Water use dynamics of dryland wheat grown under elevated CO2 with supplemental nitrogen
Shihab Uddin

A Faculty of Veterinary and Agricultural Sciences, The University of Melbourne, Creswick, Vic. 3363, Australia.
B NSW Department of Primary Industries, Wagga Wagga Agricultural Institute, Wagga Wagga, NSW 2650, Australia.
C Southern Cross Plant Science, Southern Cross University, Lismore, NSW 2480, Australia.
D Agriculture Victoria Research, Department of Energy, Environment and Climate Action, Horsham, Vic. 3400, Australia.
E Department of Animal, Plant and Soil Sciences, Centre for AgriBioscience, La Trobe University, Bundoora, Vic. 3086, Australia.
F Centre for Agricultural Innovation, School of Agriculture and Food, Faculty of Science, The University of Melbourne, Parkville, Vic. 3010, Australia.
G School of Ecosystem and Forest Sciences, Faculty of Science, The University of Melbourne, 500 Yarra Boulevard, Burnley, Vic. 3010, Australia.
H Queensland Alliance for Agriculture and Food Innovation (QAAFI), the University of Queensland, St Lucia, Qld 4067, Australia.
I Faculty of Science, The University of Melbourne, Dookie, Vic. 3647, Australia.
Abstract
Elevated atmospheric CO2 (e[CO2]) and nitrogen (N) fertilisation stimulate biomass and yield of crops. However, their interactions depend on crop growth stages and may affect water use dynamics.
This study investigated the interactive effects of two N rates, 0 and 100 kg N ha−1, and two CO2 concentrations, ambient (a[CO2], ~400 μmol mol−1) and e[CO2] (~550 μmol mol−1), on biomass, yield and water use of two wheat cultivars, Wyalkatchem (N-use efficient) and Yitpi (local), using a free air CO2 enrichment facility.
Elevated [CO2] stimulated leaf area (10%, P = 0.003) and aboveground biomass (11%, P = 0.03). In addition, e[CO2] reduced stomatal conductance (25%, P < 0.001) and increased net assimilation rates (12%, P < 0.001), resulting in greater (40%, P < 0.001) intrinsic water use efficiency. During early growth stages, e[CO2] resulted in higher water use than a[CO2]; however, this difference disappeared later in the season, resulting in similar cumulative water use under both CO2 concentrations. Supplemental N stimulated grain yield of Yitpi by 14% while decreasing that of Wyalkatchem by 7% (N × cultivar, P = 0.063). With supplemental N, Yitpi maintained greater post-anthesis leaf N, chlorophyll content, canopy cover and net assimilation rate than Wyalkatchem.
During early growth stages, the e[CO2]-induced stimulation of leaf-level water use efficiency was offset by greater biomass, resulting in higher water use. By the end of the season, similar cumulative water use under both CO2 concentrations indicates the dominating effect of the prevailing seasonal conditions in the study area. Observed yield responses of the studied cultivars to supplemental N were associated with their ability to maintain post-anthesis photosynthetic capabilities.
Our findings suggest that N-use efficiency traits and responsiveness need to be considered independently to optimise benefits from the ‘CO2 fertilisation effect’ through breeding.
Keywords: AGFACE, climate change, dryland agriculture, FACE, leaf gas exchange, N-use efficiency, root length, water use.
Introduction
Atmospheric carbon dioxide concentration ([CO2]) has risen steadily from ~280 μmol mol−1 prior to the Industrial Revolution to >421 μmol mol−1 in June 2022 (NOAA 2022) measured at Mauna Loa. If effective mitigation strategies are not implemented, atmospheric [CO2] is projected to continue increasing exponentially in the coming decades, reaching ~550 μmol mol−1 by the mid-21st Century (IPCC 2013). As the main substrate of photosynthesis, increasing atmospheric [CO2] is known to stimulate growth and grain yield of crops, particularly those with the C3 photosynthetic pathway, through the so-called ‘CO2 fertilisation effect’ (Ainsworth and Long 2005). This CO2 fertilisation effect may offset some of the negative effects of extreme climatic events (e.g. heat waves and/or droughts) on crop production (Hatfield et al. 2011) but impacts on crop water use and N dynamics are less clear (Li et al. 2004; Manderscheid et al. 2018).
Elevated atmospheric [CO2] (e[CO2]) has been extensively investigated in relation to effects on crop performance. Studies have consistently shown that an increase of ~150 μmol mol−1 above ambient [CO2] (a[CO2]) can significantly enhance both biomass and grain yield in wheat, resulting in improvements ranging from 6% to 79% (Ainsworth and Long 2005; Kimball 2016; Fitzgerald et al. 2022). However, the magnitude of the observed CO2 fertilisation effect is contingent upon various growing conditions, including water and nitrogen (N) availability (Reich et al. 2014; Gray et al. 2016). The relative CO2 fertilisation effect has been reported to be stronger when water availability is limited (Leakey et al. 2009; Kimball 2016). Reduced transpiration under e[CO2] (Leakey et al. 2009; Kimball 2016) may improve the soil-water status (Burkart et al. 2011; Hussain et al. 2013). Under drier conditions, these water savings potentially extend well into the grain-filling stages during which carbon gain can be stimulated by e[CO2], thus mitigating the effect of drought on crop productivity (Wall 2001; Manderscheid and Weigel 2007). However, some recent experimental (Gray et al. 2016; Houshmandfar et al. 2016; Parvin et al. 2018), meta-analytical (van der Kooi et al. 2016) and modelling (O’Leary et al. 2015; Jin et al. 2018) analyses have not supported this view.
This complexity arises because e[CO2] does not always lead to water savings, and water use at the canopy level is dependent on the balance of gains in leaf-level water use efficiency (WUE) and expansion of leaf area (Tausz-Posch et al. 2020). Furthermore, water savings or soil-water depletion also vary because of differential response of stomatal conductance (gs) to e[CO2] depending on soil-water availability and canopy microclimate. Increases in canopy temperature due to e[CO2] (Kimball 2016) would increase leaf-to-air vapour pressure deficit and therefore evaporative demand (Ainsworth and Rogers 2007). Studies conducted at the Australian Grains Free Air CO2 Enrichment facility (AGFACE; in a dryland Mediterranean region) (Tausz-Posch et al. 2013; Houshmandfar et al. 2016) have reported lower relative reductions of gs in response to e[CO2] than studies conducted in fully irrigated systems (Garcia et al. 1998; Wall et al. 2000). These variations in gs response may contribute to regional disparities in changes in water use under e[CO2] (Deryng et al. 2016). Therefore, it is crucial to consider the context-specific nature of water use dynamics when examining the effects of e[CO2] on crop–water interactions.
The CO2 fertilisation effect is reported to be weaker when soil N supply limits plant growth (Weigel and Manderscheid 2012; Reich et al. 2014; Ainsworth and Long 2021; Lenka et al. 2021). In N-limited soil, e[CO2]-induced stimulation of green leaf area was reduced relative to non-limiting N supply (Wang et al. 2013). In high-yielding, high-rainfall or continuously irrigated agroecosystems where growth and yield often are N-limited, several studies reported a stronger response to e[CO2] with supplemental N (Ziska et al. 1996; Weigel and Manderscheid 2012; Pleijel et al. 2019). Stimulation of biomass with supplemental N under e[CO2] influences cumulative water use and WUE (Hunsaker et al. 2000; Manderscheid et al. 2018). The interactive effect of e[CO2] and N on biomass also depends on the crop growth stage (Ziska et al. 1996; Yang et al. 2006), which may affect the water use dynamics during the growing season. This will be particularly important in relatively low-yielding dryland Mediterranean regions where water limitations interact with N availability and where intra-seasonal variability is high (Angus 2001; Li et al. 2009). If N amplifies the e[CO2]-induced stimulation of biomass early in the season, soil water will be depleted faster, which in combination with the typical end-of-season terminal drought in Mediterranean environments may increase the risk of ‘haying-off’, a situation where the rapid depletion of soil water curtails grain filling prematurely (van Herwaarden et al. 1998; Nuttall et al. 2012).
The root system plays a crucial role as the interface between plants and the soil, facilitating the uptake of essential resources such as water and nutrients (Palta and Watt 2009). In dryland Mediterranean regions, the effect of e[CO2] on root characteristics holds significant implications for crop adaptation. Although there are a few exceptions (Benlloch-Gonzalez et al. 2014a), it is generally reported that e[CO2] stimulates root growth (Wechsung et al. 1999; Madhu and Hatfield 2013; Uddin et al. 2018a). Increased root length under e[CO2] can enhance rooting depth and improve access to subsoil water (Chaudhuri et al. 1990; Uddin et al. 2018b, 2018c), providing a valuable buffer against unpredictable rainfall patterns in water-limited conditions. Utilisation of subsoil water during the grain-filling period, which often occurs later in the season, is more efficient in converting water into grain than in-season rainfall (Kirkegaard et al. 2007; Wasson et al. 2012). The response of root growth to e[CO2] varies depending on the availability of soil water and N (Stulen and den Hertog 1993; Wechsung et al. 1999) as well as the growth stage of the crop (Weigel and Manderscheid 2012). Thus, it is crucial to investigate the extent and timing of interactions between [CO2] and N rates on root growth under dryland conditions, particularly during terminal drought periods.
In addition to the influence of soil water and N availability, crop responses to e[CO2] can also be influenced by genotypic variability. Identification of traits responsible for genetic variation in CO2 responsiveness is essential for effectively harnessing the benefits of rising [CO2] through breeding approaches (Tausz et al. 2013; Bishop et al. 2015). Wheat cultivars exhibit differences in their response to e[CO2] (Ziska 2008; Tausz-Posch et al. 2012); however, limited information is available on how supplemental N may affect intra-specific variability in response to e[CO2]. Furthermore, there is a need to comprehend the underlying processes governing this response, particularly under water-limited conditions where natural soil N content is insufficient to achieve maximum yield potential.
In this study, we investigated the seasonal dynamics of biomass production and water use of wheat (Triticum aestivum L.) in semi-arid, water-limited, wheat cropping systems of south-eastern Australia, using the AGFACE facility. One of the typical features of the study area is that water availability is generally non-limiting during early in the winter-wheat growing season, either from stored soil water or early-season rainfall. However, crops often experience water deficits during the flowering and grain-filling periods, referred to as terminal drought, which is considered the major cause of grain yield variability in these regions (Turner 2004). Under such water-limited conditions, the impact of e[CO2] on wheat yield can be attributed to several factors. First, the reduction in transpiration under e[CO2] can improve soil-water status during the early growth stages (Leakey et al. 2009; Kimball 2016). Second, early-season crop growth can provide soil shading, further contributing to improved water availability (Christy et al. 2018). Third, under e[CO2], wheat exhibits a deeper and denser root proliferation, enabling enhanced acquisition of subsoil water (Madhu and Hatfield 2013). These combined effects have the potential to impact wheat yield positively (Kirkegaard et al. 2007).
Our experimental site was characterised by low background soil N (Walker et al. 2017) compared with much higher levels of soil mineral N (>250 kg NO3−-N ha−1) measured in previous AGFACE studies (O’Leary et al. 2015; Bahrami et al. 2017), where N supplementation did not show any significant biomass and yield responses (Tausz et al. 2017b). This new site facilitates investigation of how supplemental N will affect growth and biomass production and thereby water use dynamics of wheat under e[CO2]. We chose two wheat cultivars for their putative differences in N acquisition and utilisation patterns: cv. Wyalkatchem, N-use efficient; Alhabbar et al. (2018); and cv. Yitpi, local practice. The experimental design allowed testing of the following hypotheses:
Supplemental N will stimulate the CO2 fertilisation effect, leading to increased water use under e[CO2] relative to a[CO2], primarily driven by aboveground biomass and leaf area growth.
Supplemental N under e[CO2] will stimulate root length growth, thereby improving subsoil water acquisition and subsequently increasing water use.
The N-use efficient cv. Wyalkatchem will exhibit a stronger response to e[CO2] than cv. Yitpi when supplemented with additional N.
Materials and methods
Plant materials
Wheat cvv. Yitpi and Wyalkatchem were selected for their similar grain yield potential but contrasting agronomic features. Yitpi is a free-tillering cultivar with long coleoptile and broad adaptation to most soil types and rainfall regions across Australia’s wheatbelt (Seednet 2005), whereas Wyalkatchem is characterised as having poor early vigour, short coleoptiles, high harvest index and adaption to low-rainfall regions (Intergrain 2001). Under low background soil N, cv. Wyalkatchem has been found to maintain higher grain protein than cv. Yitpi (Malik et al. 2016), most likely due to superior N acquisition and utilisation efficiencies as shown by its performance on a sandy, non-alkaline soil in Western Australia (Alhabbar et al. 2018).
Experimental design
This experiment was conducted in 2016 within the AGFACE facility in Horsham (36°44′57″S, 142°06′50″E; 127 m a.m.s.l.), Victoria, Australia. The study site has been described in detail by Mollah et al. (2009) and Fitzgerald et al. (2022), but the present experiment was conducted on an adjacent site with low background soil N (Walker et al. 2017). Soil type at the site is a Vertosol (Isbell 1996) clay soil (~35% clay at the surface and 60% at 1.4 m depth). The soil at this site has an alkaline pH throughout the profile with moderate sodicity (mainly >20 cm depth) and high levels of boron and salt (>40 cm depth) (Table 1). The site has a Mediterranean climate with cool, wet winters and dry summers (Hutchinson et al. 2005). Long-term (30-year, 1981–2010) average annual rainfall is 445 mm, with 314 mm typically falling during the wheat growing season (May–November) (Bureau of Meteorology 2017, Polkemmet Road #079023). For the same time period, average maximum and minimum temperatures were 17.8°C and 5.3°C, respectively. During the study year, total annual rainfall was 550 mm, of which 406 mm fell during May–November. Average daily maximum (17.6°C) and minimum (5.3°C) temperatures were similar to the long-term average. A summary of the environmental variables recorded by an on-site weather station (MEA Premium Weather Station 103; Measurement Engineering Australia, Magill, SA, Australia) during the studied growing season is given in Fig. 1.
Soil depth (cm) | pH (1:5 water) | EC (dS m−1) | CEC (cmol(+) kg−1) | ESP (%) | Colwell P (mg kg−1) | Organic C (%) | NO3−–N (mg kg−1) | NH4+–N (mg kg−1) | Boron (mg kg−1) | BD (g cm−3) | |
---|---|---|---|---|---|---|---|---|---|---|---|
0–10 | 8.4 ± 0.1 | 0.16 ± 0.03 | 36.9 ± 0.7 | 3.2 ± 0.2 | 24.9 ± 2.8 | 0.81 ± 0.03 | 15.9 ± 1.3 | 2.1 ± 0.2 | 3.4 ± 0.1 | 1.27 ± 0.02 | |
10–20 | 8.5 ± 0.1 | 0.22 ± 0.02 | 36.3 ± 1.1 | 6.8 ± 0.5 | 5.8 ± 0.8 | 0.52 ± 0.01 | 14.5 ± 1.1 | 1.6 ± 0.1 | 4.3 ± 0.4 | 1.45 ± 0.03 | |
20–40 | 8.7 ± 0.1 | 0.25 ± 0.02 | 36.2 ± 1.0 | 13.3 ± 0.7 | 3.5 ± 0.3 | 0.43 ± 0.02 | 5.9 ± 0.5 | 1.4 ± 0.1 | 6.8 ± 0.9 | 1.44 ± 0.03 | |
40–80 | 8.8 ± 0.1 | 0.59 ± 0.08 | 37.4 ± 1.2 | 25.7 ± 0.9 | 3.3 ± 0.3 | 0.25 ± 0.02 | 3.1 ± 0.4 | 0.8 ± 0.1 | 21.3 ± 2.3 | 1.44 ± 0.02 | |
80–120 | 8.8 ± 0.1 | 0.96 ± 0.10 | 39.6 ± 0.8 | 33.2 ± 0.9 | 2.2 ± 0.1 | 0.12 ± 0.01 | 2.5 ± 0.4 | 1.0 ± 0.1 | 33.1 ± 2.4 | 1.43 ± 0.01 |
Values are means ± s.e. of n = 10 (rings), and for each ring, samples from four cores were pooled.
EC, electrical conductivity; CEC, cation exchange capacity; ESP, exchangeable sodium percentage; BD, bulk density.
Daily maximum and minimum temperatures, rainfall and reference evapotranspiration (ETₒ) recorded on-site at the Australian Grains FACE (AGFACE) facility in Horsham, Victoria, Australia in 2016. Arrows indicate stem-elongation (growth stage DC31; Zadoks et al. 1974), anthesis (DC65) and physiological maturity (DC90) of wheat cvv. Yitpi (—) and Wyalkatchem (....).
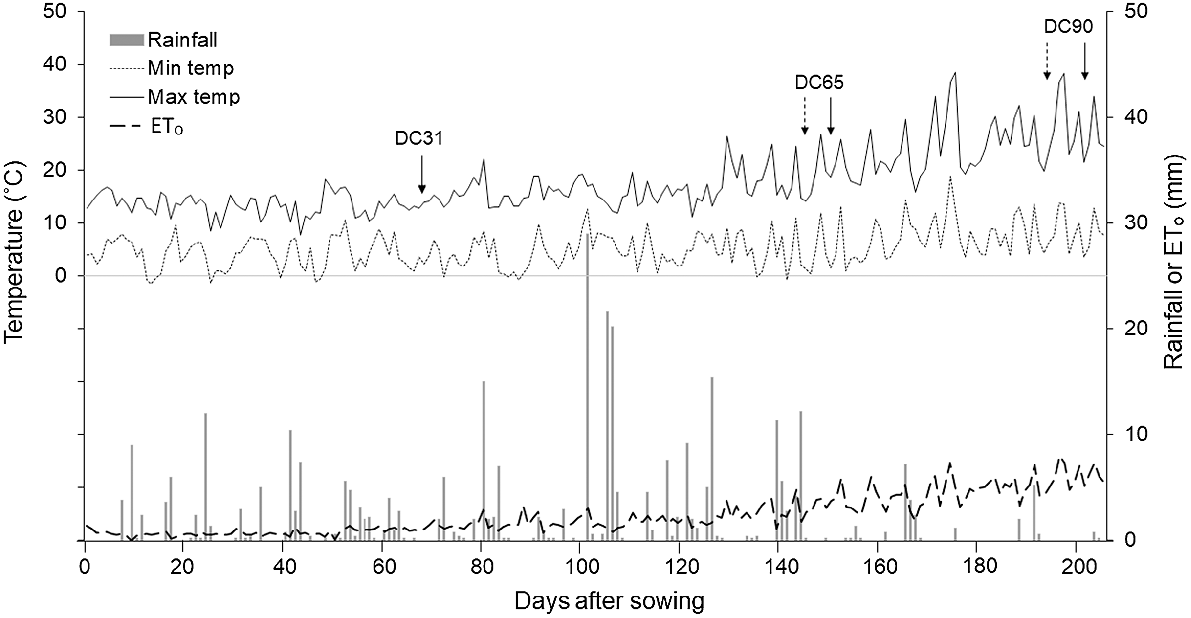
The experimental was a nested split-plot design with six replicate plots (octagonal ‘rings’) of 16 m diameter for a[CO2] (~400 μmol mol−1) and four rings for e[CO2] (~550 μmol mol−1) spaced at 60 m centre-to-centre. Normalised differences vegetation index (NDVI), profile probe, aboveground biomass and final harvest measurement were collected from the six a[CO2] rings and the four e[CO2] rings, whereas other periodic measurements (e.g. leaf gas exchange, green leaf area, N-Pen, SPAD and root growth) were acquired in four rings only for both a[CO2] and e[CO2] treatments. Within each ring, cvv. Yitpi and Wyalkatchem were randomly allocated to two subplots each, and each subplot was treated without (−N, 0 kg N ha−1) or with (+N, 100 kg N ha−1) supplemental N (see Supplementary Fig. S1) as granular urea lightly incorporated into the soil at sowing. Each subplot was 4 m long by 1.46 m wide and consisted of six rows with 0.244 m spacing. Plots were dry seeded (by drilling into 3–5 cm depth) in north–south orientation on 31 May 2016. At sowing, granular fertiliser (8.8% phosphorus (P), 11% sulfur (S)) was incorporated into the soil at rates of 8 kg P ha−1 and 10 kg S ha−1. Initial soil mineral N was 90.3 kg NO3−-N ha−1 (0–120 cm depth of soil profile).
At the perimeter of the e[CO2] octagonal rings, horizontal stainless-steel tubes were positioned about 15 cm above the canopy at any developmental stage. From sunrise to sunset, enrichment of the [CO2] of ambient air to the target of 550 μmol mol−1 was achieved by injecting pure CO2 into the air through 0.3-mm laser-drilled holes (20 holes m−1) on the upwind-side tubes. CO2 then quickly mixed with the air and was blown throughout the plot by prevailing wind. CO2 concentrations were monitored and recorded every minute with an infrared gas analyser (SBA-4; PP Systems, Amesbury, MA, USA) positioned at the centre of each ring. The performance of the CO2 delivery system is characterised in Mollah et al. (2009) and spatial variations of [CO2] within e[CO2] rings are described by Mollah et al. (2011).
Soil-water content and water use
Soil water was measured weekly with a PR2/6 Profile Probe (Delta-T Devices, Cambridge, UK) with six sensors positioned at 10, 20, 30, 40, 60 and 100 cm depth. A 100-cm-long, thin-walled fibreglass access tube (ATS1/ATL1; Delta-T Devices) was inserted into the soil in each plot and left in situ for the entire growing season (Fig. S1). To take a measurement, the PR2/6 capacitance probe was inserted into the access tube. It measures the dielectric constant (ε) via emission of an electromagnetic field 100 mm into the surrounding soil at each sensor depth. The output from each sensor is a DC voltage (in mV), which is converted to volumetric water content (m3 m−3) via a site-specific calibration curve (Whalley et al. 2004) developed for each soil depth of the AGFACE facilities in the earlier years. Soil-water content (mm) for each depth was calculated from the volumetric soil water multiplied by the corresponding depth increment and summed up to 100 cm depth. No irrigation was applied; therefore, water use between physiologically important growth stages was determined by the following equation:
where P is precipitation (mm) and SWD (mm) is the change in water content (mm) of the soil profile between the two measurements. The final measurement of soil-water content coincided with maturity of cv. Wyalkatchem, which occurred several days earlier than that of cv. Yitpi, possibly leading to an underestimate of Yitpi water use. Cumulative water use over the season was calculated by summing water use estimates at different growth stages.
Normalised difference vegetation index
Non-destructive measurements of canopy reflectance were done in all treatment subplots by using a handheld CropCircle ACS-210 with GeoSCOUT GLS-400 data logger (Holland Scientific, Lincoln, NE, USA), from which the NDVI was calculated. Measurements were taken from 51 to 189 days after sowing (DAS) at approximately weekly intervals. The sensor was held ~0.5 m above the crop canopy and oriented to measure a foot-print across the middle rows of the plot. The NDVI is calculated directly by the sensor, and mean values of transects from non-disturbed areas within the subplot were used as a surrogate for canopy cover (Perry et al. 2012).
Root length
Root length was measured at ~2-week intervals with a mini-rhizotron (CI-600; CID Bio-Science, Camas, WA, USA), which enables non-destructive monitoring of the same individual roots over the selected time intervals (Andersson and Majdi 2005). Detailed description of installing acrylic clear rhizo-tubes and acquiring the images of the root system for a similar experimental set-up in the AGFACE facility is given in Bahrami et al. (2017). In short, one 1.05-m-long rhizo-tube with internal diameter 7 cm was pre-installed at a 45° angle in each of the four subplots per ring (Fig. S1). For each rhizo-tube, four images were captured at four depths (1, 0–16 cm; 2, 17–32 cm; 3, 33–48 cm; 4, 49–64 cm) below the soil surface. The images were analysed for the captured root length per image using the software RootSnap! version 1.2.8.23 (CID Bio-Science). Total root length was calculated as the sum of root length across all four depths. The ratios of root length between the deeper (sum of Depths 3 and 4) and upper (sum of Depths 1 and 2) soil layers are reported.
Leaf gas exchange measurements
Stomatal conductance (gs) and net photosynthetic assimilation rate (Anet) of fully expanded flag leaves (or uppermost leaf when flag leaves were not yet fully expanded) were measured weekly from 90 to 170 DAS. An open-path infrared gas analyser (LI-6400; LI-COR, Lincoln, NE, USA) with a standard leaf chamber (clear-top with a maximum measurement area of 2 cm by 3 cm) was used to measure instantaneous gas exchange of a single plant per plot. The cuvette air flow rate was set to 500 μmol s−1 with [CO2] of either 400 or 550 μmol mol−1 depending on the [CO2] growth conditions. Measurements were performed on sunny days from 09:00 to 11:30, and photosynthetically active radiation ranged between 900 and 1250 μmol m−2 s−1 during measurement periods (Houshmandfar et al. 2015). Values were recorded after first stabilisation (generally after 90 s), and three measurements were recorded at an interval of 5 s and averaged. This allowed conditions in the cuvette to reach a steady state but did not allow stomata to adjust to the cuvette conditions. Vapour pressure deficit was between 0.9 and 2.2 kPa depending on measurement dates and was not different among samples and treatments on each date (Houshmandfar et al. 2015). After completion of the gas exchange measurements, the leaf surface area was calculated from the length and width of the part of the leaf enclosed in the cuvette. Values for all gas exchange parameters were calculated based on the surface area of the leaf inside the cuvette. Intrinsic WUE (iWUE) was calculated as Anet divided by gs.
Chlorophyll and N content
Surrogate measurements of chlorophyll and N content of flag leaves were taken non-destructively by chlorophyll meter (SPAD-502; Konica Minolta Sensing, Tokyo, Japan) and N analyser (N-Pen N 100; Photon Systems Instruments, Drásov, Czech Republic), at approximately weekly intervals. Both SPAD and N-Pen readings were taken on one side of the midrib and around the midpoint of the topmost leaf or flag leaf (Xiong et al. 2015). For each replicate subplot, SPAD and N-Pen values were averaged from the representative measurements of 10 and six leaves, respectively.
Growth, yield and morpho-physiological parameters
For determination of green leaf area, destructive samples were collected at approximately weekly to 2-week intervals from one week before stem elongation (DC31; Zadoks et al. 1974) until physiological maturity (DC90). Uniform individual plants were initially tagged at 40 DAS at the soil surface level to identify them clearly as single plants in later stages. Then at each sampling date, three predetermined and representative plants per plot were harvested (excluding edge rows). Green leaf area was measured with a leaf area meter (LI-3100C Area Meter; LI-COR).
For estimation of the area-based aboveground biomass, a predetermined subplot of 0.29, 0.49 and 0.73 m2 including the middle four rows was harvested at DC31, DC65 and DC90, respectively. At DC31 and DC65, samples were oven-dried for 72 h at 70°C, and at DC90, samples were oven-dried for the same time but at 40°C. Dry weights were recorded at each stage. At DC90, plant height was also recorded. Spikes were threshed to separate the grains, and both grain yield and 1000-grain weight were recorded. Harvest Index (HI) was calculated as grain yield divided by the aboveground biomass. WUE of biomass (WUEb) and grain yield (WUEy) were calculated by dividing aboveground biomass and grain yield with cumulative water use.
Statistical analyses
The experiment was a split-plot design with two [CO2] treatments as main-plots (rings) and two cultivars and two N rates in full factorial combination as subplots, with four e[CO2] and six a[CO2] replications. The effects of CO2, cultivars and N rates were considered fixed effects and ring and plot as random effects in a linear mixed effects model analysed with R package ‘nlme’ (Pinheiro et al. 2022). For repeatedly measured parameters, DAS was used as an additional fixed effect. Homogeneity of variances was evaluated visually, and the model was adjusted when stepwise evaluation of the model indicated an improvement after correction. Statistical effects were regarded as significant where P < 0.1. Data analyses were performed in R version 3.4.3 (R Core Team 2022) and mixed effects model P-values are reported in Supplementary Tables S1, S2 and S3.
Results
Plant density and phenological development
Plant density was significantly affected by cultivar (P = 0.056) and N rate (P = 0.040), where cv. Yitpi had 10% more seedlings established than cv. Wyalkatchem, and under supplemental N, 11% fewer seedlings were established than without supplemental N (Table 2). Wyalkatchem started flowering earlier and senesced faster, resulting in harvest 10 days earlier than Yitpi (Fig. 1); however, the other treatment variables had no significant effects on phenological development of either cultivar.
Response parameters | Yitpi | Wyalkatchem | P-value | |||||||||||||
---|---|---|---|---|---|---|---|---|---|---|---|---|---|---|---|---|
−N | +N | −N | +N | CO2 | N | Cv | CO2 × N | CO2 × Cv | N × Cv | CO2 × N × Cv | ||||||
a[CO2] | e[CO2] | a[CO2] | e[CO2] | a[CO2] | e[CO2] | a[CO2] | e[CO2] | |||||||||
Plant density (no. m−2) | 130.9 ± 5.4 | 130.1 ± 11.8 | 115.9 ± 8.9 | 111.0 ± 9.8 | 109.5 ± 7.3 | 123.0 ± 8.6 | 112.5 ± 3.5 | 97.7 ± 11.8 | 0.772 | 0.040 | 0.056 | 0.181 | 0.856 | 0.476 | 0.314 | |
Plant height (cm) | 108.5 ± 1.3 | 106.0 ± 1.3 | 111.8 ± 0.5 | 107.6 ± 0.7 | 87.0 ± 1.9 | 90.8 ± 2.1 | 87.3 ± 1.5 | 90.0 ± 2.5 | 0.892 | 0.449 | <0.001 | 0.511 | 0.011 | 0.340 | 0.799 | |
Aboveground biomass (g m−2) | 1280 ± 50.1 | 1409 ± 139.7 | 1470 ± 67.6 | 1654 ± 92.8 | 1133 ± 77.9 | 1286 ± 66.8 | 1320 ± 72.0 | 1450 ± 52.7 | 0.030 | 0.002 | 0.010 | 0.888 | 0.900 | 0.761 | 0.731 | |
Grain yield (g m−2) | 499.9 ± 15.9 | 538.5 ± 56.4 | 571.0 ± 33.9 | 615.1 ± 39.9 | 420.9 ± 34.4 | 500.5 ± 23.8 | 416.1 ± 35.3 | 442.1 ± 49.2 | 0.108 | 0.366 | <0.001 | 0.649 | 0.829 | 0.063 | 0.576 | |
Harvest index | 0.39 ± 0.01 | 0.38 ± 0.01 | 0.39 ± 0.01 | 0.37 ± 0.01 | 0.37 ± 0.01 | 0.39 ± 0.00 | 0.31 ± 0.01 | 0.30 ± 0.03 | 0.699 | <0.001 | <0.001 | 0.343 | 0.320 | 0.002 | 0.561 | |
1000-grain weight (g) | 46.8 ± 0.4 | 46.9 ± 0.6 | 41.1 ± 1.1 | 44.8 ± 1.9 | 43.4 ± 0.9 | 44.5 ± 0.9 | 39.4 ± 0.8 | 37.5 ± 3.4 | 0.448 | <0.001 | <0.001 | 0.872 | 0.240 | 0.604 | 0.098 | |
Cumulative water use (mm) | 272.6 ± 9.5 | 278.8 ± 10.6 | 300.7 ± 12.6 | 307.5 ± 4.5 | 274.8 ± 16.5 | 369.6 ± 12.1 | 280.4 ± 12.6 | 273.5 ± 3.5 | 0.789 | 0.099 | 0.079 | 0.776 | 0.343 | 0.264 | 0.851 | |
WUEy (kg yield ha−1 mm−1) | 18.5 ± 1.0 | 18.7 ± 1.8 | 19.3 ± 1.6 | 20.0 ± 1.5 | 15.4 ± 1.0 | 18.6 ± 1.0 | 15.1 ± 1.7 | 16.2 ± 1.9 | 0.242 | 0.967 | 0.008 | 0.706 | 0.425 | 0.309 | 0.517 | |
WUEb (kg biomass ha−1 mm−1) | 47.3 ± 2.6 | 48.8 ± 4.1 | 49.7 ± 3.9 | 53.9 ± 3.5 | 41.4 ± 2.1 | 47.9 ± 2.8 | 48.0 ± 4.3 | 53.1 ± 2.4 | 0.114 | 0.059 | 0.287 | 0.892 | 0.552 | 0.590 | 0.675 |
Data are means ± s.e. of n = 6 and 4 replicates for a[CO2] and e[CO2], respectively. P-values of mixed effects model with CO2 treatment (CO2), nitrogen rate (N) and cultivar (Cv) as well as their interactions are presented. Statistically significant (P < 0.1) effects are shown in bold.
Gas exchange parameters
Net assimilation rate (Anet) of wheat grown under e[CO2] was 12% greater than under a[CO2] averaged across all measurements (Fig. 2a, Table S1). With supplemental N, cv. Yitpi maintained higher Anet than cv. Wyalkatchem later in the season, but without supplemental N, differences between cultivars diminished as the season progressed (DAS × N × Cv, P = 0.002). Stomatal conductance was 25% lower under e[CO2] than a[CO2] averaged across all measurements, but this difference diminished as the growing season progressed (Fig. 2b). Yitpi maintained greater gs with supplemental N throughout the growing season, whereas this trend was reversed for Wyalkatchem so that, later in the season, gs was greater for wheat grown without supplemental N (DAS × N × Cv, P = 0.012). Elevated [CO2] increased iWUE, and differences between a[CO2] and e[CO2] increased later in the season with decreasing gs (Fig. 2c; DAS × CO2, P < 0.001). Early in the season, iWUE was similar for the two cultivars, but as the season progressed Yitpi had greater iWUE than Wyalkatchem (DAS × Cv, P = 0.005).
(a) Net photosynthetic assimilation rate (Anet), (b) stomatal conductance (gs), and (c) intrinsic water use efficiency (iWUE) measured weekly during the growing season of wheat cvv. Yitpi (Yit) and Wyalkatchem (Wyal) grown under ambient [CO2] (a[CO2], ~400 μmol mol−1) or elevated [CO2] (e[CO2], ~550 μmol mol−1) with (100 kg N ha−1) or without (0 kg N ha−1) supplemental N. Data are means and standard errors of n = 4 replicates.
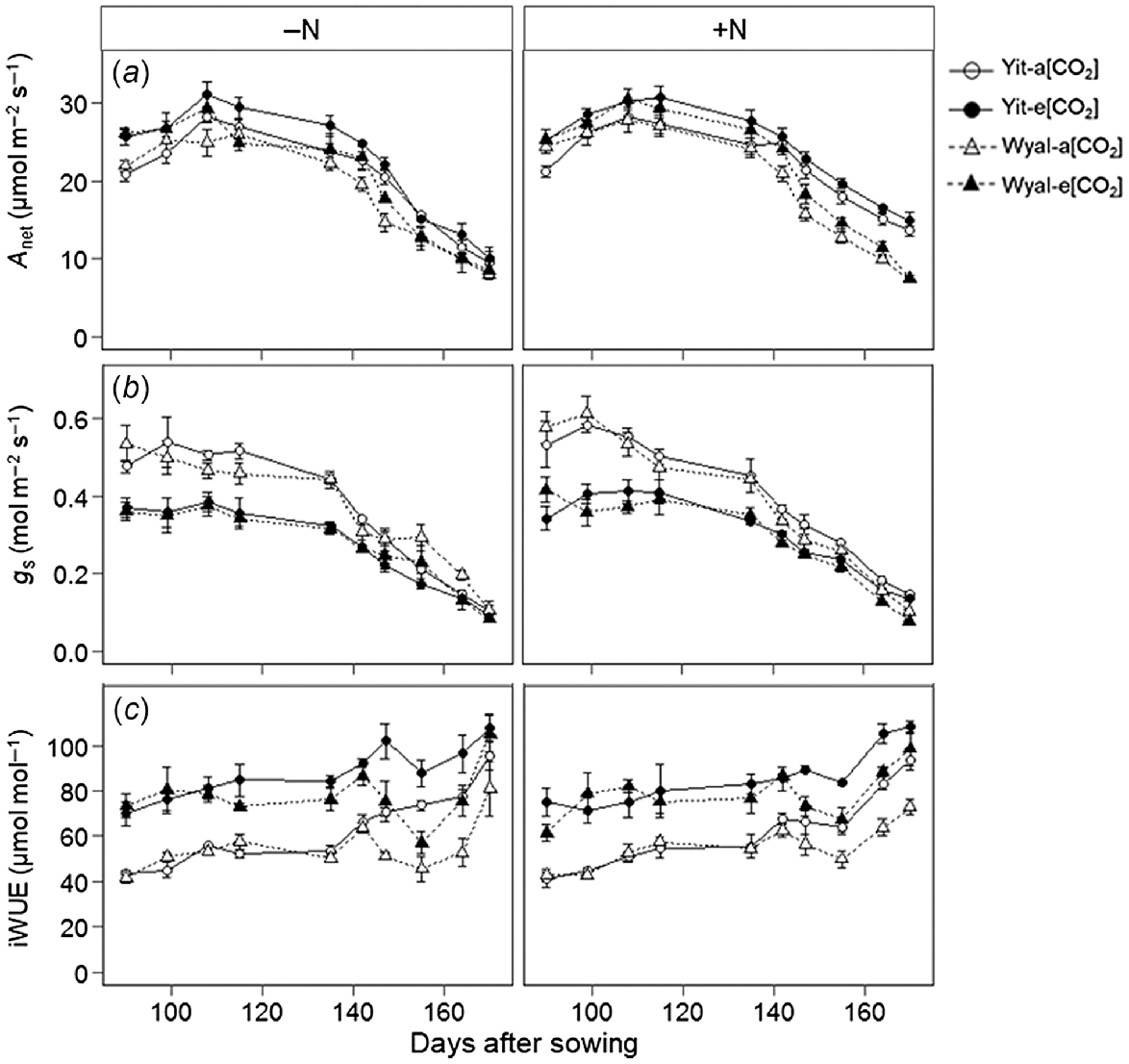
Green leaf area, chlorophyll and N content
Averaged across all measurement dates and treatments, green leaf area under e[CO2] was 10% greater than under a[CO2] (Fig. 3a, Table S1). Cv. Yitpi had greater green leaf area than cv. Wyalkatchem, with the difference increasing as the season progressed (DAS × Cv, P < 0.001). Supplemental N increased green leaf area and maintained greater leaf area for longer than when wheat was grown without supplemental N (DAS × N, P = 0.004). Supplemental N stimulated both leaf N and chlorophyll content, but to a greater extent for Yitpi than for Wyalkatchem (Fig. 3b, c). As the season progressed, Yitpi grown with supplemental N maintained greater leaf N and chlorophyll content than Wyalkatchem; however, these differences disappeared where no supplemental N was applied (DAS × N × Cv, P = 0.004 and <0.001 for leaf N and chlorophyll content, respectively).
(a) Green leaf area, (b) N concentration (N-Pen), and (c) chlorophyll content (SPAD) measured weekly during the growing season of wheat cvv. Yitpi (Yit) and Wyalkatchem (Wyal) grown under ambient [CO2] (a[CO2], ~400 μmol mol−1) or elevated [CO2] (e[CO2], ~550 μmol mol−1) with (100 kg N ha−1) or without (0 kg N ha−1) supplemental N. Data are means and standard errors of n = 4 replicates; each replicate of leaf area is an average of three plants; for N concentration and chlorophyll content, each replicate is an average of 6 and 10 measurements, respectively.
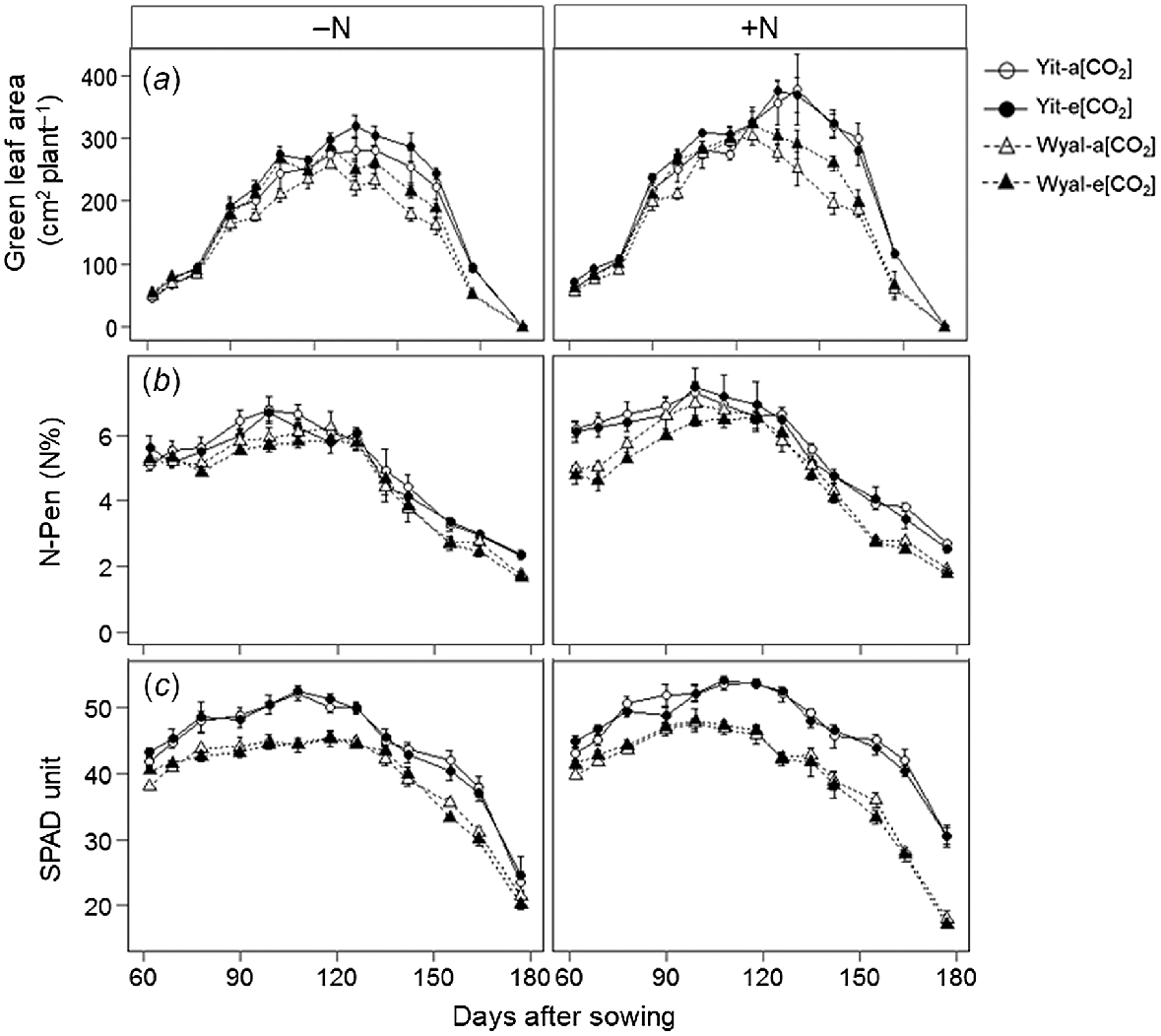
Normalised difference vegetation index
Both early and later in the season, NDVI of cv. Yitpi was greater than for cv. Wyalkatchem; however, this difference was not evident during canopy closure (maximum NDVI; Fig. 4, Table S1; DAS × Cv, P < 0.001). Wheat grown with supplemental N had higher NDVI values than wheat grown without supplemental N, and these differences increased throughout the season until maturity (DAS × N, P < 0.001).
Normalised differences vegetation index (NDVI) measured at weekly to 2-week intervals during the growing season of wheat cvv. Yitpi and Wyalkatchem grown under ambient [CO2] (a[CO2], ~400 μmol mol−1) or elevated [CO2] (e[CO2], ~550 μmol mol−1) with (100 kg N ha−1) or without (0 kg N ha−1) supplemental N. Data are means and standard errors of n = 6 and 4 replicates for a[CO2] and e[CO2], respectively.
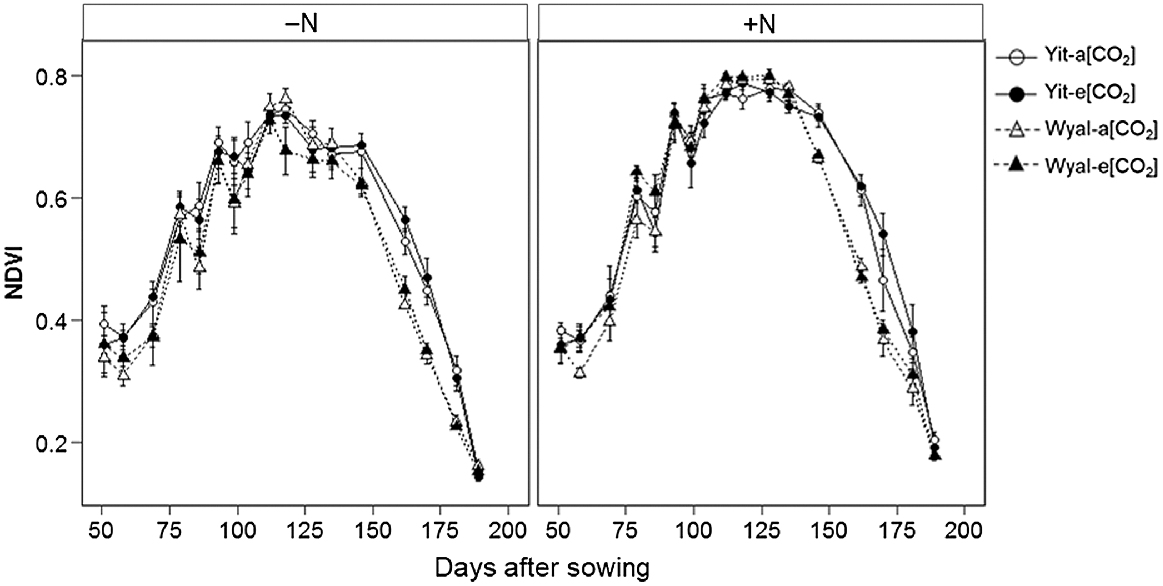
Root length
Effects of CO2, N rate and cultivar on root length depended on rooting depth (Fig. 5; CO2 × N × Cv × depth, P < 0.001). At Depth 1 (0–16 cm), root length of cv. Yitpi was smaller under e[CO2] than a[CO2] regardless of N supply (Fig. 5a, Table S2). Root length of cv. Wyalkatchem with supplemental N was greater under e[CO2] than a[CO2]; without supplemental N, [CO2] had no effect. At this depth, root length of Yitpi was greater than that of Wyalkatchem. The difference was greatest when maximum root length was achieved but was diminished before and after this time.
Captured root length at four depths of the soil profile: (a) 1, 0–16 cm; (b) 2, 17–32 cm; (c) 3, 33–48 cm; and (d) 4, 49–64 cm. (e) Total root length (sum of all depths); (f) ratio of root length between Depths 1 + 2 and Depths 3 + 4. Measurements taken at ~2-week intervals during the growing season of wheat cvv. Yitpi and Wyalkatchem grown under ambient [CO2] (a[CO2], ~400 μmol mol−1) or elevated [CO2] (e[CO2], ~550 μmol mol−1) with (100 kg N ha−1) or without (0 kg N ha−1) supplemental N. Data are means and standard errors of n = 4 replicates.
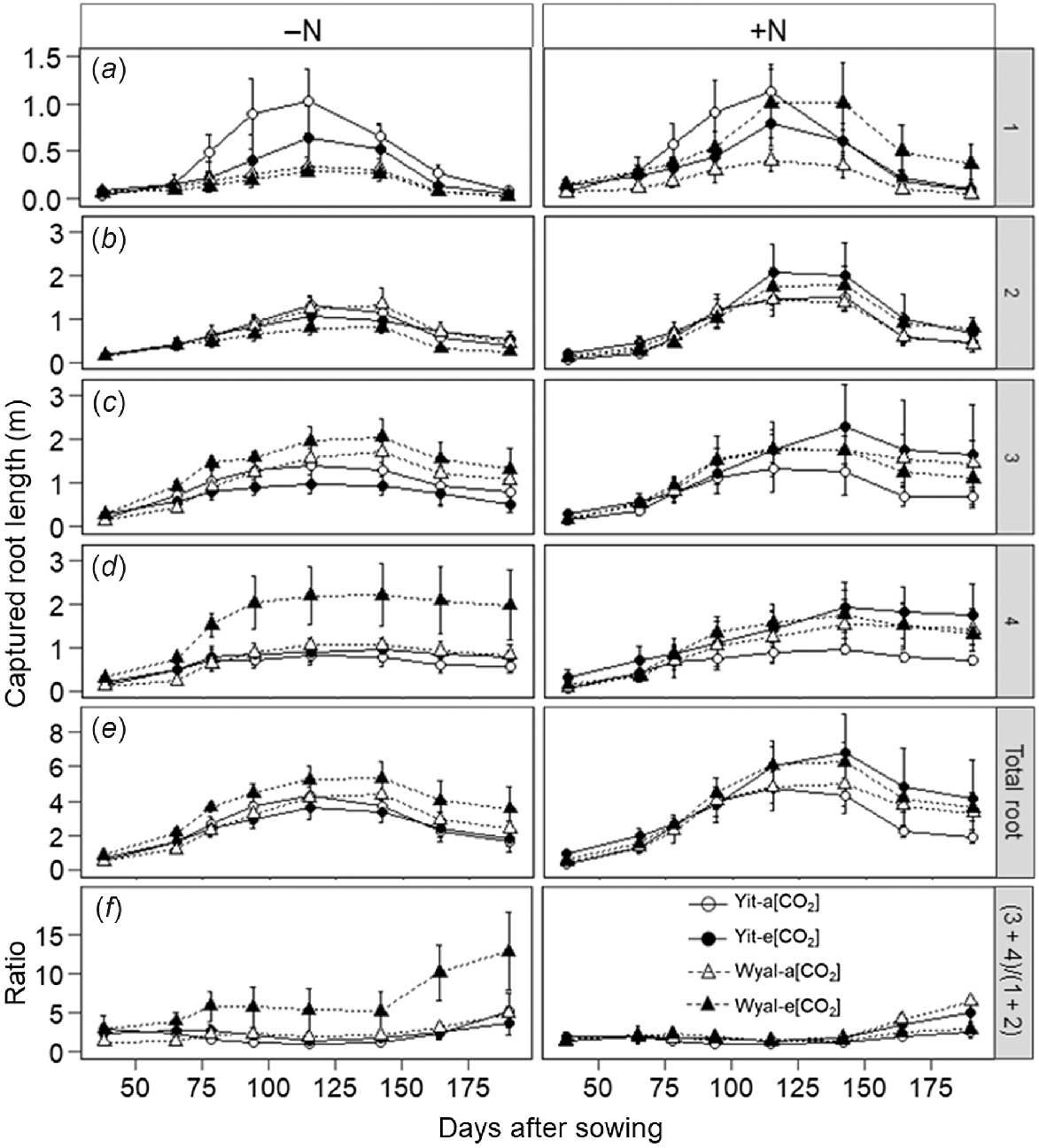
At Depth 2 (17–32 cm), root length was smaller under e[CO2] than a[CO2] in the absence of supplemental N, but with supplemental N, root length was greater under e[CO2] than a[CO2] (Fig. 5b, Table S2). At this depth, stimulation of root length by supplemental N was most pronounced during the period of maximum root length but the difference was less both before and after this time. Three-way interactions CO2 × N × Cv were significant (P < 0.001) for root length of wheat at both Depth 3 (33–48 cm) and Depth 4 (49–64 cm; Fig. 5c, d, Table S2). At Depth 3, root length of cv. Yitpi grown without supplemental N was 25% smaller under e[CO2] than a[CO2], but when N was applied, it was 62% greater under e[CO2] than a[CO2]. However, root length of cv. Wyalkatchem when grown without supplemental N was 32% greater under e[CO2] than a[CO2] but was similar for both CO2 treatments when grown with supplemental N. The trend of root length at Depth 4 was similar to that of Depth 3.
Supplementation with N stimulated total root length, with greater effect later in the season than at the early measurement dates (DAS × N, P = 0.033; Fig. 5e, Table S2). A four-way interaction DAS × CO2 × N × Cv was significant (P < 0.001) for the ratio of root length in the deeper (Depth 3 + 4) versus the upper (Depth 1 + 2) soil layers (Fig. 5f, Table S2). Elevated [CO2] increased this ratio for cv. Wyalkatchem when grown without supplemental N, but with supplemental N the ratio was greater under a[CO2] than e[CO2]. Yitpi showed an opposite trend with negligible difference between a[CO2] and e[CO2] treatments when grown without supplemental N, but with supplemental N, the ratio was greater under e[CO2] than a[CO2]. All those differences were more prominent later in the growing season.
Aboveground biomass at different growth stages
At DC31, e[CO2] stimulated aboveground biomass by 28% when wheat was grown with supplemental N, whereas this effect was negligible (<5%) when N was not applied (CO2 × N, P = 0.073; Fig. 6a). At the same growth stage, supplemental N stimulated the aboveground biomass of cv. Yitpi to a greater extent than cv. Wyalkatchem (58% vs 32%, N × Cv, P = 0.096). At DC65, aboveground biomass showed a significant three-way interaction (CO2 × N × Cv, P = 0.030; Fig. 6b). Elevated [CO2] stimulated the aboveground biomass of Yitpi by 28% when grown with supplemental N but decreased it by 9% when N was not applied. At the same growth stage, e[CO2] stimulated the aboveground biomass of Wyalkatchem to a similar extent for both N rates. At DC90, e[CO2] and supplemental N stimulated aboveground biomass by 11% (P = 0.03) and 15% (P = 0.002), respectively (Table 2, Fig. 6c). Yitpi had 12% greater (P = 0.01) aboveground biomass than Wyalkatchem.
Aboveground biomass at (a) DC31, (b) DC65, and (c) DC90 of wheat cvv. Yitpi and Wyalkatchem grown under ambient [CO2] (a[CO2], ~400 μmol mol−1) or elevated [CO2] (e[CO2], ~550 μmol mol−1) with (100 kg N ha−1) or without (0 kg N ha−1) supplemental N. Data are means and standard errors of n = 6 and 4 replicates for a[CO2] and e[CO2], respectively. Only significant (P < 0.1) P-values of the mixed effects model with main effects CO2, N rate and cultivar (Cv) as well as their interactions are presented.
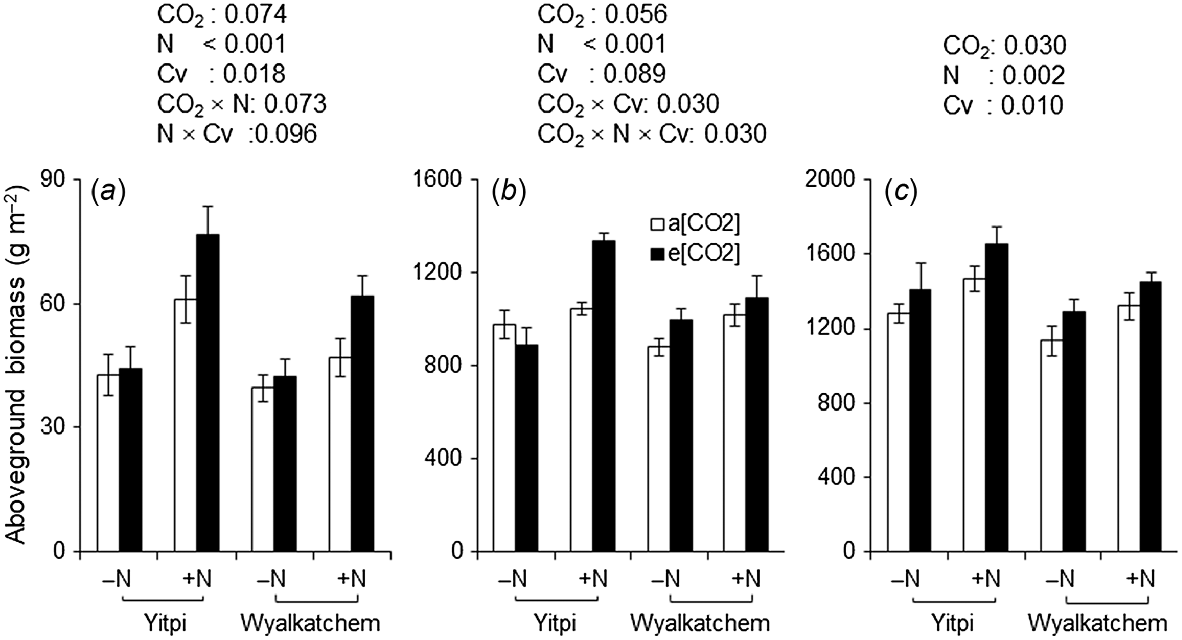
Grain yield, 1000-grain weight and harvest index
At harvest (DC90), e[CO2] increased the plant height of cv. Wyalkatchem by 4%, whereas it reduced that of cv. ‘Yitpi’ by 3% (CO2 × Cv, P = 0.011; Table 2). Supplemental N stimulated the grain yield of Yitpi by 14% while decreasing that of Wyalkatchem by 7% (N × Cv, P = 0.063; Table 2). Supplemental N decreased the HI of Wyalkatchem by 19% but did not affect that of Yitpi (N × Cv, P = 0.002). Elevated [CO2] stimulated 1000-grain weight of Yitpi grown with supplemental N (9%) but had no effect when N was not applied. For Wyalkatchem, e[CO2] decreased 1000-grain weight by 5% when grown with supplemental N and increased it by 3% when grown without supplemental N (CO2 × N × Cv, P = 0.098; Table 2).
Water use at different growth stages and water use efficiency
During early growth stages (i.e. sowing until DC31), e[CO2] resulted in 45% higher water use than a[CO2] (P = 0.009; Fig. 7a). However, during this stage, e[CO2]-induced stimulation of water use was greater for cv. Yitpi than cv. Wyalkatchem (74% vs 21%, CO2 × Cv; P = 0.073). From DC65 to DC90, Yitpi used 34% more water than Wyalkatchem (P = 0.029; Fig. 7c). Cumulative water use of Yitpi was 6% higher than that of Wyalkatchem (P = 0.079; Fig. 7d). Wheat grown with supplemental N had 5% higher cumulative water use than wheat grown without supplemental N (P = 0.099). Supplemental N increased the WUEb by 10% (P = 0.059), and Wyalkatchem had 17% lower WUEy than Yitpi (P = 0.008; Table 2).
Water use (a) from 21 DAS to DC31, (b) from DC31 to DC65, (c) from DC65 to DC90, and (d) cumulative through all growth stages of wheat cvv. Yitpi and Wyalkatchem grown under ambient [CO2] (a[CO2], ~400 μmol mol−1) or elevated [CO2] (e[CO2], ~550 μmol mol−1) with (100 kg N ha−1) or without (0 kg N ha−1) supplemental N. Data are means and standard errors of n = 6 and 4 replicates for a[CO2] and e[CO2], respectively. Only significant (P < 0.1) P-values of the mixed effects model with main effects CO2, N rate and cultivar (Cv) as well as their interactions are presented.
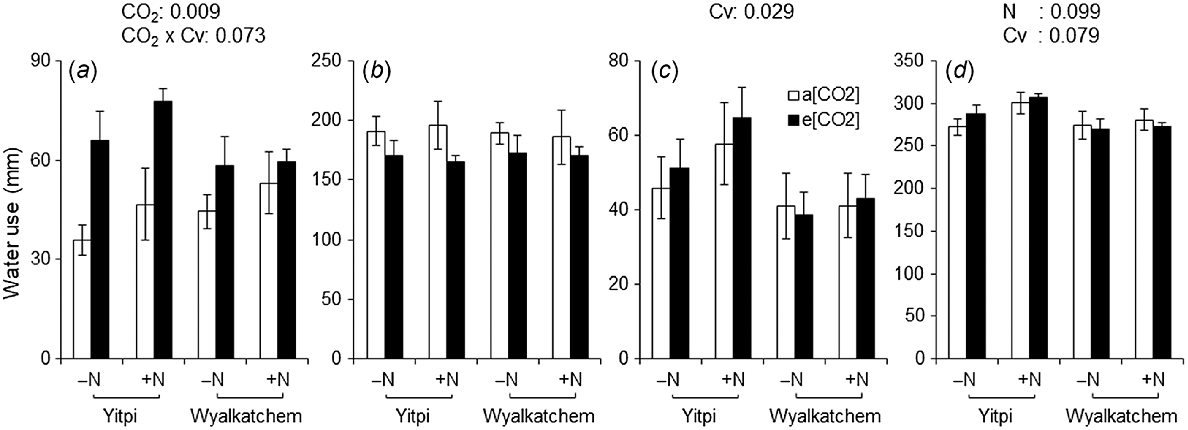
Discussion
Increased early biomass and leaf area under e[CO2] led to increased water use, offsetting leaf level intrinsic water use efficiency
Plants grown under e[CO2] conditions commonly exhibit a reduction in gs (Ainsworth and Long 2005). Our study quantified a 25% decrease in gs under e[CO2], which, in combination with a 12% increase in Anet, resulted in a 40% enhancement in iWUE compared with a[CO2] conditions. Although this indicates an improvement in leaf-level WUE, it is crucial to consider the implications at larger scales such as the plant, field or regional level. The well-known CO2 fertilisation effect stimulates plant biomass, including leaf area, which can counteract the water savings achieved at the leaf level (Ainsworth and Long 2005; Bunce 2016). Previous studies (Wu et al. 2004; Ukkola et al. 2016) support this notion. Our investigation, specifically during the early growth stage (from sowing until DC31), revealed that the increased biomass and leaf area offset the gains in leaf-level WUE, resulting in higher water use under e[CO2] than a[CO2]. Consequently, greater canopy transpiration likely contributed to soil water depletion, aligning with recent studies conducted in both controlled environment (Uddin et al. 2018d) and field (Gray et al. 2016) conditions. The elevated water use during the early growth stages under e[CO2] may subsequently lead to reduced water availability during critical stages (i.e. flowering and grain filling). This, combined with terminal drought events during low-rainfall years, can result in the phenomenon of haying-off, significantly impacting grain yield (van Herwaarden et al. 1998; Nuttall et al. 2012). To ensure optimal soil-water availability under e[CO2] for grain filling (and to capitalise on the CO2 fertilisation effect), multiple adaptations are likely necessary. These adaptations may involve advancements in genetics for enhanced transpiration efficiency (Christy et al. 2018), early-season vigour to facilitate rapid soil coverage (Bourgault et al. 2013), and strategic soil-water management practices to minimise soil evaporation (Lascano and Baumhardt 1996).
Differences in water use between a[CO2] and e[CO2] conditions during the early growth stages were no longer evident in the later growth stages, resulting in similar cumulative water use. The study area is characterised by low and unpredictable rainfall during the grain-filling period, which coupled with high evaporative demand (Fig. 1) driven by elevated temperatures and strong winds can mean that all available soil water is transpired (Sadras and Rodriguez 2007; Uddin et al. 2018a). The greater canopy cover under e[CO2] during anthesis may have mitigated the impact of evaporative water loss, leading to lower water use (although not statistically significant, P = 0.138). Despite the study period experiencing above-average rainfall primarily driven by a single large event (DAS 100–105), there were only a few small rainfall events (<5 mm) accompanied by a steady increase in reference evapotranspiration from anthesis onward, which likely masked any minor differences in water use between a[CO2] and e[CO2].
Although the increase in water use due to supplemental N was marginal during each growth stage, it led to greater cumulative water use. This observation can be primarily attributed to the significant increase in various growth parameters, including green leaf area, biomass and canopy cover, resulting from N supplementation. Of the two cultivars tested, Yitpi consistently outperformed Wyalkatchem in these growth parameters, ultimately exhibiting higher cumulative water use. Notably, during the grain-filling period, Yitpi exhibited higher water use than Wyalkatchem (Fig. 6c). Water use during this critical stage has a higher conversion efficiency into grain production (Kirkegaard et al. 2007; Wasson et al. 2012), which likely contributed to the grain yield and WUEy advantage of Yitpi over Wyalkatchem.
Stimulation of root length under e[CO2] increased crop water use through improved soil-water acquisition
The stimulation of root length observed under e[CO2] conditions in our study is consistent with earlier findings from an adjacent site with high background soil N (Bahrami et al. 2017; Uddin et al. 2018a). This consistency is further supported by reports from chamber studies (Chaudhuri et al. 1990; Benlloch-Gonzalez et al. 2014a) and other FACE experiments (Pacholski et al. 2015; Gray et al. 2016). Averaged across all measurement dates and treatments, the stimulation of root length under e[CO2] was greater (22%) than the stimulation of aboveground biomass (11%) and grain yield, which aligns with trends reported in previous studies investigating CO2 enrichment effects (Kimball et al. 2002; Benlloch-Gonzalez et al. 2014b).
The response of root growth to e[CO2] can be influenced by various factors including cultivar differences (Benlloch-Gonzalez et al. 2014a; Uddin et al. 2018a), growing conditions such as N and water availability (Stulen and den Hertog 1993; Wechsung et al. 1999; Burkart et al. 2004), and crop growth stage (Wechsung et al. 1999; Weigel and Manderscheid 2012). In the present study, the effect of supplemental N on the response of root length to e[CO2] was dependent on the cultivar and growth stage. The pattern observed in root length (at soil Depths 3 and 4) of both cultivars in response to either supplemental N or e[CO2] aligned with their respective biomass and grain yield responses. The root length in Depths 3 and 4, total root length, and ratio of root length in the deeper (Depths 3 and 4) versus the upper layers (Depths 1 and 2) were affected by complex interactions among all treatment variables. Yitpi exhibited greater stimulation of root growth with supplemental N, whereas Wyalkatchem showed the opposite trend, with greater e[CO2]-induced stimulation of root growth, particularly in the lower soil profile, when grown without supplemental N. However, there was no interaction between N rate and [CO2] in terms of grain yield, although supplemental N had a greater impact on increasing the grain yield of Yitpi than of Wyalkatchem. The stimulation of root growth by e[CO2] and/or supplemental N may be attributed to indirect effects related to increased total plant biomass and adaptive mechanisms for improved water and nutrient acquisition (Pacholski et al. 2015). Furthermore, the stimulation of root growth under e[CO2] may be necessary to sustain the increase in biomass and grain yield (Contador et al. 2015).
Elevated [CO2] stimulated the growth of roots at all measured soil depths, indicating a potential impact on the spatial pattern of soil-water and nutrient uptake within the soil profile (Fig. S2). The greater stimulation of root growth near the soil surface can be advantageous for dryland crops, because it enables them to utilise surface water that becomes temporarily available following small rainfall events (Sadras and Rodriguez 2007). Simultaneously, the stimulation of root length under e[CO2] may allow extraction of water from deeper soil layers during the critical grain-filling periods, which is often associated with terminal drought in dryland Mediterranean agroecosystems (Passioura 1983). The uptake of subsoil water during grain filling has the potential to make a substantial contribution to the grain yield of dryland crops (Angus and van Herwaarden 2001; Kirkegaard et al. 2007).
A study conducted in the AGFACE facility, utilising similar CO2 exposure treatments to those used in our study but with contrasting irrigation regimes on a soil with high background N levels, reported a positive correlation between seasonal water use and root length in deeper soil layers (Uddin et al. 2018a). Stimulation of root growth in the deeper soil layers plays a significant role in maintaining plant physiological processes (Gallardo et al. 1994; Uddin et al. 2018b) because these roots are younger and more efficient in extracting and supplying water than roots in the upper soil layers (Gregory et al. 1978). The higher water use under e[CO2] during the early growth stages suggests that root growth stimulation improves water acquisition. However, these early-stage responses were subsequently overshadowed by the prevailing dryland condition at the study site as the growing season progressed (Uddin et al. 2018a). Although early depletion of soil water can have negative effects on grain yield, particularly during seasons with dry finishes, the small variation in cumulative water use observed in this study would have a negligible impact on stored soil moisture for the following season.
The effect of supplemental N on grain yield was cultivar-specific
Elevated CO2 consistently stimulated aboveground biomass throughout the growing season, and significant interactions were observed among [CO2], N rate and cultivar treatments during the early growth stages, with positive interactions observed between e[CO2] and supplemental N. At maturity, e[CO2] resulted in similar stimulation of both aboveground biomass and grain yield, leading to an insignificant HI response to [CO2]. The lack of significance regarding the effect of [CO2] on grain yield (P = 0.108) may be attributed to the smaller sample size and larger variability of the yield data in this study. Previous FACE studies, including those conducted over multiple seasons and with larger sample sizes, have reported e[CO2]-induced stimulation of biomass and grain yield within the AGFACE facility (Fitzgerald et al. 2016; Tausz et al. 2017a; Fitzgerald et al. 2022), as well as in other locations worldwide (Weigel and Manderscheid 2012; Ziska et al. 2012; Kimball 2016; Ainsworth and Long 2021). Similarly, no significant interaction was observed between [CO2] and N rate or cultivar for grain yield, which aligns with findings from earlier FACE studies (Weigel and Manderscheid 2012; Bourgault et al. 2016; Fitzgerald et al. 2016; Tausz et al. 2017a, 2017b).
Previous research examining the interactive effect of e[CO2] and N rates has primarily focused on high-yielding systems, such as those with high rainfall or continuous irrigation, where grain yields exceed 5 t ha−1 and N rates >250 kg ha−1 stimulate the CO2 fertilisation effect (Kimball et al. 2001; Manderscheid et al. 2009). In a low-yielding, water-limited environment with high background soil N (~280 kg NO3−-N ha−1), the addition of supplemental N (50–60 kg N ha−1) has been found to have no significant impact on biomass and grain yield, and it does not alter the CO2 fertilisation effect (Tausz et al. 2017b). By contrast, in a similar environment with low background soil N, a significant positive interaction between supplemental N and e[CO2] was observed affecting aboveground biomass and grain yield in wheat (Walker et al. 2017). In the present study, the addition of supplemental N influenced grain yield, with variations depending on the cultivar studied, but not CO2 concentration.
Despite the initial expectation that cv. Wyalkatchem would outperform cv. Yitpi owing to its reputed greater N use efficiency (Alhabbar et al. 2018), the results of this study showed the opposite trend. Yitpi performed better than Wyalkatchem across various growth parameters and in grain yield, particularly when supplemental N was applied. One of the mechanisms underpinning the stimulation of grain yield by supplemental N in soil environments with low background N is the maintenance of higher Anet for an extended period (Ziska et al. 1996). During grain filling, wheat plants supplemented with N retained greater leaf N and chlorophyll contents, indicating greater photosynthetic capacity (Cannella et al. 2016). In this study, supplemental N maintained greater post-anthesis leaf N and chlorophyll content for cv. Yitpi under a low soil-N system. The combination of greater canopy cover (or green leaf area) and higher Anet in cv. Yitpi likely improved the supply of assimilates to developing grains. Late-season photosynthesis plays a crucial role in increasing grain yield under water-limited conditions (Liang et al. 2018), because most vegetative growth is completed and new photosynthates are primarily allocated to the growth and development of larger grains. This ultimately resulted in a greater yield benefit for cv. Yitpi in our study.
By contrast, application of supplemental N accelerated the post-anthesis senescence of cv. Wyalkatchem, resulting in a shorter critical grain-filling period. In dryland conditions, the remobilisation of stem water-soluble carbohydrate plays a significant role in wheat grain yield (Davidson and Chevalier 1992; van Herwaarden et al. 2003). In our study, Wyalkatchem showed early flowering and fast senescence compared with Yitpi, which is consistent with the findings of Nguyen et al. (2016). This early senescence adversely affects both the assimilate supply and complete remobilisation of stem water-soluble carbohydrate to the developing grains, leading to a substantial decrease in grain size and yield, and ultimately resulting in lower HI. The lack of a grain yield response to supplemental N in a cultivar with reputed high N use efficiency suggests that N use efficiency and responsiveness may need to be considered as separate factors (Ulas et al. 2013; Mahjourimajd et al. 2016; Ivić et al. 2021; Pujarula et al. 2021).
Conclusions
Our study demonstrates that early stimulation of biomass and leaf growth under e[CO2] can offset the effect of increased leaf-level WUE on water use in the short term, resulting in higher crop-level water use under e[CO2] than under a[CO2]. Furthermore, higher water use under e[CO2] during the early growth stages also indicates improved water acquisition through root growth stimulation. However, by the end of the season, the accumulated water use was similar under both a[CO2] and e[CO2], pointing to the dominating effect of the seasonal conditions. We observed genotypic variability in response to e[CO2] and supplemental N for many growth and physiological parameters. Although supplemental N stimulated the grain yield of cv. Yitpi to a greater extent than cv. Wyalkatchem, there was no interaction with [CO2]. Supplemental N resulted in greater post-anthesis leaf N, chlorophyll content and canopy cover for Yitpi, which in conjunction with the greater net assimilation rates would have improved assimilate supply to developing grains and thereby resulted in greater yield stimulation for this cultivar than for cv. Wyalkatchem. Our results did not show significant interactions between [CO2] and cultivar for grain yield, indicating that for future breeding N efficiency traits and responsiveness need to be considered independently to maximise the benefit from the CO2 fertilisation effect.
Data availability
The data supporting the findings of this study will be available on reasonable request from the corresponding author.
Declaration of funding
Research at the Australian Grains Free-Air CO2 Enrichment (AGFACE) project was jointly run by the University of Melbourne and Agriculture Victoria Research (Department of Energy, Environment and Climate Action) with substantial funding from The University of Melbourne, Agriculture Victoria, Grains Research and Development Corporation, the Australian Department of Agriculture and Water Resources, and the Australian Research Council. SU was supported by a Melbourne International Research Scholarship.
Author contributions
SU, GJF, ML, STP, RA, MT designed the study. SU and SP collected data. SU and ML analysed the data, and all authors contributed to interpretation of the results. SU wrote the first draft and all authors reviewed and edited it. All authors gave their final approval for publication.
Acknowledgements
The authors gratefully acknowledge the contributions of the AGFACE field team lead by Russel Argall, Mel Munn and Roger Perris (Agriculture Victoria) for agronomic management of the experiment and collecting soil-water and CropCircle data. Samuel Martín Sanchez (Technical University of Madrid) helped with root scanning and sample processing during his internship at the University of Melbourne Creswick Campus, and Chinthaka Jayasinghe (University of Melbourne) supported field work. Special thanks to Dr Helale Bahrami (University of Melbourne) for tracing root images and Dr Mahabubur Mollah (Agriculture Victoria) for operating and maintaining the CO2 enrichment technology. Bernie Dominiak reviewed an earlier version of the manuscript. We acknowledge the significant contribution of the three anonymous reviewers through their constructive feedback.
References
Ainsworth EA, Long SP (2005) What have we learned from 15 years of free-air CO2 enrichment (FACE)? A meta-analytic review of the responses of photosynthesis, canopy properties and plant production to rising CO2. New Phytologist 165, 351-372.
| Crossref | Google Scholar | PubMed |
Ainsworth EA, Long SP (2021) 30 years of free-air carbon dioxide enrichment (FACE): what have we learned about future crop productivity and its potential for adaptation? Global Change Biology 27, 27-49.
| Crossref | Google Scholar | PubMed |
Ainsworth EA, Rogers A (2007) The response of photosynthesis and stomatal conductance to rising CO2: mechanisms and environmental interactions. Plant Cell and Environment 30, 258-270.
| Crossref | Google Scholar |
Alhabbar Z, Yang R, Juhasz A, Xin H, She M, Anwar M, Sultana N, Diepeveen D, Ma W, Islam S (2018) NAM gene allelic composition and its relation to grain-filling duration and nitrogen utilisation efficiency of Australian wheat. PLoS ONE 13, e0205448.
| Crossref | Google Scholar | PubMed |
Andersson P, Majdi H (2005) Estimating root longevity at sites with long periods of low root mortality. Plant and Soil 276, 9-14.
| Crossref | Google Scholar |
Angus JF (2001) Nitrogen supply and demand in Australian agriculture. Australian Journal of Experimental Agriculture 41, 277-288.
| Crossref | Google Scholar |
Angus JF, van Herwaarden AF (2001) Increasing water use and water use efficiency in dryland wheat. Agronomy Journal 93, 290-298.
| Crossref | Google Scholar |
Bahrami H, De Kok LJ, Armstrong R, Fitzgerald GJ, Bourgault M, Henty S, Tausz M, Tausz-Posch S (2017) The proportion of nitrate in leaf nitrogen, but not changes in root growth, are associated with decreased grain protein in wheat under elevated [CO2]. Journal of Plant Physiology 216, 44-51.
| Crossref | Google Scholar | PubMed |
Benlloch-Gonzalez M, Berger J, Bramley H, Rebetzke G, Palta JA (2014a) The plasticity of the growth and proliferation of wheat root system under elevated CO2. Plant and Soil 374, 963-976.
| Crossref | Google Scholar |
Benlloch-Gonzalez M, Bochicchio R, Berger J, Bramley H, Palta JA (2014b) High temperature reduces the positive effect of elevated CO2 on wheat root system growth. Field Crops Research 165, 71-79.
| Crossref | Google Scholar |
Bishop KA, Betzelberger AM, Long SP, Ainsworth EA (2015) Is there potential to adapt soybean (Glycine max Merr.) to future CO2? An analysis of the yield response of 18 genotypes in free-air CO2 enrichment. Plant Cell and Environment 38, 1765-1774.
| Crossref | Google Scholar |
Bourgault M, Dreccer MF, James AT, Chapman SC (2013) Genotypic variability in the response to elevated CO2 of wheat lines differing in adaptive traits. Functional Plant Biology 40, 172-184.
| Crossref | Google Scholar | PubMed |
Bourgault M, Brand J, Tausz M, Fitzgerald GJ (2016) Yield, growth and grain nitrogen response to elevated CO2 of five field pea (Pisum sativum L.) cultivars in a low rainfall environment. Field Crops Research 196, 1-9.
| Crossref | Google Scholar |
Bunce JA (2016) Responses of soybeans and wheat to elevated CO2 in free-air and open top chamber systems. Field Crops Research 186, 78-85.
| Crossref | Google Scholar |
Bureau of Meteorology (2017) Climate Data Online database (Horsham Polkemmet Rd Victoria). Available at http://www.bom.gov.au/climate/data/ [Accessed 26 August 2017]
Burkart S, Manderscheid R, Weigel H-J (2004) Interactive effects of elevated atmospheric CO2 concentrations and plant available soil water content on canopy evapotranspiration and conductance of spring wheat. European Journal of Agronomy 21, 401-417.
| Crossref | Google Scholar |
Burkart S, Manderscheid R, Wittich KP, Loepmeier FJ, Weigel HJ (2011) Elevated CO2 effects on canopy and soil water flux parameters measured using a large chamber in crops grown with free-air CO2 enrichment. Plant Biology 13, 258-269.
| Crossref | Google Scholar | PubMed |
Cannella D, Möllers KB, Frigaard NU, Jensen PE, Bjerrum MJ, Johansen KS, Felby C (2016) Light-driven oxidation of polysaccharides by photosynthetic pigments and a metalloenzyme. Nature Communications 7, 11134.
| Crossref | Google Scholar |
Chaudhuri UN, Kirkham MB, Kanemasu ET (1990) Root growth of winter wheat under elevated carbon dioxide and drought. Crop Science 30, 853-857.
| Crossref | Google Scholar |
Christy B, Tausz-Posch S, Tausz M, Richards R, Rebetzke G, Condon A, McLean T, Fitzgerald G, Bourgault M, O’Leary G (2018) Benefits of increasing transpiration efficiency in wheat under elevated CO2 for rainfed regions. Global Change Biology 24, 1965-1977.
| Crossref | Google Scholar | PubMed |
Contador ML, Comas LH, Metcalf SG, Stewart WL, Porris Gomez I, Negron C, Lampinen BD (2015) Root growth dynamics linked to above-ground growth in walnut (Juglans regia). Annals of Botany 116, 49-60.
| Crossref | Google Scholar | PubMed |
Davidson DJ, Chevalier PM (1992) Storage and remobilization of water-soluble carbohydrates in stems of spring wheat. Crop Science 32, 186-190.
| Crossref | Google Scholar |
Deryng D, Elliott J, Folberth C, Müller C, Pugh TAM, Boote KJ, Conway D, Ruane AC, Gerten D, Jones JW, Khabarov N, Olin S, Schapho S, Schmid E, Yang H, Rosenzweig C (2016) Regional disparities in the beneficial effects of rising CO2 concentrations on crop water productivity. Nature Climate Change 6, 786-790.
| Crossref | Google Scholar |
Fitzgerald GJ, Tausz M, O’Leary G, Mollah MR, Tausz-Posch S, Seneweera S, Mock I, Löw M, Partington DL, McNeil D, Norton RM (2016) Elevated atmospheric [CO2] can dramatically increase wheat yields in semi-arid environments and buffer against heat waves. Global Change Biology 22, 2269-2284.
| Crossref | Google Scholar | PubMed |
Fitzgerald GJ, Tausz M, Armstrong R, Panozzo J, Trębicki P, Mollah M, Tausz-Posch S, Walker C, Nuttall JG, Bourgault M, Löw M, Partington D, Butterly CR, Lam SK, Norton RM, O’Leary GJ (2022) Elevated CO2 in semi-arid cropping systems: a synthesis of research from the Australian Grains Free Air CO2 Enrichment (AGFACE) research program. In ‘Advances in Agronomy.’ Vol. 171. Ch. 1. (Ed. DL Sparks) pp. 1–73. (Academic Press: Cambridge, MA, USA)
Gallardo M, Turner NC, Ludwig C (1994) Water relations, gas exchange and abscisic acid content of Lupinus cosentinii leaves in response to drying different proportions of the root system. Journal of Experimental Botany 45, 909-918.
| Crossref | Google Scholar |
Garcia RL, Long SP, Wall GW, Osborne CP, Kimball BA, Nie GY, Pinter PJ, Lamorte RL, Wechsung F (1998) Photosynthesis and conductance of spring-wheat leaves: field response to continuous free-air atmospheric CO2 enrichment. Plant, Cell and Environment 21, 659-669.
| Crossref | Google Scholar |
Gray SB, Dermody O, Klein SP, Locke AM, McGrath JM, Paul RE, Rosenthal DM, Ruiz-Vera UM, Siebers MH, Strellner R, Ainsworth EA, Bernacchi CJ, Long SP, Ort DR, Leakey ADB (2016) Intensifying drought eliminates the expected benefits of elevated carbon dioxide for soybean. Nature Plants 2, 16132.
| Crossref | Google Scholar | PubMed |
Gregory PJ, McGowan M, Biscoe PV (1978) Water relations of winter wheat: 2. Soil water relations. The Journal of Agricultural Science 91, 103-116.
| Crossref | Google Scholar |
Hatfield JL, Boote KJ, Kimball BA, Ziska LH, Izaurralde RC, Ort D, Thomson AM, Wolfe D (2011) Climate impacts on agriculture: implications for crop production. Agronomy Journal 103, 351-370.
| Crossref | Google Scholar |
Houshmandfar A, Fitzgerald GJ, Armstrong R, Macabuhay AA, Tausz M (2015) Modelling stomatal conductance of wheat: an assessment of response relationships under elevated CO2. Agricultural and Forest Meteorology 214–215, 117-123.
| Crossref | Google Scholar |
Houshmandfar A, Fitzgerald GJ, Macabuhay AA, Armstrong R, Tausz-Posch S, Low M, Tausz M (2016) Trade-offs between water-use related traits, yield components and mineral nutrition of wheat under Free-Air CO2 Enrichment (FACE). European Journal of Agronomy 76, 66-74.
| Crossref | Google Scholar |
Hunsaker DJ, Kimball BA, Pinter PJ, Wall GW, LaMorte RL, Adamsen FJ, Leavitt SW, Thompson TL, Matthias AD, Brooks TJ (2000) CO2 enrichment and soil nitrogen effects on wheat evapotranspiration and water use efficiency. Agricultural and Forest Meteorology 104, 85-105.
| Crossref | Google Scholar |
Hussain MZ, Vanloocke A, Siebers MH, Ruiz-Vera UM, Markelz RJC, Leakey ADB, Ort DR, Bernacchi CJ (2013) Future carbon dioxide concentration decreases canopy evapotranspiration and soil water depletion by field-grown maize. Global Change Biology 19, 1572-1584.
| Crossref | Google Scholar | PubMed |
Hutchinson MF, McIntyre S, Hobbs RJ, Stein JL, Garnett S, Kinloch J (2005) Integrating a global agro-climatic classification with bioregional boundaries in Australia. Global Ecology and Biogeography 14, 197-212.
| Crossref | Google Scholar |
Intergrain (2001) Wyalkatchem. Intergrain, Perth, WA, Australia. Available at http://www.intergrain.com/WheatDetail.aspx?VarietyId=18 [Accessed 9 January 2018]
IPCC (2013) Technical summary. In ‘Climate Change 2013: The physical science basis. Contribution of Working Group I to the Fifth Assessment Report of the Intergovernmental Panel on Climate Change’. (Eds TF Stocker, D Qin, G-K Plattner, M Tignor, SK Allen, J Boschung, A Nauels, Y Xia, V Bex, PM Midgley) pp. 33–115. (Cambridge University Press: Cambridge, UK, and New York, NY, USA)
Ivić M, Grljušić S, Plavšin I, Dvojković K, Lovrić A, Rajković B, Maričević M, Černe M, Popović B, Lončarić Z, Bentley AR, Swarbreck SM, Šarčević H, Novoselović D (2021) Variation for nitrogen use efficiency traits in wheat under contrasting nitrogen treatments in South-Eastern Europe. Frontiers in Plant Science 12, 682333.
| Crossref | Google Scholar |
Jin ZN, Ainsworth EA, Leakey ADB, Lobell DB (2018) Increasing drought and diminishing benefits of elevated carbon dioxide for soybean yields across the US Midwest. Global Change Biology 24, E522-E533.
| Crossref | Google Scholar | PubMed |
Kimball BA (2016) Crop responses to elevated CO2 and interactions with H2O, N, and temperature. Current Opinion in Plant Biology 31, 36-43.
| Crossref | Google Scholar | PubMed |
Kimball BA, Morris CF, Pinter PJ, Wall GW, Hunsaker DJ, Adamsen FJ, LaMorte RL, Leavitt SW, Thompson TL, Matthias AD, Brooks TJ (2001) Elevated CO2, drought and soil nitrogen effects on wheat grain quality. New Phytologist 150, 295-303.
| Crossref | Google Scholar |
Kirkegaard JA, Lilley JM, Howe GN, Graham JM (2007) Impact of subsoil water use on wheat yield. Australian Journal of Agricultural Research 58, 303-315.
| Crossref | Google Scholar |
Lascano RJ, Baumhardt RL (1996) Effects of crop residue on soil and plant water evaporation in a dryland cotton system. Theoretical and Applied Climatology 54, 69-84.
| Crossref | Google Scholar |
Leakey ADB, Ainsworth EA, Bernacchi CJ, Rogers A, Long SP, Ort DR (2009) Elevated CO2 effects on plant carbon, nitrogen, and water relations: six important lessons from FACE. Journal of Experimental Botany 60, 2859-2876.
| Crossref | Google Scholar | PubMed |
Lenka NK, Lenka S, Yashona DS, Jat D (2021) Elevated temperature and low nitrogen partially offset the yield, evapotranspiration, and water use efficiency of winter wheat under carbon dioxide enrichment. Agricultural Water Management 250, 106856.
| Crossref | Google Scholar |
Li Z, Yagi K, Sakai H, Kobayashi K (2004) Influence of elevated CO2 and nitrogen nutrition on rice plant growth, soil microbial biomass, dissolved organic carbon and dissolved CH4. Plant and Soil 258, 81-90.
| Crossref | Google Scholar |
Liang X, Liu Y, Chen J, Adams C (2018) Late-season photosynthetic rate and senescence were associated with grain yield in winter wheat of diverse origins. Journal of Agronomy and Crop Science 204, 1-12.
| Crossref | Google Scholar |
Madhu M, Hatfield JL (2013) Dynamics of plant root growth under increased atmospheric carbon dioxide. Agronomy Journal 105, 657-669.
| Crossref | Google Scholar |
Mahjourimajd S, Kuchel H, Langridge P, Okamoto M (2016) Evaluation of Australian wheat genotypes for response to variable nitrogen application. Plant and Soil 399, 247-255.
| Crossref | Google Scholar |
Malik AI, Veres S, Rengel Z (2016) Differential nitrogen-use efficiency in wheat parents of doubled-haploid mapping populations. Plant and Soil 408, 311-325.
| Crossref | Google Scholar |
Manderscheid R, Weigel H-J (2007) Drought stress effects on wheat are mitigated by atmospheric CO2 enrichment. Agronomy for Sustainable Development 27, 79-87.
| Crossref | Google Scholar |
Manderscheid R, Pacholski A, Fruehauf C, Weigel H-J (2009) Effects of free air carbon dioxide enrichment and nitrogen supply on growth and yield of winter barley cultivated in a crop rotation. Field Crops Research 110, 185-196.
| Crossref | Google Scholar |
Manderscheid R, Dier M, Erbs M, Sickora J, Weigel H-J (2018) Nitrogen supply – a determinant in water use efficiency of winter wheat grown under free air CO2 enrichment. Agricultural Water Management 210, 70-77.
| Crossref | Google Scholar |
Mollah M, Norton R, Huzzey J (2009) Australian grains free-air carbon dioxide enrichment (AGFACE) facility: design and performance. Crop & Pasture Science 60, 697-707.
| Crossref | Google Scholar |
Mollah M, Partington D, Fitzgerald G (2011) Understand distribution of carbon dioxide to interpret crop growth data: Australian grains free-air carbon dioxide enrichment experiment. Crop & Pasture Science 62, 883-891.
| Crossref | Google Scholar |
Nguyen GN, Panozzo J, Spangenberg G, Kant S (2016) Phenotyping approaches to evaluate nitrogen-use efficiency related traits of diverse wheat varieties under field conditions. Crop & Pasture Science 67, 1139-1148.
| Crossref | Google Scholar |
NOAA (2022) Trends in atmospheric carbon dioxide. National Oceanic and Atmospheric Administration. Available at https://www.esrl.noaa.gov/gmd/ccgg/trends/monthly.html [Accessed 15 June 2022]
Nuttall JG, O’Leary GJ, Khimashia N, Asseng S, Fitzgerald G, Norton R (2012) ‘Haying-off’ in wheat is predicted to increase under a future climate in south-eastern Australia. Crop & Pasture Science 63, 593-605.
| Crossref | Google Scholar |
O’Leary GJ, Christy B, Nuttall J, Huth N, Cammarano D, Stöckle C, Basso B, Shcherbak I, Fitzgerald G, Luo Q, Farre-Codina I, Palta J, Asseng S (2015) Response of wheat growth, grain yield and water use to elevated CO2 under a free-air CO2 enrichment (FACE) experiment and modelling in a semi-arid environment. Global Change Biology 21, 2670-2686.
| Crossref | Google Scholar | PubMed |
Pacholski A, Manderscheid R, Weigel H-J (2015) Effects of free air CO2 enrichment on root growth of barley, sugar beet and wheat grown in a rotation under different nitrogen supply. European Journal of Agronomy 63, 36-46.
| Crossref | Google Scholar |
Parvin S, Uddin S, Bourgault M, Roessner U, Tausz-Posch S, Armstrong R, O’Leary G, Fitzgerald G, Tausz M (2018) Water availability moderates N2 fixation benefit from elevated CO2: a 2-year free-air CO2 enrichment study on lentil (Lens culinaris MEDIK.) in a water limited agroecosystem. Plant, Cell and Environment 41, 2418-2434.
| Crossref | Google Scholar |
Passioura JB (1983) Roots and drought resistance. Agricultural Water Management 7, 265-280.
| Crossref | Google Scholar |
Perry EM, Fitzgerald GJ, Poole N, Craig S, Whitlock A (2012) NDVI from active optical sensors as a measure of canopy cover and biomass. In ‘XXII Isprs Congress, Technical Commission VIII.’ Vol. 39-B8. (Eds M Shortis, H Shimoda, K Cho) pp. 317–319. (The International Archives of the Photogrammetry, Remote Sensing and Spatial Information Sciences: Hannover, Germany)
Pinheiro J, Bates D, DebRoy S, Sarkar D, Team RC (2022) nlme: nlme: Linear and nonlinear mixed effects models. R package version 3.1-155. R Foundation for Statistical Computing, Vienna, Austria. Available at https://CRAN.R-project.org/package=nlme
Pleijel H, Broberg MC, Högy P, Uddling J (2019) Nitrogen application is required to realize wheat yield stimulation by elevated CO2 but will not remove the CO2-induced reduction in grain protein concentration. Global Change Biology 25, 1868-1876.
| Crossref | Google Scholar | PubMed |
Pujarula V, Pusuluri M, Bollam S, Das RR, Ratnala R, Adapala G, Thuraga V, Rathore A, Srivastava RK, Gupta R (2021) Genetic variation for nitrogen use efficiency traits in global diversity panel and parents of mapping populations in pearl millet. Frontiers in Plant Science 12 625915.
| Crossref | Google Scholar |
R Core Team (2022) R: A language and environment for statistical computing. R Foundation for Statistical Computing, Vienna, Austria. Available at https://www.R-project.org/
Reich PB, Hobbie SE, Lee TD (2014) Plant growth enhancement by elevated CO2 eliminated by joint water and nitrogen limitation. Nature Geoscience 7, 920-924.
| Crossref | Google Scholar |
Sadras VO, Rodriguez D (2007) The limit to wheat water-use efficiency in eastern Australia. II. Influence of rainfall patterns. Australian Journal of Agricultural Research 58, 657-669.
| Crossref | Google Scholar |
Seednet (2005) Yitpi. Seednet, Horsham, Vic., Australia. Available at http://www.seednet.com.au/documents/YitpiFactsheet2005.pdf [Accessed 25 August 2017]
Stulen I, den Hertog J (1993) Root growth and functioning under atmospheric CO2 enrichment. Vegetatio 104, 99-115.
| Crossref | Google Scholar |
Tausz M, Tausz-Posch S, Norton RM, Fitzgerald GJ, Nicolas ME, Seneweera S (2013) Understanding crop physiology to select breeding targets and improve crop management under increasing atmospheric CO2 concentrations. Environmental and Experimental Botany 88, 71-80.
| Crossref | Google Scholar |
Tausz M, Bilela S, Bahrami H, Armstrong R, Fitzgerald G, O’Leary G, Simon J, Tausz-Posch S, Rennenberg H (2017a) Nitrogen nutrition and aspects of root growth and function of two wheat cultivars under elevated [CO2]. Environmental and Experimental Botany 140, 1-7.
| Crossref | Google Scholar |
Tausz M, Norton RM, Tausz-Posch S, Löw M, Seneweera S, O’Leary G, Armstrong R, Fitzgerald GJ (2017b) Can additional N fertiliser ameliorate the elevated CO2-induced depression in grain and tissue N concentrations of wheat on a high soil N background? Journal of Agronomy and Crop Science 203, 574-583.
| Crossref | Google Scholar |
Tausz-Posch S, Seneweera S, Norton RM, Fitzgerald GJ, Tausz M (2012) Can a wheat cultivar with high transpiration efficiency maintain its yield advantage over a near-isogenic cultivar under elevated CO2? Field Crops Research 133, 160-166.
| Crossref | Google Scholar |
Tausz-Posch S, Norton RM, Seneweera S, Fitzgerald GJ, Tausz M (2013) Will intra-specific differences in transpiration efficiency in wheat be maintained in a high CO2 world? A FACE study. Physiologia Plantarum 148, 232-245.
| Crossref | Google Scholar | PubMed |
Tausz-Posch S, Tausz M, Bourgault M (2020) Elevated [CO2] effects on crops: advances in understanding acclimation, nitrogen dynamics and interactions with drought and other organisms. Plant Biology 22, 38-51.
| Google Scholar | PubMed |
Turner NC (2004) Sustainable production of crops and pastures under drought in a Mediterranean environment. Annals of Applied Biology 144, 139-147.
| Crossref | Google Scholar |
Uddin S, Löw M, Parvin S, Fitzgerald G, Bahrami H, Tausz-Posch S, Armstrong R, O’Leary G, Tausz M (2018a) Water use and growth responses of dryland wheat grown under elevated CO2 are associated with root length in deeper, but not upper soil layer. Field Crops Research 224, 170-181.
| Crossref | Google Scholar |
Uddin S, Löw M, Parvin S, Fitzgerald GJ, Tausz-Posch S, Armstrong R, O’Leary G, Tausz M (2018b) Elevated [CO2] mitigates the effect of surface drought by stimulating root growth to access sub-soil water. PLoS ONE 13, e0198928.
| Crossref | Google Scholar |
Uddin S, Löw M, Parvin S, Fitzgerald GJ, Tausz-Posch S, Armstrong R, Tausz M (2018c) Yield of canola (Brassica napus L.) benefits more from elevated CO2 when access to deeper soil water is improved. Environmental and Experimental Botany 155, 518-528.
| Crossref | Google Scholar |
Uddin S, Parvin S, Löw M, Fitzgerald GJ, Tausz-Posch S, Armstrong R, Tausz M (2018d) The water use dynamics of canola cultivars grown under elevated CO2 are linked to their leaf area development. Journal of Plant Physiology 229, 164-169.
| Crossref | Google Scholar | PubMed |
Ukkola AM, Prentice IC, Keenan TF, van Dijk AIJM, Viney NR, Myneni RB, Bi J (2016) Reduced streamflow in water-stressed climates consistent with CO2 effects on vegetation. Nature Climate Change 6, 75-78.
| Crossref | Google Scholar |
Ulas A, Behrens T, Wiesler F, Horst WJ, Schulte auf’m Erley G (2013) Does genotypic variation in nitrogen remobilisation efficiency contribute to nitrogen efficiency of winter oilseed-rape cultivars (Brassica napus L.)? Plant and Soil 371, 463-471.
| Crossref | Google Scholar |
van der Kooi CJ, Reich M, Löw M, De Kok LJ, Tausz M (2016) Growth and yield stimulation under elevated CO2 and drought: a meta-analysis on crops. Environmental and Experimental Botany 122, 150-157.
| Crossref | Google Scholar |
van Herwaarden AF, Farquhar GD, Angus JF, Richards RA, Howe GN (1998) ‘Haying-off’, the negative grain yield response of dryland wheat to nitrogen fertiliser. I. Biomass, grain yield, and water use. Australian Journal of Agricultural Research 49, 1067-1081.
| Crossref | Google Scholar |
van Herwaarden A, Richards R, Angus JF (2003) Water-soluble carbohydrates and yield in wheat. In ‘Solutions for a better environment. Proceedings of the 11th Australian Agronomy Conference’ 2–6 February 2003, Geelong, Vic., Australia. (Eds M Unkovich, G O’Leary) pp. 1–4. (Australian Society of Agronomy Inc, VIDA: Horsham, Vic., Australia)
Walker C, Armstrong R, Panozzo J, Partington D, Fitzgerald G (2017) Can nitrogen fertiliser maintain wheat (Triticum aestivum) grain protein concentration in an elevated CO2 environment? Soil Research 55, 518-523.
| Crossref | Google Scholar |
Wall GW (2001) Elevated atmospheric CO2 alleviates drought stress in wheat. Agriculture, Ecosystems & Environment 87, 261-271.
| Crossref | Google Scholar |
Wall GW, Adam NR, Brooks TJ, Kimball BA, Pinter PJ, Jr., LaMorte RL, Adamsen FJ, Hunsaker DJ, Wechsung G, Wechsung F, Grossman-Clarke S, Leavitt SW, Matthias AD, Webber AN (2000) Acclimation response of spring wheat in a free-air CO2 enrichment (FACE) atmosphere with variable soil nitrogen regimes. 2. Net assimilation and stomatal conductance of leaves. Photosynthesis Research 66, 79-95.
| Crossref | Google Scholar | PubMed |
Wang L, Feng Z, Schjoerring JK (2013) Effects of elevated atmospheric CO2 on physiology and yield of wheat (Triticum aestivum L.): a meta-analytic test of current hypotheses. Agriculture, Ecosystems & Environment 178, 57-63.
| Crossref | Google Scholar |
Wasson AP, Richards RA, Chatrath R, Misra SC, Prasad SVS, Rebetzke GJ, Kirkegaard JA, Christopher J, Watt M (2012) Traits and selection strategies to improve root systems and water uptake in water-limited wheat crops. Journal of Experimental Botany 63, 3485-3498.
| Crossref | Google Scholar | PubMed |
Wechsung G, Wechsung F, Wall GW, Adamsen FJ, Kimball BA, Pinter PJ, Jr., Lamorte RL, Garcia RL, Kartschall T (1999) The effects of free-air CO2 enrichment and soil water availability on spatial and seasonal patterns of wheat root growth. Global Change Biology 5, 519-529.
| Crossref | Google Scholar |
Weigel H-J, Manderscheid R (2012) Crop growth responses to free air CO2 enrichment and nitrogen fertilization: rotating barley, ryegrass, sugar beet and wheat. European Journal of Agronomy 43, 97-107.
| Crossref | Google Scholar |
Whalley WR, Cope RE, Nicholl CJ, Whitmore AP (2004) In-field calibration of a dielectric soil moisture meter designed for use in an access tube. Soil Use and Management 20, 203-206.
| Crossref | Google Scholar |
Wu DX, Wang GX, Bai YF, Liao JX (2004) Effects of elevated CO2 concentration on growth, water use, yield and grain quality of wheat under two soil water levels. Agriculture, Ecosystems & Environment 104, 493-507.
| Crossref | Google Scholar |
Xiong D, Chen J, Yu T, Gao W, Ling X, Li Y, Peng S, Huang J (2015) SPAD-based leaf nitrogen estimation is impacted by environmental factors and crop leaf characteristics. Scientific Reports 5, 13389.
| Crossref | Google Scholar |
Yang L, Huang J, Yang H, Dong G, Liu G, Zhu J, Wang Y (2006) Seasonal changes in the effects of free-air CO2 enrichment (FACE) on dry matter production and distribution of rice (Oryza sativa L.). Field Crops Research 98, 12-19.
| Crossref | Google Scholar |
Zadoks JC, Chang TT, Konzak CF (1974) A decimal code for the growth stages of cereals. Weed Research 14, 415-421.
| Crossref | Google Scholar |
Ziska LH (2008) Three-year field evaluation of early and late 20th century spring wheat cultivars to projected increases in atmospheric carbon dioxide. Field Crops Research 108, 54-59.
| Crossref | Google Scholar |
Ziska LH, Weerakoon W, Namuco OS, Pamplona R (1996) The influence of nitrogen on the elevated CO2 response in field-grown rice. Functional Plant Biology 23, 45-52.
| Crossref | Google Scholar |
Ziska LH, Bunce JA, Shimono H, Gealy DR, Baker JT, Newton PCD, Reynolds MP, Jagadish KSV, Zhu C, Howden M, Wilson LT (2012) Food security and climate change: on the potential to adapt global crop production by active selection to rising atmospheric carbon dioxide. Proceedings of the Royal Society B: Biological Sciences 279, 4097-4105.
| Crossref | Google Scholar | PubMed |