistic basis, it would be of great value to strategically target the data gaps that have been identified in this review. This includes gaps in geographical coverage and specific marine mammals such as seals and baleen whales. More data for specimens sourced from urban and isolated locations would provide a better understanding of contaminant sources.
The soft tissues recommended for sampling and analysis of metals are liver and kidney. In addition, brain and muscle tissue samples are useful in understanding mercury bioaccumulation and its potential impacts. In studies concerning mercury bioaccumulation, selenium concentrations in tissues should also be measured as they provide a powerful insight into the speciation and potential toxicity of mercury.
Coordinated efforts to catalogue, collect, preserve and archive samples from stranded animals on a national scale would assist in addressing the shortage of samples for certain species. Elsewhere in the world, nationally coordinated specimen banking programs have been put in place (e.g. USA National Marine Mammal Tissue Bank) (NOAA 2022) to address this issue.
Minimally invasive sampling of indicator tissues such as skin, fur, bristle, feathers and blood are attractive alternatives to sampling of dead organisms. However, further studies are required to understand the associations between trace element concentrations in indicator tissues and key organs such as liver and kidney. A meta-analysis of existing global data on this topic would be useful. Notwithstanding sample storage and preservation issues, bird feathers also provide an opportunity for temporal trend analysis over many decades as they are often stored in archive collections in museums.
As originally recommended by Kemper et al. (1994), a comprehensive pathological assessment of specimens is advisable. This enables other reasons for organism stress or disease to be identified and accounted for when assessing the impacts of contaminants on organism health. This information would reduce the tendency for speculative conclusions on the causes of poor organism health based on contaminants data alone.
References
Agusa T, Takagi K, Kubota R, Anan Y, Iwata H, Tanabe S (2008) Specific accumulation of arsenic compounds in green turtles (Chelonia mydas) and hawksbill turtles (Eretmochelys imbricata) from Ishigaki Island, Japan. Environmental Pollution 153, 127-136.
| Crossref | Google Scholar |
Aubail A, Méndez-Fernandez P, Bustamante P, Churlaud C, Ferreira M, Vingada JV, Caurant F (2013) Use of skin and blubber tissues of small cetaceans to assess the trace element content of internal organs. Marine Pollution Bulletin 76, 158-169.
| Crossref | Google Scholar |
Bacher GJ (1985) Mercury concentrations in the australian fur seal Arctocephalus pusillus from SE Australian Waters. Bulletin of Environmental Contamination and Toxicology 35, 490-495.
| Crossref | Google Scholar |
Baird RW (2009) False killer whale. In ‘Encyclopedia of Marine Mammals’, 2nd edn. (Eds WF Perrin, B Wursig, JGM Thewissen) pp. 405–406. (Academic Press: Burlington, MA, USA) 10.1016/B978-0-12-373553-9.00097-3
Becker PR, Mackey EA, Demiralp R, Schantz MM, Koster BJ, Wise SA (1997) Concentrations of chlorinated hydrocarbons and trace elements in marine mammal tissues archived in the U.S. National Biomonitoring Specimen Bank. Chemosphere 34, 2067-2098.
| Crossref | Google Scholar |
Bond AL, Lavers JL (2011) Trace element concentrations in feathers of flesh-footed shearwaters (Puffinus carneipes) from across their breeding range. Archives of Environmental Contamination and Toxicology 61, 318-326.
| Crossref | Google Scholar |
Bond AL, Hobson KA, Branfireun BA (2015) Rapidly increasing methyl mercury in endangered ivory gull (Pagophila eburnea) feathers over a 130 year record. Proceedings of the Royal Society B: Biological Sciences 282, 20150032.
| Crossref | Google Scholar |
Bowles D (1999) An overview of the concentrations and effects of metals in cetacean species. Journal of Cetacean Research and Management 1, 125-148.
| Crossref | Google Scholar |
Brasso RL, Drummond BE, Borrett SR, Chiaradia A, Polito MJ, Rey AR (2013) Unique pattern of molt leads to low intraindividual variation in feather mercury concentrations in penguins. Environmental Toxicology and Chemistry 32(10), 2331-2334.
| Crossref | Google Scholar |
Braune BM, Gaskin DE (1987) Mercury levels in Bonaparte’s gulls (Larus Philadelphia) during autumn molt in the Quoddy region, New Brunswick, Canada. Archives of Environmental Contamination and Toxicology 16, 539-549.
| Crossref | Google Scholar |
Brothers N (1991) Albatross mortality and associated bait loss in the Japanese longline fishery in the Southern Ocean. Biological Conservation 55, 255-268.
| Crossref | Google Scholar |
Brothers NP, Brown MJ (1987) The potential use of fairy prions (Pachyptila turtur) as monitors of heavy metal levels in Tasmanian waters. Marine Pollution Bulletin 18, 132-134.
| Crossref | Google Scholar |
Brunborg LA, Graff IE, Frøyland L, Julshamn K (2006) Levels of non-essential elements in muscle from harp seal (Phagophilus groenlandicus) and hooded seal (Cystophora cristata) caught in the Greenland Sea area. Science of the Total Environment 366, 784-798.
| Crossref | Google Scholar |
Burger J, Gochfeld M (1991) Lead, mercury, and cadmium in feathers of tropical terns in Puerto Rico and Australia. Archives of Environmental Contamination and Toxicology 21, 311-315.
| Crossref | Google Scholar |
Burger J, Gochfeld M (1995) Biomonitoring of heavy metals in the Pacific basin using avian feathers. Environmental Toxicology and Chemistry 14, 1233-1239.
| Crossref | Google Scholar |
Burger J, Gochfeld M (1999) Interspecific and locational differences in heavy metal levels in four species of birds near Sydney, Australia. Environmental Monitoring and Assessment 58, 105-119.
| Crossref | Google Scholar |
Burger J, Gochfeld M (2001) Effects of chemicals and pollution on seabirds. Chapter 15. In ‘Biology of Marine Birds’. (Eds EA Schreiber, J Burger) pp. 485–524 (CRC Press: Boca Raton, FL, USA) 10.1201/9781420036305
Burger J, Gochfeld M (2004) Marine birds as sentinels of environmental pollution. EcoHealth 1, 263-274.
| Crossref | Google Scholar |
Butterfield N, Gaylard S (2005) The heavy metal status of South Australian Dolphins. South Australian EPA report. Available at www.epa.sa.gov.au/files/8556_heavy_dolphins.pdf
Cagnazzi D, Broadhurst MK, Reichelt-Brushett A (2019) Metal contamination among endangered, threatened and protected marine vertebrates off south-eastern Australia. Ecological Indicators 107, 105658.
| Crossref | Google Scholar |
Cagnazzi D, Harrison PL, Parra GJ, Reichelt-Brushett A, Marsili L (2020) Geographic and temporal variation in persistent pollutants in Australian humpback and snubfin dolphins. Ecological Indicators 111, 105990.
| Crossref | Google Scholar |
Canella EG, Kitchener DJ (1992) Differences in mercury levels in female sperm whale. Australian Mammalogy 15, 121-123.
| Crossref | Google Scholar |
Carravieri A, Bustamante P, Churlaud C, Cherel Y (2013) Penguins as bioindicators of mercury contamination in the Southern Ocean: birds from the Kerguelen Islands as a case study. Science of the Total Environment 454–455, 141-148.
| Crossref | Google Scholar |
Commonwealth of Australia (2016) Australia New Zealand Food Standards – Schedule 19. Maximum levels of contaminants and natural toxicants. Available at www.foodstandards.gov.au/code/Documents/Sched 19 Contaminant MLs v157.pdf
Cortés-Gómez AA, Romero D, Girondot M (2017) The current situation of inorganic elements in marine turtles: a general review and meta-analysis. Environmental Pollution 229, 567-585.
| Crossref | Google Scholar |
Crain DD, Friedlaender AS, Johnston DW, Nowacek DP, Roberts BL, Urian KW, Waples DM, Read AJ (2014) A quantitative analysis of the response of short-finned pilot whales, Globicephala macrorhynchus, to biopsy sampling. Marine Mammal Science 30, 819-826.
| Crossref | Google Scholar |
Cuvin-Aralar MLA, Furness RW (1991) Mercury and selenium interaction: a review. Ecotoxicology and Environmental Safety 21, 348-364.
| Crossref | Google Scholar |
Das K, Debacker V, Bouquegneau JM (2000) Metallothioneins in marine mammals. Cellular and Molecular Biology 46, 283-294.
| Google Scholar |
Denton GRW, Breck WG (1981) Mercury in tropical marine organisms from north Queensland. Marine Pollution Bulletin 12, 116-121.
| Crossref | Google Scholar |
Denton GRW, Marsh H, Heinsohn GE, Burdon-Jones C (1980) The unusual metal status of the dugong Dugon dugon. Marine Biology 57, 201-219.
| Crossref | Google Scholar |
Dietz R, Nørgaard J, Hansen JC (1998) Have Arctic marine mammals adapted to high cadmium levels? Marine Pollution Bulletin 36, 490-492.
| Crossref | Google Scholar |
Dunlop JN, McNeill S (2017) Local movements, foraging patterns, and heavy metals exposure in Caspian Terns Hydroprogne caspia breeding on Penguin Island, Western Australia. Marine Ornithology 45, 115-120.
| Google Scholar |
Eagles-Smith CA, Ackerman JT, Adelsbach TL, Takekawa JY, Miles AK, Keister RA (2008) Mercury correlations among six tissues for four waterbird species breeding in San Francisco Bay, California, USA. Environmental Toxicology and Chemistry 27, 2136-2153.
| Crossref | Google Scholar |
El Begearmi MM, Sunde ML, Ganther HE (1977) A mutual protective effect of mercury and selenium in Japanese quail. Poultry Science 56, 313-322.
| Crossref | Google Scholar |
Erftemeijer PLA, Djunarli , Moka W (1993) Stomach content analysis of a dugong (Dugong dugon) from South Sulawesi, Indonesia. Marine and Freshwater Research 44, 229-233.
| Crossref | Google Scholar |
Ewald JD, Kirk JL, Li M, Sunderland EM (2019) Organ-specific differences in mercury speciation and accumulation across ringed seal (Phoca hispida) life stages. Science of the Total Environment 650, 2013-2020.
| Crossref | Google Scholar |
Fea NI, Harcourt R, Lalas C (1999) Seasonal variation in the diet of New Zealand fur seals (Arctocephalus forsteri) at Otago Peninsula, New Zealand. Wildlife Research 26, 147-160.
| Crossref | Google Scholar |
Finger A, Lavers JL, Dann P, Nugegoda D, Orbell JD, Robertson B, Scarpaci C (2015) The Little Penguin (Eudyptula minor) as an indicator of coastal trace metal pollution. Environmental Pollution 205, 365-377.
| Crossref | Google Scholar |
Finger A, Lavers JL, Orbell JD, Dann P, Nugegoda D, Scarpaci C (2016) Seasonal variation and annual trends of metals and metalloids in the blood of the Little Penguin (Eudyptula minor). Marine Pollution Bulletin 110, 261-273.
| Crossref | Google Scholar |
Finger A, Lavers JL, Dann P, Kowalczyk ND, Scarpaci C, Nugegoda D, Orbell JD (2017) Metals and metalloids in Little Penguin (Eudyptula minor) prey, blood and faeces. Environmental Pollution 223, 567-574.
| Crossref | Google Scholar |
Fraga NS, Martins AS, Faust DR, Sakai H, Bianchini A, da Silva CC, Aguirre AA (2018) Cadmium in tissues of green turtles (Chelonia mydas): a global perspective for marine biota. Science of the Total Environment 637–638, 389-397.
| Crossref | Google Scholar |
Friberg L, Elinder CG, Kjellstrom T (1992) Environmental health criteria 134 Cadmium. International programme on chemical safety. Available at inchem.org/documents/ehc/ehc/ehc134.htm
Friedman MA, Eaton LR, Carter WH (1978) Protective effects of freeze dried swordfish on methylmercury chloride toxicity in rats. Bulletin of Environmental Contamination and Toxicology 19, 436-443.
| Crossref | Google Scholar |
Fujihara J, Kunito T, Kubota R, Tanabe S (2003) Arsenic accumulation in livers of pinnipeds, seabirds and sea turtles: subcellular distribution and interaction between arsenobetaine and glycine betaine. Comparative Biochemistry and Physiology Part C: Toxicology & Pharmacology 136, 287-296.
| Crossref | Google Scholar |
Ganther HE, Goudie C, Sunde ML, Kopecky MJ, Wagner P, Oh SH, Hoekstra WG (1972) Selenium: relation to decreased toxicity of methylmercury added to diets containing tuna. Science 175, 1122-1124.
| Crossref | Google Scholar |
Garrigue C, Derville S (2022) Behavioral responses of humpback whales to biopsy sampling on a breeding ground: the influence of age-class, reproductive status, social context, and repeated sampling. Marine Mammal Science 38, 102-117.
| Crossref | Google Scholar |
Gaus C, Grant S, Jin NL, Goot K, Villa A, Neugebauer F, Qi L, Limpus C (2012) Investigation of contaminant levels in green turtles from Gladsone. Entox final report. 10.13140/RG.2.2.10491.11045
Gerpe MS, De León AP, Bastida R, Moreno VJ, Rodriguez DH (2009) Sharp accumulation of heavy metals after weaning in the South American fur seal Arctocephalus australis. Marine Ecology Progress Series 375, 239-245.
| Crossref | Google Scholar |
Gilmour ME, Holmes ND, Fleishman AB, Kriwoken LK (2019a) Temporal and interspecific variation in feather mercury in four penguin species from Macquarie Island, Australia. Marine Pollution Bulletin 142, 282-289.
| Crossref | Google Scholar |
Gilmour ME, Lavers JL, Lamborg C, Chastel O, Kania SA, Shaffer SA (2019b) Mercury as an indicator of foraging ecology but not the breeding hormone prolactin in seabirds. Ecological Indicators 103, 248-259.
| Crossref | Google Scholar |
Gladstone W (1996) Trace metals in sediments, indicator organisms and traditional seafoods of the Torres Strait. Great Barrier Reef Marine Park Authority Report. Available at http://hdl.handle.net/11017/262
Gochfeld M (1980) Tissue distribution of mercury in normal and abnormal young Common Terns. Marine Pollution Bulletin 11, 362-366.
| Crossref | Google Scholar |
Guirlet E, Das K (2012) Cadmium toxicokinetics and bioaccumulation in turtles: trophic exposure of Trachemys scripta elegans. Ecotoxicology 21, 18-26.
| Crossref | Google Scholar |
Haynes D, Carter S, Gaus C, Müller J, Dennison W (2005) Organochlorine and heavy metal concentrations in blubber and liver tissue collected from Queensland (Australia) dugong (Dugong dugon). Marine Pollution Bulletin 51, 361-369.
| Crossref | Google Scholar |
Heinsohn GE, Birch WR (1972) Foods and feeding habits of the dugong, Dugong dugong (erxleben), in Northern Queensland, Australia. Mammalia 36, 414-422.
| Crossref | Google Scholar |
Hindell MA, Brothers N, Gales R (1999) Mercury and cadmium concentrations in the tissues of three species of southern albatrosses. Polar Biology 22, 102-108.
| Crossref | Google Scholar |
Honda K, Tatsukawa R, Itano K, Miyazaki N, Fujiyama T (1983) Heavy metal concentrations in muscle, liver and kidney tissue of striped dolphin, Stenella coeruleoalba, and their variations with body length, weight, age and sex. Agricultural and Biological Chemistry 47, 1219-1228.
| Crossref | Google Scholar |
Horne RSC (1985) Diet of royal and rockhopper penguins at Macquarie Island. Emu - Austral Ornithology 85, 150-156.
| Crossref | Google Scholar |
Hume F, Hindell MA, Pemberton D, Gales R (2004) Spatial and temporal variation in the diet of a high trophic level predator, the Australian fur seal (Arctocephalus pusillus doriferus). Marine Biology 144, 407-415.
| Crossref | Google Scholar |
Ikonomopoulou MP, Olszowy H, Limpus C, Francis R, Whittier J (2011) Trace element concentrations in nesting flatback turtles (Natator depressus) from Curtis Island, Queensland, Australia. Marine Environmental Research 71, 10-16.
| Crossref | Google Scholar |
Jepson PD, Deaville R, Barber JL, Aguilar À, Borrell A, Murphy S, Barry J, Brownlow A, Barnett J, Berrow S, Cunningham AA, Davison NJ, Ten Doeschate M, Esteban R, Ferreira M, Foote AD, Genov T, Giménez J, Loveridge J, et al. (2016) PCB pollution continues to impact populations of orcas and other dolphins in European waters. Scientific Reports 6, 18573.
| Crossref | Google Scholar |
Julshamn K, Grahl-Nielsen O (2000) Trace element levels in harp seal (Pagophilus groenlandicus) and hooded seal (Cystophora cristata) from the Greenland Sea. A multivariate approach. Science of the Total Environment 250, 123-133.
| Crossref | Google Scholar |
Kampalath R, Gardner SC, Méndez-Rodríguez L, Jay JA (2006) Total and methylmercury in three species of sea turtles of Baja California Sur. Marine Pollution Bulletin 52, 1816-1823.
| Crossref | Google Scholar |
Kemper C, Gibbs P, Obendorf D, Marvanek S, Lenghaus C (1994) A review of heavy metal and organochlorine levels in marine mammals in Australia. Science of the Total Environment 154, 129-139.
| Crossref | Google Scholar |
Koeman JH, Peeters WHM, Koudstaal-Hol CHM, Tjioe PS, De Goeij JJM (1973) Mercury-selenium correlations in marine mammals. Nature 245, 385-386.
| Crossref | Google Scholar |
Komoroske LM, Lewison RL, Seminoff JA, Deheyn DD, Dutton PH (2011) Pollutants and the health of green sea turtles resident to an urbanized estuary in San Diego, CA. Chemosphere 84, 544-552.
| Crossref | Google Scholar |
Kuenstl L, Griesel S, Prange A, Goessler W (2009) Arsenic speciation in bodily fluids of harbor seals (Phoca vitulina) and harbor porpoises (Phocoena phocoena). Environmental Chemistry 6, 319-327.
| Crossref | Google Scholar |
Labrada-Martagón V, Tenorio Rodríguez PA, Méndez-Rodríguez LC, Zenteno-Savín T (2011) Oxidative stress indicators and chemical contaminants in East Pacific green turtles (Chelonia mydas) inhabiting two foraging coastal lagoons in the Baja California peninsula. Comparative Biochemistry and Physiology Part C: Toxicology & Pharmacology 154, 65-75.
| Crossref | Google Scholar |
Lavers JL, Bond AL (2013) Contaminants in indigenous harvests of apex predators: the Tasmanian Short-tailed Shearwater as a case study. Ecotoxicology and Environmental Safety 95, 78-82.
| Crossref | Google Scholar |
Lavers JL, Bond AL, Hutton I (2014) Plastic ingestion by Flesh-footed Shearwaters (Puffinus carneipes): implications for fledgling body condition and the accumulation of plastic-derived chemicals. Environmental Pollution 187, 124-129.
| Crossref | Google Scholar |
Lavery TJ, Butterfield N, Kemper CM, Reid RJ, Sanderson K (2008) Metals and selenium in the liver and bone of three dolphin species from South Australia, 1988–2004. Science of the Total Environment 390, 77-85.
| Crossref | Google Scholar |
Law RJ, Fileman CF, Hopkins AD, Baker JR, Harwood J, Jackson DB, Kennedy S, Martin AR, Morris RJ (1991) Concentrations of trace metals in the livers of marine mammals (seals, porpoises and dolphins) from waters around the British Isles. Marine Pollution Bulletin 22, 183-191.
| Crossref | Google Scholar |
Law RJ, Morris RJ, Allchin CR, Jones BR, Nicholson MD (2003) Metals and organochlorines in small cetaceans stranded on the east coast of Australia. Marine Pollution Bulletin 46, 1206-1211.
| Crossref | Google Scholar |
Lischka A, Betty EL, Braid HE, Pook CJ, Gaw S, Bolstad KSR (2021) Trace element concentrations, including Cd and Hg, in long-finned pilot whales (Globicephala melas edwardii) mass stranded on the New Zealand coast. Marine Pollution Bulletin 165, 112084.
| Crossref | Google Scholar |
Littnan CL, Arnould JPY, Harcourt R (2007) Effect of proximity to the shelf edge on the diet of female Australian fur seals. Marine Ecology Progress Series 338, 257-267.
| Crossref | Google Scholar |
Lock JW, Thompson DR, Furness RW, Bartle JA (1992) Metal concentrations in seabirds of the New Zealand region. Environmental Pollution 75, 289-300.
| Crossref | Google Scholar |
Long M, Reid RJ, Kemper CM (1998) Cadmium accumulation and toxicity in the bottlenose dolphin Tursiops truncatus, the common dolphin Delphinus delphis, and some dolphin prey species in South Australia. Australian Mammalogy 20, 25-33.
| Crossref | Google Scholar |
López-Berenguer G, Peñalver J, Martínez-López E (2020) A critical review about neurotoxic effects in marine mammals of mercury and other trace elements. Chemosphere 246, 125688.
| Crossref | Google Scholar |
Luoma SN, Rainbow PS (2008) ‘Metal contamination in aquatic environments: science and lateral management.’ (Cambridge University Press: Cambridge, UK). Available at www.cabdirect.org/cabdirect/abstract/20083330272
Lynch M, Kirkwood R, Gray R, Robson D, Burton G, Jones L, Sinclair R, Arnould JPY (2012) Characterization and causal investigations of an alopecia syndrome in Australian fur seals (Arctocephalus pusillus doriferus). Journal of Mammalogy 93, 504-513.
| Crossref | Google Scholar |
Manning T, Ross GA, Symons R (2008) Environmental contaminants in white-bellied sea eagles (Halialeetus leucogaster) found in Sydney, Australia. Australiasian Journal of Ecotoxicology 14, 21-30.
| Crossref | Google Scholar |
Marcovecchio JE, Gerpe MS, Moreno VJ, Bastida RO, Rodriguez DH (1991) Organ tissue distribution of total mercury in South American Fur Seal, Arctocephalus australis. In ‘Heavy Metals in the Environment Proceedings of the 8th International Conference’, Edinburgh, UK. Available at http://hdl.handle.net/1834/26696
Marsh HE (1989) Mass Stranding of Dugongs by a tropical cyclone in Northern Australia. Marine Mammal Science 5, 78-84.
| Crossref | Google Scholar |
Mazzaro LM, Dunn St JL, St. Aubin DJ, Andrews GA, Chavey PS (2004) Serum indices of body stores of iron in northern fur seals (Callorhinus ursinus) and their relationship to hemochromatosis. Zoo Biology 23, 205-218.
| Crossref | Google Scholar |
McIntyre AD, Kinne O (1979) Marine Ecology. The Journal of Animal Ecology 48, 332-333.
| Crossref | Google Scholar |
McManus TJ, Wapstra JE, Guiler ER, Munday BL, Obendorf DL (1984) Cetacean strandings in Tasmania from February 1978 to May 1983. Papers and Proceedings of the Royal Society of Tasmania 118, 117-135.
| Crossref | Google Scholar |
Monk A, Charlton-Robb K, Buddhadasa S, Thompson RM (2014) Comparison of mercury contamination in live and dead dolphins from a newly described species, Tursiops australis. PLoS One 9, e104887.
| Crossref | Google Scholar |
Moser R, Zaccarini F, Alber T, Kerbl R (2020) First finding of tiemannite, HgSe, in human bladder stones: an electron microprobe study. Micron 138, 102928.
| Crossref | Google Scholar |
Munday BL (1985) Mercury levels in the musculature of stranded whales in Tasmania. Tasmanian Fisheries Research 27, 11-13.
| Google Scholar |
Nakazawa E, Ikemoto T, Hokura A, Terada Y, Kunito T, Tanabe S, Nakai I (2011) The presence of mercury selenide in various tissues of the striped dolphin: Evidence from μ-XRF-XRD and XAFS analyses. Metallomics 3, 719-725.
| Crossref | Google Scholar |
NOAA (2022) National marine mammal tissue bank. Available at www.fisheries.noaa.gov/national/marine-mammal-protection/national-marine-mammal-tissue-bank
Noren DP, Mocklin JA (2012) Review of cetacean biopsy techniques: factors contributing to successful sample collection and physiological and behavioral impacts. Marine Mammal Science 28, 154-199.
| Crossref | Google Scholar |
O’Shea TJ, Moore JF, Kochman HI (1984) Contaminant concentrations in Manatees in Florida. The Journal of Wildlife Management 48, 741-748.
| Crossref | Google Scholar |
Peterson SA, Ralston NVC, Peck DV, Sickle JV, Robertson JD, Spate VL, Morris JS (2009) How might selenium moderate the toxic effects of mercury in stream fish of the Western U.S.? Environmental Science & Technology 43, 3919-3925.
| Crossref | Google Scholar |
Pople AR, Gordon AN, Ng J (1998) Trace metal concentrations in livers and kidneys of sea turtles from south-eastern Queensland, Australia. Marine and Freshwater Research 49, 409-414.
| Crossref | Google Scholar |
Puget Sound water Quality Authority (1994) Recommended guidelines for sampling marine mamal tissue for chemical analysis in Puget Sound. Available at https://www.cascadiaresearch.org/files/sampling.pdf
Puskic PS, Lavers JL, Adams LR, Bond AL (2020) Ingested plastic and trace element concentrations in Short-tailed Shearwaters (Ardenna tenuirostris). Marine Pollution Bulletin 155, 111143.
| Crossref | Google Scholar |
Rainbow PS (Ed.) (2018) Coastal seas and oceans. In ‘Trace Metals in the Environment and Living Organisms: The British Isles as a Case Study’. (Ed. PS Rainbow) pp. 565–654. (Cambridge University Press: Cambridge, UK) 10.1017/9781108658423.008
Ralston NVC, Ralston CR, Blackwell III JL, Raymond LJ (2008) Dietary and tissue selenium in relation to methylmercury toxicity. NeuroToxicology 29, 802-811.
| Crossref | Google Scholar |
Riget F, Muir D, Kwan M, Savinova T, Nyman M, Woshner V, O’Hara T (2005) Circumpolar pattern of mercury and cadmium in ringed seals. Science of the Total Environment 351–352, 312-322.
| Crossref | Google Scholar |
Rosen DAS, Worthy G (2018) Nutrition and energetics. In ‘CRC Handbook of Marine Mammal Medicine’, 3rd edn. (Eds FMD Gulland, LA Dierauf, KL Whitman) pp. 791–827. (CRC Press: Boca Raton, FL, USA) 10.1201/9781315144931
SA EPA (2000) Monitoring Report: heavy metals and PCBs in dolphins, sediment and fish. South Australian Environmental Protection agency report. Available at http://www.epa.sa.gov.au/files/477410_dolphin.pdf
Savery LC, Evers DC, Wise SS, Falank C, Wise J, Gianios C, Kerr I, Payne R, Thompson WD, Perkins C, Zheng T, Zhu C, Benedict L, Wise JP (2013) Global mercury and selenium concentrations in skin from free-ranging sperm whales (Physeter macrocephalus). Science of the Total Environment 450–451, 59-71.
| Crossref | Google Scholar |
Savery LC, Wise SS, Falank C, Wise J, Gianios C, Douglas Thompson W, Perkins C, Zheng T, Zhu C, Wise JP (2014) Global assessment of oceanic lead pollution using sperm whales (Physeter macrocephalus) as an indicator species. Marine Pollution Bulletin 79, 236-244.
| Crossref | Google Scholar |
Stavros HCW, Bossart GD, Hulsey TC, Fair PA (2008) Trace element concentrations in blood of free-ranging bottlenose dolphins (Tursiops truncatus): Influence of age, sex and location. Marine Pollution Bulletin 56, 371-379.
| Crossref | Google Scholar |
Stavros HCW, Stolen M, Durden WN, McFee W, Bossart GD, Fair PA (2011) Correlation and toxicological inference of trace elements in tissues from stranded and free-ranging bottlenose dolphins (Tursiops truncatus). Chemosphere 82, 1649-1661.
| Crossref | Google Scholar |
Stockin KA, Law RJ, Duignan PJ, Jones GW, Porter L, Mirimin L, Meynier L, Orams MB (2007) ) Trace elements, PCBs and organochlorine pesticides in New Zealand common dolphins (Delphinus sp.). Science of the Total Environment 387, 333-345.
| Crossref | Google Scholar |
Sun X, Yu RQ, Zhang M, Zhang X, Chen X, Xiao Y, Ding Y, Wu Y (2017) Correlation of trace element concentrations between epidermis and internal organ tissues in Indo-Pacific humpback dolphins (Sousa chinensis). Science of the Total Environment 605–606, 238-245.
| Crossref | Google Scholar |
Teigen SW, Andersen RA, Daae HL, Skaare JU (1999) Heavy metal content in liver and kidneys of grey seals (Halichoerus grypus) in various life stages correlated with metallothionein levels: some metal—binding characteristics of this protein. Environmental Toxicology and Chemistry 18, 2364-2369.
| Crossref | Google Scholar |
Thomas CR, Bennett WW, Garcia C, Simmonds A, Honchin C, Turner R, Madden Hof CA, Bell I (2020) Coastal bays and coral cays: multi-element study of Chelonia mydas forage in the Great Barrier Reef (2015–2017). Science of the Total Environment 740, 140042.
| Crossref | Google Scholar |
UN Environment (2019) ‘Global Mercury Assessment 2018.’ (UN Environment Programme, Chemicals and Health Branch: Geneva, Switzerland) Available at https://www.unep.org/resources/publication/global-mercury-assessment-2018?_ga=2.44504609.409835767.1671522531-1889810577.1671235716
UNEP (2010) ‘Ridding the World of POPs: A Guide to the Stockholm Convention.’ (United Nations Environmental Program: Geneva, Switzerland) Available at https://digitallibrary.un.org/record/752644?ln=en
van de Merwe JP, Hodge M, Olszowy HA, Whittier JM, Lee SY (2010) Using blood samples to estimate persistent organic pollutants and metals in green sea turtles (Chelonia mydas). Marine Pollution Bulletin 60, 579-588.
| Crossref | Google Scholar |
Villa CA, Flint M, Bell I, Hof C, Limpus CJ, Gaus C (2017) Trace element reference intervals in the blood of healthy green sea turtles to evaluate exposure of coastal populations. Environmental Pollution 220, 1465-1476.
| Crossref | Google Scholar |
Villa CA, Bell I, Madden Hof C, Limpus CJ, Gaus C (2019) Elucidating temporal trends in trace element exposure of green turtles (Chelonia mydas) using the toxicokinetic differences of blood and scute samples. Science of the Total Environment 651, 2450-2459.
| Crossref | Google Scholar |
Wagemann R, Muir DCG (1984) Concentrations of heavy metals and organochlorines in marine mammals of northern waters: overview and evaluation. Canadian Technical Report of Fisheries and Aquatic Sciences No. 1279. Available at http://pubs.aina.ucalgary.ca/misc/14594.pdf
Wagemann R, Trebacz E, Boila G, Lockhart WL (1998) Methylmercury and total mercury in tissues of arctic marine mammals. Science of the Total Environment 218, 19-31.
| Crossref | Google Scholar |
Wagemann R, Trebacz E, Boila G, Lockhart WL (2000) Mercury species in the liver of ringed seals. Science of the Total Environment 261, 21-32.
| Crossref | Google Scholar |
Waterhouse J, Apte SC, Petus C, Angel BM, Wolanski E, Bainbridge S, Tracey D, Jarolimek CV, King J, Mellors J, Brodie J (2021) Assessing the influence of the Fly River discharge on the Torres Strait. Report to the National Environmental Science Program. Available at nesptropical.edu.au/wp-content/uploads/2021/06/NESP-TWQ-Project-5.14-Final-Report_COMPLETE.pdf
Weijs L, Vijayasarathy S, Villa CA, Neugebauer F, Meager JJ, Gaus C (2016) Screening of organic and metal contaminants in Australian humpback dolphins (Sousa sahulensis) inhabiting an urbanised embayment. Chemosphere 151, 253-262.
| Crossref | Google Scholar |
Woolford L, Franklin C, Whap T, Loban F, Lanyon JM (2015) Pathological findings in wild harvested dugongs Dugong dugon of central Torres Strait, Australia. Diseases of Aquatic Organisms 113, 89-102.
| Crossref | Google Scholar |
Woshner V, Knott K, Wells R, Willetto C, Swor R, O’Hara T (2008) Mercury and selenium in blood and epidermis of bottlenose dolphins (Tursiops truncatus) from Sarasota Bay, FL: interaction and relevance to life history and hematologic parameters. EcoHealth 5, 360-370.
| Crossref | Google Scholar |