Application of elemental chemostratigraphy to refine the stratigraphy of the Adavale Basin, Queensland
David Riley A * , Tim Pearce A , Morven Davidson A , Eva Sirantoine B , Chris Lewis

A Chemostrat Ltd, Welshpool, Powys, UK.
B Chemostrat Australia Pty, Perth, WA, Australia.
C Geoscience Australia, 101 Jerrabomberra Avenue, Symonston, ACT 2609, Australia.
The APPEA Journal 63 207-219 https://doi.org/10.1071/AJ22108
Submitted: 20 December 2022 Accepted: 14 February 2023 Published: 11 May 2023
© 2023 The Author(s) (or their employer(s)). Published by CSIRO Publishing on behalf of APPEA. This is an open access article distributed under the Creative Commons Attribution 4.0 International License (CC BY)
Abstract
A prerequisite to understanding the evolution and resource potential of a basin is to establish a reliable stratigraphic framework that enables the correlation of rock units across multiple depocentres. Establishing a stratigraphic model for the Adavale Basin is challenging due to its structurally complexity, lack of well penetration and its lateral changes in facies. Biostratigraphy appears broad-scale, and despite providing chronostratigraphic control for the Lower Devonian Gumbardo Formation when combined with U/Pb zircon geochronology, the rest of the Devonian succession is hampered by a lack of microfossil assemblages and their poor preservation. The aim of this study is to establish an independent chemostratigraphic correlation across the Adavale Basin using whole rock inorganic geochemistry. Within this study, a total of 1489 cuttings samples from 10 study wells were analysed by Inductively Coupled Plasma – Optical Emission Spectrometry and Inductively Coupled Plasma – Mass Spectrometry for whole rock geochemistry, in order to establish an independent chemostratigraphic zonation scheme. Based on key elemental ratios selected to reflect changes in feldspars, clay minerals and provenance, the Devonian-aged stratigraphy is characterised into four chemostratigraphic mega-sequences that encompass the Gumbardo Formation (Mega-sequence 1); the Eastwood Formation, the Log Creek Formation and the Lissoy Sandstone (Mega-sequence 2); the Bury Limestone and the Boree Salt formations (Mega-sequence 3); and the Etonvale and the Buckabie formations (Mega-sequence 4). These mega-sequences have been further subdivided into a series of chemostratigraphic sequences that can be correlated across the study wells, establishing a regional correlation framework.
Keywords: Adavale Basin, Boree Salt, Buckabie Formation, Bury Limestone, chemostratigraphy, Devonian, Etonvale Formation, Gumbardo Formation, inorganic geochemistry, Log Creek Formation, stratigraphy.
Introduction
A component of the Australian Government’s Data Driven Discoveries program, led by Geoscience Australia, focuses on the Devonian strata of the Adavale Basin, complementing other studies conducted under the Exploring for the Future Program. The Adavale Basin is one of several underexplored Devonian depocentres in central Queensland that developed on the eastern Gondwanan active margin. The basin extends over 60 000 km2, has an estimated total thickness of sediments up to 8.5 km and has been the subject of numerous studies (e.g. Passmore and Sexton 1984; Raymond et al. 2018). However, it has been historically challenging to define a robust stratigraphic model for the basin (Slannis and Netzel 1967; Tanner 1968; Galloway 1970; Auchincloss 1976; Paten 1977; Finlayson et al. 1988; Remus and Tindale 1988; de Boer 1996; Hashemi and Playford 2005; McKillop 2013; Troup and Gorton 2017). Partial well penetrations, structural complexities, a coeval siliciclastic–carbonate system and limited outcropping of Devonian stratigraphy in central Queensland have hampered attempts at stratigraphic correlation. Palynological assemblages are recorded from the Eastwood Formation, Log Creek Formation, Bury Limestone, Lissoy Sandstone and Etonvale Formation (Hashemi and Playford 2005). However, given the limited palaeontological coverage across the wider region, current stratigraphic models are integrated with seismic data to correlate the Adavale Basin to the Darling Basin, New South Wales, and the Drummond Basin/Anakie Basin and Burdekin Basin, Queensland (Draper et al. 2004; Khalifa 2010).
Isotopic dating has been undertaken for the Lower Ordovician volcanics from the Cothalow-1 and Gumbardo-1 wells with Sensitive High Resolution Ion Microprobe dates of 483.6 ± 5.9 Ma, whereas analysis of pyroxenes by K/Ar dating from Gumbardo-1 yielded dates of 489 ± 50 Ma (McKillop et al. 2005, fig. 8). The most recent addition to the stratigraphic model comes from detrital zircon geochronology, which demonstrate significant reworking of Silurian zircons into the base of the Gumbardo Formation and a maximum deposition age for the Eastwood Formation as Eifelian-age. These dates imply that the Eifelian hiatus proposed by McKillop et al. (2005) is not present and that the overlying Log Creek Formation is conformable (Asmussen et al. 2018; Asmussen 2020).
The aim of this study is to establish an independent chemostratigraphic correlation across the Adavale Basin using whole rock inorganic geochemistry from selected well penetrations (Fig. 1). Elemental chemostratigraphy is a correlation technique using whole rock geochemical data. The variations observed within the whole rock geochemistry are inferred back to their mineralogical variations, which are also linked to either change in provenance, climate, depositional environment and diagenesis. Elemental variations linked to changes in provenance and climate have the greatest correlation potential. Chemostratigraphy itself is a relative dating tool and develops a hierarchical zonation scheme like the group, formation and member structure used in lithostratigraphy.
Methods
For this study, 1489 cuttings samples from 10 wells located in the Adavale Basin were analysed by Inductively Coupled Plasma – Optical Emission Spectrometry (ICP-OES) and Inductively Coupled Plasma – Mass Spectrometry (ICP-MS). Eight hundred samples were also analysed for whole rock mineralogy by X-Ray Diffraction (XRD) as summarised in Table 1.
Well | Study interval top (m) | Study interval base (m) | ICP-OES/MS | XRD |
Allandale-1 | 2152 | 3003 | 88 | 57 |
Boree-1 | 1375 | 2673 | 105 | 58 |
Bury-1 | 1588 | 2742 | 126 | 57 |
Collabara-1 | 2252 | 3925 | 156 | 111 |
Etonvale-1 | 1932 | 3456 | 157 | 80 |
Gilmore-1 | 1954 | 4340 | 217 | 100 |
Log Creek-1 | 1923 | 4438 | 221 | 128 |
Quilbury-1 | 1027 | 3039 | 192 | 95 |
Stafford-1 | 1631 | 3136 | 133 | 64 |
Yongala-1 | 2097 | 3104 | 94 | 50 |
Note: Depths are quoted as m Measured Depth (MD).
Nine wells were sampled in May and June 2022 by Geoscience Australia from the Geoscience Australia repository, Canberra. The remaining well, Collabara-1, was jointly sampled by Chemostrat and Geoscience Australia in June 2022 at the Exploration Data Centre in Brisbane (Approval No. 27284). To support this study, lithostratigraphic tops were provided by Geoscience Australia. Wireline log data and well completion reports were downloaded from the Geological Survey of Queensland Open Data Portal (Queensland Government 2022).
ICP sample preparation and analysis
Cuttings were prepared by sieving the whole sample (sieves used 1 mm and 500 µm), with the 500 µm fraction put forward for hand grinding and ICP analysis. Before grinding, a magnet was run over the sample to remove any loose metal shavings. Cuttings were ground by hand to a powder in an agate pestle and mortar, with the sample powders prepared for ICP-OES/MS analyses using the lithium metaborate (alkali) fusion procedure (Jarvis and Jarvis 1992a, 1992b).
Following the fusion process, the samples were analysed on a Thermo ICAP 7200 ICP-OES and a Thermo ICAP RQICPMS ICP-MS to obtain data for 50 elements, including major, trace and rare earth elements (REEs).
The precision of the geochemical data acquired by the ICP-OES/MS analyses were determined by replicate analyses of certified rock ‘standard reference materials’ (SRMs – samples of a known chemical composition) together with the analyses of the cuttings samples from the study wells. The closer the comparison between the data obtained from the SRMs across the entire analytical run and their known element concentrations, the better the precision of the inorganic geochemical data. Any deviations from the known concentrations of the SRMs can be readily identified in the dataset and corrected. Major elements were reported as weight percent oxide with trace and REEs reported parts per million (ppm).
XRD sample preparation and analysis
The sample was first disaggregated gently using a pestle and mortar. A 2 g split of this material was then ‘microionised’ using a McCrone Microionising Mill to obtain an XRD ‘powder’ with a mean particle diameter between 5 and 10 µm. The sample was dried overnight at 80°C, re-crushed to a fine powder and back-packed into an aluminium (Al) cavity mount, producing a randomly orientated sample for presentation to the X-ray beam.
Samples were subsequently analysed on the PANalytical X’pert3 diffractometer instrument with a copper anode tube. Samples are analysed between 4.5° and 75° 2θ (theta) at a step size of 0.013 and nominal time per step of 0.2 s (continuous scanning mode) using X-ray radiation from a copper anode at 40 kV, 40 mA.
Identification of unknown minerals was achieved by using X-Ray Mineral Services ‘Traces’ and ‘Search-Match’ software to compare the XRD pattern from the unknown sample with the International Centre for Diffraction Data PDF-4 Minerals database, which contains reference patterns for more than 157 000 phases.
The techniques are an implementation of Rietveld analysis (Post and Bish 1989; Bish and Post 1993), which was used first to understand and refine crystal structure as determined by XRD. Since the 1980s, the method has been extended to quantification of phases in mineral samples. The Rietveld equation allows a synthetic diffractogram to be calculated for a mineral mixture. This takes account of material properties and practical difficulties, such as dispersion, absorption, particle size and preferred orientation. Results obtained from Autoquan software can be regarded as quantitative.
Results and discussions
Key ratios
Of the 50 elements quantified using ICP, a decision was required as to which of these elements will be most useful in order to geochemically characterise the stratigraphy and achieve the study aims. It is also best practice to link the elemental data back to a mineralogical affinity in order to aid interpretation. Elements such as calcium (Ca) may have limited correlation potential and are associated with calcite cement phases. Elements associated with heavy minerals and provenance, such as zirconium (Zr), niobium (Nb), and elements associated with clay minerals, such as gallium (Ga), rubidium (Rb) and caesium (Cs), have significantly greater correlation potential. Determining key elemental-mineral affinities is either done using statistical testing or with direct comparison to a mineralogical dataset generated on identical samples. Both techniques are utilised for this study.
Principal component analysis (PCA) can highlight recurrent element associations within a dataset, which in turn can contribute towards determining the mineral affinities. PCA has previously been utilised in many chemostratigraphic studies (e.g. Pearce et al. 1999, 2005) as a cluster analysis tool, but as a large robust quantitative mineralogy dataset has been obtained as part of this study, it is the outcomes of the XRD data, and its comparison to the geochemical data, that underpin the element-mineral affinities in this study.
A summary of selected XRD data is illustrated within Fig. 2. Halite is predominantly associated with the penetrations of the Boree Salt; although carbonates (calcite and dolomite) are associated with the Bury Limestone and the Cooladdie Dolomite, higher carbonate is also noted within the siliciclastic intervals of Gilmore-1 and Quilberry-1, reflecting the inferred occurrence of carbonate cement phases. Potassium (K)-feldspar and quartz are noted within most siliciclastic prone intervals. Plagioclase is recorded within the Gumbardo Formation (e.g. Allandale-1, Fig. 2) and within the Etonvale Formation (e.g. Gilmore-1, Fig. 2).
XRD data collected over some of the study wells demonstrating the feldspar variation within the Gumbardo Formation, the carbonates of the Bury Limestone and the halite of the Boree Salt.
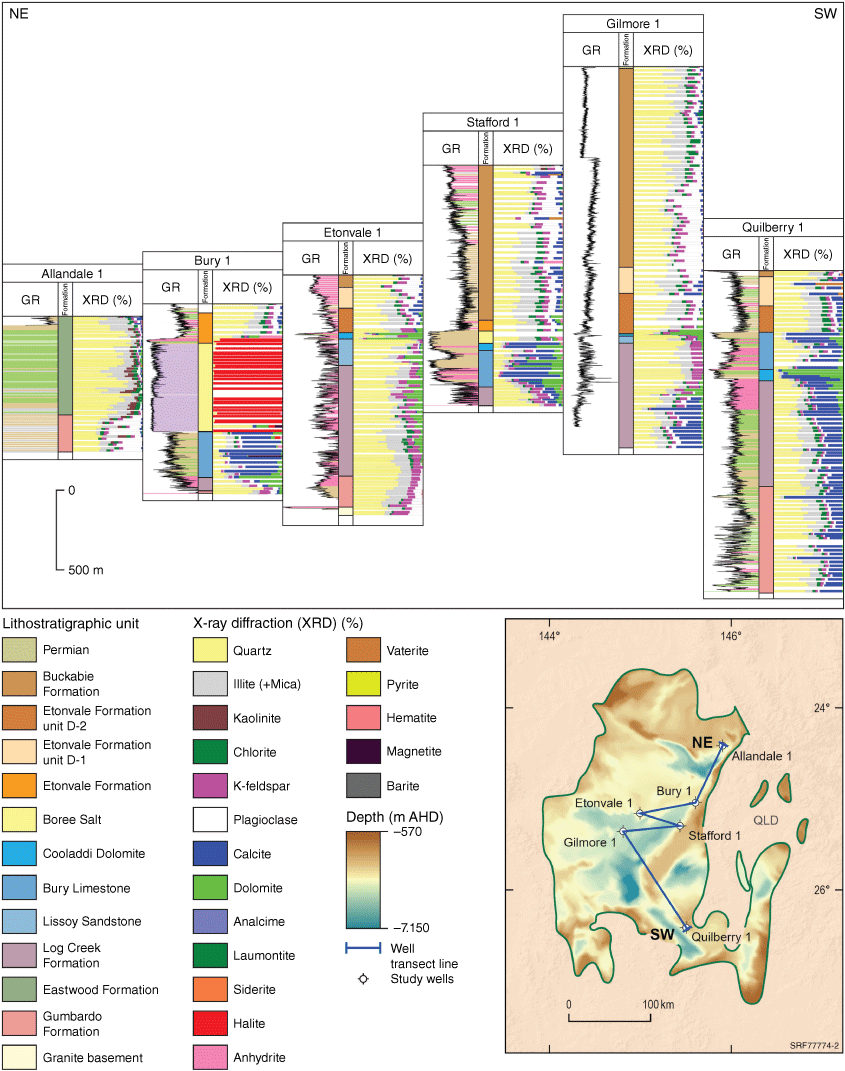
As XRD and ICP data represent a whole rock analysis, direct comparison between them demonstrates typical trends; for example, quartz and silica (Si) are related. There is also an association with other framework minerals and elements,K, which is associated with K-feldspar and sodium (Na), which has a dual affinity and is associated with both halite and plagioclase feldspar. Typically, chlorine (Cl) data is used to distinguish whether Na is associated with halite or plagioclase feldspar, but Cl abundance is not quantified by ICP analysis. Alternatively, a Na/Al value of five is used as a cut off, with values higher than five typically associated with halite occurrence and values lower than five associated with Na-felspar. Clay minerals are concentrated in the finer-grained sediments and are associated with elements such as Al, Ga, Rb and Cs. Carbonate intervals are associated with calcite and dolomite as well as associated elements, such as Ca, magnesium (Mg) and manganese (Mn).
Using the element-mineral affinities determined using the PCA and the XRD data, the key elements and ratios for this study are identified and their geological significance suggested (see Table 2).
Element/ratio | Geological control | Geological interpretation |
Al/base | Weathering | The ratio of Al/bases reflects the accumulation of insoluble materials (clay) with respect to soluble materials (Ca, Mg, Na and K), which are liberated by hydrolytic weathering (Retallack 1997) |
Ca and Mg | Carbonate | Ca is associated with carbonate, whereas Mg is predominantly associated with dolomite. |
Cs/Th | Clay minerals/HM | Cs is predominantly associated with marine smectite, whereas Th can be associated with clay minerals but can also be substituted into heavy minerals, such as zircon or phosphates. High ratio values possibly indicate flooding events with abundant smectite. Lower values reflect changes in clay composition but may reflect the occurrence of heavy mineral rich sediments, such as silt and fine sand. |
Fe/Mg | Clay minerals/carbonate | Fe is associated with haematite and clay minerals, predominantly chlorite, whereas Mg is associated with dolomite. Fe may show some association with the dolomites and other carbonates. |
Fe/Mn | Clay minerals/carbonate | Fe is associated with haematite and clay minerals, predominantly chlorite. Mn is associated with carbonate minerals. Fe may show some association with the dolomites and other carbonates. |
Ga/Rb | Clay minerals | Ga is associated with kaolinite, whereas Rb is predominantly associated with illite. This ratio therefore models the relationship between kaolinite and illite. Kaolinite is typically the result of subaerial exposure with enhanced geochemical weathering; as such, this ratio may act as a proxy for changes in climate and/or water depth. |
K/Al | K-feldspar | K is associated with the occurrence of K-feldspar and illite. High K/Al values (>0.35) in conjunction with high K/Rb values are indicative of K-feldspar abundance. |
K/Rb | K-feldspar/illite | K and Rb are both associated with K-feldspar and illite, with the latter being preferentially enriched in Rb. High values are indicative of the K being present in K-feldspar, whereas lower values demonstrate relative illite abundance. |
Na/Al | Feldspar variation/halite | The Na/Al ratio is typically used to represent the relative abundance of plagioclase feldspar (Na) within the section; however, within salt prone intervals, this ratio will represent the abundance of halite. Na/Al can be plotted in the same track as K/Al to highlight relative abundance of plagioclase feldspar (Na) to K-feldspar (K). |
Nb/Th | Provenance | Nb and Th are both associated with heavy minerals by substituting into the crystal lattice of Ti-oxides and zircons, or phosphatic heavy minerals, respectively. High values are indicative of abundant Ti-oxide heavy minerals, whereas low values are indicative zircons. |
Si/Al | Geological process | Si is associated to total quartz, whereas Al is associated to clay minerals. Higher values are indicative of coarse-grained sediment, whereas lower values indicate fine-grained sediment. In addition, this ratio reflects mineralogical maturity of the sediment, and it is used to differentiate between clastic geochemical lithotypes. |
Th/Sc | Compositional variation | The compositional variation ratio was proposed by McLennan et al. (1993). Th is indicative of a felsic, evolved source, whereas Sc is associated with a mafic, primitive source. High values are indicative of a felsic source, whereas lower values reflect a primitive source. |
Zr/Sc | Geological process | The sediment reworking ratio was proposed by McLennan et al. (1993). Reworked sediments are enriched in Zr, reflecting concentrations of the heavy mineral zircon, whereas Sc is associated with clay minerals and is winnowed out during the reworking. |
Chemostratigraphic scheme
Chemostratigraphic nomenclature is hierarchical, similar to the lithostratigraphic hierarchy, group, formation and member. For this study, the hierarchy established is chemostratigraphic mega-sequence, sequence and package (Figs 3–5). Across the Adavale Basin, mega-sequences have the highest correlation potential and reflect regional-scale geochemical changes. However, the chemostratigraphic packages have less correlation potential and are characterised within one or more closely spaced wells. As chemostratigraphic packages are limited to specific areas, they do not help achieve the key aims of this study, and as such, they will not be discussed further.
A total of four chemostratigraphic mega-sequences are recognised within the study wells, summarised within Table 3. A fifth mega-sequence (MS5) is also defined but corresponds to overlying younger (?Permian) strata outside the scope of this study and will not be discussed further.
Chemostratigraphic mega-sequence | Northern study well | Central study wells | Western study well | Southern well |
Mega-sequence 4 | Higher Cs/Th, Rb (ppm), K (%) combined with lower Zr/Sc, Th/Sc | Higher Al/Base, K (%), Rb (ppm), Fe/Mg combined with lower Mg (%), Ca (%), K/Rb | Higher Al/Base, Fe/Mg, combined with lower Ca (%) | Higher Al/Base, K (%), Rb (ppm), Fe/Mg, combined with lower Ca (%), Mg (%) |
Mega-sequence 3 | – | Higher Ca (%), Mg (%), K/Rb, Nb/Th, K/Al, Na/Al combined with lower Al/Base, K (%), Rb (ppm) | Higher Ca (%), Zr/Sc, Cs/Th combined with lower Fe/Mn, Al/Base | Higher Ca (%), Mg (%) combined with lower Al/Base, K (%) Rb (ppm) |
Mega-sequence 2 | Higher Al/Base, Zr/Sc, Th/Sc, Fe/Mg, combined with lower K (%), Rb (ppm) | Higher K (%), Rb (ppm), combined with lower Ca (%), Mg (%), Ga/Rb, Cs/Th, Fe/Mn | – | Higher Zr/Sc, K (%), Rb (ppm), Al/Base, combined with lower Ca (%), Mg (%), Cs/Th |
Mega-sequence 1 | Higher Na/Al, Ga/Rb, Nb/Th, combined with lower Al/Base, U (ppm) | Higher Na/Al, Fe/Mg, Zr/Sc, Th/Sc, K, combined with lower U (ppm) | Higher Na/Al. Fe/Mg, Al/Base, combined with lower Zr/Sc, Th/Sc, Cs/Th | Higher Zr/Sc, Al/Base, U (ppm), Ca (%), combined with lower Nb/Th, |
U; uranium.
MS1 is correlated across the Adavale Basin; however, the distribution of the chemostratigraphic sequences suggests the intervals may not be directly correlatable across the entire basin. MS1 is predominantly characterised by higher Na values, which is associated with plagioclase occurrence, and higher Ga/Rb values combined with lower Fe/Mg and Si/Al values, in comparison with the overlying mega-sequence. Lateral variation within MS1 is illustrated by the variation between the wells Allandale-1, which is high in Na, and Etonvale-1, which is characterised by higher K (%) (Table 3). This reflects an abundance K-feldspar. In contrast, the higher Ca values within Quilberry-1 well reflect higher calcite present within the study well, suggesting perhaps localised carbonate cementation. The absence of MS1 within the Stafford-1, Collabarra-1, Gilmore-1 and Log Creek-1 wells is attributed to limited penetration of MS1 due to the total depth of the well. The Etonvale-1 well penetrates directly into granite basement (Lewis and Kyranis 1962). MS1 is subdivided into three chemostratigraphic sequences, which are coeval to the different subdivisions of the Gumbardo Formation.
Chemostratigraphic sequence MS1.S5, represents the lowest part of the Gumbardo Formation and is only identified within the southern well, Quilberry-1 (Fig. 5). The absence of MS1.S5 within the other wells could be attributed to the lack of sufficient well penetration. The higher Si/Al and Zr/Sc values suggest the interval hosts well-sorted, recycled sediment. Lower Si/Zr values over this interval suggest the sands are relatively fine-grained. Higher Ca values have been demonstrated to associate with high calcite abundance – possibly relating to diagenetic cement phases. Chemostratigraphic sequence MS1.S10 is recognised within the Boree-1, Bury-1, Etonvale-1 and Quilbury-1 wells. Relative to the overlying sequence, MS1.S10 is characterised by higher K and lower Na values, which reflect a change in feldspar populations from a K-feldspar to a plagioclase feldspar, as seen in the XRD data (Fig. 2). MS1.S20 is the uppermost sequence within MS1 and is equivalent to the upper part of the Gumbardo Formation. Recognised within Allandale-1, Boree-1, Bury-1 and Yongala-1 wells, high Na/Al values define this sequence and are a reflection in the increased abundance of plagioclase, as demonstrated by the XRD data (Fig. 2).
MS2 is broadly equivalent to the Eastwood Formation, the Log Creek Formation and the Lissoy Sandstone. Within the Gilmore-1 and Log Creek-1 wells, the lowest boundary of this mega-sequence is defined by the base of the sampling interval. MS2 is absent from the Yongala-1 well, either due to non-deposition or subsequent erosion. Relative to MS3, MS2 has higher K, Rb and Zr/Sc values, combined with lower Cs/Thorium (Th), Ca and Mg. MS2 is recognised as broadly equivalent to the Log Creek and the Lissoy Sandstone within the central, western and southern study wells. MS2 has been subdivided into six chemostratigraphic sequences.
The lowest most chemostratigraphic sequence, MS2.S10 within MS2, is broadly equivalent to the basal part of the Log Creek Formation within most of the wells, but it also corresponds to the lower Eastwood Formation within the Allandale-1 well. MS2.S10 is characterised by high Fe/Mn, Fe/Mg and Al/Base values (which are indicative of a subaerially exposed surface or a paleosol that has experienced enhanced chemical weathering), combined with lower Th/Scandium (Sc) and Zr/Sc values than in the overlying sequence, suggesting the sediments were deposited shortly after being weathered, with an inferred mafic component.
Sequence MS2.S20 is characterised by higher Si values and lower Mg and Sc values than the overlying Sequence MS2.S30, which infers that Sequence MS2.S20 is made up of a generally coarser-grained sediment, with a lower abundance of clay minerals. The sequence is thickest within the Quilberry-1 and Etonvale-1 wells (366 and 354 m, respectively) and is generally coeval to the Log Creek Formation. The exception is in the Allandale-1 well, where it is associated with the Eastwood Formation. Sequence MS2.S30 is characterised by higher Mg values and lower Si values relative to Sequence MS2.S40 and this reflects an increase in the abundance of dolomite.
Sequence MS2.S40 is recognised within the northernmost well, Allandale-1; the central wells, Etonvale-1, Gilmore-1 and Log Creek-1; and the southern well, Quilberry-1. Lithostratigraphically, it is approximately equivalent to the Log Creek Formation/Lissoy Sandstone, except for the northern well, Allandale-1, in which the chemostratigraphic sequence is associated with the Eastwood Formation. This sequence is geochemically differentiated from the overlying MS2.S50 by higher Ca, Mn and iron (Fe) values, reflecting an increase in the occurrence of carbonate minerals. Lower Th/Sc and Rb values compared to the overlying MS2.S50 also inferr an increase in the occurrence of clays with mafic affinities.
Sequence MS2.S50 is recognised within most of the central well cluster and is the only MS2 sequence recognised within the wells Bury-1, Boree-1 and Stafford-1. The sequence is absent from the northern, western and southern study wells. Geochemically, MS2.S50 is distinguished from the overlying Sequence MS2.S60 by higher titanium (Ti) and Fe abundances, in addition to lower Th/Sc and Rb values. Higher Fe and Ti combined with lower Th/Sc is typically indicative of a mafic component to the sediment.
Sequence MS2.S60 is the uppermost sequence within MS2 and is only recognised within the central study wells Collabara-1, Gilmore-1 and Log Creek-1. It is distinguished from the underlying MS2.S50 by higher Th/Sc and Rb values, combined with lower Ti and Fe values, suggesting that these rocks are compositionally more mature than the Sequence MS2.S50 sediments.
MS3 is broadly comparable to the Bury Limestone, Cooladdi Dolomite and Boree Salt. The thickest occurrences of MS3 are observed within the Boree-1, Bury-1 and Stafford-1 wells. In these locations, sample gaps are associated with the occurrence of the Boree Salt due the intervals having been cored. Thinner occurrences are recognised within the other study wells and are often associated with the previously recognised occurrence of the Cooladdi Dolomite. MS3 is absent from the northern most study well, Allandale-1. MS3 is predominantly characterised by the occurrence of the high Mg and Ca as well as lower Al/Base, K and Rb values.
Sequence MS3.S10 is recognised as generally equivalent to the Bury Limestone (e.g. within Stafford-1), but it is also recognised as equivalent to the Cooladdi Dolomite (Boree-1). Geochemically, MS3.S10 is distinguished from MS3.S20 by its higher Si/Al and K/Rb values, combined with lower Zr/Sc and Al/Base values. This sequence represents the switching from a clastic system to a carbonate system as the higher Si/Al and K/Rb values suggest the basin received detrital siliciclastic input.
Sequence MS3.S20 is lithostratigraphically equivalent to the Bury Limestone and the Cooladdi Dolomite and is recognised within wells Etonvale-1, Collabara-1, Gilmore-1, Log Creek-1, Stafford-1 and Quilberry-1. The thickest occurrences of this sequence are hosted within the Stafford-1 and Bury-1 wells.
Geochemically, MS3.S20 is distinguished from the overlying sequences by higher Ca values with lower Nb/Th and Fe/Mn values, which reflect the abundance of carbonate and a relative depletion of clastic (clay) minerals present. Alternating high and low Ca values (e.g. the Stafford-1 well) likely reflects interfingering as the result of an intermittent clastic sediment source. High Mg intervals within some horizons are indicative of dolomite abundance, possibly reflecting the Cooladdi Dolomite or just Mg rich calcite layers.
Sequence MS3.S30 is recognised within the Boree-1 and Bury-1 wells. This chemostratigraphic sequence is equivalent to the Boree Salt, which is predominantly halite (Paterson et al. 2022). It should be noted that a large sample gap within the Boree-1 well makes this correlation somewhat tentative. Furthermore, Paterson et al. (2022) noted that the salts within the Boree-1 and Bury-1 wells represent two different seismically defined salt bodies, and so, the correlation here is likely driven by the rocks similar lithology, rather than reflecting chemical changes, which define it as unique and suggest continuity. The predominant geochemical characteristic of Sequence MS3.S30 is the high Na/Al values reflecting the occurrence of the halite. Clastic driven geochemistry includes higher K/Rb and Nb/Th, combined with lower Al/Base values, compared to the overlying Sequence MS3.S40. Geochemically, it may be possible to distinguish between the salts identified by Paterson et al. (2022), using an analysis of the additional section by X-ray Fluorescence (XRF), to analyse elements such as Cl and bromine, which have been used for the characterisation of evaporites. However, this methodology does not always work, as the trace elements in salts often account for only a few ppm, which may be close to the limit of detection on an XRF spectrometer, especially when the salts are relatively ‘pure’ with few impurities.
Sequence MS3.S40 is the uppermost sequence assigned within MS3 and is only identified in the eastern central wells (Boree-1, Bury-1 and Stafford-1). MS3.S40 is approximately the lithostratigraphic equivalent to the Boree Salt in the Boree-1 and Stafford-1 wells and in the Etonvale Formation in the Bury-1 well. It can be geochemically differentiated from the underlying Sequence MS3.S30 sequence by higher Ca and Mg values (indicative of a carbonate sequence) combined with a marked drop in Na and K/Rb values.
MS4 is broadly equivalent to the Etonvale and Buckabie formations. Relative to the MS3, this mega-sequence is characterised by generally higher K, Rb and Al/Base values, combined with lower Ca and Mg values. This mega-sequence is generally assigned at the top of the study interval, and so, the upper boundary is rarely chemically defined. Thicker penetrations of MS4 are recognised within the Collabara-1, Gilmore-1 and Log Creek-1 wells. Generally, this mega-sequence is regarded as the most correlatable between the study wells, with an apparent ‘layer-cake’ correlation.
Sequence MS4.S10 is equivalent to the Etonvale Formation in wells Boree-1 and Bury-1, whereas in Allandale-1, it is equivalent to the top of the Eastwood Formation, the bulk of which has been assigned to MS2 (see previous).
Geochemically, Sequence MS4.S10 is distinguished from the overlying MS4.S20 by higher K/Rb and Si/Al values, combined with lower Cs/Th and Al/Bases values.
Sequence MS4.S20 is recognised within all the study wells, except for Allandale-1, and this is attributed to the upper sampling limit within the well. Sequence MS4.S20 appears thicker within the Boree-1 well, but the upper boundary is defined by the sampling limits and is not geochemically defined. The sequence appears generally thicker within the central well cluster and thins to the south into Quilberry-1. Geochemically, MS4.S20 is characterised by higher Zr/Sc and Fe/Mg values combined with lower Cs/Th and Ga/Rb values than those in the overlying sequence, MS4.S30. MS4.S20 appears to thicken from Stafford-1 to the central wells (e.g. Gilmore-1) and thicken to the south in the Quilberry-1 well.
Geochemically, MS4.S30 is characterised by higher Cs/Th and K/Al values, combined with lower Fe/Mg and Ga/Rb values than those in the overlying MS4.S40.
Sequence MS4.S40 is coeval to the Buckabie Formation and is present in Yongala-1, Quilberry-1 and all the wells in the central area. The thickest occurrence of Sequence MS4.S40 is in Log Creek-1, where it is assumed there was greater accommodation space. It is geochemically distinguished by higher Na/Al and Ga/Rb values and lower Cs/Th and Al/Base values than those in Sequence MS4.S50.
Sequence MS4.S50 is present in all the central wells, except for Etonvale-1, with thicknesses ranging from 313 m in Log Creek-1 to 404 m in Gilmore-1. The sequence then thins to the west into the Yongala-1 well. MS4.S50 is the uppermost MS4 sequence assigned within the interval studied within wells Stafford-1 and Yongala-1. Geochemically, MS4.S50 is characterised by higher Cs/Th and Ga/Rb values and lower Th/Sc and Nb/Th relative to the overlying MS4.S60.
Sequence MS4.S60 has been identified in three of the central wells: Collabara-1, Gilmore-1 and Log Creek-1, with sequence thicknesses ranging from 280 to 370 m. MS4.S60 is distinguishable from the overlying MS4.S70 by a marked increase in Th/Sc and Zr/Sc. Both ratios are used by McLennan et al. (1993) to show compositional variation and sediment reworking and suggest that the sediment assigned to Sequence MS4.S60 has been extensively reworked. Higher Th/Sc values, as seen in MS4.S60, likely indicate a more felsic rather than mafic source material, whereas higher Zr/Sc values indicate a greater degree of sediment reworking.
Sequence MS4.S70 is the uppermost MS4 sequence and is only present in the Gilmore-1 and Collabara-1 wells, and this is likely to do with the geographic position of the wells within the centre of the basin where there is more accommodation space. Sequence MS4.S70 is defined by higher Cs/Th and Nb/Th values combined with lower Fe/Mn and Th/Sc values in comparison to the underlying Sequence MS4.S60.
Conclusion
This study demonstrates the application of elemental chemostratigraphy to the Devonian-aged stratigraphy of the Adavale Basin and presents a correlation scheme established on the selected well penetrations.
The Devonian-aged stratigraphy is subdivided based on changes in clay mineralogy, feldspar compositions and mineralogical composition associated with shifts in provenance. Four chemostratigraphic mega-sequences are defined, which correspond to the Early Devonian Gumbardo Formation (MS1); Middle Devonian Eastwood Formation, Log Creek Formation and Lissoy Sandstone (MS2); Middle Devonian Bury Limestone, Boree Salt Formation and Cooladdi Dolomite (MS3); and the Late Devonian Etonvale and Buckabie Formations (MS4). These mega-sequences have then been further subdivided into a series of chemostratigraphic sequences that are correlated across the study wells, establishing a regional correlation framework across the Adavale Basin. Following this application of chemostratigraphy to the Adavale Basin, the suggested correlation now requires additional testing against other datasets to validate the interpretation.
Acknowledgements
C. Lewis and C. Wainman publish with the permission of the CEO, Geoscience Australia. D. Riley, M. Davidson, T. Pearce and E. Sirantoine publish with the permission of Geoscience Australia and with the support of Chemostrat Ltd directors. We acknowledge the Geoscience Australia staff who conducted the sampling and the analytical expertise of the Chemostrat laboratory staff. The authors acknowledge the reviews and comments of M. Bouma, D. Edwards of Geoscience Australia and C. Tansell of Chemostrat for internally reviewing and commenting on the manuscript.
References
Asmussen P (2020) Insights from the Devonian Adavale Basin on the Tectonic History of the Thomson Orogen. PhD thesis, Queensland University of Technology, Brisbane, Australia.Asmussen P, Bryan SE, Allen CM, Purdy DJ (2018) Geochronology and geochemistry of the Devonian Gumbardo Formation (Adavale Basin): evidence for cratonisation of the central Thomson Orogen by the Early Devonian. Australian Journal of Earth Sciences 65, 1133–1159.
| Geochronology and geochemistry of the Devonian Gumbardo Formation (Adavale Basin): evidence for cratonisation of the central Thomson Orogen by the Early Devonian.Crossref | GoogleScholarGoogle Scholar |
Auchincloss G (1976) Adavale Basin. In ‘Economic Geology of Australia and Papua New Guinea. Vol. 3’. Petroleum. (Eds CL Knight, RB Leslie, HJ Evans) pp. 309–315. (Australia Institute of Mining and Metallurgy)
Bish DL, Post JE (1993) Quantitative mineralogical analysis using the Rietveld full-pattern fitting method. American Mineralogist 78, 932–940.
de Boer RA (1996) The integrated development of Gilmore Field and an independent power plant. The APPEA Journal 36, 117–129.
| The integrated development of Gilmore Field and an independent power plant.Crossref | GoogleScholarGoogle Scholar |
Draper JJ, Boreham CJ, Hoffman KL, McKellar JL (2004) Devonian Petroleum Systems in Queensland. In ‘PESA’s Eastern Australasian Basins Symposium II’, 19–22 September 2004 conference proceedings, Adelaide. (Eds PJ Boult, DR Johns, SC Lang) pp. 297–318. (PESA: Perth, WA)
Finlayson DM, Leven JH, Etheridge MA (1988) Structural styles and basin evolution in Eromanga region, eastern Australia. The American Association of Petroleum Geologists, Bulletin 72, 33–48.
| Structural styles and basin evolution in Eromanga region, eastern Australia.Crossref | GoogleScholarGoogle Scholar |
Galloway M (1970) ‘Adavale, Queensland. 1:250 000 geological map series explanatory notes, SG 55-05.’ (Bureau of Mineral Resources: Canberra, Australia)
Hashemi H, Playford G (2005) Devonian spore assemblages of the Adavale Basin, Queensland (Australia): descriptive systematics and stratigraphic significance. Revista Española de Micropaleontologia, 37, 317–417.
Jarvis I, Jarvis KE (1992a) Inductively coupled plasma-atomic emission spectrometry in exploration geochemistry. Journal of Geochemical Exploration 44, 139–200.
| Inductively coupled plasma-atomic emission spectrometry in exploration geochemistry.Crossref | GoogleScholarGoogle Scholar |
Jarvis I, Jarvis KE (1992b) Plasma spectrometry in the earth sciences: techniques, applications and future trends. Chemical Geology 95, 1–33.
| Plasma spectrometry in the earth sciences: techniques, applications and future trends.Crossref | GoogleScholarGoogle Scholar |
Khalifa M (2010) Correlation of the Devonian Formations in the Blantyre Sub-basin, New South Wales with the Adavale Basin, Queensland. Journal and Proceedings of the Royal Society of New South Wales 143, 19–33.
Lewis JH, Kyranis N (1962) Phillips – Sunray Etonvale No. 1 Authority to Prospect 84P, Queensland, Well Completion Report. (Phillips Petroleum Company: Brisbane)
McKillop MD (2013) Adavale Basin. In ‘Geology of Queensland’. (Ed. PA Jell) pp. 175–183. (Geological Survey of Queensland: Brisbane)
McKillop MD, McKellar JL, Draper JJ, Hoffmann KL (2005) The Adavale Basin: stratigraphy and depositional environments. In ‘Proceedings of the Central Australian Basins Symposium’, 16–18 August 2005, Alice Springs. (Eds TJ Munson, GJ Ambrose) pp. 82–107. (Northern Territory Geological Survey: Darwin, NT)
McLennan SM, Hemming S, McDaniel DK, Hanson GN (1993) Geochemical approaches to sedimentation, provenance, and tectonics. In ‘Processes controlling the composition of clastic sediment’. (Eds MJ Johnsson, A Basu) pp. 21–40. (Geological Society of America Special Paper, 284)
Passmore VL, Sexton MJ (1984) The structural development and hydrocarbon potential of Palaeozoic source rocks in the Adavale Basin region. The APPEA Journal 24, 393–411.
| The structural development and hydrocarbon potential of Palaeozoic source rocks in the Adavale Basin region.Crossref | GoogleScholarGoogle Scholar |
Paten RJ (1977) The Adavale Basin, Queensland. In ‘Petroleum in Queensland - a stocktake for the future.’ (The Petroleum Exploration Society of Australia, Queensland Branch, Queensland Division: Brisbane)
Paterson R, Feitz AJ, Wang L, Rees S, Keetley J (2022) From A preliminary 3D model of the Boree Salt in the Adavale Basin, Queensland. In ‘Exploring for the Future: Extended Abstracts’. (Ed. Czarnota K) (Geoscience Australia: Canberra)
| Crossref |
Pearce TJ, Besly BM, Wray DS, Wright DK (1999) Chemostratigraphy: a method to improve interwell correlation in barren sequences — a case study using onshore Duckmantian/Stephanian sequences (West Midlands, U.K.). Sedimentary Geology 124, 197–220.
| Chemostratigraphy: a method to improve interwell correlation in barren sequences — a case study using onshore Duckmantian/Stephanian sequences (West Midlands, U.K.).Crossref | GoogleScholarGoogle Scholar |
Pearce T, Wray D, Ratcliffe K, Wright D (2005) Chemostratigraphy of the Schooner Formation, Southern North Sea. Proceedings of the Yorkshire Geological Society, Occasional Publication 7, 147–164.
Post JE, Bish DL (1989). Rietveld refinement of crystal structures using powder X-ray diffraction Data. In ‘Modern Powder Diffraction. Vol. 20’. (Eds DL Bish, JE Post) pp. 277–308. (De Gruyter)
Queensland Government (2022) GSQ Open Data Portal. Available at https://geoscience.data.qld.gov.au/ [viewed 19 May 2022]
Raymond OL, Totterdell JM, Stewart AJ, Woods MA (2018) ‘ Australian Geological Provinces’, 2018.01 edn. (Geoscience Australia: Canberra) Available at http://pid.geoscience.gov.au/dataset/ga/116823 [viewed 9 December 2022]
Remus D, Tindale K (1988) The Pleasant Creek Arch, Adavale Basin, a mid-Devonian to mid-Carboniferous thrust system. The APPEA Journal 28, 208–216.
| The Pleasant Creek Arch, Adavale Basin, a mid-Devonian to mid-Carboniferous thrust system.Crossref | GoogleScholarGoogle Scholar |
Retallack GJ (1997) ‘Colour guide to paleosols.’ (John Wiley & Sons Ltd: Chichester)
Slannis A, Netzel R (1967) ‘Geological Review of ATP 109P and 125P, Queensland, Australia.’ (Department of Natural Resources and Mines: Queensland)
Tanner JJ (1968) Devonian of the Adavale Basin, Queensland, Australia. In ‘International Symposium on the Devonian System’, Proceedings, 1967, Calgary. (Ed DH Oswald) pp. 82–107. (Alberta Society of Petroleum Geologists: Calgary)
Troup A, Gorton J (2017) Analysis and characterisation of petroleum source rocks in Queensland. The APPEA Journal 57, 806–809.
| Analysis and characterisation of petroleum source rocks in Queensland.Crossref | GoogleScholarGoogle Scholar |
![]() David Riley completed a MGeol and PhD from the University of Leicester, UK, and joined Chemostrat Ltd as a Chemostratigrapher in October 2012. Since joining Chemostrat Ltd, he has worked on projects from eastern Canada, UK North Sea, Middle East, Southeast Asia and Australia. In 2022, he became Chemostrat Ltd Stratigraphy Manager, providing technical oversight on all chemostratigraphic studies. He is also a Fellow of the Geological Society of London. |
![]() Tim Pearce completed his BSc from the University of Liverpool and his PhD from the Kingston University. Shortly after founding the company Chemostat Ltd., Chemostrat Ltd later became Hafren Scientific, a group of companies developing innovative technologies in geological sources primarily focused on the energy sector. Tim is currently the CEO of the Hafren group, including Chemostrat Australia Pty. Ltd. He is a Fellow of the Geological Society of London and a member of the Petroleum Exploration Society of Great Britain and the American Association of Petroleum Geologists. |
![]() Morven Davidson graduated with a MESci Geology in 2019 from the University of Liverpool. Her master’s research project focussed on the tectonic history and microstructural study of peridotites from NW Italy. She initially worked for Chemostrat Ltd as a Junior Geotech, conducting a range of laboratory analysis, including benchtop X-ray fluorescence (XRF), handheld XRF and magnetic susceptibility to support the work of the geological team. Following this, she spent 1 year working as a Mudlogger in the North Sea (UK) before re-joining Chemostrat as a Junior Geologist and has worked on projects from Australia, Middle East and UK North Sea. |
![]() Eva Sirantoine graduated with a Bachelor of Science, majoring in Earth Sciences, from Ecole Normale Superieure (Lyon) in 2013 and went on to complete a Master of Science, majoring in Earth Sciences in 2015 from Ecole Normale Superieure, specialising in Palaeontology, Sedimentology and Palaeoenvironments. In 2016, Eva completed a Master of Education, Biology and Geology from Ecole Normale Superieure, Lyon, and went on to teach secondary school biology and geology at Lycee Saint Exupery. From 2017 to 2022, Eva completed her PhD at The University of Western Australia. In May 2022, Eva joined Chemostrat Australia Pty. Ltd as a Geologist. Eva is a Committee Member of the Geological Society of Australia, WA branch, Equity and Diversity chair. She is a member of the Petroleum Exploration Society of Australia. |
![]() Chris Lewis holds a BSc from the University of Adelaide. Since joining Geoscience Australia in 2010, Chris has held numerous positions across the scientific and professional areas of the organisation. Chris is the current Director of Regional Geology and Drilling at Geoscience Australia. His technical background is on the use of geochronology and isotope geochemistry to aid in understanding the paleogeographic and stratigraphic evolution of the Earth. Chris has undertaken studies across most Australian states and territories as well as within the offshore areas of Australia’s exclusive economic zone. |
![]() Carmine Wainman holds a MSci in Geology from the University of Southampton and a PhD in Geosciences from the University of Adelaide. His professional expertise includes sedimentology, stratigraphy, palynology and basin analysis. He currently works at Geoscience Australia as a Basin Analyst in the Minerals, Energy and Groundwater Division. Carmine has over 9 years of industry and research experience both in Australia and the UK, including with the RSK Group, Woodside Energy and the University of Adelaide. Carmine also participated on the International Ocean Discovery Program Expedition 369 in late 2017, investigating Australian Cretaceous climate and tectonics, and he is a Fellow of the Geological Society of London. |